- College of Veterinary Medicine, South China Agricultural University, Guangzhou, China
Rabies, caused by rabies virus (RABV), is a zoonotic disease infecting mammals including humans. Studies have confirmed that glycoprotein (G) is most related to RABV pathogenicity. In the present study, to discover more amino acid sites related to viral pathogenicity, artificial mutants have been constructed in G of virulent strain GD-SH-01 backbone. Results showed that pathogenicity of GD-SH-01 significantly decreased when Gly349 was replaced by Glu349 through in vivo assays. Gly349→Glu349 of G did not significantly influence viral growth and spread in NA cells. Gly349→Glu349 of G increased the immunogenicity of GD-SH-01 in periphery and induced more expression of interferon alpha (IFN-α) in the brain in mice. It was observed that Gly349→Glu349 of G led to enhanced blood–brain barrier (BBB) permeability at day 5 postinfection. All together, these data revealed that Gly349→Glu349 of G mutation decreased RABV pathogenicity through enhanced immune response and increased BBB permeability. This study provides a new referenced site G349 that could attenuate pathogenicity of RABV.
Introduction
Rabies is an ancient zoonotic disease affecting the central nervous system (CNS) and continues to be a worldwide health problem. In humans, once the rabies symptoms manifest, the mortality rate is almost 100%. The causative agent of rabies is the rabies virus (RABV), which causes fatal encephalitis in warm-blooded animals (Jackson, 2003). RABV, an unsegmented, negative-stranded RNA virus, belongs to the genus Lyssavirus of the family Rhabdoviridae. The RABV genome is ∼12 kb in size and comprises five genes, encoding nucleoprotein (N), phosphoprotein (P), matrix protein (M), glycoprotein (G), and the RNA-dependent RNA polymerase (L) (Schnell et al., 2010). Glycoprotein, the sole protein exposed on the surface of the virion, is the most important determinant of RABV pathogenicity and is the major protein to induce virus neutralizing antibody (VNA) (Dietzschold et al., 1983; Morimoto et al., 1999; Ito et al., 2001; Faber et al., 2002, 2004). Different RABV strains possess different virulence mainly based on the different sequences of G proteins. Amino acid residues 333, 194, 37, and 242/255/268 in G have been demonstrated to be strongly related to pathogenicity (Faber et al., 2005; Takayama-Ito et al., 2006a, b; Yamada et al., 2014b). However, mutations in the G protein that contribute to the pathogenicity are sometimes strain dependent (Takayama-Ito et al., 2004, 2006b). Therefore, it is likely that some amino acid residues correlated to virulence have still not been discovered and reported in the literature. RABV G is comprised of a signal peptide, an ectodomain, a transmembrane domain, and a cytoplasmic tail (Wunner et al., 1988; Kuzmina et al., 2013). The ectodomain and cytoplasmic of G have been linked to the pathogenicity and immune evasion of the virus (Coulon et al., 1998; Prehaud et al., 2010; Virojanapirom et al., 2012; Huang et al., 2017). In addition, four major and one minor antigenic sites of G were identified using monoclonal antibodies (Lafon et al., 1984; Benmansour et al., 1991). Residues 34–42 and 198–200 of G have been recognized antigenic site II (Prehaud et al., 1988). Previous studies suggest that site III is from amino acids 330 to 338 in G (Seif et al., 1985; Wunner et al., 1988). Amino acid arginine at position 333 of G, which locates in site III, is virulent for adult mice (Dietzschold et al., 1983; Tuffereau et al., 1989). Therefore, most of the antigenic sites described locate in ectodomain of glycoprotein.
A highly pathogenic GD-SH-01 strain, which caused pigs’ death in a farm in Southern China, was isolated from a rabid pig (Luo et al., 2013). The analysis of whole-genome phylogeny and the comparison of nucleotide acid sequences suggested that the GD-SH-01 strain was closely associated with clade I of China, and it was found to be more pathogenic than challenge virus standard 24 (CVS-24) based on the pathogenicity index comparison (Luo et al., 2013). In an attempt to discover selective amino acids in G related to pathogenicity of RABV, and consequently provide some new referenced sites to generate highly attenuated rabies vaccines, we compared and analyzed the amino acid sequences of G from different RABV strains and conducted artificial amino acid mutation(s) on the backbone of GD-SH-01, with the corresponding amino acid(s) of HEP-Flury, which is one of the most attenuated rabies fixed strains and does not kill adult mice and was widely used as a vaccine strain (Fox et al., 1957; Sharpless et al., 1957; Morimoto et al., 2011). The pathogenicity of all mutants was then tested in adult mice. Here, we described a new amino acid site of RABV that related to its pathogenicity.
Materials and Methods
Cells, Viruses, and Animals
Mouse neuroblastoma (NA) cells (Wuhan Institute of Biological Products, Wuhan, China) were cultured in Roswell Park Memorial Institute (RPMI) 1640 medium (Gibco, Suzhou, China) with 10% fetal bovine serum (FBS) (Gibco, Grand Island, NY, United States). Baby hamster kidney (BHK-21) cells (Wuhan Institute of Biological Products, Wuhan, China) were maintained in Dulbecco’s modified Eagle’s medium (DMEM) (Gibco, Suzhou, China) containing 10% FBS. HEP-Flury (from Jiangsu Academy of Agricultural Sciences, China) were propagated in NA cells. The virulent wild-type strain GD-SH-01 was previously isolated from a rabid pig (Luo et al., 2013). Mutant RABV containing Arg333→Gln333 of G on the backbone of GD-SH-01 was rescued by our laboratory previously (unpublished data, here as a positive control of attenuated RABV strain). Female Kunming (KM) mice (6–7 weeks old), which are an outbreeding strain from Swiss mice, were purchased from the Center for Laboratory Animal Science of the Southern Medical University (Guangzhou, China). All animal experiments were performed under specific pathogen-free conditions in the Laboratory Animal Center of South China Agricultural University. All animal experiments were approved by the Ethics Committee for Animal Experiments of the South China Agricultural University and conducted in compliance with National Institutes of Health (NIH) guidelines (Zhang et al., 2016). All possible efforts were made to minimize the suffering of laboratory animals.
Selection of Amino Acid in G Protein
To choose the potential amino acids that could attenuate RABV GD-SH-01 strain (Luo et al., 2012), several pathogenic and non-pathogenic RABV strains were selected, and the amino acid sequences of the G’s ectodomain were compared, using MEGA 6 software [MEGA 6.06 (6140226)]. In this study, we selected partial strains including wild-type strains (from different hosts) and attenuated strains (pathogenic and non-pathogenic strains), together with interested wild-type strain GD-SH-01 (Table 1). The selected viral sequences were obtained from GenBank.
Amino acid site that determine pathogenicity of RABV is regularly different between pathogenic strains and non-pathogenic strains. Therefore, we selected amino sites that are different between pathogenic strains and non-pathogenic strains or between wild-type strains and attenuated strains. Therefore, selective amino acids, which differed in selected strains in the G of HEP-Flury were chosen: amino acid at position 19 in G (G19), G194, and G349. In addition, different amino acid residue of G243 between wild-type and attenuated strains was selected. Amino acid residues in the G protein of GD-SH-01 that are different from most other selected strains were chosen: G96 and G132. The selected strains’ information and amino acids are shown in Table 1. All the selected amino acid sites above were mutated artificially on the backbone of GD-SH-01 with the corresponding amino acid of HEP-Flury.
Construction of the Mutant Full-Length Genome cDNAs and Rescue of the Viruses
The plasmid containing the full-length genome complementary DNA (cDNA) of GD-SH-01 (rGDSH) was constructed and described previously (Tian et al., 2017). To construct the full-length genome cDNA that contains the G19, G96, G132, G194, G243, or G349 mutations of GD-SH-01, the plasmid of rGDSH was amplified using respective primers (Table 2). The amino acids at respective position of GD-SH-01 were replaced with the corresponding amino acids of HEP-Flury. The seamless cloning was performed using One Step Cloning Kit (Vazyme Biotech, Nanjing, China) according to the manufacturer’s protocols. Successful insertion was confirmed by DNA sequencing. All the mutant viruses were rescued in BHK-21 cells as described previously (Inoue et al., 2003; Luo et al., 2017). Rescued viruses were confirmed in NA cells by direct fluorescent antibody assay (dFA) with fluorescein isothiocyanate (FITC)-labeled anti-RABV N antibodies (Fujirabio Diagnostics, Malvern, PA, United States).
Virus Propagation and Titration
All the rescued mutant viruses (rGDSH-G19, rGDSH-G96, rGDSH-G132, rGDSH-G194, rGDSH-G243, and rGDSH-G349), rGDSH-G333, HEP-Flury and GD-SH-01 were propagated in NA cells (Neuro-cells which is sensitive to RABV). Virus titers were determined by dFA as described previously (Luo et al., 2016). Briefly, NA or BHK-21 cells grown in 96-well cell-culture plates were inoculated with 10-fold serial dilutions of the indicated virus in RPMI 1640 medium and incubated at 37°C with 5% CO2 for 2 days. Then, culture medium was discarded and cells were fixed with 80% acetone for 30 min at −20°C. Cells were washed with phosphate-buffered saline (PBS) three times and then stained with FITC-labeled anti-RABV N antibodies at 37°C for 60 min. Subsequently, antigen-positive foci were counted under a fluorescence microscope (AMG, Washington, United States), and virus titers were calculated as focus forming units (FFUs) per milliliter (FFU/ml) using the Karber method (Ramakrishnan, 2016).
Pathogenicity of RABV in Adult Mice
Pathogenicity of mutant strains was conducted in adult mice. KM mice (6–7 weeks of age) were inoculated intramuscularly (i.m.) with 1.0 × 105 FFU or intracerebrally (i.c.) with 2.0 × 103 FFU of HEP-Flury, GD-SH-01, rGDSH-G19, rGDSH-G96, rGDSH-G132, rGDSH-G194, rGDSH-G243, rGDSH-G349, or rGDSH-G333 (as a positive control of attenuated RABV strain). Each group consisted of five or six mice. Mortality was recorded daily for 21 days.
Virus Growth Curve in NA Cells
Monolayer cultures of 2 × 106 NA cells were infected with virus at a multiplicity of infection (MOI) of 0.1 FFU. Cells were then incubated at 37°C and harvested at 24, 48, 72, and 96 h postinoculation (hpi). Virus titers of samples were determined in NA cells by dFA, as described above. All titrations were carried out in triplicate.
Virus Spread Assay
The virus spread assay was performed in NA cells in 60-mm cell cultural dishes as described previously (Mei et al., 2019). Briefly, monolayer NA cells were infected with RABV at an MOI of 0.01 and incubated at 37°C. Cells were stained with FITC-labeled anti-RABV N antibodies at 24, 36, 48, and 60 hpi. Fluorescent foci in each dish were observed under a fluorescence microscope. The diameter of each fluorescent foci was measured using Adobe Photoshop CS software (Adobe, San Jose, United States) based on its scale. At least six fluorescent foci in each dish were measured.
Virus-Neutralizing Antibody Investigation in Adult Mice
Groups of five KM mice (6–7 weeks of age) were inoculated via the i.m. injection of 1.0 × 105 FFU rGDSH-G349 or GD-SH-01. RPMI 1640 medium was used for mock infection. Serum was collected from caudal vein at 9 days postinfection (dpi) and used to determine VNA levels by means of fluorescent antibody virus neutralization (FAVN) tests, as described previously (Cliquet et al., 1998).
Flow Cytometry
Flow cytometry was carried out to investigate the percentage of immune cells in the spleen after RABV infection. Briefly, KM mice (6–7 weeks of age) were infected i.m. with 1.0 × 105 FFU of rGDSH-G349 or GD-SH-01 or RPMI 1640 medium, respectively. Mouse spleen were harvested at 9 dpi. Single-cell suspensions were prepared followed by treatment with red blood cell lysis buffer (Beyotime, Shanghai, China) following the manufacturer’s instructions, and stained with antibodies against markers of T cells (FITC-CD3e, PE-CD4, PerCP-Cy5.5-CD8a) and B cells (FITC-CD19, PE-CD40) (all antibodies were purchased from Affymetrix eBioscience, United States) by incubation for 30 min on ice. A minimum of 50,000 events were counted using CytoFLEX flow cytometer (Beckman Coulter, United States). Data were analyzed using FlowJo software (Tree Star, Ashland, United States).
Quantitative Real-Time PCR
Kunming mice (6–7 weeks of age) were inoculated via the i.m. injection of 1.0 × 105 FFU rGDSH-G349 or GD-SH-01. At 5 and 9 dpi, mice were anesthetized with ketamine/xylazine (100/10 mg/kg) and then perfused by intracardiac injection of PBS. Whole brain tissues were harvested and then lysed in Magzol reagent (Magen, Guangzhou, China). Groups of three mice were used for each virus at one time point. Total RNA of each brain tissue sample was extracted using the HiPure Universal RNA Kit (Magen, Guangzhou, China) according to the manufacturer’s protocol. Reverse transcription was carried out using the RevertAid First Strand cDNA Synthesis Kit (Thermo Fisher Scientific, United States) following the manufacturer’s instructions. Quantitative real-time PCR (qRT-PCR) was performed using SYBR Green Master Mix (Vazyme Biotech Co., Ltd., Nanjing, China) in a CFX Connect Real-Time System (Bio-Rad, Hercules, CA, United States). Expression levels of interferon alpha (IFN-α) and immunoglobulin G (IgG) Ê-L chain were normalized to the house-keeping gene glyceraldehyde-3-phosphate dehydrogenase (GAPDH). Genomic RNA was determined with primers amplifying leader RNA and partial N. Primers used to amplify target and reference genes were described previously (Luo et al., 2017).
Measurement of Viral Load in Periphery
To evaluate RABV load at the inoculation site, KM mice (6–7 weeks of age) were inoculated in the right hind leg with 1.0 × 105FFU rGDSH-G349 or GD-SH-01. After the mice were humanely killed, the right hind leg muscles of five mice from each group were removed from infected mice at 1, 3, 5, 7, and 9 dpi and grinded in liquid nitrogen and then lysed in Magzol reagent (Magen). Total RNA was extracted using the HiPure Universal RNA Kit (Magen), and qRT-PCR was performed as described previously (Wang et al., 2014) to determine RABV genomic RNA in muscles using primers amplifying leader RNA and partial N. Genomic RNA was normalized to GAPDH.
Virus Growth Curve in Mouse Brain
Rabies virus growth curves in vivo were performed in mouse brain. KM mice (6–7 weeks of age) were inoculated i.m. with 1.0 × 105 FFU of rGDSH-D255G or GD-SH-01 in 30 μl RPMI 1640 medium. Three infected mice of each group were euthanized at 1, 3, 5, 7, and 9 dpi, and brains were harvested to detect the RABV genome using qRT-PCR as described previously (Luo et al., 2018). Three infected mouse brains were homogenized in a ninefold volume of RPMI 1640 medium and centrifuged at 12,000 × g for 10 min at 4°C following repeated freezing and thawing to investigate virus titer in brains. Supernatants were harvested, and virus titer was determined as described above.
Measurement of BBB Permeability Using Sodium Fluoride Uptake
Groups of three KM mice (6–7 weeks of age) were inoculated via the i.m. injection of 1.0 × 105 FFU rGDSH-G349 or GD-SH-01. The mock-infected mice were treated with RPMI 1640 medium. Blood–brain barrier (BBB) permeability was measured through the uptake of sodium fluoride as described previously (Luo et al., 2018) at 1, 3, 5, and 9 dpi. Data are expressed as fold change relative to mock-infected mice.
Statistical Analysis
Data were analyzed using GraphPad Prism 6 software (GraphPad Software, San Jose, CA, United States). The statistical significance was determined using the Student’s t-test. P < 0.05 was considered to be significantly different.
Results
Rescue of Mutant Viruses
Based on the full-length cDNA sequence of GD-SH-01, amino acid (Ile) at G19 was replaced by Leu (Ile19→Leu19), designated as rGDSH-G19; amino acid (Ser) at G96 was replaced by Ala (Ser96→Ala96), designated as rGDSH-G96; amino acid (Phe) at G132 was replaced by Leu (Phe132→Leu132), designated as rGDSH-G132; amino acid (Asn) at G194 was replaced by His (Asn194→His194), designated as rGDSH-G194; amino acid (Ile) at G243 was replaced by Met (Ile243→Met243), designated as rGDSH-G243; and amino acid (Gly) at G349 was replaced by Glu (Gly349→Glu349), designated as rGDSH-G349. Mutant RABV containing Arg333→Gln333 of G on the backbone of GD-SH-01 was termed rGDSH-G333 (Figure 1). The mutant strains were rescued in BHK-21 cells and each virus was verified in NA cells by immunofluorescence staining using FITC-conjugated antibodies against RABV N protein. Successful single amino acid mutation was confirmed by DNA sequencing.
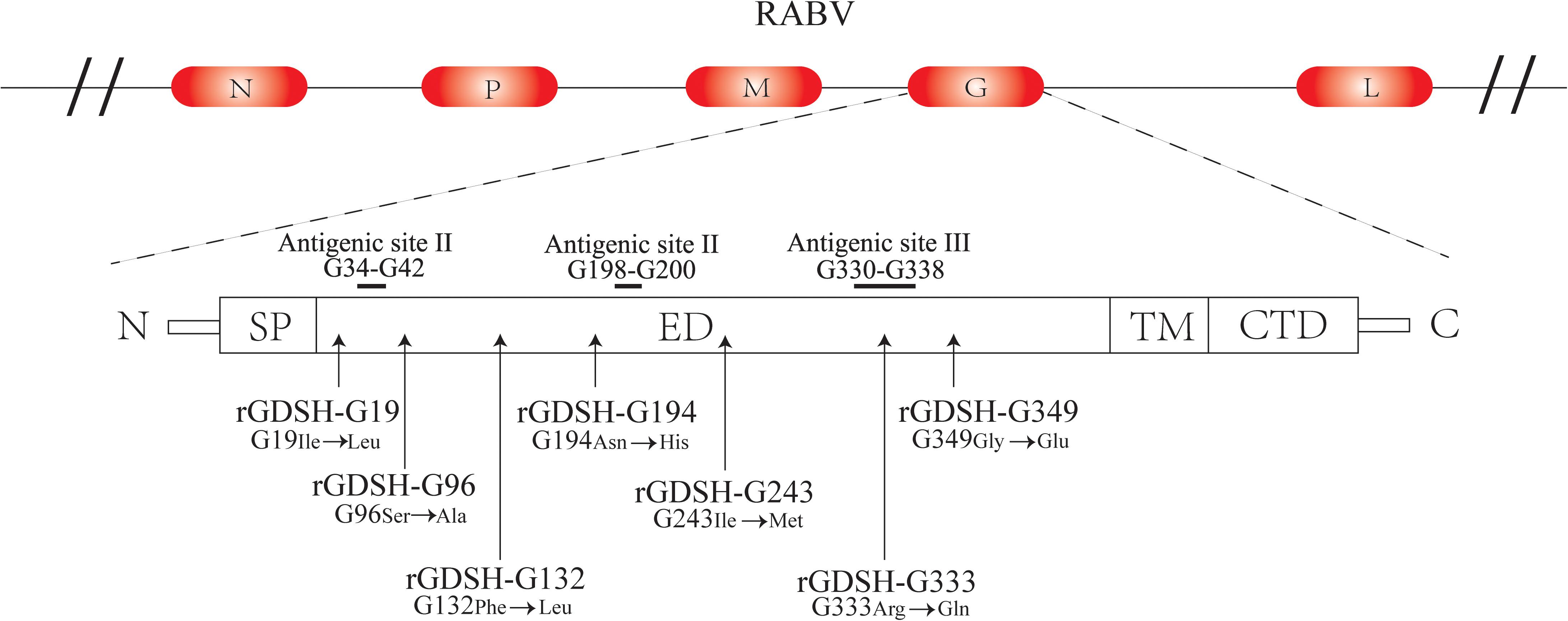
Figure 1. Schematic diagrams of mutations in glycoprotein of rabies virus (RABV). N, nucleoprotein; P, phosphoprotein; M, matrix protein; G, glycoprotein; L, RNA-dependent RNA polymerase. SP, signal peptide; ED, ectodomain; TM, transmembrane domain; CTD, cytoplasmic tail.
Pathogenicity of Mutant Strains in Adult Mice
To investigate if the selected mutations really attenuated the pathogenicity of RABV, adult KM mice (6–7 weeks) were i.m. or i.c. inoculated with each mutant strain. As shown in Figure 2, virulent strain GD-SH-01 caused 100% mortality by 14 dpi, while, as expected, all the mice survived the infection with the avirulent HEP-Flury through both i.m. and i.c. infection. As shown in Figure 2A, mutants rGDSH-G19, rGDSH-G96, rGDSH-G132, rGDSH-G194, and rGDSH-G243 caused 40, 60, 20, 20, and 40% mortality, respectively, while rGDSH-G349 caused no mice death through i.m. infection, same as the contrast group rGDSH-G333. As shown in Figure 2B, mutants rGDSH-G19, rGDSH-G96, rGDSH-G132, rGDSH-G194, and rGDSH-G243 caused 100% mortality, while rGDSH-G349 caused 50% mortality through i.c. infection. Contrast group rGDSH-G333 did not kill adult mice through i.c. infection (Figure 2B). These results indicated that mutations of G19, G96, G132, G194, or G243 decreased parental pathogenicity after i.m. inoculation, whereas they displayed the same level of pathogenicity as the parent GD-SH-01 after i.c. inoculation. In contrast, the G349 mutation showed to be a promising mutation, as it significantly attenuated GD-SH-01 without killing adult mice through i.m. inoculation.
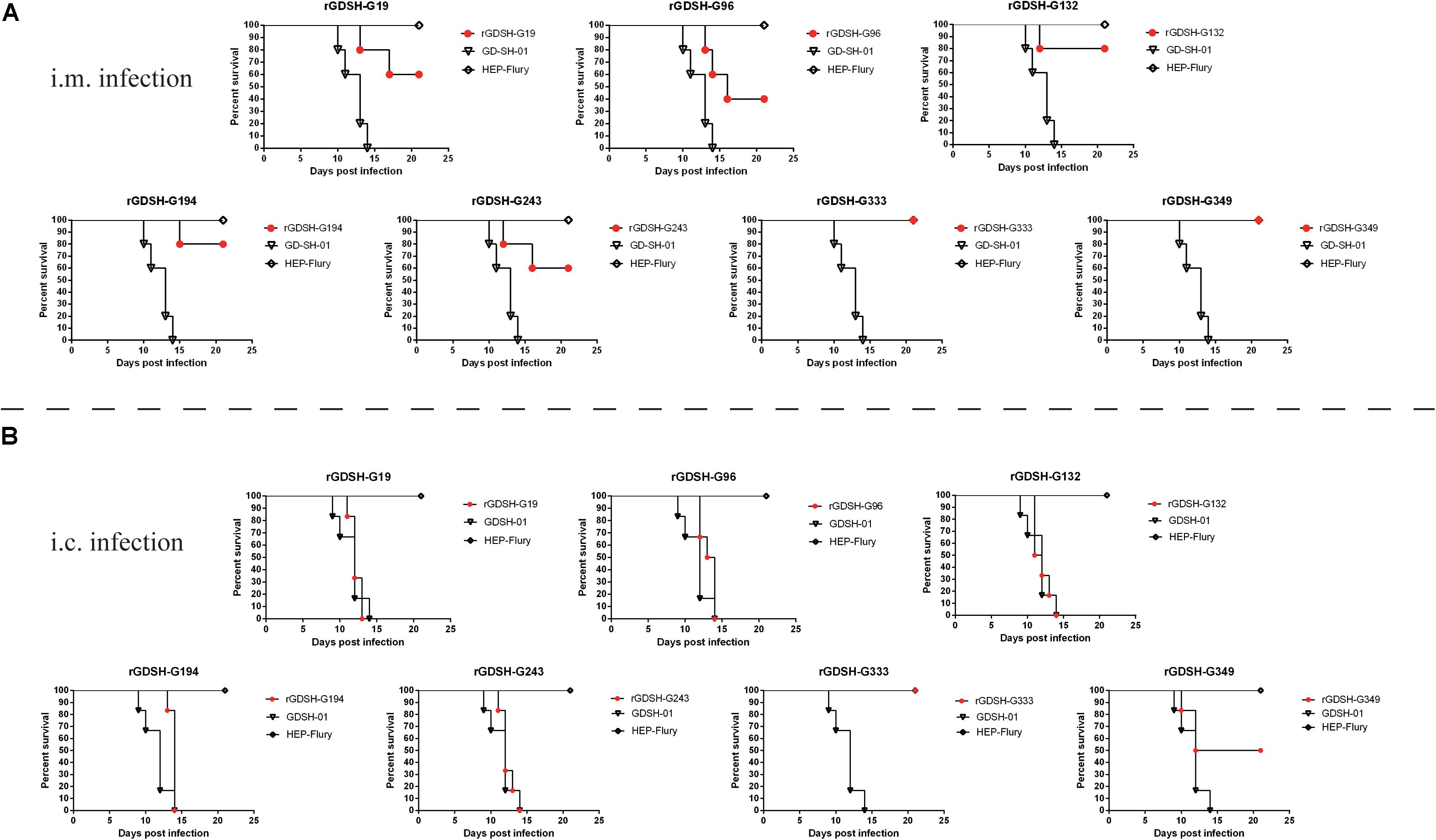
Figure 2. Pathogenicity of mutant rabies virus (RABV) strains in adult mice. Female Kunming (KM) mice (6–7 weeks of age) were inoculated i.m. (A) with 1.0 × 105 or i.c. (B) with 2.0 × 103FFU of HEP-Flury, GD-SH-01, rGDSH-G19, rGDSH-G96, rGDSH-G132, rGDSH-G194, rGDSH-G243, rGDSH-G333, or rGDSH-G349. Each group consisted of five or six mice. Mortality was recorded daily for 21 days.
Virus Growth Curve in NA Cells
Gly349→Glu349 of G significantly decreased RABV pathogenicity as described above. We therefore will focus on the investigation of rGDSH-G349. The in vitro growth curves of rGDSH-G349 were investigated in NA cells. As shown in Figure 3A, G349 mutation strain showed same growth curves compared with parent GD-SH-01. However, rGDSH-G349 reached the highest virus titers at 72 hpi, which was higher than GD-SH-01.
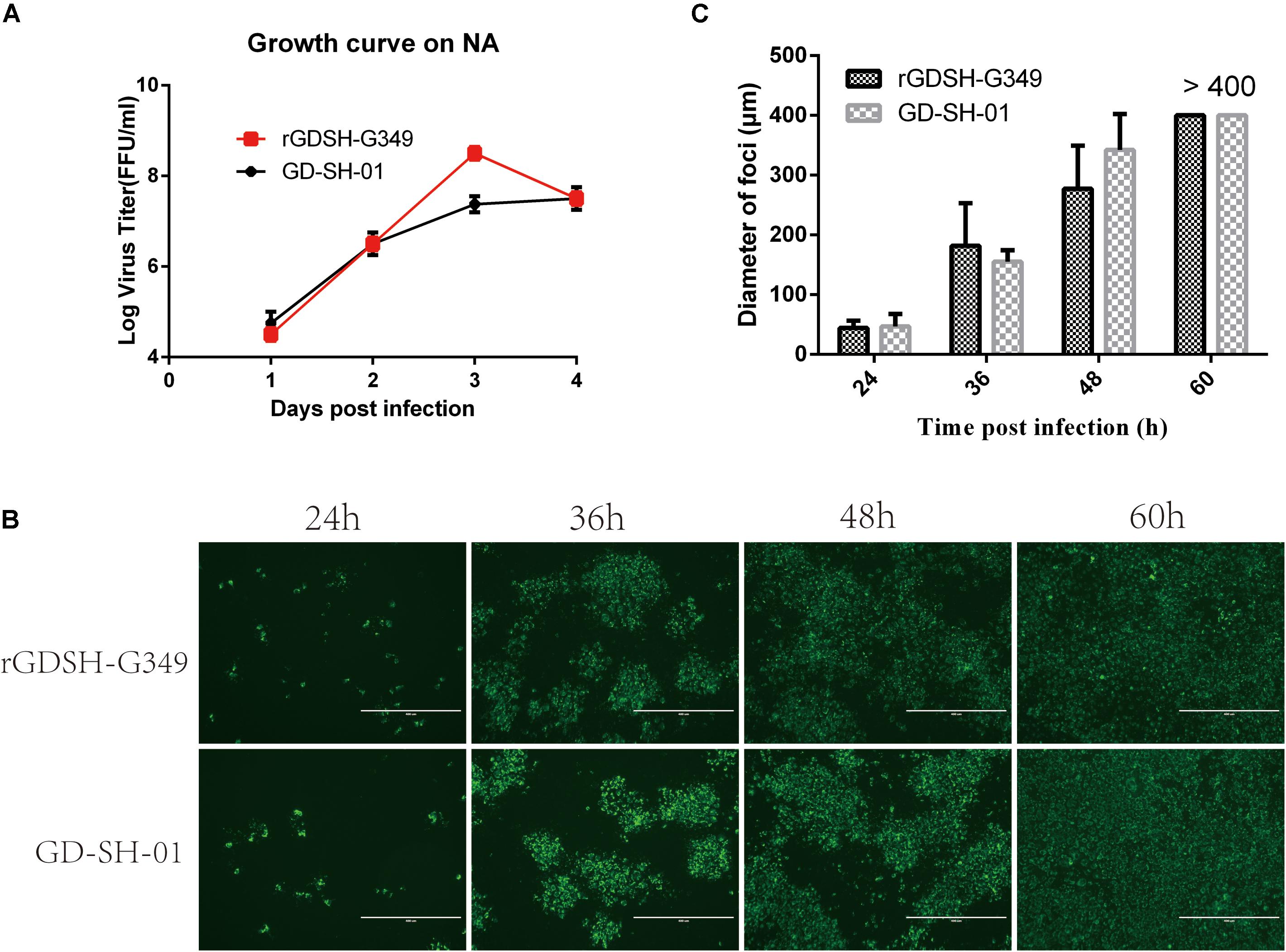
Figure 3. Growth curves and spread ability of rabies virus (RABV) in NA cells. (A) Growth curves. NA cells were infected with rGDSH-G349 or GD-SH-01, respectively, at a multiplicity of infection (MOI) of 0.1. At 1, 2, 3, and 4 dpi, culture supernatants were harvested, and virus titers were determined. (B,C) Viral spread in NA cells. Monolayer NA cells were infected with rGDSH-G349 or GD-SH-01 at an MOI of 0.01 and incubated at 37°C. Cells were stained with fluorescein isothiocyanate (FITC)-labeled anti-rabies virus (RABV) N antibodies at 24, 36, 48, and 60 hpi. The stained cells were examined under a fluorescence microscope, and for each group, the presentative image out of three replicates was shown (B). (C) The diameter of each fluorescent foci was measured based on its scale. Data are presented as mean values ± SE.
Spread of Viruses in NA Cells
G exposed on the surface of virion that is responsible for the interaction with host cells. Here, we investigated whether G349 mutations affect viral spread in NA cells. As shown in Figures 3B,C, Gly349→Glu349 mutation in G did not affect viral spread compared with parent GD-SH-01.
Immunogenicity of rGDSH-G349 in Adult Mice
After discovering that rGDSH-G349 significantly attenuated GD-SH-01 pathogenicity, an attempt was made to investigate its immunogenicity, which is essential in the clearance of RABV (Kaplan et al., 1975; Hooper et al., 1998). To investigate the immune response after Gly349→Glu349 mutation, flow cytometry was conducted to determine contents/counts of CD19 + CD40 + B cells, CD4 + T cells, and CD8 + T cells in spleen at 9 dpi. Figure 4A illustrates the gating strategy to identify CD19 + CD40 + B cells, and Figure 4B illustrates the gating strategy to identify CD4 + T cells and CD8 + T cells. As shown in Figure 4C, rGDSH-G349 recruited more CD19 + CD40 + B cells and CD8 + T cells (from CD3 + T cells) than parent GD-SH-01 in the spleen after i.m. infection. The counts of CD4 + T cells (from CD3 + T cells) induced by GD-SH-01 were more than those induced by rGDSH-G349.
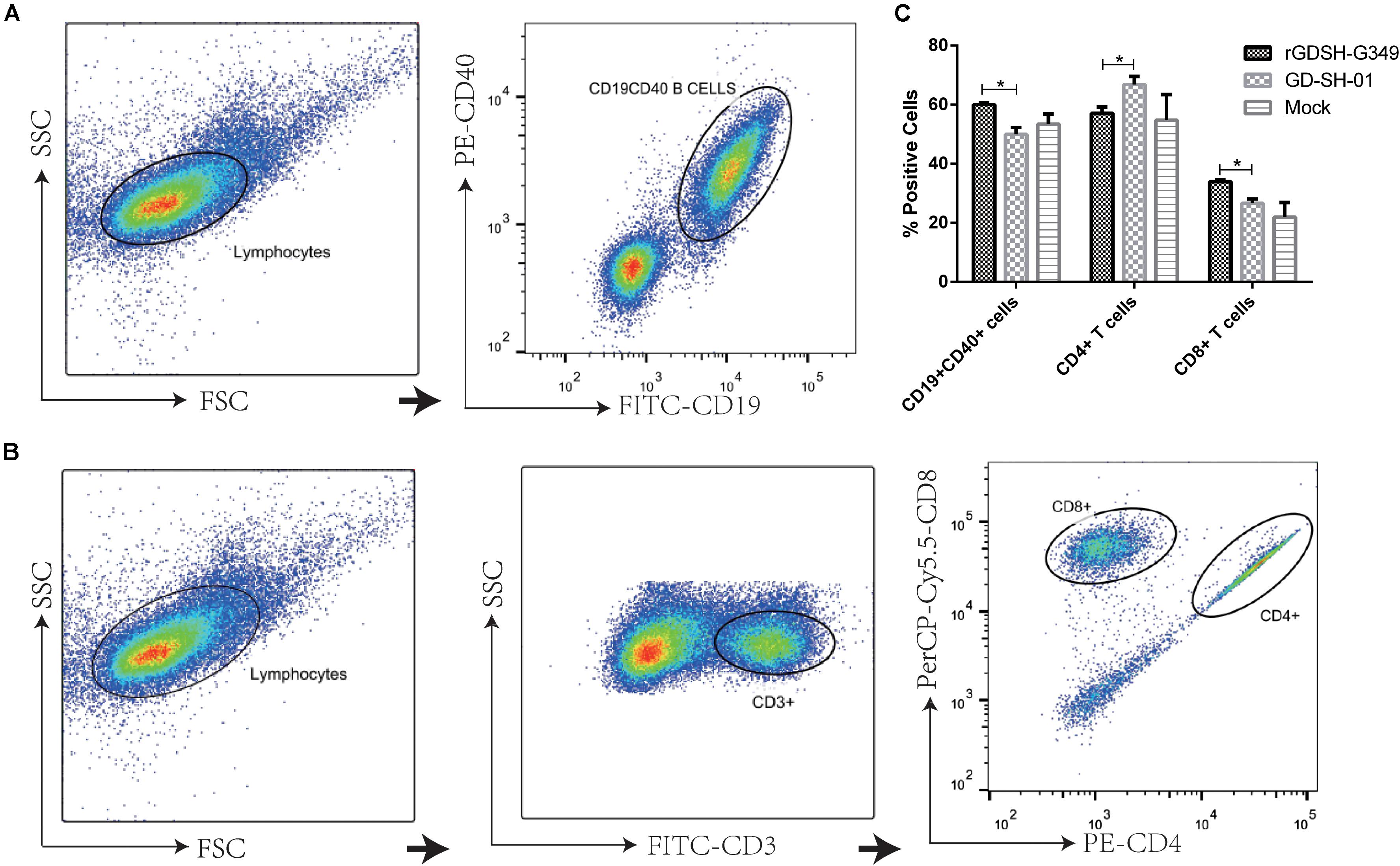
Figure 4. Flow cytometric analysis of immune cells in spleen. Female KM mice (6–7 weeks of age) were infected i.m. with 1.0 × 105FFU of rGDSH-G349, GD-SH-01, or medium alone (mock infection). Spleens were harvested at 9 dpi, and single-cell suspensions were prepared and stained with antibodies against markers of T cells (FITC-CD3e, PE-CD4, PerCP-Cy5.5-CD8a) and B cells (FITC-CD19, PE-CD40). Data were collected and analyzed with a CytoFLEX flow cytometer (Beckman Coulter) and FlowJo software (Tree Star). (A) Representative flow cytometric pseudocolor showing the gating strategy to identify CD19 + CD40 + B cells. (B) Representative flow cytometric pseudocolor showing the gating strategy to identify CD4 + T cells and CD8 + T cells. (C) Percentages of CD19 + CD40 + B cells, CD4 + T cells and CD8 + T cells in spleen (n ≥ 3 per group). Values are presented as mean ± SE. Asterisks indicate significant differences between groups, as calculated by Student’s t-test (*P < 0.05).
Virus neutralizing antibody in the periphery blood was determined at 9 dpi in mice after i.m. infection. As shown in Figure 5A, rGDSH-G349 was able to induce a higher level of VNA in periphery compared with parent GD-SH-01. In sum, Gly349→Glu349 mutation in GD-SH-01 strain enhances its immunogenicity in mice.
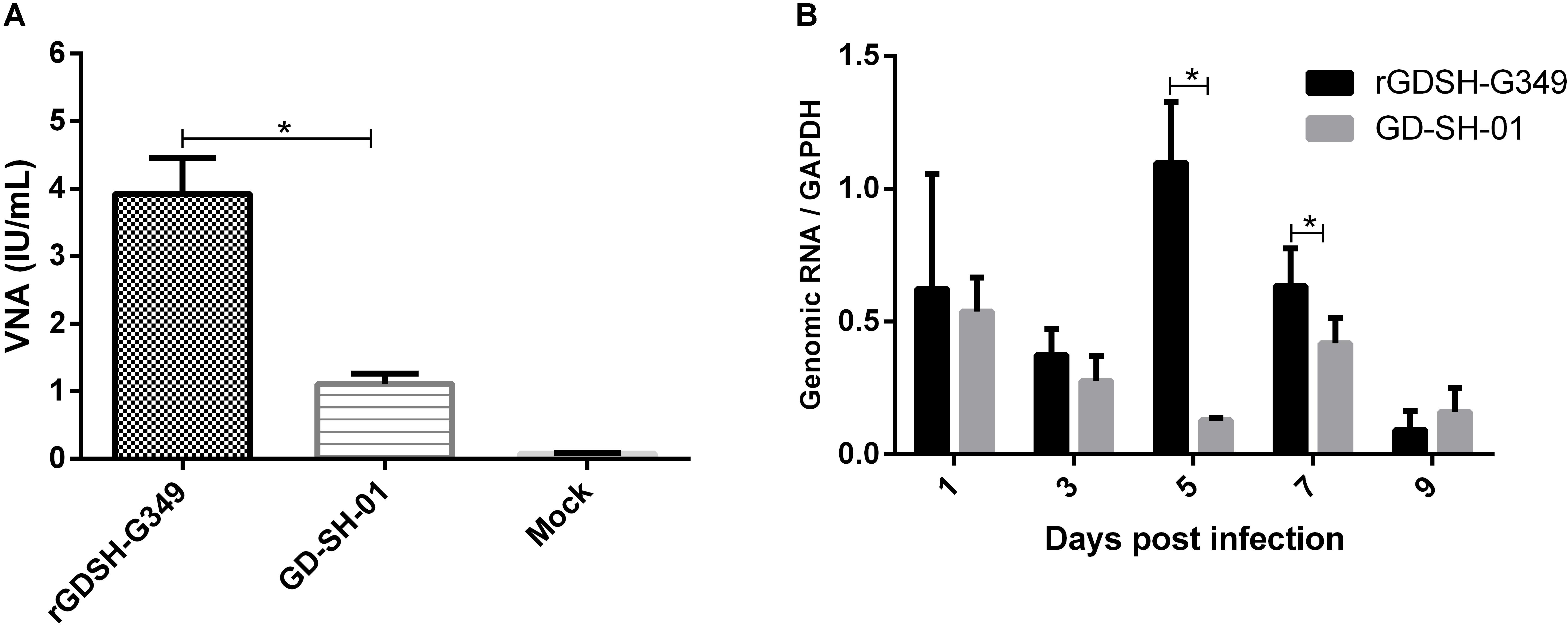
Figure 5. (A) Virus neutralizing antibody (VNA) in periphery. Groups of five female Kunming (KM) mice (6–7 weeks of age) were inoculated i.m. with 1.0 × 105 FFU of rGDSH-G349, GD-SH-01 or medium alone (mock infection). Peripheral blood was obtained at 9 dpi, and serum virus-neutralizing antibody was ascertained using fluorescent antibody virus neutralization test, as described in section “Materials and Methods.” (B) Viral load at inoculation site. KM mice (6–7 weeks of age) were inoculated in the right hind leg with 1.0 × 105 FFU rGDSH-G349 or GD-SH-01 and the right hind leg muscles were collected from infected mice at 1, 3, 5, 7, and 9 dpi. Genomic RNA of RABV were investigated by quantitative real-time PCR in a CFX Connect Real-Time System. Expression level were normalized to the housekeeping gene GAPDH messenger RNA (mRNA). Data were analyzed using BioRad CFX Manager and GraphPad Prism 6. Results were shown as the mean ± SE. Asterisks indicate significant differences between groups, as analyzed by t-test (*P < 0.05).
Viral Load of RABV at Inoculation Site
Viral load of RABV at the inoculation site was evaluated in KM mice after i.m. infection with rGDSH-G349 or GD-SH-01. As shown in Figure 5B, more genomic RNA of rGDSH-G349 than parent GD-SH-01 were determined at 5 and 7 dpi. Comparable viral load were detected in mice infected with rGDSH-G349 and GD-SH-01 at 1, 3, and 9 dpi.
Immune Effectors in CNS After RABV Infection
To investigate the innate immune response caused by RABVs in CNS, mice were immunized with rGDSH-G349 or GD-SH-01 via i.m. route. The messenger RNA (mRNA) levels of IFN-α was investigated using qRT-PCR. As shown in Figure 6A, rGDSH-G349 induced more IFN-α than parent GD-SH-01 at 5 dpi. This indicated that G349 mutation induced a stronger innate immune response in CNS at early stage.
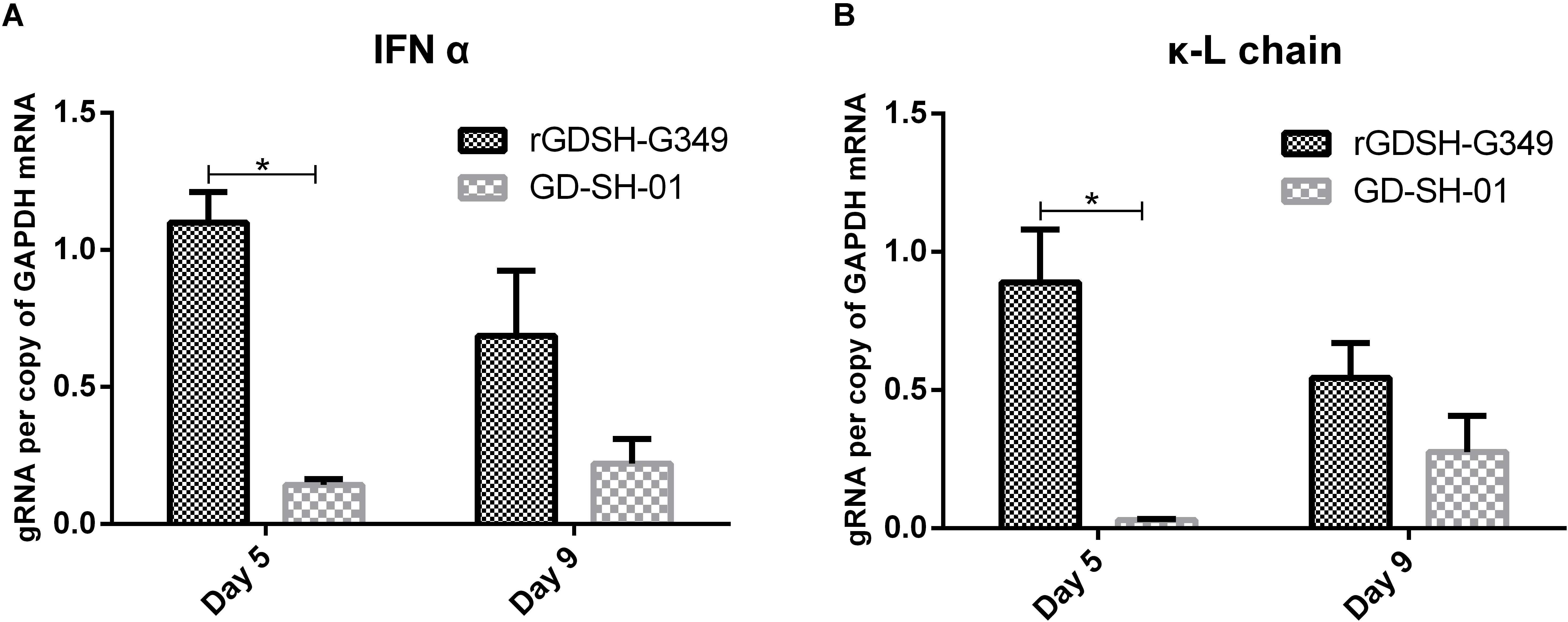
Figure 6. Expression of interferon alpha (IFN-α) (A) and immunoglobulin G (IgG) Ê-L chain (B) in the CNS. Female adult Kunming (KM) mice were inoculated i.m. with 1.0 × 105 FFU of rGDSH-G349 orGD-SH-01. At 5 and 9 dpi, mice were anesthetized, and brains were harvested following the perfusion with PBS. Expression of interferon alpha (IFN-α) and immunoglobulin G (IgG) Ê-L chain in brain tissues were investigated by quantitative real-time PCR in a CFX Connect Real-Time System. Expression level were presented per copy of the housekeeping gene glyceraldehyde-3-phosphate dehydrogenase (GAPDH) messenger RNA (mRNA0. Results were shown as the mean ± SE. Asterisks indicate significant differences among groups, as calculated by Student’s t-test (*P < 0.05).
IgG κ-L chain mRNA expression was determined to evaluate antibody level in brain tissues (Phares et al., 2006; Lebrun et al., 2015). As shown in Figure 6B, rGDSH-G349 induced more expression of IgG Ê-L chain than parent GD-SH-01 at 5 dpi. rGDSH-G349 infection triggered more immune effectors infiltrated into CNS than GD-SH-01.
Virus Growth Curve in Mouse Brain
Mice were inoculated i.m. with rGDSH-G349 or GD-SH-01, and viral genomes and live virus particles were detected at various times postinoculation to further investigate whether G349 mutation affect virus replication in the CNS. Comparable viral genome and live virus particle levels were detected in mice infected between rGDSH-G349 and GD-SH-01 at 1, 3, and 5 dpi (Figure 7). However, the levels of viral genome and live virus particle of rGDSH-G349 were significantly lower than that of GD-SH-01 at 7 and 9 dpi (Figure 7).
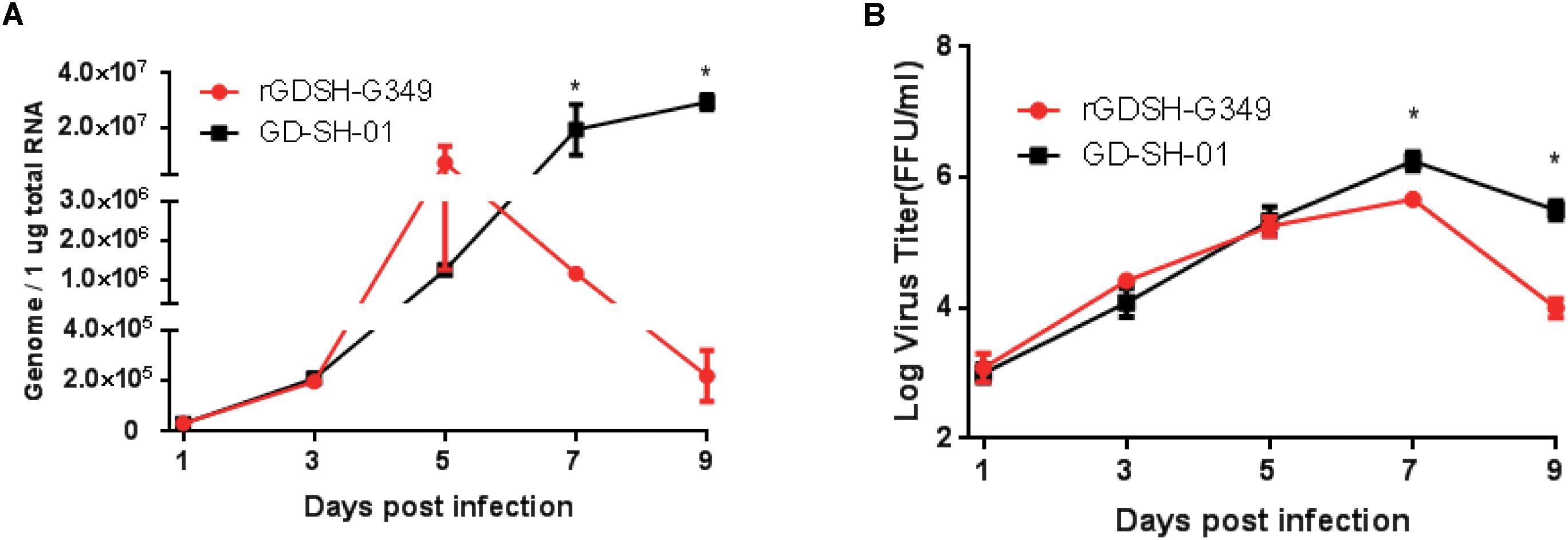
Figure 7. Growth curves of rGDSH-G349 and GD-SH-01 in mouse brain. Kunming (KM) mice were inoculated with 1.0 × 105 FFU of rGDSH-G349 or GD-SH-01 via an i.m. route. Mice were euthanized at 1, 3, 5, 7, and 9 dpi, and brains were harvested to determine rabies virus (RABV) genome (A) using quantitative real-time PCR and live virus titers (B) as described in Materials and Methods. Three mice were used for RABV genome investigations, and three mice were used for virus titers investigations per group. Data are presented as mean values ± SE. Asterisks indicate significant differences between the two groups, as calculated using Student’s t-test (*P < 0.05).
BBB Permeability Caused by rGDSH-G349 in Adult Mice
Enhanced BBB permeability may contribute to the clearance of infected RABV in CNS. Here, we investigated whether G349 mutation changed BBB permeability after infection. As shown in Figure 8, mice infected with rGDSH-G349 exhibited increased levels of NaF compared with GD-SH-01 in both the cerebrum and cerebellum at 5 dpi. This suggests that G349 mutation strain enhanced BBB permeability.
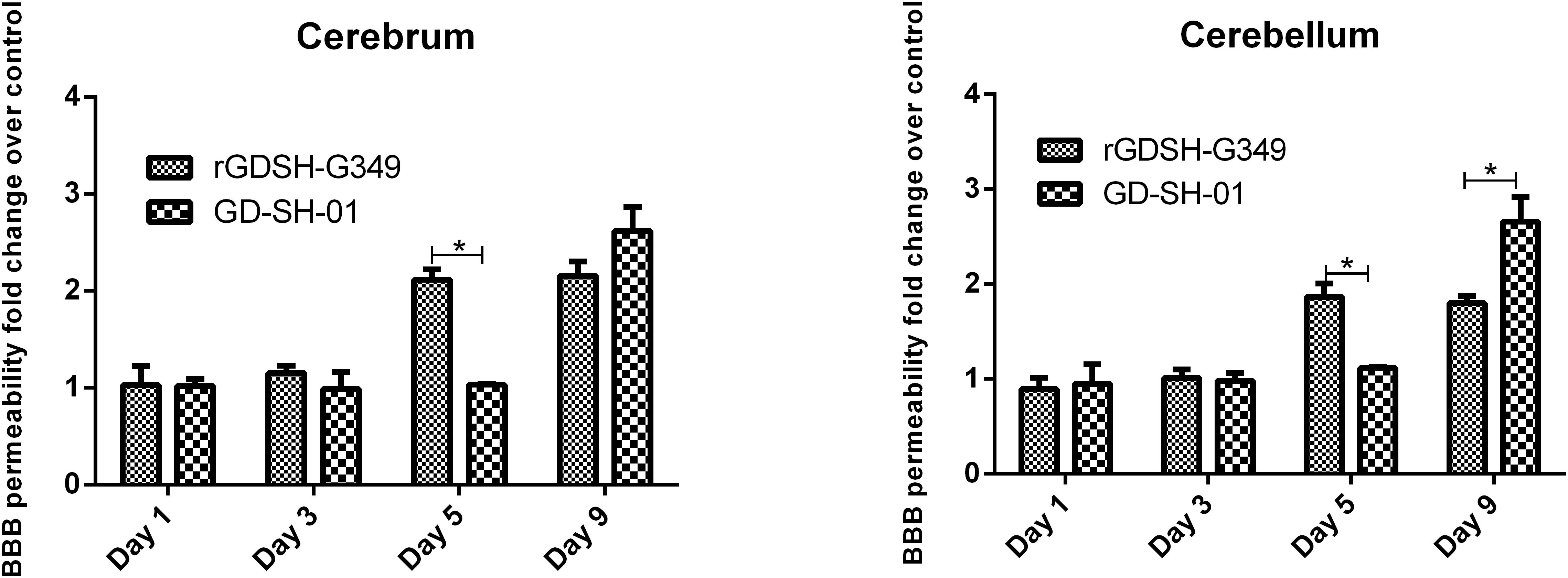
Figure 8. Blood–brain barrier (BBB) permeability in the cerebrum and cerebellum of mice infected with rabies virus (RABV). Kunming (KM) mice (6–7 weeks old) were inoculated i.m. with either rGDSH-G349 or GD-SH-01. RPMI 1640 medium was used for the mock infection. At 1, 3, 5, or 9 dpi, brains were harvested, and BBB permeability was measured by NaF uptake in the cerebrum and cerebellum. Data are presented as fold change over mock infection. *Indicate significant differences among groups, as calculated by Student’s t-test (*P < 0.05).
Discussion
Reverse genetic technology is a powerful tool to investigate RABV, and it has been used since the first RABV was rescued from cloned cDNA (Schnell et al., 1994). Since then, several studies have successfully used reverse genetic technology to construct mutant strains of RABV to investigate their pathogenicity or immunogenicity (Takayama-Ito et al., 2006a, b; Yamada et al., 2014b; Nakagawa et al., 2017). Previous studies have confirmed that Ala242, Asp255, Ile268, Lys330, and Arg333 are all involved in viral pathogenicity (Tuffereau et al., 1989; Coulon et al., 1998; Faber et al., 2005; Takayama-Ito et al., 2006b), suggesting that more than one amino acid are associated with RABV pathogenicity. Here, we speculate that, in addition to the reported amino acid, other novel amino acid sites in G determine the pathogenicity of RABV. By comparing the ectodomain of G from various strains, we selected six potential amino sites, which might be related to pathogenicity of RABV. In this study, mutations were conducted in the backbone of GD-SH-01, which is a highly virulent strain isolated from pig (Luo et al., 2013). To illustrate the relationship between amino acid site and pathogenicity, the viral strain was compared to the avirulent strain HEP-Flury, and the corresponding referenced amino acids were matched and replaced. All the mutant strains were rescued, and the pathogenicity was investigated. Here, we found that Gly349→Glu349 mutation in G decreased RABV pathogenicity, which was not reported previously.
Interestingly, the comparison of selected G gene sequences highlighted that the HEP-Flury strain exclusively contained Glu at G349, while other strains contained a Gly in that position (Table 1). In addition, only HEP-Flury strain presented Gln at G333, while other strains (in Table 1) contained an Arg. Previous studies had indicated that pathogenicity of RABV decreased significantly when Arg or Lys at G333 was replaced by other amino acids (Seif et al., 1985; Tuffereau et al., 1989; Tao et al., 2010). Therefore, selecting potential sites present in avirulent strains, but not in virulent strains, is an approved strategy for studying the effect of mutation on pathogenicity. Of note, RC-HL strain contains Arg at G333, but it is an avirulent strain (Ito et al., 1994); therefore, mutations related to pathogenicity of RABV are strains dependent. Further studies are necessary to investigate if Glu at G349 could attenuate other RABV strains.
Previous study indicates that by exchanging the ectodomain of G from wild-type with attenuated RABVs, the virus titers and spread ability were altered (Huang et al., 2017). In this study, we discovered that the single mutation at G349 did not significantly alter the virus growth curves and spread ability in NA cells. It has been reported that multiple mutations at positions 252/255/268 of G protein of RC-HL strain did not alter the virus titers in NA cells (Ito et al., 2010). However, single amino mutation at position 37 or 146 of G protein of 1088 strain significantly increased virus replication in NA cells (Yamada et al., 2014b). In addition, mutation of Asn194 of G protein attenuated strain SPBNGA with Ser had no effect on virus production, while mutation with Lys decreases virus production (Faber et al., 2005). These previous findings suggest that whether a single or multiple mutations of the G protein of RABV really exerts effect on virus replication is dependent on the specific mutant site or the specific RABV strains, or on both events.
Previous studies indicate that R196S mutation and D247N mutation of G in strain 1088 variants, which led to an additional N-glycosylation and decrease in pathogenicity of RABV (Yamada et al., 2014a, b). Therefore, N-glycosylation in G may influence pathogenicity of RABV (Yamada et al., 2014b). In this study, G349 mutation does not increase or decrease N-glycosylation. Normally, pathogenic RABV infection causes adult mice death while, attenuated RABV does not kill adult mice (Clark, 1978; Morimoto et al., 1998; Takayama-Ito et al., 2004; Shimizu et al., 2007; Tao et al., 2010; Wirblich and Schnell, 2011; Tian et al., 2016; Miao et al., 2017). Here, we found that mutation from Gly to Glu at G349 significantly attenuated GD-SH-01, the same as mutation of G333, without killing adult mice by i.m. inoculation. Neuroinvasiveness do not contribute to the attenuated virulence of G349 mutation because rGDSH-G349 showed the same growth ability compared with parent GD-SH-01 at an early stage of infection in the CNS. There must be other factors that direct the clearance of infected rGDSH-G349. Both innate immune response and adaptive immune response are essential in the clearance of RABV (Kaplan et al., 1975; Hooper et al., 1998; Chopy et al., 2011).
G plays the most important role in the induction of VNA (Cox et al., 1977). A previous study has confirmed that variant 1088-N30 induces higher level of VNA than street strain 1088 in serum in the earlier phase of infection after i.m. inoculation (Yamada et al., 2012). In this study, we found that VNA titer in mice induced by rGDSH-G349 was higher than parent GD-SH-01 after i.m. infection. What’s more, rGDSH-G349 recruited more CD19 + CD40 + B cells and CD8 + T cells in spleen. The immunogenicity of rGDSH-G349 is stronger than GD-SH-01, and this may be due to more viral load at inoculation site as the results showed. In addition, Gly349→Glu349 mutation attenuated RABV pathogenicity, and this may contribute to the activation of dendritic cells (Yang et al., 2015). In addition, G349 mutation enhanced IgG Ê-L chain and IFN-α expression in CNS. Therefore, the enhanced innate immune response in CNS and infiltration of immune effectors induced by G349 mutation might contribute to the clearance of infected RABV. This explained why the virus load of rGDSH-G349 decreased at a late stage of infection in CNS.
Recent studies have confirmed the crystal structures of RABV G and its interaction with neutralizing antibodies (Hellert et al., 2020; Yang et al., 2020). Residues from G333–G350 of ectodomain from three strains (CVS-11, Flury, and SAD-B19) are observed to bond to antibody 523-11 (Yang et al., 2020). They also found that antibody 523-11 could block the G-mediated syncytia formation of CVS-11, Flury, and SAD-B19 strains. However, antibody 523-11 only inhabits the infection of cells by Flury. The different residues from G333 to G350 were G333 (Gln: Arg) and G349 (Glu: Gly) between Fulry and CVS-11or SAD-B19. In this study, interested G349 locates the region that is a target of neutralizing antibodies, and its mutation may change the interaction with host cells. Further work is needed to confirm this speculation.
Enhanced BBB permeability caused by attenuated RABV also contribute to the clearance of infected RABV (Phares et al., 2006; Roy et al., 2007; Hooper et al., 2009; Chai et al., 2015). In this study, we found that rGDSH-G349 rather than parent GD-SH-01 significantly enhanced BBB permeability at 5 dpi. This might contribute to the enhanced inflammation in CNS and subsequently clear the infected RABV. In addition, VNA in serum may also contribute to the clearance of RABV in the CNS when BBB permeability opens (Huang et al., 2014). rGDSH-G349 induced higher levels of VNA in periphery after i.m. infection.
Previous studies indicated that attenuation caused by a single mutation in G of RBAV may revert according to reverse mutation or new mutation occurred in other amino acid site (Faber et al., 2005; Tao et al., 2010). Multiple amino acid changes could extensively attenuate the pathogenicity of RBAV and improve the stability of attenuation phenotype (Mebatsion, 2001; Dietzschold et al., 2004; Faber et al., 2005; Masatani et al., 2011; Nakagawa et al., 2017). Therefore, G349 could be a potential site when construct multiple amino acid changes to extensively attenuate the RABV pathogenicity.
In summary, by comparing the amino acid sequence of G from different RABV strains, we selected six potential pathogenic amino acid positions. Gly349→Glu349 mutation in G significantly decreased RABV pathogenicity through enhanced immune response rather than decreased replication. G349 mutation enhanced BBB permeability and might contribute to the clearance of RABV in CNS. In conclusion, this study discovered a new amino acid site of RABV related to pathogenicity.
Data Availability Statement
The datasets generated for this study are available on request to the corresponding author.
Ethics Statement
The animal study was reviewed and approved by the Ethics Committee for Animal Experiments of the South China Agricultural University.
Author Contributions
XG and JL conceived and designed the experiments. JL, BZ, and YW performed the experiments. JL and XG analyzed the data. JL and XG wrote the manuscript. All authors read and approved the final manuscript.
Funding
This study was partially supported by the National Nature Science Foundation of China (No. 31772742), the National Key Research and Development Program of China (No. 2016YFD0500400), and the Natural Science Foundation of Guangdong (No. 2015A03031103).
Conflict of Interest
The authors declare that the research was conducted in the absence of any commercial or financial relationships that could be construed as a potential conflict of interest.
References
Benmansour, A., Leblois, H., Coulon, P., Tuffereau, C., Gaudin, Y., Flamand, A., et al. (1991). Antigenicity of rabies virus glycoprotein. J. Virol. 65, 4198–4203. doi: 10.1128/jvi.65.8.4198-4203.1991
Chai, Q., She, R., Huang, Y., and Fu, Z. F. (2015). Expression of neuronal CXCL10 induced by rabies virus infection initiates infiltration of inflammatory cells, production of chemokines and cytokines, and enhancement of blood-brain barrier permeability. J. Virol. 89, 870–876. doi: 10.1128/JVI.02154-14
Chopy, D., Detje, C. N., Lafage, M., Kalinke, U., and Lafon, M. (2011). The type I interferon response bridles rabies virus infection and reduces pathogenicity. J. Neurovirol. 17, 353–367. doi: 10.1007/s13365-011-0041-6
Clark, H. F. (1978). Rabies viruses increase in virulence when propagated in neuroblastoma cell culture. Science 199, 1072–1075. doi: 10.1126/science.628831
Cliquet, F., Aubert, M., and Sagne, L. (1998). Development of a fluorescent antibody virus neutralisation test (FAVN test) for the quantitation of rabies-neutralising antibody. J. Immunol. Methods 212, 79–87. doi: 10.1016/s0022-1759(97)00212-3
Coulon, P., Ternaux, J. P., Flamand, A., and Tuffereau, C. (1998). An avirulent mutant of rabies virus is unable to infect motoneurons in vivo and in vitro. J. Virol. 72, 273–278. doi: 10.1128/jvi.72.1.273-278.1998
Cox, J. H., Dietzschold, B., and Schneider, L. G. (1977). Rabies virus glycoprotein. II. Biological and serological characterization. Infect. Immun. 16, 754–759. doi: 10.1128/iai.16.3.754-759.1977
Dietzschold, B., Wunner, W. H., Wiktor, T. J., Lopes, A. D., Lafon, M., Smith, C. L., et al. (1983). Characterization of an antigenic determinant of the glycoprotein that correlates with pathogenicity of rabies virus. Proc. Natl. Acad. Sci. U.S.A. 80, 70–74. doi: 10.1073/pnas.80.1.70
Dietzschold, M. L., Faber, M., Mattis, J. A., Pak, K. Y., and Schnell, M. J. (2004). In vitro growth and stability of recombinant rabies viruses designed for vaccination of wildlife. Vaccine 23, 518–524. doi: 10.1016/j.vaccine.2004.06.031
Faber, M., Faber, M. L., Papaneri, A., Bette, M., Weihe, E., Dietzschold, B., et al. (2005). A single amino acid change in rabies virus glycoprotein increases virus spread and enhances virus pathogenicity. J. Virol. 79, 14141–14148. doi: 10.1128/jvi.79.22.14141-14148.2005
Faber, M., Pulmanausahakul, R., Hodawadekar, S. S., Spitsin, S., McGettigan, J. P., Schnell, M. J., et al. (2002). Over expression of the rabies virus glycoprotein results in enhancement of apoptosis and antiviral immune response. J. Virol. 76, 3374–3381. doi: 10.1128/jvi.76.7.3374-3381.2002
Faber, M., Pulmanausahakul, R., Nagao, K., Prosniak, M., Rice, A. B., Koprowski, H., et al. (2004). Identification of viral genomic elements responsible for rabies virus neuroinvasiveness. Proc. Natl. Acad. Sci. U.S.A. 101, 16328–16332. doi: 10.1073/pnas.0407289101
Fox, J. P., Koprowski, H., Conwell, D. P., Black, J., and Gelfand, H. M. (1957). Study of antirabies immunization of man; observations with HEP Flury and other vaccines, with and without hyperimmune serum, in primary and recall immunizations. Bull. World Health Organ. 17, 869–904.
Hellert, J., Buchrieser, J., Larrous, F., Minola, A., de Melo, G. D., Soriaga, L., et al. (2020). Structure of the prefusion-locking broadly neutralizing antibody RVC20 bound to the rabies virus glycoprotein. Nat. Commun. 11:596. doi: 10.1038/s41467-020-14398-7
Hooper, D. C., Morimoto, K., Bette, M., Weihe, E., Koprowski, H., Dietzschold, B., et al. (1998). Collaboration of antibody and inflammation in clearance of rabies virus from the central nervous system. J. Virol. 72, 3711–3719. doi: 10.1128/jvi.72.5.3711-3719.1998
Hooper, D. C., Phares, T. W., Fabis, M. J., and Roy, A. (2009). The production of antibody by invading B cells is required for the clearance of rabies virus from the central nervous system. PLoS Negl. Trop. Dis. 3:e535. doi: 10.1371/journal.pntd.0000535
Huang, C. T., Li, Z., Huang, Y., Zhang, G., Zhou, M., Chai, Q., et al. (2014). Enhancement of blood-brain barrier permeability is required for intravenously administered virus neutralizing antibodies to clear an established rabies virus infection from the brain and prevent the development of rabies in mice. Antiviral Res. 110, 132–141. doi: 10.1016/j.antiviral.2014.07.013
Huang, J., Zhang, Y., Huang, Y., Gnanadurai, C. W., Zhou, M., Zhao, L., et al. (2017). The ectodomain of rabies virus glycoprotein determines dendritic cell activation. Antiviral Res. 141, 1–6. doi: 10.1016/j.antiviral.2017.01.022
Inoue, K., Shoji, Y., Kurane, I., Iijima, T., and Sakai, T. (2003). An improved method for recovering rabies virus from cloned cDNA. J. Virol. Methods 107, 229–236. doi: 10.1016/s0166-0934(02)00249-5
Ito, H., Minamoto, N., Watanabe, T., Goto, H., Rong, L. T., Sugiyama, M., et al. (1994). A unique mutation of glycoprotein gene of the attenuated RC-HL strain of rabies virus, a seed virus used for production of animal vaccine in Japan. Microbiol. Immunol. 38, 479–482. doi: 10.1111/j.1348-0421.1994.tb01812.x
Ito, N., Takayama, M., Yamada, K., Sugiyama, M., and Minamoto, N. (2001). Rescue of rabies virus from cloned cDNA and identification of the pathogenicity-related gene: Glycoprotein gene is associated with virulence for adult mice. J. Virol. 75, 9121–9128. doi: 10.1128/JVI.75.19.9121-9128.2001
Ito, Y., Ito, N., Saito, S., Masatani, T., Nakagawa, K., Atoji, Y., et al. (2010). Amino acid substitutions at positions 242, 255 and 268 in rabies virus glycoprotein affect spread of viral infection. Microbiol. Immunol. 54, 89–97. doi: 10.1111/j.1348-0421.2009.00192.x
Jackson, A. C. (2003). Rabies virus infection: an update. J. Neurovirol. 9, 253–258. doi: 10.1080/13550280390193975
Kaplan, M. M., Wiktor, T. J., and Koprowski, H. (1975). Pathogenesis of rabies in immunodeficient mice. J. Immunol. 114, 1761–1765.
Kuzmina, N. A., Kuzmin, I. V., Ellison, J. A., Taylor, S. T., Bergman, D. L., Dew, B., et al. (2013). A reassessment of the evolutionary timescale of bat rabies viruses based upon glycoprotein gene sequences. Virus Gen. 47, 305–310. doi: 10.1007/s11262-013-0952-9
Lafon, M., Ideler, J., and Wunner, W. H. (1984). Investigation of the antigenic structure of rabies virus glycoprotein by monoclonal antibodies. Dev. Biol. Stand. 57, 219–225.
Lebrun, A., Portocarrero, C., Kean, R. B., Barkhouse, D. A., Faber, M., Hooper, D. C., et al. (2015). T-bet is required for the rapid clearance of attenuated rabies virus from central nervous system tissue. J. Immunol. 195, 4358–4368. doi: 10.4049/jimmunol.1501274
Luo, J., Shi, H., Tan, Y., Niu, X., Long, T., Zhao, J., et al. (2016). Two potential recombinant rabies vaccines expressing canine parvovirus virion protein 2 induce immunogenicity to canine parvovirus and rabies virus. Vaccine 34, 4392–4398. doi: 10.1016/j.vaccine.2016.07.020
Luo, J., Zhang, B., Wu, Y., Tian, Q., Mo, M., Long, T., et al. (2018). Recombinant rabies virus expressing interleukin-6 enhances the immune response in mouse brain. Arch. Virol. 163, 1889–1895. doi: 10.1007/s00705-018-3808-8
Luo, J., Zhang, B., Wu, Y., Tian, Q., Zhao, J., Lyu, Z., et al. (2017). Expression of interleukin-6 by a recombinant rabies virus enhances its immunogenicity as a potential vaccine. Vaccine 35, 938–944. doi: 10.1016/j.vaccine.2016.12.069
Luo, Y., Zhang, Y., Liu, X., Yang, Y., and Yang, X. (2013). Characterization of a wild rabies virus isolate of porcine origin in China. Infect. Genet. Evol. 17, 147–152. doi: 10.1016/j.meegid.2013.03.046
Luo, Y., Zhang, Y., Liu, X., Yang, Y., Yang, X., Zhang, D., et al. (2012). Complete genome sequence of a highly virulent rabies virus isolated from a rabid pig in south China. J. Virol. 86, 12454–12455. doi: 10.1128/JVI.02234-12
Masatani, T., Ito, N., Shimizu, K., Ito, Y., Nakagawa, K., Abe, M., et al. (2011). Amino acids at positions 273 and 394 in rabies virus nucleoprotein are important for both evasion of host RIG-I-mediated antiviral response and pathogenicity. Virus Res. 155, 168–174. doi: 10.1016/j.virusres.2010.09.016
Mebatsion, T. (2001). Extensive attenuation of rabies virus by simultaneously modifying the dynein light chain binding site in the P protein and replacing Arg333 in the G protein. J. Virol. 75, 11496–11502. doi: 10.1128/JVI.75.23.11496-11502.2001
Mei, M., Long, T., Zhang, Q., Zhao, J., Tian, Q., Peng, J., et al. (2019). Phenotypic consequence of rearranging the n gene of RABV HEP-Flury. Viruses 11:E402. doi: 10.3390/v11050402
Miao, F. M., Zhang, S. F., Wang, S. C., Liu, Y., Zhang, F., Hu, R. L., et al. (2017). Comparison of immune responses to attenuated rabies virus and street virus in mouse brain. Arch. Virol. 162, 247–257. doi: 10.1007/s00705-016-3081-7
Morimoto, K., Hooper, D. C., Carbaugh, H., Fu, Z. F., Koprowski, H., Dietzschold, B., et al. (1998). Rabies virus quasispecies: implications for pathogenesis. Proc. Natl. Acad. Sci. U.S.A. 95, 3152–3156. doi: 10.1073/pnas.95.6.3152
Morimoto, K., Hooper, D. C., Spitsin, S., Koprowski, H., and Dietzschold, B. (1999). Pathogenicity of different rabies virus variants inversely correlates with apoptosis and rabies virus glycoprotein expression in infected primary neuron cultures. J. Virol. 73, 510–518. doi: 10.1128/jvi.73.1.510-518.1999
Morimoto, K., Kawai, A., Sato, Y., and Ohkubo, A. (2011). A unique transcription mode of rabies virus high egg passage-Flury strain detected in infected baby hamster kidney-21 cells. Microbiol. Immunol. 55, 558–564. doi: 10.1111/j.1348-0421.2011.00354.x
Nakagawa, K., Nakagawa, K., Omatsu, T., Katayama, Y., Oba, M., Mitake, H., et al. (2017). Generation of a novel live rabies vaccine strain with a high level of safety by introducing attenuating mutations in the nucleoprotein and glycoprotein. Vaccine 35, 5622–5628. doi: 10.1016/j.vaccine.2017.08.050
Phares, T. W., Kean, R. B., Mikheeva, T., and Hooper, D. C. (2006). Regional differences in blood-brain barrier permeability changes and inflammation in the apathogenic clearance of virus from the central nervous system. J. Immunol. 176, 7666–7675. doi: 10.4049/jimmunol.176.12.7666
Prehaud, C., Coulon, P., LaFay, F., Thiers, C., and Flamand, A. (1988). Antigenic site II of the rabies virus glycoprotein: structure and role in viral virulence. J. Virol. 62, 1–7. doi: 10.1128/jvi.62.1.1-7.1988
Prehaud, C., Wolff, N., Terrien, E., Lafage, M., Megret, F., Babault, N., et al. (2010). Attenuation of rabies virulence: takeover by the cytoplasmic domain of its envelope protein. Sci. Signal. 3:a5. doi: 10.1126/scisignal.2000510
Ramakrishnan, M. A. (2016). Determination of 50% endpoint titer using a simple formula. World J. Virol. 5, 85–86. doi: 10.5501/wjv.v5.i2.85
Roy, A., Phares, T. W., Koprowski, H., and Hooper, D. C. (2007). Failure to open the blood-brain barrier and deliver immune effectors to central nervous system tissues leads to the lethal outcome of silver-haired bat rabies virus infection. J. Virol. 81, 1110–1118. doi: 10.1128/JVI.01964-06
Schnell, M. J., McGettigan, J. P., Wirblich, C., and Papaneri, A. (2010). The cell biology of rabies virus: using stealth to reach the brain. Nat. Rev. Microbiol. 8, 51–61. doi: 10.1038/nrmicro2260
Schnell, M. J., Mebatsion, T., and Conzelmann, K. K. (1994). Infectious rabies viruses from cloned cDNA. EMBO J. 13, 4195–4203. doi: 10.1002/j.1460-2075.1994.tb06739.x
Seif, I., Coulon, P., Rollin, P. E., and Flamand, A. (1985). Rabies virulence: effect on pathogenicity and sequence characterization of rabies virus mutations affecting antigenic site III of the glycoprotein. J. Virol. 53, 926–934. doi: 10.1128/jvi.53.3.926-934.1985
Sharpless, G. R., Black, J., Cox, H. R., and Ruegsegger, J. M. (1957). Preliminary observations in primary antirabies immunization of man with different types of high-egg-passage Flury virus. Bull. World Health Organ. 17, 905–910.
Shimizu, K., Ito, N., Mita, T., Yamada, K., Hosokawa-Muto, J., Sugiyama, M., et al. (2007). Involvement of nucleoprotein, phosphoprotein, and matrix protein genes of rabies virus in virulence for adult mice. Virus Res. 123, 154–160. doi: 10.1016/j.virusres.2006.08.011
Takayama-Ito, M., Inoue, K., Shoji, Y., Inoue, S., Iijima, T., Sakai, T., et al. (2006a). A highly attenuated rabies virus HEP-Flury strain reverts to virulent by single amino acid substitution to arginine at position 333 in glycoprotein. Virus Res. 119, 208–215. doi: 10.1016/j.virusres.2006.01.014
Takayama-Ito, M., Ito, N., Yamada, K., Sugiyama, M., and Minamoto, N. (2006b). Multiple amino acids in the glycoprotein of rabies virus are responsible for pathogenicity in adult mice. Virus Res. 115, 169–175. doi: 10.1016/j.virusres.2005.08.004
Takayama-Ito, M., Ito, N., Yamada, K., Minamoto, N., and Sugiyama, M. (2004). Region at amino acids 164 to 303 of the rabies virus glycoprotein plays an important role in pathogenicity for adult mice. J. Neurovirol. 10, 131–135. doi: 10.1080/13550280490279799
Tao, L., Ge, J., Wang, X., Zhai, H., Hua, T., Zhao, B., et al. (2010). Molecular basis of neurovirulence of flury rabies virus vaccine strains: importance of the polymerase and the glycoprotein R333Q mutation. J. Virol. 84, 8926–8936. doi: 10.1128/JVI.00787-10
Tian, D., Luo, Z., Zhou, M., Li, M., Yu, L., Wang, C., et al. (2016). Critical role of k1685 and k1829 in the large protein of rabies virus in viral pathogenicity and immune evasion. J. Virol. 90, 232–244. doi: 10.1128/JVI.02050-15
Tian, Q., Wang, Y., Zhang, Q., Luo, J., Mei, M., Luo, Y., et al. (2017). Rescue of a wild-type rabies virus from cloned cDNA and assessment of the proliferative capacity of recombinant viruses. Virus Gen. 53, 573–583. doi: 10.1007/s11262-017-1458-7
Tuffereau, C., Leblois, H., Benejean, J., Coulon, P., Lafay, F., Flamand, A., et al. (1989). Arginine or lysine in position 333 of ERA and CVS glycoprotein is necessary for rabies virulence in adult mice. Virology 172, 206–212. doi: 10.1016/0042-6822(89)90122-0
Virojanapirom, P., Khawplod, P., Sawangvaree, A., Wacharapluesadee, S., Hemachudha, T., Yamada, K., et al. (2012). Molecular analysis of the mutational effects of Thai street rabies virus with increased virulence in mice after passages in the BHK cell line. Arch. Virol. 157, 2201–2205. doi: 10.1007/s00705-012-1402-z
Wang, Y., Tian, Q., Xu, X., Yang, X., Luo, J., Mo, W., et al. (2014). Recombinant rabies virus expressing IFNalpha1 enhanced immune responses resulting in its attenuation and stronger immunogenicity. Virology 46, 621–630. doi: 10.1016/j.virol.2014.09.010
Wirblich, C., and Schnell, M. J. (2011). Rabies virus (RV) glycoprotein expression levels are not critical for pathogenicity of RV. J. Virol. 85, 697–704. doi: 10.1128/JVI.01309-10
Wunner, W. H., Larson, J. K., Dietzschold, B., and Smith, C. L. (1988). The molecular biology of rabies viruses. Rev. Infect. Dis. 10(Suppl. 4), S771– S784.
Yamada, K., Noguchi, K., and Nishizono, A. (2014a). Characterization of street rabies virus variants with an additional N-glycan at position 247 in the glycoprotein. Arch. Virol. 159, 207–216. doi: 10.1007/s00705-013-1805-5
Yamada, K., Noguchi, K., and Nishizono, A. (2014b). Efficient N-glycosylation at position 37, but not at position 146, in the street rabies virus glycoprotein reduces pathogenicity. Virus Res. 179, 169–176. doi: 10.1016/j.virusres.2013.10.015
Yamada, K., Park, C. H., Noguchi, K., Kojima, D., Kubo, T., and Komiya, N. (2012). Serial passage of a street rabies virus in mouse neuroblastoma cells resulted in attenuation: potential role of the additional N-glycosylation of a viral glycoprotein in the reduced pathogenicity of street rabies virus. Virus Res. 165, 34–45. doi: 10.1016/j.virusres.2012.01.002
Yang, F., Lin, S., Ye, F., Yang, J., Qi, J., Chen, Z., et al. (2020). Structural analysis of rabies virus glycoprotein reveals pH-Dependent conformational changes and interactions with a neutralizing antibody. Cell Host Microb. 27, 441–453.e7. doi: 10.1016/j.chom.2019.12.012
Yang, Y., Huang, Y., Gnanadurai, C. W., Cao, S., Liu, X., Cui, M., et al. (2015). The inability of wild-type rabies virus to activate dendritic cells is dependent on the glycoprotein and correlates with its low level of the de novo-synthesized leader RNA. J. Virol. 89, 2157–2169. doi: 10.1128/JVI.02092-14
Keywords: rabies virus, mutation, pathogenicity, glycoprotein, immunogenicity
Citation: Luo J, Zhang B, Wu Y and Guo X (2020) Amino Acid Mutation in Position 349 of Glycoprotein Affect the Pathogenicity of Rabies Virus. Front. Microbiol. 11:481. doi: 10.3389/fmicb.2020.00481
Received: 19 November 2019; Accepted: 05 March 2020;
Published: 03 April 2020.
Edited by:
Francois Villinger, University of Louisiana at Lafayette, United StatesCopyright © 2020 Luo, Zhang, Wu and Guo. This is an open-access article distributed under the terms of the Creative Commons Attribution License (CC BY). The use, distribution or reproduction in other forums is permitted, provided the original author(s) and the copyright owner(s) are credited and that the original publication in this journal is cited, in accordance with accepted academic practice. No use, distribution or reproduction is permitted which does not comply with these terms.
*Correspondence: Xiaofeng Guo, eGZndW9Ac2NhdS5lZHUuY24=