- 1School of Geography and Earth Science, Faculty of Science, McMaster University, Hamilton, ON, Canada
- 2Department of Biochemistry and Molecular Medicine, Faculty of Medicine, Université de Montréal, Montreal, QC, Canada
- 3Laboratory for the Study of Calcified Tissues and Biomaterials, Faculty of Dentistry, Université de Montréal, Montreal, QC, Canada
- 4CSIRO, Land and Water, Lucas Heights, NSW, Australia
- 5Department of Civil and Mineral Engineering, Faculty of Applied Science and Engineering, University of Toronto, Toronto, ON, Canada
Here, we experimentally expand understanding of the reactions and enzymes involved in Acidithiobacillus thiooxidans ATCC 19377 S0 and metabolism by developing models that integrate gene expression analyzed by RNA-Seq, solution sulfur speciation, electron microscopy and spectroscopy. The A. thiooxidans metabolism model involves the conversion of to , S0 and mediated by the sulfur oxidase complex (Sox), tetrathionate hydrolase (TetH), sulfide quinone reductase (Sqr), and heterodisulfate reductase (Hdr) proteins. These same proteins, with the addition of rhodanese (Rhd), were identified to convert S0 to , and polythionates in the A. thiooxidans S0 metabolism model. Our combined results shed light onto the important role specifically of TetH in metabolism. Also, we show that activity of Hdr proteins rather than Sdo are likely associated with S0 oxidation. Finally, our data suggest that formation of intracellular is a critical step in S0 metabolism, and that recycling of internally generated occurs, through comproportionating reactions that result in . Electron microscopy and spectroscopy confirmed intracellular production and storage of S0 during growth on both S0 and substrates.
Introduction
The stepwise oxidation of reduced sulfur species from sulfide to sulfate can occur via several pathways involving a variety of sulfur oxidation intermediate (SOI) compounds that are dynamically influenced by environmental and geochemical characteristics as well as the microbes involved (Schippers et al., 1996; Schippers and Sand, 1999; Nordstrom, 2015). This range of sulfur oxidation states contributes to a complex, and only partially constrained biogeochemical cycle in which sulfur compounds can be variably reduced, oxidized and disproportionated via abiotic and/or biotic processes depending on environmental conditions (Johnston and McAmish, 1973; Kelly and Baker, 1990; Pronk et al., 1990; Druschel, 2002; Zopfi et al., 2004; Bernier and Warren, 2007; Boyd and Druschel, 2013). The geochemical challenges to closing the sulfur biogeochemical cycle reflect the existence of multiple semi-stable SOI compounds, which are either not comprehensively constrained to date and/or lack readily available analytical methods for their characterization (Miranda-Trevino et al., 2013). For instance, the challenges in measuring polythionates and other higher oxidation state sulfur compounds have impeded the delineation of their roles in the chain of reactions culminating in sulfate (Johnson and Hallberg, 2003; Nordstrom et al., 2015). The complexities of sulfur chemistry underscore the need for mass balance of all sulfur within systems, in order to quantify how much sulfur may be tied up in a currently unidentified or, as referred to here, “other SOI” pool. However, sulfur mass balance is rarely employed in studies of sulfur cycling.
Further, microbial catalysis, dependent on the specific bacteria, growth stage and sulfur substrates involved, is important for initiating or accelerating rates for some of these sulfur oxidation reactions (Bacelar-Nicolau and Johnson, 1999; Druschel et al., 2004; Bernier and Warren, 2005, 2007; Beller et al., 2006; Warren et al., 2008; Bobadilla Fazzini et al., 2013). Several studies have demonstrated flexibility of the sulfur oxidation metabolism by assessing the solution chemical changes in some intermediate sulfur species, or inferred pathways from what is known about identified sulfur metabolism genes within an organism or community (Bobadilla Fazzini et al., 2013; Jones et al., 2014; Yin et al., 2014; Houghton et al., 2016). Intermediate species of sulfur, especially S0, , and polythionates [ (n > 2)], are important in microbial processing of sulfur, even though their concentrations in solution may be low. Indeed, these intermediate sulfur compounds are thought to be involved in the so-called “cryptic” sulfur cycle, an enigmatic process in which sulfur is recycled amongst lower state sulfur species that is not well-characterized to date (Thamdrup et al., 1994; Jørgensen and Nelson, 2004; Canfield et al., 2010; Houghton et al., 2016).
Further, gaps in understanding of which proteins catalyze specific sulfur pathways also exist (Friedrich et al., 2001; Sauvé et al., 2007; Valdes et al., 2011; Jones et al., 2014). The literature to date indicates that some sulfur metabolic enzymes catalyze a broad suite of sulfur oxidative reactions, e.g., the Sox (sulfur oxidizing) complex, while others seem to catalyze more specific sulfur reactions, e.g., Sdo (sulfur dioxygenase) (Kelly et al., 1997; Friedrich et al., 2001; Rohwerder and Sand, 2003; Hensen et al., 2006; Sauvé et al., 2007; Wang et al., 2019). Some microorganisms capable of sulfur oxidation can possess a suite of these genes, enabling them to carry out many different reactions, while others have a more limited set of sulfur genes, restricting them to select reactions only (Hallberg and Johnson, 2003; Ghosh and Dam, 2009; Zhu et al., 2012; Nuñez et al., 2017). Recent works reviewing Acidithiobacillus spp. sulfur metabolism have identified diverse pathways for this genus dependent on the species, as well as the sulfur substrate(s) (S0, , ) and the different sulfur metabolism genes available to them (Wang et al., 2019; Zhan et al., 2019). These studies have provided updated models for A. caldus and A. ferrooxidans based on the existing literature of studies using either genomics, proteomics or sulfur chemistry analyses. For both species, S0 metabolism is proposed as oxidation to via Sdo, followed by oxidation to via the sulfate adenylyltransferase dissimilatory-type (SAT) gene (Wang et al., 2019). While the metabolism is proposed to differ between the two species, where in A. caldus it is through the S4I pathway and Sox complex, and in A. ferrooxidans via the S4I pathway and thiosulfate dehydrogenase (TSD) (Wang et al., 2019; Zhan et al., 2019). The S4I pathway utilizing the doxD (thiosulfate:quinone oxidoreductase) and tetH (tetrathionate hydrolase) genes (Wang et al., 2019). While further notable genes present in the sulfur metabolism for Acidithiobacillus spp. include the sqr (sulfide quinone reductase), sor (sulfur oxygenase reductase), rhd (rhodanese) and the heterodisulfide reductase or Hdr-like complex (hdrA, hdrB, and hdrC) (Ghosh and Dam, 2009; Valdes et al., 2011; Jones et al., 2014; Yin et al., 2014; Wang et al., 2019).
Here, the objectives were to characterize both the levels of gene expression at high resolution (RNA-Seq) for Acidithiobacillus thiooxidans, and the changes in sulfur speciation associated with its experimental growth on either S0 or to generate models for A. thiooxidans sulfur metabolism. A. thiooxidans is a strict autotroph only able to carry out sulfur oxidation/disproportionation reactions (Figure 1A) and a well-studied sulfur oxidizing microorganism (Kelly et al., 1997; Suzuki et al., 1999; Masau et al., 2001; Rohwerder and Sand, 2003). The model organism A. thiooxidans ATCC 19377 used here, encodes at least 10 known proteins or protein complexes thought to be involved in sulfur metabolism, which includes the aforementioned S4I pathway and Sox complex in the periplasm, and the Hdr-like complex in the cytoplasm (Valdes et al., 2011; Bobadilla Fazzini et al., 2013; Yin et al., 2014) (Figure 1B). Our integrated approach provides important novel insights since previous studies have designed models for this species based solely on solution chemistry (Bobadilla Fazzini et al., 2013) or gene expression (Yin et al., 2014).
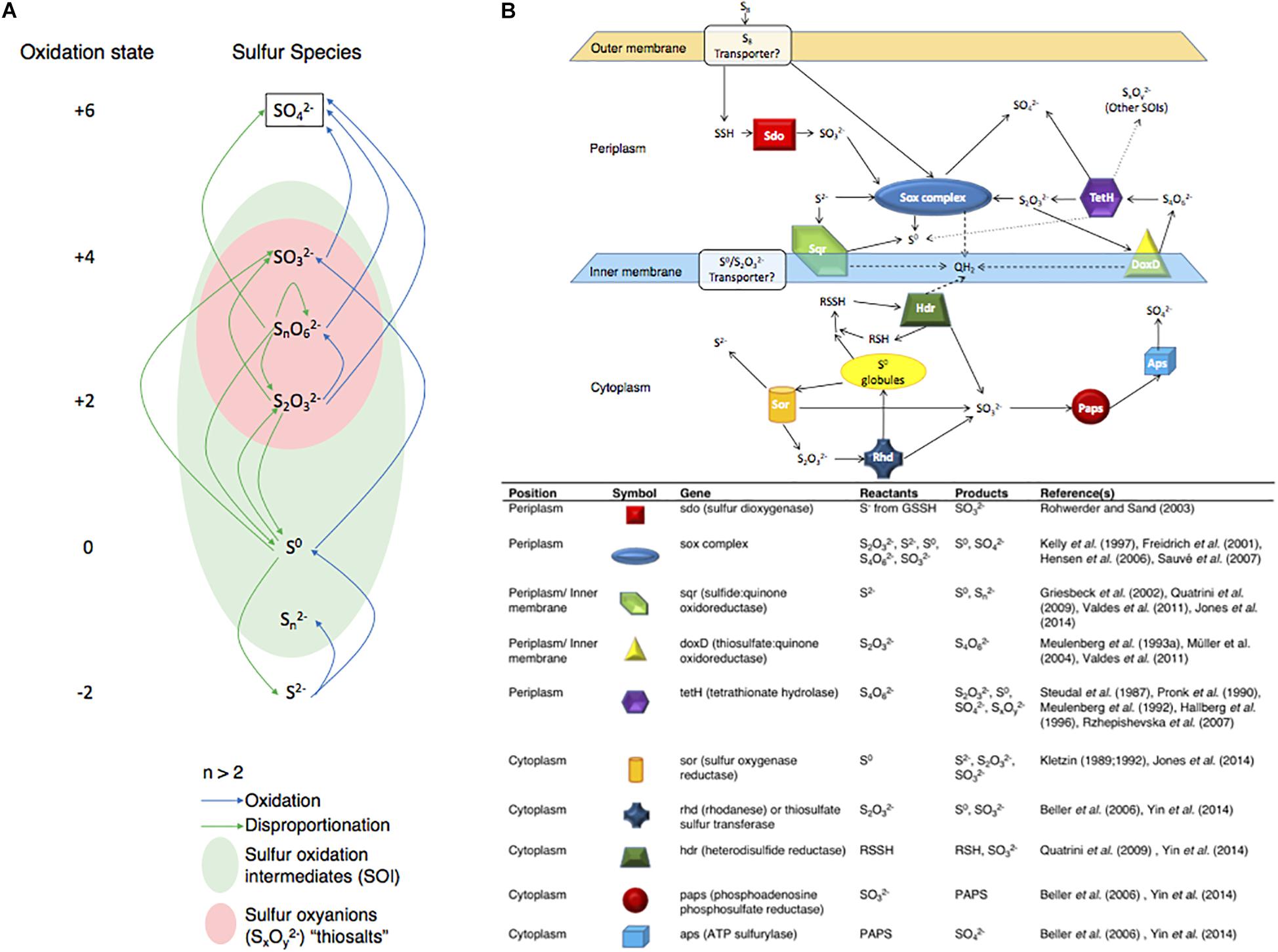
Figure 1. Biological sulfur capabilities and gene layout for Acidithiobacillus thiooxidans. (A) Biological sulfur reaction capabilities across all known sulfur species and oxidation states and (B) a theoretical map for the locations of sulfur genes analyzed and information on potential reactants and products of these genes. Showing proven sulfur reactions in solid arrows, theorized reactions in dotted arrows and cytochrome C proton reactions in dashed arrows. Based on the referenced literature.
Materials and Methods
Experimental Design, Cell Growth, and Counting
Experimental Design
In order to jointly assess both gene expression and changes in sulfur speciation, the experimental design included collection of samples for cell counts, gene expression, microscopy, S speciation and pH for A. thiooxidans grown in both S0 and treatments over 12 days to ensure both exponential and stationary phases were encompassed in the characterization. Greater details on collection and analyses of samples for each of these variables are provided subsequently.
Culture Conditions
Acidithiobacillus thiooxidans ATCC 19377 cells were grown in liquid elemental sulfur or thiosulfate media (Staley et al., 1989). The media contained two components, the salt medium and the sulfur source. Elemental sulfur salt medium: (NH4)2SO4, 0.2 g; MgSO4 × 7 H2O, 0.5 g; CaCl2 × 2 H2O, 0.331 g; KH2PO4, 3.0 g; FeSO4 × 7 H2O, 9.15 mg; distilled water, 1,000 ml. The salt medium was sterilized by passing through a 0.22 μm filter. Elemental sulfur powder was heated in an oven at 100°C for 30 min and the cycle was repeated three times. The salt medium was then added to the culture flasks and the final sulfur concentration was 1% (m/v). Thiosulfate medium: salt medium as above and Na2S2O3 was added at 0.2% (m/v), followed by filter sterilization (0.22 μm filter). For both cultures, the total volume of medium corresponded to a fifth of the total volume of the Erlenmeyer flask. All cultures were initially inoculated at 5% v/v with cultures pre-grown in the corresponding media; the inoculant bacteria were washed with sterile 1% NaCl solution prior to inoculation. All cultures were grown under aerobic conditions at 30°C and flasks were shaken at 120 rpm.
Fluorescence-Activated Cell Sorting (FACS)
Cells were harvested at the desired time points (days 1, 2, 3, 4, 5, 8, 10, and 12) and washed with 1% NaCl. Optical density (O.D.) values were determined to generate cell counts; however errors introduced by S0 clumping precluded their use for these experiments. Thus, for the growth curves, 2 μl of the Live/Dead marker mixture of component A and component B at a ratio of 1:1 (L7012 LIVE/DEAD® BacLight, Bacterial Viability Kit, Thermo Fisher Scientific) were added to 1.5 ml of bacterial suspension. The rationale behind the Live/Dead stain is that all cells will be stained green, because SYTO 9 penetrates into live and dead cells and stains their DNA, whereas propidium iodide (red stain) penetrates only into dead or damaged cells with leaky membranes staining their DNA. For the negative control (dead cells), the cells were first washed with 1% NaCl and then incubated in 70% ethanol for 1 h, followed by washing with 1% NaCl. Propidium iodide (Component B) was added (0.66 μl for 1 ml of bacterial suspension). For the positive control, 0.66 μl of SYTO 9 (Component A) was added to 1 ml of bacterial suspension. All samples were incubated in the dark at room temperature for 15 min, followed by counting in a FACS BD Canto II instrument. Experiments were conducted in triplicates.
Genetic Methods and Analyses
DNA Purification
Genomic DNA was purified from cells from 50 ml bacterial culture grown on elemental sulfur by manual cell disruption with a pestle in the presence of small glass beads (<106 μm diameter; sufficient to form a thick paste). Genomic DNA was purified from combined washes with TE buffer (10 mM Tris, 1 mM EDTA, pH 8) following essentially the instructions of the Qiagen Genomic G20 kit, resulting in 10 μg of purified total DNA.
Illumina DNA Sequencing
For paired-end Illumina sequencing (MISEQ-PE300, i.e., 300 nucleotides read length), a TruSeq library was constructed with sized DNA fragments (570 to 650 bp). The reads received from the sequencing service (McGill and Génome Québec Innovation Centre; Montreal, QC, Canada) were cleaned from adapters and quality-clipped with the Trimmomatic software (Bolger et al., 2014), resulting in a total of 2,254,174 read pairs. In addition, a Nextera mate-pair library (insert size 7–8 kbp) was sequenced on two flow cells of Illumina HISEQ (rapid mode; 150 nucleotides read length), and cleaned with Trimmomatic (8,224,769 read pairs).
Genome Assembly and Annotation
The genome was assembled with Spades v. 3.6.1 (Bankevich et al., 2012) using a coverage cutoff value of 3.0. The resulting set of contigs was annotated with Prokka v.1.13.3 (Seemann, 2014).
Total RNA Extraction
Cells were harvested on day 3 (exponential phase; pH 2.5) and 5 (stationary phase; pH 1.5) for S0 media and day 5 (stationary phase; pH 2.5) for media, and washed with ice-cold NaCl 1%. They were then lysed and total RNA was extracted using the High Pure RNA Isolation Kit (Roche). Instead of 4 μl of lysozyme as indicated in the kit, 20 μl were added to efficiently break the cells. The lysozyme solution was prepared from egg white lysozyme (Bio Basic, Inc.; activity: 20,000 U/mg) at a final concentration of 50 mg/ml. The genomic DNA was removed using the TURBO DNA-free KitTM (Ambion). The concentration of total RNA was determined using a Nanodrop instrument and the quality of the preparation was assessed by agarose gel electrophoresis to monitor 16S and 23S ribosomal RNA. Samples were conserved at −80°C; experiments were conducted in biological triplicates.
High-Throughput RNA Sequencing and Bioinformatics
Sequencing was done using Illumina Hi-seq technology (100 bases paired-end). Quality controls, DNA library construction from isolated RNA and sequencing were performed at the Génome Québec Innovation Centre (Montreal, QC, Canada). Bioinformatics analysis was done using software available on the Galaxy server1 (Giardine et al., 2005; Blankenberg et al., 2010; Goecks et al., 2010). Full-length reads (100 bases) were trimmed so that only portion 11 to 80 of each read was conserved. Quality control of the reads was done using FastQC (Galaxy Tool Version 0.63) before and after trimming to ensure quality of the reads. The quality format was changed to “Sanger & Illumina 1.8 +” using FASTQ Groomer (Galaxy Tool Version 1.0.4). Reads were mapped as paired-end using Tophat (Galaxy Tool Version 0.9). The mean inner distance between mate pairs was set to 150 bases and the standard deviation to 20. The reference genome of A. thiooxidans (Valdes et al., 2011) was used as guide to help align the reads and the defaults parameters of Tophat were selected. Finally, differential expression was analyzed using Cufflinks (Galaxy Tool Version 2.2.1.0). The “max intron length” was set to 300,000, the “min isoform fraction” was set to 0.1 and the “pre mRNA fraction” to 0.15. Cufflinks only counted fragments compatible with the reference annotation of the genome and it performed a biased correction using the genome assembly. Default Cufflinks parameters were selected.
Sulfur Chemistry Methods and Analyses
Biogeochemical Experiments
Nine sterile 1 L flasks were prepared for batch experimentation: six containing salt medium with 1% S0 and three with 0.2% culture medium, followed by A. thiobacillus inoculation as described above. For each treatment, one flask was sacrificed for sulfur chemical analyses from the S0 cultures on days 0, 1, 2, 3, 4, and 5 and from the cultures on days 0, 2, and 4. For each sampling time, the bulk solution pH was measured (Denver Instrument Model 225, Bohemia, NY, United States) prior to sampling for sulfur analyses. Triplicate samples were then collected for dissolved (<0.45 μm), total sulfur (ΣSaq) and sulfur speciation (, S2–, , S0, and ) analyses as described subsequently.
ΣSaq – Determination by ICP-AES
For total S (ΣSaq), 40 ml of water samples were filtered by Pall Acrodisc® 25 mm 0.45 μm Supor® membrane via polypropylene syringes into 50 ml FalconTM tubes, followed immediately by addition of 80 μL of HNO3 (Optima grade, Fisher Chemical) to each tube before storing at 4°C until analyses. To enable sulfur mass balance calculations, ΣSaq analyses were performed by inductively coupled argon plasma emission spectrometry (ICPAES) (Varian730 ES, Mulgrave, VIC, Australia) using the operating conditions recommended by the manufacturer. Sulfur calibration standards were prepared from certified reference stock solutions (AccuStandard, New Haven, CT, United States) in 2% v/v HNO3. The limit of detection (LOD) for sulfur was 1 mg L–1 (calculated as three times the standard deviation of the mean blank). Subtracting the sum of all measured solution sulfur species concentrations, described subsequently (, S2–, , S0, and ) from the total sulfur (ΣSaq) concentration, allowed us to quantify the concentration of S occurring within an unresolved or “Other” SOI pool.
and S2– – Determination by Spectrophotometry
At each sampling time point, samples were immediately fixed and analyzed using the HACH SulfaVer 4 Method and Methylene Blue Method for and S2–, respectively (Hach Company, Loveland, CO, United States) by spectrophotometry (Pharmacia Biotech Ultrospec 3000 UV/Visible Spectrophotometer).
, S0, and – Determination by HPLC
Sampling and analyses for individual SOI species , S0, and were concomitant with those for total S, ΣSaq, and redox end members, and S2–. At each sampling time point, samples were taken and immediately preserved using a monobromobimane derivatization procedure for SOI analyses by HPLC (Rethmeier et al., 1997). The Alltima HP C18 (5 μm × 150 mm × 4.6 mm) reverse phase column and Shimadzu LC-20AD prominence HPLC instrument were used for all SOI analyses. Solvents used in protocols were: A = Water, B = Methanol, C = Acetonitrile, D = Acetic acid 0.25% v/v pH 3.5 adjusted with NaOH (1N). and were assessed via fluorescence excitation at 380 nm and emission at 480 nm. Standards and calibrations for (0–10 mM) and (0–1.7 mM) were made with and , respectively. The thiosulfate and sulfite elution protocol was as follows: 0–1 min, 1 ml/min flow; 1–6 min, 1 to 0.85 ml/min flow linear gradient; 0–8 min B 35%, D 65% to B 40%, D 60% linear gradient, oven heated to 35°C. Sample size was 5 μl and elution times were 3 min for and 6.5 min for . S0 was extracted with chloroform from both filtered (<0.45 μm, i.e., colloidal) and unfiltered samples (i.e., particulate and/or colloidal) and analyzed with reverse-phase HPLC and UV-absorption at 263 nm. Standards and calibrations (0–32 mM) were made from S0 dissolved in chloroform. S0 elution protocol: 1 ml/min flow, B 65%, C 35% isocratic; the sample size was 10 μl and the elution time was at 5 min.
Microscopy and Spectroscopy Analyses
Transmission Electron Microscopic (TEM) Analysis
25 ml cultures of bacteria were grown in 1% S0 or 0.2% media, respectively. Cells were sedimented and rinsed three times with 0.1M phosphate buffer at pH 7.2 to eliminate the remaining medium. Cells were fixed with 4% paraformaldehyde (Acros Organics, Morris Plains, NJ, United States) and 0.1% glutaraldehyde (Electron Microscopy Sciences, Fort Washington, PA, United States) for 30 min at 4°C, followed by three wash with 0.1M phosphate buffer before osmification using 1% osmium tetroxide for 1 h at room temperature. The pellets were dehydrated using a graded ethyl-alcohol series and then processed for embedding in epon (Marivac, Halifax, NS, Canada). Ultrathin sections of 80–100 nm thickness were cut with a diamond knife, collected on Formvar-carbon (polyvinyl formate) coated 200-mesh nickel grids. Sections were then stained with 2% uranyl acetate and lead citrate and examined with a FEI Tecnai 12 (Eindhoven, Netherlands) transmission electron microscope operating at 80 kV.
Energy-Dispersive X-Ray Spectroscopy and Wavelength-Dispersive Spectroscopy Analysis
Bacterial sections were imaged using a transmission electron microscope (Jeol JEM-2100F, JEOL, Ltd., Tokyo, Japan) equipped for elemental analysis by energy-dispersive X-ray spectroscopy (EDS). In addition, a scanning electron microscope (Jeol JSM-7600F, JEOL, Ltd., Tokyo, Japan) was used for wavelength dispersive X-ray Spectroscopy (WDS) analysis to obtain a better isolation of the peaks of interest for quantitative analysis.
Statistical Analyses
Growth curve and pH results for the two treatments were compared by t-test analyses: paired two samples for means via Microsoft Excel 2016, with each treatment having three replicates per data point. RNA-seq analysis is a whole genome approach allowing the detection of low and highly expressed genes using the parameter fragments per kilobase of transcript per million mapped reads (FPKM), and the standard deviations between each treatment’s triplicates. Further analyses on FPKM values was carried out to make pairwise comparisons using independent t-test on the FPKM between RNA-seq experiments and for the relative levels of gene expression based on Log2 values between samples for the suite of known sulfur genes: (1) across growth curve stage within the S0 media, (2) between S0 and media at the same solution pH and (3) at the same growth curve stage via Microsoft Excel 2016. The chemical relationships between the different S species and [H+] (pH) were tested using ANOVA regression statistics via Microsoft Excel 2016 and significance of p-value < 0.05 are stated. Intracellular S0 globules were analyzed after TEM to determine the quantity and size of globules found inside the cells using Image J software.2 Manual modeling and stoichiometric balancing methodology is presented in Supplementary Text.
Results
Growth, pH, and Sulfur Species Related to Gene Expression
We cultivated A. thiooxidans on minimal media with S0 or as the source of energy. The results indicate that the organism can extract energy with equal efficiency from both compounds, as evidenced by statistically identical growth patterns for the two media (p < 0.05) (Figure 2A). However, the amount of acid generated was higher in the S0 media (final pH of 1.5 compared to 2.5 in the media) with a corresponding higher slope of pH decrease (0.68 vs. 0.45) as compared to the results on media over the experimental time period (days 0–5) (Figure 2B). These results indicate A. thiooxidans catalyzes sulfur substrate-dependent metabolic reactions, which may correspondingly be reflected in differential gene induction profiles.
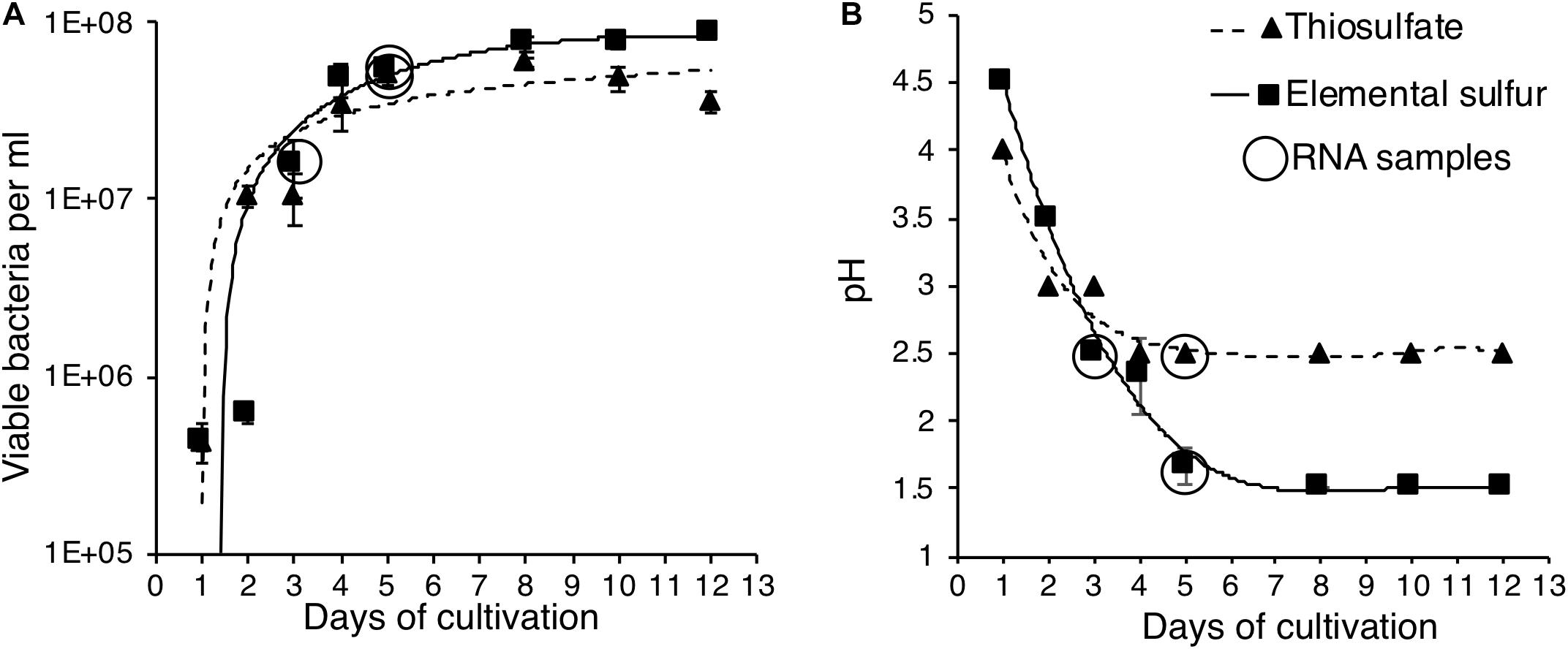
Figure 2. Identification of growth and changes of pH for A. thiooxidans grown with alternative energy sources. (A) Growth curves for A. thiooxidans grown with S0 (squares, solid line) and (triangles, dotted line) as energy source. (B) Changes of pH in the media for A. thiooxidans grown with S0 (squares, solid line) and (triangles, dotted line) as energy source. Time points of samples analyzed by RNA-Seq analyses are indicated by circles. The data are the result of analyses conducted in triplicates and where not visible, error bars for pH measurements were smaller than the symbols plotted for mean pH values.
Genomic Analyses
Sequencing, Assembly, and Annotation of the A. thiooxidans Genome
To correlate the results of the analysis of sulfur species in the medium with expression of the sulfur metabolism genes using RNA-seq we first needed to generate a more robust genome sequence than the available draft version (Valdes et al., 2011). The published draft genome sequence (GenBank: AFOH01000000) has 164 contigs at low coverage and a total genome size of 3,019,868 bp, which may lead to incomplete transcriptome analyses. For this reason, we re-sequenced the genome of A. thiooxidans ATCC 19377 and Table 1 shows the characteristics of the assembly comprising 22 unique contigs and a total of 3,404,101 bp (almost 13% larger than previously published), with the largest contig (2,390,830 bp) spanning 70% of the total sequence. Two contigs have a highly elevated genome coverage, most likely representing circular plasmids. 27 small contigs (size range between 129 and 7,095 bp) carry polymorphic sites and are therefore not counted in the total genome size but included in the GenBank submission. This Whole Genome Shotgun project has been deposited at DDBJ/ENA/GenBank under the accession SZUV00000000. The version described in this paper is version SZUV01000000. A significantly larger fraction of RNA-seq reads (92% for all growth conditions) aligned to our new genome assembly as compared to the previous draft (29–60%) showing that the quality of assembly was greatly improved over the published GenBank record (Table 2). Gene annotation identified all known genes encoding enzymes of sulfur metabolism such as sdo (sulfur dioxygenase), the Sox (sulfur oxidation) complex (soxA, soxB, soxX, soxY, and soxZ), sqr (sulfide quinone reductase), doxD (thiosulfate:quinone oxidoreductase), tetH (tetrathionate hydrolase), sor (sulfur oxygenase reductase), rhd (rhodanese), the heterodisulfide reductase (hdrA, hdrB, and hdrC), paps (phosphoadenosine phosphosulfate reductase) and aps (ATP sulfurylase) (Figure 3 and Supplementary Tables S1–S4) (Kletzin, 1989, 1992; Griesbeck et al., 2002; Rzhepishevska et al., 2007; Valdes et al., 2008; Quatrini et al., 2009; Valdes et al., 2009; Mangold et al., 2011; You et al., 2011). The genome contains three copies of sdo, two operons encoding the Sox complex, two copies of rhd and three copies of hdrA. The plasmids apparently do not code for genes that are of interest in this context, with the potential exception of a gene for a “divalent metal cation transporter” (MntH), which may have been recruited via a plasmid to manage the high metal ion concentrations in its natural environment.
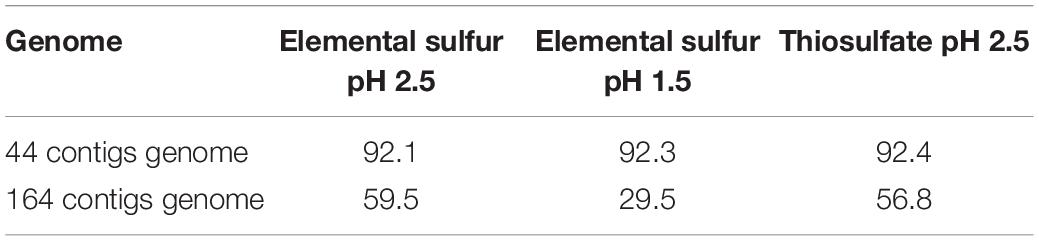
Table 2. Comparison of the percentage of concordant pair alignments between RNA-seq data and the new assembly of the A. thiooxidans ATCC 19377 genome and the published draft genome with 164 contigs.
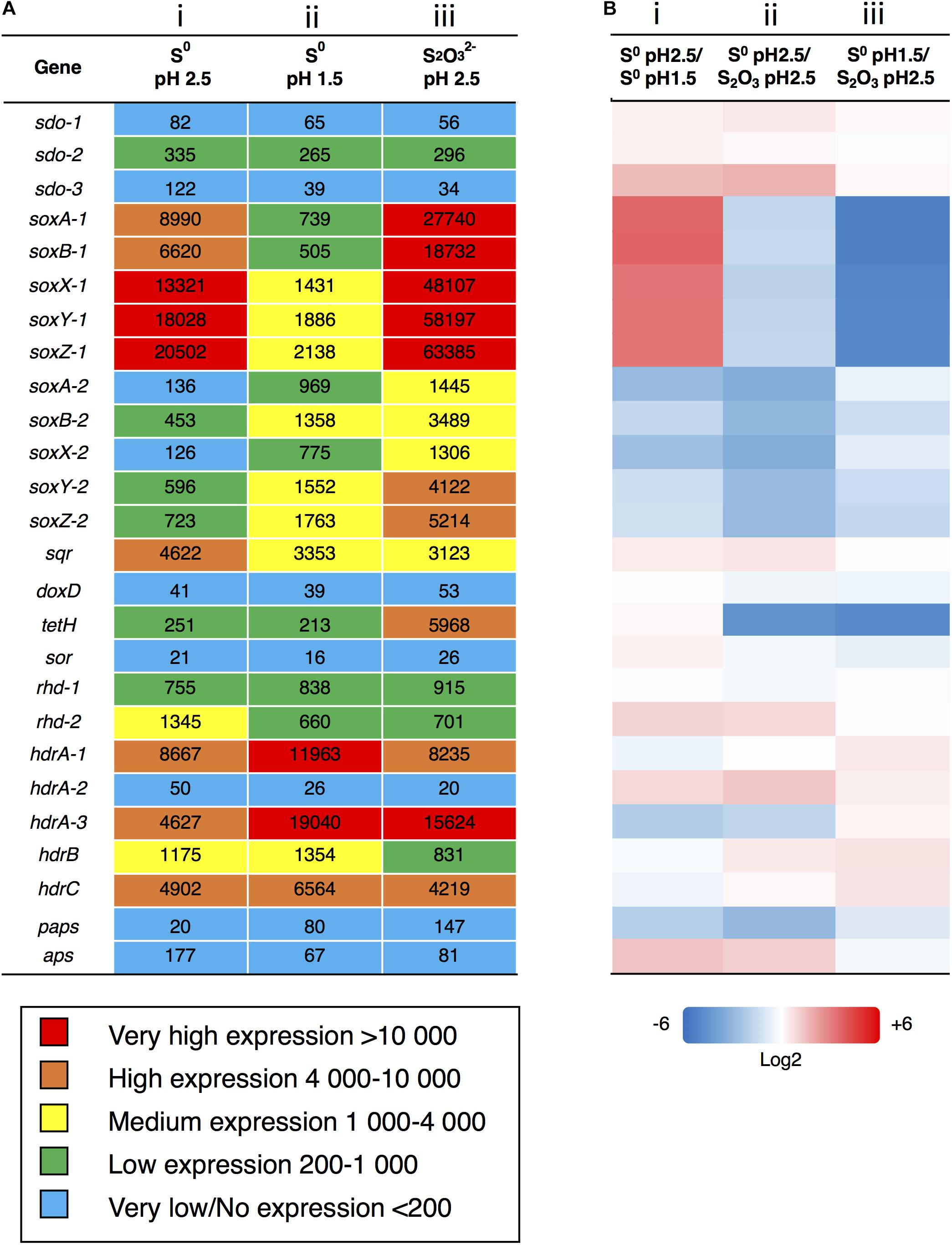
Figure 3. Analysis of gene expression after growth with S0 or as energy source. (A) Gene expression based on FPKM values was analyzed after growth on S0 to (i) pH-value 2.5 and at (ii) 1.5 and on (iii) to pH-value 2.5. Color scale against indicates relative expression values with blue being the very low, green is low, yellow is intermediate, orange is high and red represents very highly expressed genes. (B) Comparative gene expression for FPKM values based on Log2 ratio. (i) Growth on same substrate (S0) at different points on pH and growth curve (pH 2.5 = day 3/pH 1.5 = day 5), (ii) growth to same pH (2.5) on different substrates and points on growth curve (S0 = day 3/ = day 5), (iii) growth until day 5 on different substrates and to different pH values (S0 = pH 1.5/ = pH 2.5). Color scale against each comparison test based on Log2 values; blue = –6 (i.e., numerator expressed less than denominator), white = 0 (i.e., expression equal), red = + 6 (i.e., numerator expressed more than denominator).
Expression Analysis of the Sulfur Metabolism Genes Using RNA-Seq
For transcriptome analysis, we collected total RNA from cultures of A. thiooxidans grown on elemental S0 and on media (three biological replicates) to compare gene expression on two differing oxidation state sulfur substrates. RNA-Seq analysis is a whole genome approach allowing the detection of low and highly expressed genes using the parameter fragments per kilobase of transcript per million mapped reads (FPKM) [Sequence Read Archive (SRA) accession: PRJNA541131]. To assess the quality of mapping of the RNA-Seq sequences on the genome assembly, we compared the percentage of concordant pair alignments using the same raw RNA-Seq data and the two available genomes [our new assembly and the previously published draft genome (Valdes et al., 2011)]. We observed an increase of more than 30% of the total concordant pair alignments of the RNA-Seq data for the newly assembled genome for each individual sample as compared to the draft (Table 2). These data underline the quality of the new genome assembly that was used for all the following analyses. A direct representation of the FPKM values, i.e., relative expression levels for the three growth conditions (exponential and stationary growth phases on S0 and stationary growth phase on ) is shown in Figure 3A. FPKM values under 200 are interpreted as low to no expression, as compared to low expression (200–1,000 FPKM), medium expression (1,000–4,000 FPKM), high (4,000–10,000 FPKM), and very highly expressed (more than 10,000 FPKM).
The genes encoding the Sox complex (soxA, B, X, Y, Z) are generally highly expressed, but interestingly the relative expression of the two sox operons changes during growth on elemental sulfur at pH 2.5 (day 3) and pH 1.5 (day 5); sox-1 strongly decreases and sox-2 increases to medium levels. In contrast, the sox-1 operon is very highly expressed during growth on and we also observe medium to high expression of the sox-2 operon showing the importance of the gene products under this condition.
The sqr gene is medium to highly expressed in all three conditions at comparable levels suggesting that the gene product sulfide quinone reductase also plays an important role in A. thiooxidans S metabolism. Other genes are relatively weakly expressed, and whereas there is some variation of gene expression, it is difficult to assess whether they provide major contributions to sulfur metabolism under these conditions (aps, doxD, sor, and paps). We observe low expression of the rhd gene and medium to very high expression of hdrA, hdrB, hdrC genes under all conditions. In the case of sdo, encoding sulfur dioxygenase required for the entry of elemental sulfur into the cell, the expression of one copy is low under all conditions, whereas two gene copies are below 200 FKPM values (Figure 3A).
Further Pairwise Expression Analysis of the Sulfur Metabolism Genes Using RNA-seq
Expression of most of the A. thiooxidans sulfur genes (with exceptions of the sox-2 operon, hdr, all but hdrA-2, and paps genes) was higher on day 3 during exponential growth on S0 media, as compared to day 5 when cells were in the stationary phase (Figure 3B-i). It thus appears that A. thiooxidans exhibits greater metabolic variability in the genes involved, producing higher oxidation state sulfur species (e.g., polythionates) (Figure 1A), during exponential phase, which shifts during stationary phase to a greater processing of polythionates and decreasing pH values (Figure 2). In addition, hdrA-1 and hdrA-3 expression strongly increases at pH 1.5 as compared to pH 2.5 during growth on sulfur, suggesting an increased importance of heterodisulfide reductase in the late growth phase. In contrast, the tetrathionate hydrolase encoding gene (tetH) is highly expressed only during stationary growth on thiosulfate (day 5), suggesting that this protein plays a specific role in growth on this SOI compound.
Acidithiobacillus thiooxidans gene expression also differed between the two growth media, when an identical pH of 2.5 had been reached. Higher expression levels of the sox complex, tetH, hdrA-3, and paps genes were observed for growth on (day 5, stationary phase), whilst higher expression levels of the all the sdo copies, sqr, rhd-2, aps and all the hdr genes except hdrA-3 were observed during growth on S0 (day 3, exponential phase) (Figure 3B-ii). Gene expression levels also differed for day 5 (stationary phase) for A. thiooxidans growth in the two sulfur media (Figure 3B-iii): all sulfur genes with the exceptions of sdo-1, sdo-3, sqr and all hdr genes were more highly expressed when grown on compared to growth on S0.
Genome Wide Analysis of Gene Expression
While the analysis of sulfur genes is vital to the comprehension of autotrophic metabolism, the analysis of the complete transcriptome may lead to the identification of genes that are correlated with this metabolic adaptation. To this effect, we conducted pairwise comparisons of relative gene expression levels (FPKM values) to identify additional up- and down-regulated genes. Analysis of gene expression after growth on elemental sulfur at pH 2.5 compared to pH 1.5 (Supplementary Figure S1a), showed that 20% of the genes (660) are upregulated and 12% (404) are downregulated. The top 50 upregulated genes with the highest degree of differential expression are presented in Supplementary Table S5; several of these genes encode chemotaxis and flagellar components. We also analyzed the top 50 downregulated genes and most encode hypothetical proteins (Supplementary Table S6). Analysis of gene expression after growth on elemental sulfur at pH 2.5 compared to thiosulfate pH 2.5 (Supplementary Figure S1b), shows that 18% (594) are upregulated and 8% (269) are downregulated. The top 50 upregulated genes comprise genes encoding chemotaxis components as well as ATP synthase subunits (Supplementary Table S7). We analyzed the top 50 downregulated genes finding hypothetical proteins as well as transcription factors involved in osmoregulation as well as proteins cytochrome C biogenesis among them (Supplementary Table S8). Finally, analysis of gene expression after growth on thiosulfate at pH 2.5 compared to elemental sulfur at pH 1.5 (Supplementary Figure S1c) shows that 8% of the genes are upregulated (271) and 11% are downregulated (347). The top 50 upregulated genes comprise genes encoding components of cytochrome C biogenesis and of proteins involved in protein folding and outer membrane stability (Supplementary Table S9). Analysis of the top 50 downregulated showed that most encode hypothetical proteins (Supplementary Table S10). Further discussion on these broader metabolic characteristics can be found in Supplementary Text.
Insights Into Sulfur Pathways Catalyzed by A. thiooxidans Grown on S0 and
Consistent with the notion that A. thiooxidans catalyzes sulfur substrate-dependent metabolic reactions suggested by differential acid production (Figure 2), solution sulfur speciation also differed in the two growth media (Figure 4). A. thiooxidans growth on S0 resulted in relatively higher concentrations of produced Other SOI (i.e., unresolved S species; 25.3 mM versus 6.9 mM on ) and (13.7 mM versus 7.8 mM on ; Figures 4A,B), while growth on resulted in near equal generation of Other SOI, sulfate and S0 (Figures 4A,B). Further, S2– and were largely non-detectable in solution, with the exception of a very low amount of on day 5 in the S0 growth experiment (Supplementary Table S11), while both sulfur species were detected at low concentrations (<0.5 mM) throughout growth on (Supplementary Table S11).
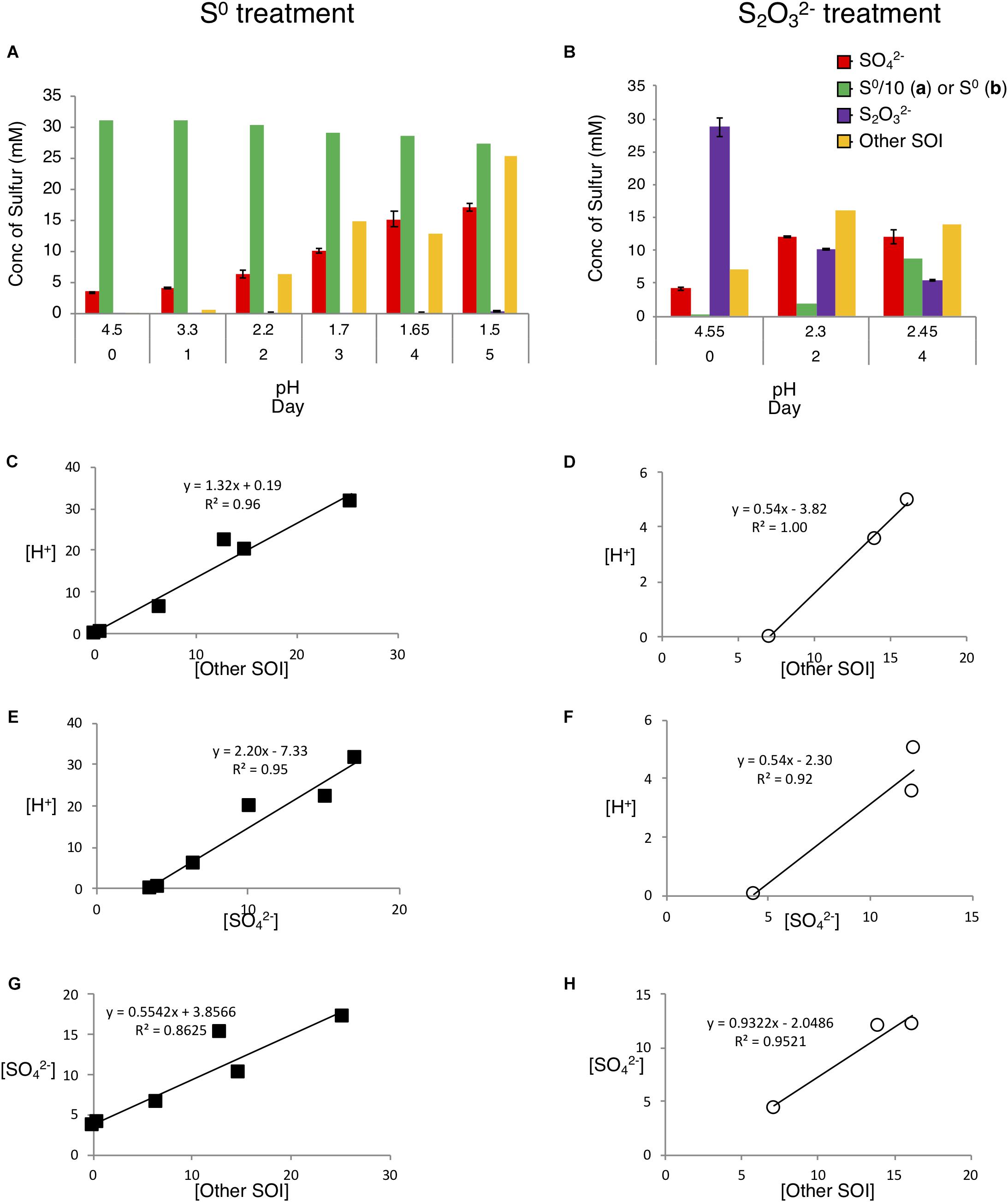
Figure 4. Analysis of sulfur chemistry after growth with S0 or as energy source. A. thiooxidans was cultivated with different substrates for up to 5 days, followed by determination of the production of different sulfur species in the media and comparison of [H+] production to S species on S0 media (filled squares) and media (empty circles). (A) Production of sulfur species during growth on S0. (B) Production of sulfur species during growth on . (C,D) [H+] production vs. [Other SOI], (E,F) [H+] production vs. [], (G,H) [ ] production vs. [Other SOI]. The difference in S0 concentration scale between figures, where in (A) the value shown is in 1/10 actual value. Concentrations of all S species are given in mM in mol of S (e.g., 1 mM of = 1 mm S, while 1 mM = 2 mM S).
Sulfur mass balance identified that concentrations of unresolved sulfur species, Other SOI, occurred at appreciable levels under both growth conditions (Figures 4A,B). This Other SOI pool may variably comprise a number of possible sulfur intermediate oxidation compounds, such as species associated with oxidation pathways, i.e., polythionates, as well as products of disproportionation reactions, i.e., polysulfides. While our results do not identify the specific species sulfur species occurring within this pool, insights provided through analysis of the relationships between changes in (1) [Other SOI] and (2) [ ] to [H+] (Figures 4C–H), suggest that the unresolved sulfur species differ in their composition between the two growth treatments. The high correlations and statistical significance (p-value < 0.05) for Figures 4C–H assist in providing a strong rationale for the basis of stoichiometric reactions occurring in the respective sulfur substrates individual metabolism. The higher slopes observed during growth on S0 (Figures 4C,E) alongside the greater overall H+ generation (10-fold higher total H+ increase) imply greater overall oxidation compared to growth on (Figures 4D,F and Supplementary Figure S2a). During growth on S0, a decrease in ΔS0, and increases in both ΔOther SOI and Δ imply that S0 is first converted to higher oxidation state SOI, e.g., polythionate species, and ultimately to (Supplementary Figure S2a); consistent with predominantly oxidative (i.e., acid generating) pathways (i.e., Eqs 2–6; Table 3). During growth on , ΔOther SOI and Δ increase from days 0 to 2, while, ΔOther SOI subsequently decreases and Δ does not change from days 2 to 4 (Supplementary Figure S2b), These results are consistent with oxidative pathways occurring initially (i.e., Eqs 5, 6, 9, and 10; Table 3), followed by disproportionating pathways (e.g., Eq. 11; Table 3; as shown further and in Supplementary Text), reflected in an increase in ΔS0. Consistent with a potential shift from oxidative (i.e., greater acid generating) to disproportionating reactions dominating, Δ[H+] increased between days 0 and 2, and subsequently decreased from days 2 to 4 (Supplementary Figure S2b).
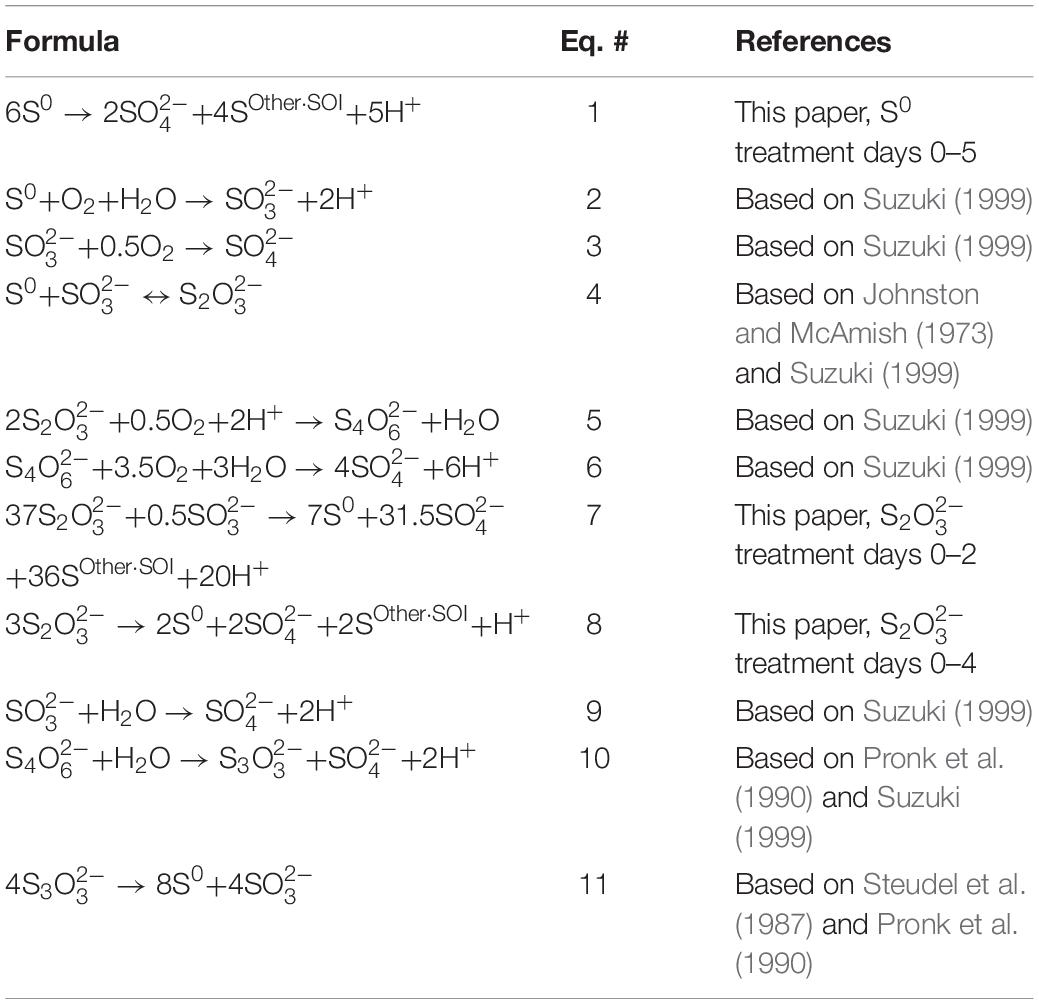
Table 3. Mass balance S reactions for the two treatments and potential S abiotic and biotic reactions important for stoichiometric balancing.
Electron Microscopic and Spectroscopic Analyses of Intracellular S0 Storage
Transmission electron microscopy in tandem with energy-dispersive X-ray spectroscopy (EDS) and wavelength dispersive spectroscopy (WDS) revealed sulfur globule formation in the cells (Figures 5A–H). The globules did not differ in size (Supplementary Figure S3), but quantification indicated that a higher number (45.6 per 100 bacteria) were observed for A. thiooxidans grown on S0, while a lower number of internal S0 globules (13.5 per 100 bacteria) occurred for A. thiooxidans grown on (Figures 5A,B vs. C,D), consistent with sulfur speciation and mass balance results (Supplementary Table S12).
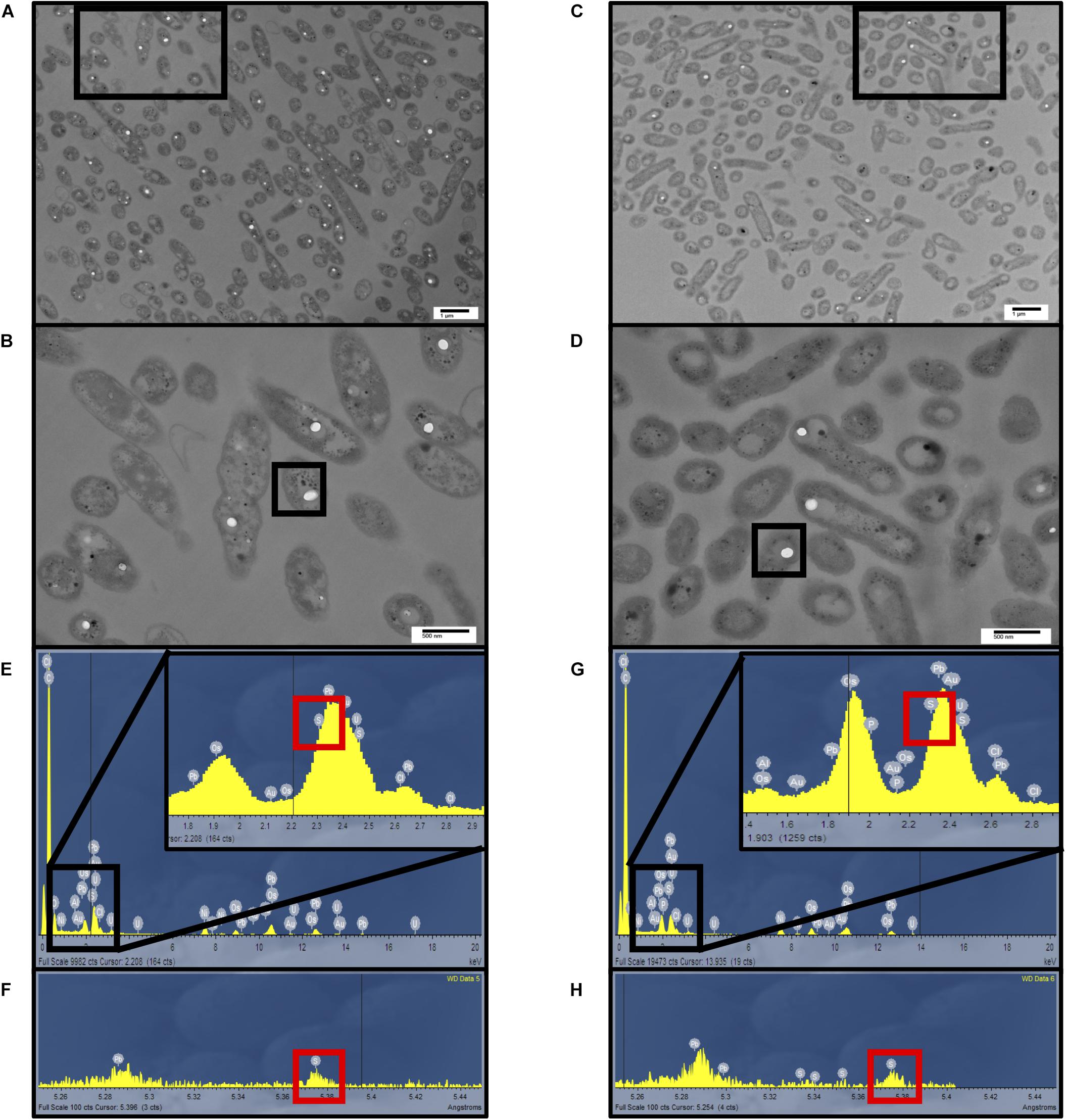
Figure 5. Electron microscopic analysis of sulfur globule formation. Transmission electron microscopy of A. thiooxidans cells grown in S0 media at pH 1.5 or 2.5 (A,B) and media at pH 2.5 (C,D), respectively. Scale bars in (A,C) indicate 1 μm and 500 nm in (B,D). (E,G) Energy-dispersive X-ray spectroscopy (EDS) analysis was conducted on the sulfur globules indicated in (B,D), revealing the presence of different elements shown by their characteristic emission energies. (F,H) To better separate the signals, wavelength-dispersive X-ray spectroscopy (WDS) analysis was conducted on the sulfur globules indicated in (B,D), confirming the presence of sulfur.
Sulfur Metabolism Models
Stoichiometric Sulfur Metabolism Arrays
We developed A. thiooxidans metabolism models by combining observed solution S speciation and [H+] changes with FPKM gene expression levels to elucidate the most likely pathways being catalyzed. The generated A. thiooxidans S0 metabolism model identifies conversion of S0 into 1/3 and 2/3 SOther SOI [Eq. 1; assumption of initial Other SOI generated to be ; the initial metabolism reaction from (Eq. 5, Table 3)]. While there are uncertainties as to whether the Other SOI pool is solely polythionate species and/or comprises the same polythionates at any given sampling point in either treatment, the highly significant correlations between acid generation and this specific sulfur pool (Figures 4G,H) are consistent with this assumption (Eqs 1, 7, and 8, Figures 6A–C and Table 3, respectively).
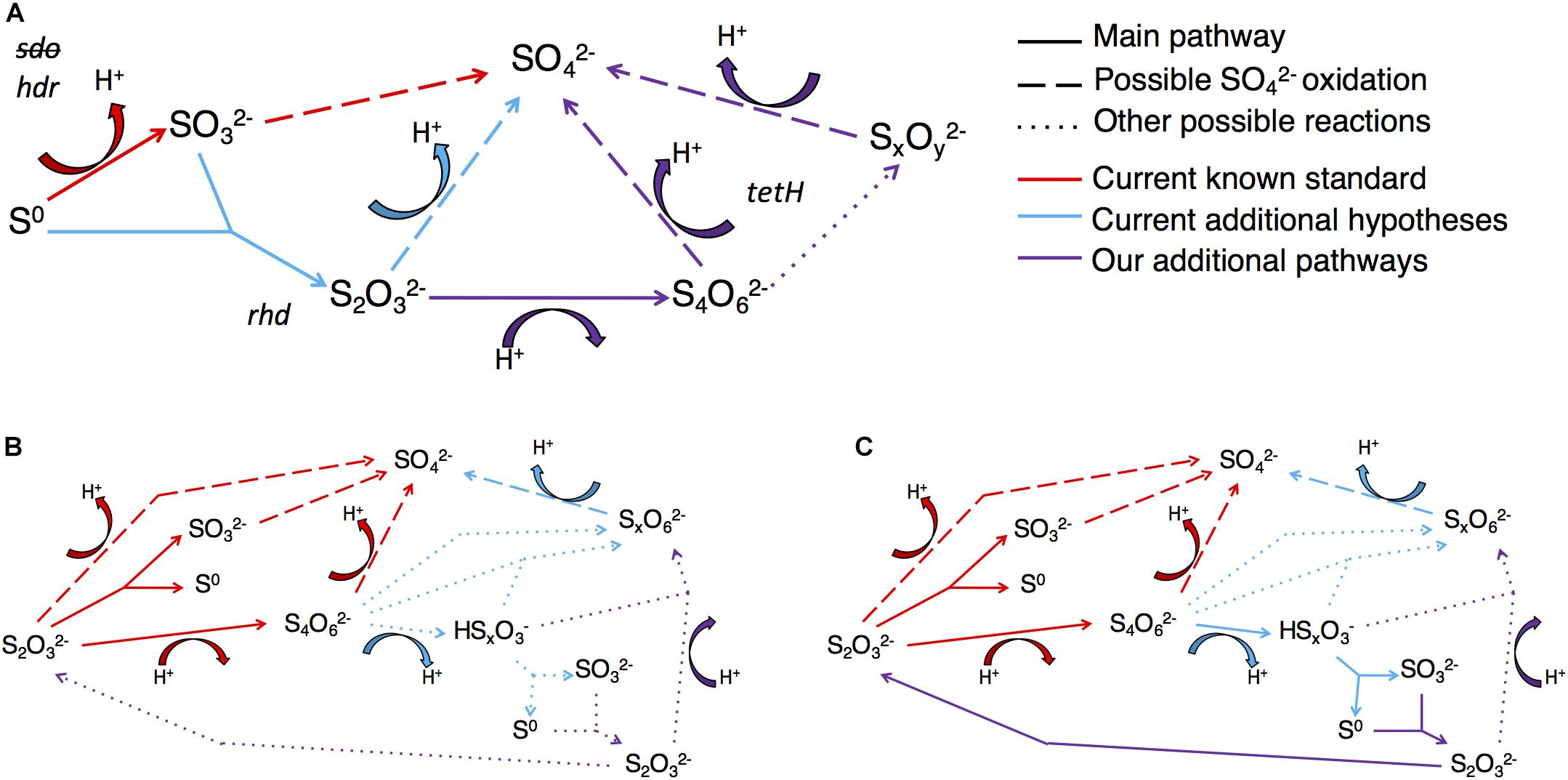
Figure 6. Models of theorized A. thiooxidans metabolism for different S reaction pathways, the genes catalyzing them and H+ consumption or production reactions based on observed experiments’ mass balance and known potential reactions in literature. (A) S0 initial reduced S source over total time of experiment (Days 0–5). (B) initial reduced S source early reactions (days 0–2). (C) initial reduced S source for total time of experiment (days 0–4). can be oxidized both biotically via H2O releasing H+ and abiotically via O2 which is neutral.
Thus our A. thiooxidans S0 metabolism model identifies the following suite of reactions occur throughout the time course of the experiment (Figure 6A).
The model stoichiometrically balances the observed changes in elemental sulfur concentration. However, the model predicts a greater acid generation than observed. Specifically, the model predicts production of 6H+ for every 6S0 converted to 2SO_4^2– and 4S (as Other SOI); whereas we observe 5H+. The same observed lower H+ generation relative to expected, also occurs for a model incorporating successive oxidative processing of sulfur by an alternative set of pathways that would exclude oxidation to (Eq. 3), and proceed via oxidation of and and other polythionates to (Table 3, Eqs. 5 and 6).
A stoichiometrically balanced sulfur and H+ model of A. thiooxidans metabolism developed for days 0–2 or for the entire time course of days 0–4 (Table 3; Eqs. 7 and 8, respectively) identifies the most likely occurring reactions would include conversion of to , S0 and polythionates (Other SOI) and ultimately to , with the reverse of Eq. 4 followed by Eqs. 3, 5, 6, and 9 as the dominant reactions (Figure 6B) (Table 3).
However, the metabolism model of A. thiooxidans for days 2–4 indicates disproportionation of and to S0 and are occurring (Table 3, Eqs. 10 and 11).
These disproportionation reactions would recycle sulfur back to , continuing to consume H+ via regenerated reduced SOI species such as and S0 over the time period of days 2–4. model reaction arrays (Figures 6B,C) can also be stoichiometrically balanced via other pathways involving oxidation of polythionates and thiosulfate to sulfate. However, informed by gene expression, results, S metabolism for days 0–2 and days 2–4 is more consistent with the reactions identified above. The most robust model for days 0–4 based on currently theorized/known sulfur reactions follows the series of reactions shown in Figure 6C, identifying the important formation and accumulation of S0. Stepwise reactions for Figure 6 are identified in Supplementary Text.
Models of A. thiooxidans S0 and Metabolism
By combining the analysis of gene expression, solution sulfur speciation and electron microscopy, our results provide new insights into A. thiooxidans sulfur metabolism revealing the importance of intracellular pathways. Based on these data we propose models for the metabolism of A. thiooxidans grown on S0 (Figures 7A,B) suggesting that the Sox complex plays a major role initiating metabolism after entry of S0 into the cell via unknown transporters. There is little published information on the transport of S0 into cells to date, however, it has been postulated by other studies to occur via outer membrane proteins (Sugio et al., 1991; Buonfiglio et al., 1999; Rohwerder and Sand, 2003). Sdo is not highly expressed, but it may also contribute to S0 metabolism. The intracellular S0 is metabolized subsequently through both oxidative and comproportionating pathways. Cytoplasmic Hdr catalyzes S0 oxidation generating intracellular . While it is not certain which gene(s) are involved in intracellular S0 comproportionation and buildup of sulfur granules, the high expression of genes responsible for production (hdr) yet low concentrations in bulk solution, suggest that this pathway generates . We believe that this pathway is active, because we observe medium-level expression of Rhd known to catalyze disproportionation (Figure 7A), possibly acting in a reverse reaction utilizing the high intracellular S0 and to produce , which is then oxidized to higher order S species (e.g., tetra- and other polythionates). This possibility is consistent with the observed increased concentration of the Other SOI pool and ultimately (Figure 4A). These higher oxidation state S species (i.e., and/or other higher chain polythionates represented, we believe, in the Other SOI fraction based on S speciation, [H+] changes and gene expression results presented above) generated through S0 comproportionation are oxidized through TetH catalysis resulting in . The observed increase in Hdr expression from exponential growth (Figure 7A) to stationary growth (Figure 7B) supports the notion that this pathway would catalyze growth through intracellular recycling of sulfur, and implies the synthesis of sulfur storage granules.
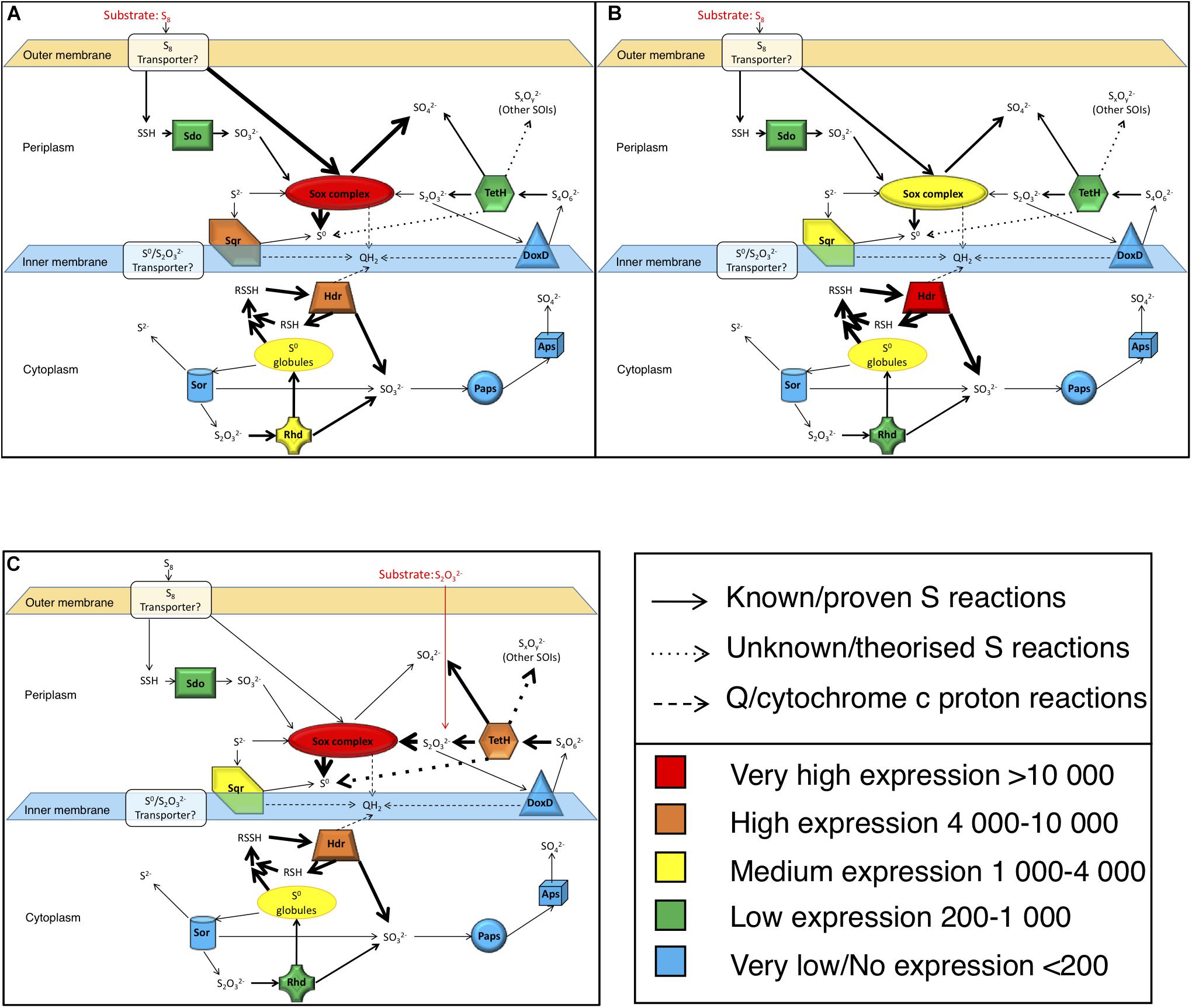
Figure 7. Model of A. thiooxidans S metabolism based on the analyses of the expression of key genes encoding S-metabolizing enzymes and of S solution chemistry. Gene expression values are based on the results shown in Figure 3A (FPKM). (A) Model for exponential growth phase in S0 media (day 3, pH 2.5); (B) model for stationary growth phase in S0 media (day 5, pH 1.5); (C) model for stationary growth phase in media (day 5, pH 2.5); models taking results of gene expression analysis and sulfur chemistry into account. Thickness of the arrows reflects importance of the pathways.
The model for growth of A. thiooxidans on implies that the Sox complex catalyzes disproportionation to S0 and (Figure 7C), while TetH catalyzes oxidation and conversion of to other higher chain polythionates [consistent with detection of Other SOI, which would include these unresolved S compounds (Figure 4B)]. These higher oxidation S compounds are then disproportionated via the Sox complex and/or TetH catalysis, resulting in intracellular S0, and the subsequent intracellular generation of indicated to occur by the high level of Hdr expression (Figure 7C). Comproportionation reforming from the high intracellular S0 and catalyzed by Rhd may also be possible, thereby recycling S within the cell. Alternatively, the low levels of expression of DoxD (Figure 3A), suggest that either TetH may be catalyzing a reverse reaction from to (or to Other SOI), or there may be other proteins responsible for oxidation to higher chain polythionates.
Discussion
Novel Insights Into S-Metabolism: Importance of S0, , and Intracellular Reactions
Comparisons to Previous Literature
The models of A. thiooxidans sulfur metabolism that were developed through integrated analysis of gene expression, sulfur chemistry, sulfur mass balance and electron microscopy reveal new insights into the importance of intracellular reactions involving TetH- and Hdr-catalyzed transformation of S0 into species, compared to previous models. Bobadilla Fazzini et al. (2013) analyzed the solution concentrations of two sulfur species, S0 and , for A. thiooxidans DSM 17318 at stationary phase when grown in S0 and media at low pH (1.8 and 2.5, respectively). Their chemically based model identified the same comproportionation reaction involving S0 and to form (Eq. 4; Table 3) as identified here for A. thiooxidans S0 growth. However, they speculated that Sdo was the most important protein for production, while our results are more consistent with the Hdr protein catalyzing this reaction. Further, their S0 metabolism model does not account for activity of the TetH enzyme, resulting in less S0 storage and Other SOI (e.g., polythionates) production. Bobadilla Fazzini et al. (2013) also modeled A. thiooxidans growth on and suggest, based only on their chemical analyses of S0 and that S0 production from polythionates occurs with no involvement of TetH. In contrast, our combined chemical and gene expression results assessing A. thiooxidans growth on , show that TetH is highly expressed (Figure 7C) and associated with the evident production of intracellular S0 determined by microscopy and elemental analyses (Figure 5C). This intracellular S0 plays a central role in metabolism (Figure 6C). The Bobadilla Fazzini et al. (2013) model did not predict any S0 storage for A. thiooxidans grown on S0, or storage in tandem with TetH activity for A. thiooxidans grown on and did not include the hdr, rhd, paps, and aps genes.
Yin et al. (2014) examined A. thiooxidans A01 via gene expression proposing a similar sulfur gene model to our A. thiooxidans ATCC 19377 model. Due to our improved draft genome of A. thiooxidans ATCC 19377, we were able to find and confirm the previously elusive sor gene (Valdes et al., 2011) identifying that the same sulfur genes are present in the two strains (Figure 1B). However, differences in the number of gene copies identified for hdrA, rhd, paps, and aps exist between the strains, where for A. thiooxidans ATCC 19377, we found three copies of hdrA, two copies of rhd and one copy each for paps and aps (Figure 3). In contrast, for A. thiooxidans A01 one copy of hdrA, five copies of rhd, three copies of paps, and two copies of aps were identified (Yin et al., 2014). That study found that most sulfur metabolic genes were more strongly expressed in A. thiooxidans A01 when grown on S0 compared to during exponential growth phase (Yin et al., 2014), showing opposing results to our relative expression levels for A. thiooxidans ATCC 19377 stationary phase growth on S0 compared to (Figure 3B-iii), These results suggest that relative gene expression switches from lower to higher in , and higher to lower in S0, as A. thiooxidans goes from exponential to stationary growth phase. However, the Yin et al., 2014 study only examined gene expression during exponential growth phase, and their hypothetic models included pathways identified from previous studies depicting models for other Acidithiobacillus species (Yin et al., 2014). Thus, their model was not able to identify the importance of intracellular S0, and and the hdr gene as observed here.
In comparison to other Acidithiobacillus species models of S metabolism, our gene model for A. thiooxidans shows closest similarity to A. caldus. Differing only that in A. caldus, Sdo has been determined to be located in the cytoplasm instead of the periplasm (Wu et al., 2017) and it has the addition of SAT responsible for oxidation of sulfite to sulfate (Wang et al., 2019). While the A. ferrooxidans gene model shows greater differences to our A. thiooxidans model, most notably in its absence of the Sox complex and sor gene, and its inclusion of SAT and TSD (Wang et al., 2019; Zhan et al., 2019). For A. caldus and A. ferrooxidans, the current S0 metabolism is proposed to be oxidation to via Sdo, followed by oxidation to via SAT, with the bacteria acquiring S0 from extracellular sources (Mangold et al., 2011; Zhan et al., 2019). This differs to our proposed S0 metabolic pathway (Figure 6A), which is elaborated upon further below. The current proposed model of oxidation metabolism, shows that both A. caldus and A. ferrooxidans utilize the S4I pathway, however, A. caldus also uses the Sox system while A. ferrooxidans also uses TSD (Ghosh and Dam, 2009; Wang et al., 2019). Our A. thiooxidans S metabolism model follows the same oxidation metabolism as A. caldus employing both the S4I pathway and the Sox system.
Integrated Gene Expression and Sulfur Chemistry A. thiooxidans Metabolism Models
The models generated here provide new insights into the likely pathways involved in A. thiooxidans sulfur metabolism, closing some of the gaps in the current understanding. Specifically, our results identify internal cell S0 generation, storage and use, as well as the importance and rapid conversion of in these models, both confirming the speculated importance of these two S compounds (Suzuki et al., 1992) and explaining why they have not previously been definitively confirmed by solution chemical characterization alone.
Based on the published studies to date, the first step in microbial S0 metabolism is thought to be a relatively linear pathway beginning with oxidation to , followed by further oxidation to (Suzuki et al., 1992; Rohwerder and Sand, 2003). However, here the model developed through combined sulfur chemical and gene expression analyses indicates that oxidation/disproportionation reactions are occurring as formation of significant amounts of Other SOI (i.e., indicating the presence of polythionates) and small amounts of are observed (Figure 4A). Consistent with these pathways, expression specifically of tetH and rhd, genes known to encode enzymes for and polythionate oxidation/disproportionation reactions were being expressed (Figures 7A,B) (Meulenberg et al., 1992; Hallberg et al., 1996; Beller et al., 2006; Rzhepishevska et al., 2007).
Further lending support to these alternative pathways, higher relative expression of the hdr genes was observed (Figure 3A), which should result in high levels of , and thus subsequent high values. However, our results here indicate lower values of than expected, consistent with recycling of this through comproportionating reactions that would generate instead. The specific presence of , despite likely abiotic disproportionation at this low pH < 2, (Supplementary Table S11) and activity of hdr genes associated with sulfur back reactions, underscore the formation of as a critical step in S0 metabolism (Figure 6A) generating the precursor to most reactions involving the increased pool of Other SOI, e.g., polythionates (Meulenberg et al., 1993; Müller et al., 2004).
The formation of from comproportionation during A. thiooxidans S0 metabolism is supported by three lines of evidence. First, the intracellular neutral pH of A. thiooxidans (Suzuki et al., 1999) makes the neutrophilic reaction combining S0 with to form favorable (Eq. 4, Table 1). Second, A. thiooxidans possesses rhodanese/sulfur transferase (Yin et al., 2014), which may include a rhodanese capable of binding a sulfane group sulfur (e.g., S0) to to form (Hildebrandt and Grieshaber, 2008; Zhang et al., 2013). Third, the metabolic bonding of S0 with is mediated via the Sox complex, which is highly expressed by A. thiooxidans grown on S0 (Figures 7A,B). The versatility of the Sox complex would support this pathway (Sauvé et al., 2007; Wang et al., 2019). The gene expression results are consistent with comproportionation, as results here identify that within the Sox complex, SoxYZ (carriers) and SoxAX (binders) are more highly expressed than the oxidizing enzyme (SoxB) in all analyses (Figure 3A).
Metabolic modeling results from growth on indicate A. thiooxidans oxidation closely follows the S4I pathway proposed in the literature, further suggesting higher oxidation chain polythionate formation (Figures 6B,C and Supplementary Text) (Hallberg et al., 1996; Masau et al., 2001; Müller et al., 2004; Ghosh and Dam, 2009). However, the ability to effectively measure all the possible sulfur species remains an analytical challenge (Houghton et al., 2016), which precludes 100% certainty in our model fitting.
The occurrence of S0 within the cells when grown on S0, can be attributed to the intake of the sulfur globules from the media via transport enzymes and outer membrane proteins (Rohwerder and Sand, 2003), and/or from SOI cycling through mechanisms such as oxidation via the Sox complex when missing SoxCD, a characteristic for sulfur globule formation in bacteria species (Figure 5A) (Steudel et al., 1987; Pronk et al., 1990). However, formation of S0 within the cells was also observed when A. thiooxidans was grown on associated with SOI cycling (Figure 5C), though at lower levels than that observed for A. thiooxidans grown on S0 (Figures 5A vs. C).
Relevance of Gene Expression Analysis
Relative Expression Levels Between Variable Conditions in S Metabolism
Results assessing relative changes in gene expression identify that the Sox complex, Sqr, Hdr, TetH, and Rhd are important in both S0 and metabolism by A. thiooxidans. While measurements of gene expression does not allow firm conclusions on absolute protein levels or enzyme activities, they do identify specific genes and encoded enzymes likely to be important in a metabolic pathway. Relative levels of expression of these genes however differ between the two sulfur media and between growth stages for S0 (Figure 3A). The results illustrate the importance of the Sox complex and of TetH for metabolism (Figure 7C). The Sox complex is a very important metabolic enzyme complex during growth on both substrates since it is highly expressed under all conditions (Figure 3A). Gene expression results indicate the sox-1 operon is active in cells at less acidic pH values and underscore the geochemical flexibility and viability of the Sox complex as expression of the sox-2 operon is used under more acidic conditions and with higher thiosulfate concentrations (Figure 3A). These observations are similar to those reported by others (Zhu et al., 2012; Jones et al., 2014; Yin et al., 2014; Li et al., 2017; Wang et al., 2019), whereby different gene copies of the same enzyme express at differing levels due to environmental parameters. The differential expression of these gene copies (Figure 3A) indicates that gene expression can provide insights into the geochemical conditions associated with sulfur metabolism.
Insight Into the Importance of Hdr Toward S Metabolism
Our results identify a key role of Hdr in A. thiooxidans ATCC 19377 S0 metabolism expanding the understanding of important genes and their roles in A. thiooxidans sulfur metabolism. Relatively high hdr expression levels were observed under all conditions in comparison to the low levels of sdo (Figure 3A). The inclusion of solution chemical data and electron microscopy suggest that Hdr is likely the primary S0 oxidizing enzyme rather than Sdo, which was previously identified as important for internal generation of and during growth on S0 (Rohwerder and Sand, 2003; Bobadilla Fazzini et al., 2013; Yin et al., 2014; Koch and Dahl, 2018). Catalysis by Hdr rather than Sdo is energetically more favorable since conversion of S0 to is a non-quinone/cytochrome metabolic step for Sdo. Thus it would result in a loss of approximately 50% of the available potential energy considering the ΔG of −500 to 550 kJ per mol S in oxidation of S0 to (Kelly, 1999). In contrast, catalysis of the hdr gene also found in A. caldus (Mangold et al., 2011), A. ferrooxidans (Quatrini et al., 2009) and A. thiooxidans A01 (Yin et al., 2014) enables A. thiooxidans to metabolize and access this energy. The identification of its role in sulfur metabolism here, may assist taxonomic classification and facilitate better understanding of the potential for sulfur metabolism across all Acidithiobacilli (Nuñez et al., 2017; Cao et al., 2018; Koch and Dahl, 2018; Wang et al., 2019) and other sulfur oxidizing microbes.
Conclusion
Here we are able to provide greater insight into the specific reactions being catalyzed by known sulfur genes and newly highlight the role of Hdr in A. thiooxidans sulfur metabolism by integrating gene expression levels with bulk solution S speciation. Our results further confirm the importance specifically of and in A. thiooxidans sulfur metabolism, which have been widely accepted in the literature to be important, though not definitively shown to date prior to this study (Suzuki et al., 1992; Suzuki, 1999; Bobadilla Fazzini et al., 2013). Further, our results generate new insights into the central role of intracellular S0 generation, transformation and pathways in both and S0 metabolism and that comproportionation to is a critical step in S0 metabolism. Collectively these results highlight how the integration of molecular biology and chemistry approaches can better inform our understanding of biogeochemical cycling of sulfur by microbes.
Data Availability Statement
The datasets generated for this study can be found in NCBI GenBank and NCBI Short Read Archive (SRA) repository, submitted and accession number for DNA is SZUV00000000, the accession number for RNA is PRJNA541131.
Author Contributions
DC and RF did all experimentation, analyses, and wrote manuscript. AF did TEM, EDS, and WDS work. SA provided analyses on ΣS via ICP-AES. AN, BL, CB, and LW provided funding and laboratory expertise. CB and LW also provided manuscript edits and were main supervisors to this work.
Funding
Work in the laboratory of LW was financed by Ontario Genomics Seed Grant, NSERC Discovery Grant and Glencore Sudbury INO and NRCCan MEND Secretariat. Research was funded by Genome Canada GC LSARP 2015 Grant Number OGI-124, and ORF-RE Round 8 Grant Number RE08-007. Work in the laboratories of CB and BL was financed by NSERC Discovery grants.
Conflict of Interest
The authors declare that the research was conducted in the absence of any commercial or financial relationships that could be construed as a potential conflict of interest.
Acknowledgments
We would like to thank Serge Sénéchal in the Université de Montréal Department of Microbiology, Infectiology and Immunology for assistance with FACS analysis, Matt Sarrasin (Department of Biochemistry and Molecular Medicine) for assistance with bioinformatics work and Benoit Bessette (Department of Biochemistry and Molecular Medicine) for technical assistance. EDS and WDS analysis were conducted in the CM2 facility at Polytechnique Montréal (https://www.polymtl.ca/expertises/centre-de-caracterisation-microscopique-des-materiaux-cm2). AN is recipient of a Tier 1 Canada Research Chair in Calcified tissues, Biomaterials and Structural Imaging.
Supplementary Material
The Supplementary Material for this article can be found online at: https://www.frontiersin.org/articles/10.3389/fmicb.2020.00411/full#supplementary-material
Footnotes
References
Bacelar-Nicolau, P., and Johnson, D. B. (1999). Leaching of pyrite by acidophilic heterotrophic iron-oxidizing bacteria in pure and mixed cultures. Appl. Environ. Microbiol. 65, 585–590. doi: 10.1128/aem.65.2.585-590.1999
Bankevich, A., Nurk, S., Antipov, D., Gurevich, A. A., Dvorkin, M., Kulikov, A. S., et al. (2012). SPAdes: a new genome assembly algorithm and its applications to single-cell sequencing. J. Comp. Biol. 19, 455–477. doi: 10.1089/cmb.2012.0021
Beller, H. R., Chain, P. S., Letain, T. E., Chakicherla, A., Larimer, F. W., Richardson, P. M., et al. (2006). The genome sequence of the obligately chemolithoautotrophic, facultatively anaerobic bacterium Thiobacillus denitrificans. J. Bacteriol. 188, 1473–1488. doi: 10.1128/JB.188.4.1473-1488.2006
Bernier, L., and Warren, L. (2005). Microbially driven acidity generation in a tailings lake. Geobiology 3, 115–133. doi: 10.1111/j.1472-4669.2005.00047.x
Bernier, L., and Warren, L. A. (2007). Geochemical diversity in S processes mediated by culture-adapted and environmental-enrichments of Acidithiobacillus spp. Geochim. Cosmochim. Acta 71, 5684–5697. doi: 10.1016/j.gca.2007.08.010
Blankenberg, D., Von Kuster, G., Coraor, N., Ananda, G., Lazarus, R., Mangan, M., et al. (2010). Galaxy: a web-based genome analysis tool for experimentalists. Curr. Protoc. Mol. Biol. Chapter 1, Unit19.10.1–21. doi: 10.1002/0471142727.mb1910s89
Bobadilla Fazzini, R. A., Cortés, M. P., Padilla, L., Maturana, D., Budinich, M., Maass, A., et al. (2013). Stoichiometric modeling of oxidation of reduced inorganic sulfur compounds (Riscs) in Acidithiobacillus thiooxidans. Biotechnol. Bioeng. 110, 2242–2251. doi: 10.1002/bit.24875
Bolger, A. M., Lohse, M., and Usadel, B. (2014). Trimmomatic: a flexible trimmer for Illumina sequence data. Bioinformatics 30, 2114–2120. doi: 10.1093/bioinformatics/btu170
Boyd, E. S., and Druschel, G. K. (2013). Involvement of intermediate sulfur species in biological reduction of elemental sulfur under acidic, hydrothermal conditions. Appl. Environ. Microbiol. 79, 2061–2068. doi: 10.1128/AEM.03160-12
Buonfiglio, V., Polidoro, M., Soyer, F., Valenti, P., and Shively, J. (1999). A novel gene encoding a sulfur-regulated outer membrane protein in Thiobacillus ferrooxidans. J. Biotechnol. 72, 85–93. doi: 10.1016/s0168-1656(99)00097-8
Canfield, D. E., Stewart, F. J., Thamdrup, B., De Brabandere, L., Dalsgaard, T., Delong, E. F., et al. (2010). A cryptic sulfur cycle in oxygen-minimum–zone waters off the Chilean coast. Science 330, 1375–1378. doi: 10.1126/science.1196889
Cao, X., Koch, T., Steffens, L., Finkensieper, J., Zigann, R., Cronan, J. E., et al. (2018). Lipoate-binding proteins and specific lipoate-protein ligases in microbial sulfur oxidation reveal an atpyical role for an old cofactor. Elife 7:37439. doi: 10.7554/eLife.37439
Druschel, G. K. (2002). Sulfur Biochemistry: Kinetics of Intermediate Sulfur Species Reactions in the Environment. Ph.D. dissertation, University of Wisconsin – Madison, Madison, WI.
Druschel, G. K., Baker, B. J., Gihring, T. M., and Banfield, J. F. (2004). Acid mine drainage biogeochemistry at Iron Mountain, California. Geochem. Trans. 5:13. doi: 10.1186/1467-4866-5-13
Friedrich, C. G., Rother, D., Bardischewsky, F., Quentmeier, A., and Fischer, J. (2001). Oxidation of reduced inorganic sulfur compounds by bacteria: emergence of a common mechanism? Appl. Environ. Microbiol. 67, 2873–2882. doi: 10.1128/AEM.67.7.2873-2882.2001
Ghosh, W., and Dam, B. (2009). Biochemistry and molecular biology of lithotrophic sulfur oxidation by taxonomically and ecologically diverse bacteria and archaea. FEMS Microbiol. Rev. 33, 999–1043. doi: 10.1111/j.1574-6976.2009.00187.x
Giardine, B., Riemer, C., Hardison, R. C., Burhans, R., Elnitski, L., Shah, P., et al. (2005). Galaxy: a platform for interactive large-scale genome analysis. Genome Res. 15, 1451–1455. doi: 10.1101/gr.4086505
Goecks, J., Nekrutenko, A., Taylor, J., and Galaxy, T. (2010). Galaxy: a comprehensive approach for supporting accessible, reproducible, and transparent computational research in the life sciences. Genome Biol. 11:R86. doi: 10.1186/gb-2010-11-8-r86
Griesbeck, C., Schütz, M., Schödl, T., Bathe, S., Nausch, L., Mederer, N., et al. (2002). Mechanism of sulfide-quinone reductase investigated using site-directed mutagenesis and sulfur analysis. Biochemistry 41, 11552–11565. doi: 10.1021/bi026032b
Hallberg, K. B., Dopson, M., and Lindstrom, E. B. (1996). Reduced sulfur compound oxidation by Thiobacillus caldus. J. Bacteriol. 178, 6–11. doi: 10.1128/jb.178.1.6-11.1996
Hallberg, K. B., and Johnson, D. B. (2003). Novel acidophiles isolated from moderately acidic mine drainage waters. Hydrometallurgy 71, 139–148. doi: 10.1016/s0304-386x(03)00150-6
Hensen, D., Sperling, D., Trüper, H. G., Brune, D. C., and Dahl, C. (2006). Thiosulphate oxidation in the phototrophic sulphur bacterium Allochromatium vinosum. Mol. Microbiol. 62, 794–810. doi: 10.1111/j.1365-2958.2006.05408.x
Hildebrandt, T. M., and Grieshaber, M. K. (2008). Three enzymatic activities catalyze the oxidation of sulfide to thiosulfate in mammalian and invertebrate mitochondria. FEBS J. 275, 3352–3361. doi: 10.1111/j.1742-4658.2008.06482.x
Houghton, J. L., Foustoukos, D., Flynn, T. M., Vetriani, C., Bradley, A. S., and Fike, D. A. (2016). Thiosulfate oxidation by Thiomicrospira thermophila: metabolic flexibility in response to ambient geochemistry. Environ. Microbiol. 18, 3057–3072. doi: 10.1111/1462-2920.13232
Johnson, D. B., and Hallberg, K. B. (2003). The microbiology of acidic mine waters. Res. Microbiol. 154, 466–473. doi: 10.1016/s0923-2508(03)00114-1
Johnston, F., and McAmish, L. (1973). A study of the rates of sulfur production in acid thiosulfate solutions using S-35. J. Colloid Interface Sci. 42, 112–119. doi: 10.1016/0021-9797(73)90013-1
Jones, D. S., Schaperdoth, I., and Macalady, J. L. (2014). Metagenomic evidence for sulfide oxidation in extremely acidic cave biofilms. Geomicrobiol. J. 31, 194–204. doi: 10.1080/01490451.2013.834008
Jørgensen, B. B., and Nelson, D. C. (2004). Sulfide oxidation in marine sediments: geochemistry meets microbiology. Geol. Soc. Am. 379, 63–81.
Kelly, D. P. (1999). Thermodynamic aspects of energy conservation by chemolithotrophic sulfur bacteria in relation to the sulfur oxidation pathways. Arch. Microbiol. 171, 219–229. doi: 10.1007/s002030050703
Kelly, D. P., and Baker, S. C. (1990). The organosulphur cycle: aerobic and anaerobic processes leading to turnover of C1 -sulphur compounds. FEMS Microbiol. Lett. 87, 241–246. doi: 10.1111/j.1574-6968.1990.tb04919.x
Kelly, D. P., Shergill, J. K., Lu, W., and Wood, A. P. (1997). Oxidative metabolism of inorganic sulfur compounds by bacteria. Antonie Van Leeuwenhoek. 71, 95–107.
Kletzin, A. (1989). Coupled enzymatic production of sulfite, thiosulfate, and hydrogen sulfide from sulfur: purification and properties of a sulfur oxygenase reductase from the facultatively anaerobic archaebacterium Desulfurolobus ambivalens. J. Bacteriol. 171, 1638–1643. doi: 10.1128/jb.171.3.1638-1643.1989
Kletzin, A. (1992). Molecular characterization of the sor gene, which encodes the sulfur oxygenase/reductase of the thermoacidophilic Archaeum Desulfurolobus ambivalens. J. Bacteriol. 174, 5854–5859. doi: 10.1128/jb.174.18.5854-5859.1992
Koch, T., and Dahl, C. (2018). A novel bacterial sulfur oxidation pathway provides a new link between the cycles of organic and inorganic sulfur compounds. ISME J. 12, 2479–2491. doi: 10.1038/s41396-018-0209-7
Li, L. F., Fu, L. J., Lin, J. Q., Pang, X., Liu, X. M., Wang, R., et al. (2017). The sigma(54)-dependent two-component system regulating sulfur oxidization (Sox) system in Acidithiobacillus caldus and some chemolithotrophic bacteria. Appl. Microbiol. Biotechnol. 101, 2079–2092. doi: 10.1007/s00253-016-8026-2
Mangold, S., Valdes, J., Holmes, D. S., and Dopson, M. (2011). Sulfur metabolism in the extreme acidophile Acidithiobacillus caldus. Front. Microbiol. 2:17. doi: 10.3389/fmicb.2011.00017
Masau, R. J. Y., Oh, J. K., and Suzuki, I. (2001). Mechanism of oxidation of inorganic sulfur compounds by thiosulfate-grown Thiobacillus thiooxidans. Can. J. Microbiol. 47, 348–358. doi: 10.1139/w01-015
Meulenberg, R., Pronk, J. T., Hazeu, W., Bos, P., and Kuenen, J. G. (1992). Oxidation of reduced sulphur compounds by intact cells of Thiobacillus acidophilus. Arch. Microbiol. 157, 161–168. doi: 10.1007/bf00245285
Meulenberg, R., Pronk, J. T., Hazeu, W., van Dijken, J. P., Frank, J., Bos, P., et al. (1993). Purification and partial characterization of thiosulphate dehydrogenase from Thiobacillus acidophilus. J. Gen. Microbiol. 139, 2033–2039. doi: 10.1099/00221287-139-9-2033
Miranda-Trevino, J. C., Pappoe, M., Hawboldt, K., and Bottaro, C. (2013). The importance of thiosalts speciation: review of analytical methods, kinetics, and treatment. Crit. Rev. Environ. Sci. Technol. 43, 2013–2070. doi: 10.1080/10643389.2012.672047
Müller, F. H., Bandeiras, T. M., Urich, T., Teixeira, M., Gomes, C. M., and Kletzin, A. (2004). Coupling of the pathway of sulphur oxidation to dioxygen reduction: characterization of a novel membrane−bound thiosulphate: quinone oxidoreductase. Mol. Microbiol. 53, 1147–1160. doi: 10.1111/j.1365-2958.2004.04193.x
Nordstrom, D. K. (2015). Baseline and premining geochemical characterization of mined sites. Appl. Geochem. 57, 17–34. doi: 10.1016/j.apgeochem.2014.12.010
Nordstrom, D. K., Blowes, D. W., and Ptacek, C. J. (2015). Hydrogeochemistry and microbiology of mine drainage: an update. Appl. Geochem. 57, 3–16. doi: 10.1016/j.apgeochem.2015.02.008
Nuñez, H., Moya-Beltrán, A., Covarrubias, P. C., Issotta, F., Cardenas, J. P., Gonzalez, M., et al. (2017). Molecular systematics of the genus Acidithiobacillus: insights into the phylogenetic structure and diversification of the taxon. Front. Microbiol. 8:30. doi: 10.3389/fmicb.2017.00030
Pronk, J., Meulenberg, R., Hazeu, W., Bos, P., and Kuenen, J. (1990). Oxidation of reduced inorganic sulphur compounds by acidophilic Thiobacilli. FEMS Microbiol. Lett. 75, 293–306. doi: 10.1111/j.1574-6968.1990.tb04103.x
Quatrini, R., Appia-Ayme, C., Denis, Y., Jedlicki, E., Holmes, D. S., and Bonnefoy, V. (2009). Extending the models for iron and sulfur oxidation in the extreme acidophile Acidithiobacillus ferrooxidans. BMC Genomics 10:394. doi: 10.1186/1471-2164-10-394
Rethmeier, J., Rabenstein, A., Langer, M., and Fischer, U. (1997). Detection of traces of oxidized and reduced sulfur compounds in small samples by combination of different high-performance liquid chromatography methods. J. Chromatogr. A 760, 295–302. doi: 10.1016/s0021-9673(96)00809-6
Rohwerder, T., and Sand, W. (2003). The sulfane sulfur of persulfides is the actual substrate of the sulfur-oxidizing enzymes from Acidithiobacillus and Acidiphilium spp. Microbiology 149, 1699–1710. doi: 10.1099/mic.0.26212-0
Rzhepishevska, O. I., Valdes, J., Marcinkeviciene, L., Gallardo, C. A., Meskys, R., Bonnefoy, V., et al. (2007). Regulation of a novel Acidithiobacillus caldus gene cluster involved in metabolism of reduced inorganic sulfur compounds. Appl. Environ. Microbiol. 73, 7367–7372. doi: 10.1128/AEM.01497-7
Sauvé, V., Bruno, S., Berks, B. C., and Hemmings, A. M. (2007). The SoxYZ complex carries sulfur cycle intermediates on a peptide swinging arm. J. Biol. Chem. 282, 23194–23204. doi: 10.1074/jbc.m701602200
Schippers, A., Jozsa, P., and Sand, W. (1996). Sulfur chemistry in bacterial leaching of pyrite. Appl. Environ. Microbiol. 62, 3424–3431. doi: 10.1128/aem.62.9.3424-3431.1996
Schippers, A., and Sand, W. (1999). Bacterial leaching of metal sulfides proceeds by two indirect mechanisms via thiosulfate or via polysulfides and sulfur. Appl. Environ. Microbiol. 65, 319–321. doi: 10.1128/aem.65.1.319-321.1999
Seemann, T. (2014). Prokka: rapid prokaryotic genome annotation. Bioinformatics 30, 2068–2069. doi: 10.1093/bioinformatics/btu153
Staley, J. T., Bryant, M. P., Pfennig, N., and Holt, J. G. (1989). “Acidithiobacillus,” in Bergey’s Manual of Systematic Bacteriology, 1st Edn, Vol. 3, ed. W. A. Wilkins (Baltimore, MD: Springer), 1842–1858.
Steudel, R., Holdt, G., Göbel, T., and Hazeu, W. (1987). Chromatographic separation of higher polythionates SnO 62⊖(n= 3…22) and their detection in cultures of Thiobacillus ferroxidans; Molecular Composition of Bacterial Sulfur Secretions. Angewandte Chemie Int. Ed. Engl. 26, 151–153. doi: 10.1002/anie.198701511
Sugio, T., Suzuki, H., Oto, A., Inagaki, K., Tanaka, H., and Tano, T. (1991). Purification and some properties of a hydrogen sulfide- binding protein that is involved in sulfur oxidation of Thiobacillus ferrooxidans. Agric. Biol. Chem. 55, 2091–2097. doi: 10.1271/bbb1961.55.2091
Suzuki, I. (1999). Oxidation of inorganic sulfur compounds: chemical and enzymatic reactions. Can. J. Microbiol. 45, 97–105. doi: 10.1139/w98-223
Suzuki, I., Chan, C. W., and Takeuchi, T. L. (1992). Oxidation of elemental sulfur to sulfite by Thiobacillus thiooxidans cells. Appl. Environ. Microbiol. 58, 3767–3769. doi: 10.1128/aem.58.11.3767-3769.1992
Suzuki, I., Lee, D., Mackay, B., Harahuc, L., and Oh, J. K. (1999). Effect of various ions, pH, and osmotic pressure on oxidation of elemental sulfur by Thiobacillus thiooxidans. Appl. Environ. Microbiol. 65, 5163–5168. doi: 10.1128/aem.65.11.5163-5168.1999
Thamdrup, B., Fossing, H., and Jørgensen, B. B. (1994). Manganese, iron and sulfur cycling in a coastal marine sediment, Aarhus Bay, Denmark. Geochim. Cosmochim. Acta 58, 5115–5129. doi: 10.1016/0016-7037(94)90298-4
Valdes, J., Ossandon, F., Quatrini, R., Dopson, M., and Holmes, D. S. (2011). Draft genome sequence of the extremely acidophilic biomining bacterium Acidithiobacillus thiooxidans ATCC 19377 provides insights into the evolution of the Acidithiobacillus genus. J. Bacteriol. 193, 7003–7004. doi: 10.1128/JB.06281-11
Valdes, J., Pedroso, I., Quatrini, R., Dodson, R. J., Tettelin, H., and Blake, R. II (2008). Acidithiobacillus ferrooxidans metabolism: from genome sequence to industrial applications. BMC Genomics 9:597. doi: 10.1186/1471-2164-9-597
Valdes, J., Quatrini, R., Hallberg, K., Dopson, M., Valenzuela, P. D., and Holmes, D. S. (2009). Draft genome sequence of the extremely acidophilic bacterium Acidithiobacillus caldus ATCC 51756 reveals metabolic versatility in the genus Acidithiobacillus. J. Bacteriol. 191, 5877–5878. doi: 10.1128/JB.00843-09
Wang, R., Lin, J. Q., Liu, X. M., Pang, X., Zhang, C. J., Gao, X. Y., et al. (2019). Sulfur oxidation in the acidophilic autotrophic Acidithiobacillus spp. Front. Microbiol. 9:3290. doi: 10.3389/fmicb.2018.03290
Warren, L., Norlund, K. I., and Bernier, L. (2008). Microbial thiosulphate reaction arrays: the interactive roles of Fe (III), O2 and microbial strain on disproportionation and oxidation pathways. Geobiology 6, 461–470. doi: 10.1111/j.1472-4669.2008.00173.x
Wu, W., Pang, X., Lin, J., Liu, X., Wang, R., Lin, J., et al. (2017). Discovery of a new subgroup of sulfur dioxygenases and characterization of sulfur dioxygenases in the sulfur metabolic network of Acidithiobacillus caldus. PLoS One 12:e0183668. doi: 10.1371/journal.pone.0183668
Yin, H., Zhang, X., Li, X., He, Z., Liang, Y., Guo, X., et al. (2014). Whole-genome sequencing reveals novel insights into sulfur oxidation in the extremophile Acidithiobacillus thiooxidans. BMC Microbiol. 14:179. doi: 10.1186/1471-2180-14-179
You, X. Y., Guo, X., Zheng, H. J., Zhang, M. J., Liu, L. J., Zhu, Y. Q., et al. (2011). Unraveling the Acidithiobacillus caldus complete genome and its central metabolisms for carbon assimilation. J. Genet. Genomics 38, 243–252. doi: 10.1016/j.jgg.2011.04.006
Zhan, Y., Yang, M., Zhang, S., Zhao, D., Duan, J., Wang, W., et al. (2019). Iron and sulfur oxidation pathways of Acidithiobacillus ferrooxidans. World J. Microbiol. Biotechnol. 35:60. doi: 10.1007/s11274-019-2632-y
Zhang, L., Liu, X., Liu, J., and Zhang, Z. (2013). Characteristics and function of sulfur dioxygenase in echiuran worm Urechis unicinctus. PLoS One 8:e81885. doi: 10.1371/journal.pone.0081885
Zhu, J., Jiao, W., Li, Q., Liu, X., Qin, W., Qiu, G., et al. (2012). Investigation of energy gene expressions and community structures of free and attached acidophilic bacteria in chalcopyrite bioleaching. J. Ind. Microbiol. Biotechnol. 39, 1833–1840. doi: 10.1007/s10295-012-1190-1
Zopfi, J., Ferdelman, T. G., and Fossing, H. (2004). “Distribution and fate of sulfur intermediates–sulfite, tetrathionate, thiosulfate, and elemental sulfur –in marine sediments,” in Sulfur Biogeochemistry — Past and Present Special Paper 379, eds J. P. Amend, K. Edwards, and T. W. Lyons (Boulder, CO: Geological Society of America), 97–116.
Keywords: sulfur metabolism, gene expression, geochemistry, Acidithiobacillus thiooxidans, sulfur oxidation, modeling
Citation: Camacho D, Frazao R, Fouillen A, Nanci A, Lang BF, Apte SC, Baron C and Warren LA (2020) New Insights Into Acidithiobacillus thiooxidans Sulfur Metabolism Through Coupled Gene Expression, Solution Chemistry, Microscopy, and Spectroscopy Analyses. Front. Microbiol. 11:411. doi: 10.3389/fmicb.2020.00411
Received: 26 February 2019; Accepted: 27 February 2020;
Published: 13 March 2020.
Edited by:
Gordon T. Taylor, Stony Brook University, United StatesReviewed by:
Eric D. van Hullebusch, Université de Paris, FranceDelong Meng, Central South University, China
Copyright © 2020 Camacho, Frazao, Fouillen, Nanci, Lang, Apte, Baron and Warren. This is an open-access article distributed under the terms of the Creative Commons Attribution License (CC BY). The use, distribution or reproduction in other forums is permitted, provided the original author(s) and the copyright owner(s) are credited and that the original publication in this journal is cited, in accordance with accepted academic practice. No use, distribution or reproduction is permitted which does not comply with these terms.
*Correspondence: Lesley A. Warren, bGVzbGV5LndhcnJlbkB1dG9yb250by5jYQ==
†These authors have contributed equally to this work and share senior authorship