- 1Centro de Investigación Biomédica en Red de Enfermedades Hepáticas y Digestivas, Department of Pharmacology, School of Pharmacy, University of Granada, Granada, Spain
- 2Department of Microbiology, Instituto de Hortofruticultura Subtropical y Mediterránea “La Mayora”, University of Málaga, Málaga, Spain
- 3Department of Biomedical Informatics, University of Arkansas for Medical Sciences, Little Rock, AR, United States
- 4Department of Biochemistry and Molecular Biology II, School of Pharmacy, University of Granada, Granada, Spain
- 5Department of Environmental Protection, Estación Experimental del Zaidín, Consejo Superior de Investigaciones Científicas, Granada, Spain
Pseudomonas aeruginosa is an ubiquitous gram-negative opportunistic human pathogen which is not considered part of the human commensal gut microbiota. However, depletion of the intestinal microbiota (Dysbiosis) following antibiotic treatment facilitates the colonization of the intestinal tract by Multidrug-Resistant P. aeruginosa. One possible strategy is based on the use of functional foods with prebiotic activity. The bifidogenic effect of the prebiotic inulin and its hydrolyzed form (fructooligosaccharide: FOS) is well established since they promote the growth of specific beneficial (probiotic) gut bacteria such as bifidobacteria. Previous studies of the opportunistic nosocomial pathogen Pseudomonas aeruginosa PAO1 have shown that inulin and to a greater extent FOS reduce growth and biofilm formation, which was found to be due to a decrease in motility and exotoxin secretion. However, the transcriptional basis for these phenotypic alterations remains unclear. To address this question we conducted RNA-sequence analysis. Changes in the transcript level induced by inulin and FOS were similar, but a set of transcript levels were increased in response to inulin and reduced in the presence of FOS. In the presence of inulin or FOS, 260 and 217 transcript levels, respectively, were altered compared to the control to which no polysaccharide was added. Importantly, changes in transcript levels of 57 and 83 genes were found to be specific for either inulin or FOS, respectively, indicating that both compounds trigger different changes. Gene pathway analyses of differentially expressed genes (DEG) revealed a specific FOS-mediated reduction in transcript levels of genes that participate in several canonical pathways involved in metabolism and growth, motility, biofilm formation, β-lactamase resistance, and in the modulation of type III and VI secretion systems; results that have been partially verified by real time quantitative PCR measurements. Moreover, we have identified a genomic island formed by a cluster of 15 genes, encoding uncharacterized proteins, which were repressed in the presence of FOS. The analysis of isogenic mutants has shown that genes of this genomic island encode proteins involved in growth, biofilm formation and motility. These results indicate that FOS selectively modulates bacterial pathogenicity by interfering with different signaling pathways.
Introduction
The human pathogen Pseudomonas aeruginosa causes a wide array of life-threatening acute and chronic infections, particularly in immunocompromised, cancer, burn wound, and cystic fibrosis patients (Juhas, 2015). This bacterium is moreover one of the leading causes of nosocomial infections affecting hospitalized patients (Buhl et al., 2015) and mortality associated with hospital-acquired P. aeruginosa infectious like ventilator-associated pneumonia or bacteremia is above 35% (Lynch et al., 2017).
Moreover, under continuous antibiotic treatment the intestinal microbiota integrity is compromised and bears depletion of the intestinal microbiota (Dysbiosis), hence, physiological colonization resistance subsequently facilitates the establishment of the Pseudomonas aeruginosa in the intestinal ecosystem which might be considered an important internal source for P. aeruginosa infection (Ohara and Itoh, 2003; Von Klitzing et al., 2017). It is important to note that pathological alterations of the intestinal microbiota (dysbiosis) is related with continuous antibiotic treatment, obesity, diabetes and fatty liver, and of course alterations of the intestinal barrier function (IBF) as in inflammatory bowel disease and metabolic syndrome (Cano et al., 2013; Miura and Ohnishi, 2014).
The severity and permanence of these infections are related to the ability of P. aeruginosa to resist the effect of antibiotics through the formation of biofilms (Mah et al., 2003; Hoiby et al., 2010; Taylor et al., 2014). Important research efforts have been made to study the molecular mechanisms related to the formation maturation and subsequent dispersion of the biofilm (Stoodley et al., 2002; Flemming et al., 2007). A number of surface proteins and appendages, including flagella and type IV pili, were found to be associated with biofilm formation (Klausen et al., 2003; Anyan et al., 2014). Furthermore, this species is characterized by its ability to synthesize the virulent factors exotoxin A and pyocyanin (Ortiz-Castro et al., 2014) that block protein synthesis consequently leading to cell death (Gaines et al., 2007).
Treatment of P. aeruginosa infections can be particularly challenging because this bacterium is intrinsically resistant to multiple antibiotics and can easily acquire new resistances (Breidenstein et al., 2011). In fact, over the past three decades, antibiotic resistance among P. aeruginosa has escalated globally, via the global dissemination of several multidrug-resistant epidemic clones (Miyoshi-Akiyama et al., 2017). Pseudomonas aeruginosa infections thus represent a severe threat to human health worldwide and the World Health Organization has declared this bacterium the second priority pathogen for research and development of new strategies to fight it (WHO, 2017).
Besides conventional treatments, one possible strategy is based on the use of functional foods with prebiotic activity which are non-digestible foods (mostly oligosaccharides) that selectively stimulate the growth of a limited number of host-friendly colonic bacteria (Froebel et al., 2019). Thus, from a chemical standpoint, resistance to human digestive enzymes and low absorption are key for these compounds to reach the distal parts of the gut, where they can be fermented by the microbiota, which in turn is selectively modified in the process. These additional actions of prebiotics tend to enhance the capacity of the mucosa to contain luminal microorganisms and their components, i.e., intestinal barrier function (IBF). Normally, passage of microorganisms and/or their components such as Lipopolysaccharides (LPS) to the mucosa and from there to the bloodstream (translocation) is minimal, and the immune system develops tolerance to the microbiota, without inflammation. Conversely, when IBF is compromised translocation ensues, depending on the nature of the dysfunction and the physiological/pathological context. Therefore, inflammation of the intestine is considered to stem from augmented translocation, which engages the adaptive immune system, ultimately resulting in uncontrolled inflammation. Thus, reinforcing IBF may be protective and is viewed as therapeutic in this context (Natividad and Verdu, 2012; Duseja and Chawla, 2014).
A significant number of natural compounds have been found to inhibit bacterial growth, although their mechanisms of action frequently remain unclear (Amer et al., 2010). Fructooligosaccharides (FOS) are short-chain oligosaccharides that are generated by hydrolysis of the polysaccharide inulin, which is composed of two to 60 fructose monomers. Inulin is found in different nutrients such as wheat, onion, garlic and banana (Lattimer and Haub, 2010) and is the most common used fiber in prebiotics that, when used in combination with other probiotics, is able to promote the growth of specific beneficial gut bacteria such as bifidobacteria (Gibson et al., 1995; Bosscher et al., 2006).
A number of studies illustrate that FOS and inulin exert a number of different effects on humans and animals. For example, most oraly delivered plant substrate supplements that prevent gastrointestinal infections such as prebiotinTM and symbioramTM contain inulin and FOS, indicating that these compounds are also able to reduce bacterial infection. Moreover, oligosaccharids and in particular inulin and FOS were found to have beneficial effects on intestinal immunity and intestinal barrier function (IBF) (Capitán-Cañadas et al., 2013, 2016). Another study has shown that oligosaccharides from goats milk as well as galactooligosaccharides, modulate cytokine production by intestinal epithelial cells and monocytes via a mechanism involving Toll-like receptor 4 (TLR4) (Capitán-Cañadas et al., 2013). TLRs are located on the cell membrane and in endosomes, where they recognize components of cell membranes (TLR2/6, TLR2/TLR4), nucleic acids (TLR3, 7, 8, and 9) and flagellin (TLR5). TLR4 is one of the non-pathogen recognition receptors (PRRs), which are key elements in the communication between the host and the microbiota (Sánchez de Medina et al., 2013). However, further clinical oral applications will require studies on the potential effects of these natural substrates on the human body, which corresponds to the research need addressed in this article.
In addition to the bacterial growth promoting role of inulin and FOS, we reported that FOS inhibited bacterial growth and biofilm formation of P. aeruginosa PAO1 (Ortega-González et al., 2014). Additionally, both compounds caused opposing effects on bacterial motility. While FOS inhibited motility, an increased motility was observed in the presence of inulin. Moreover, in co-cultures with eukaryotic cells (macrophages) FOS, and to a lesser extent inulin, reduced the secretion of the inflammatory cytokines IL-6, IL-10, and TNF-α. We were also able to show that the reduction in cytokine secretion is due to a FOS-mediated modulation of the NF-κβ signal transduction pathway (Ortega-González et al., 2014). To gain insight into the detailed molecular processes triggered by FOS and inulin, we report here results from RNA-seq studies.
Materials and Methods
Materials
Inulin and FOS were purchased from BENEO-Orafti (Tienen Belgium). Stock solutions at 200 g/L in modified M9 minimal medium were sterilized using 0.22 μm cut-off filters and aliquots were stored at −20°C.
Culture and Growth Conditions
Pseudomonas aeruginosa PAO1 was grown overnight at 37°C in minimum M9 medium. The resulting cultures were then used to inoculate 50 ml of minimum M9 medium supplemented by 5 mM of citrate (MM9) (in 250 ml Erlenmeyer flasks) to an initial OD600 of 0.01 and incubated with shaking at 200 rpm at 37°C. When cultures reached OD600 = 0.05, FOS or inulin were added to a final concentration of 20 mg/ml and cultures were harvested for analysis 1 h later.
RNA Extraction, Library Preparation and RNA Sequencing
Total RNA was extracted with the TRI reagent (Ambion) using the manufacturer’s instructions. The RNase inhibitor RiboLock (Fermentas) was added to the samples and DNA was removed by treatment with DNase I (Fermentas). The integrity of the RNA samples was assessed with an Agilent 2100 Bioanalyzer (Agilent Technologies). Subsequently, the 23S, 16S, and 5S rRNAs were removed by subtractive hybridization using the MICROBExpress kit (Ambion) following the protocol reported by Gómez-Lozano et al. (2014). Removal of the rRNA was confirmed by an analysis with an Agilent 2100 Bioanalyzer (Agilent Technologies). Sequencing libraries were prepared using the TruSeq Stranded mRNA Sample Preparation kit (Illumina). After each step, the samples were validated using an Agilent 2100 Bioanalyzer (Agilent Technologies) and the final RNA concentration was measured using a Qubit 2.0 Fluorometer (Invitrogen). The libraries were sequenced using the Illumina HiSeq2000 platform with a paired-end protocol and read lengths of 100 nucleotides.
RNA-seq Analysis
The quality of sequenced reads was assessed using FastQC software, version 0.11.5 (Andrews and Fast, 2010). Single-end reads were aligned to the reference genome of P. aeruginosa PAO1 (GenBank accession number: AE004091.2) using SAMtools v 0.1.19 (Li et al., 2009). BAM files from SAM tools were used as input for the feature counts function (Liao et al., 2014) from the Rsubread package (Liao et al., 2013) of Bioconductor version 3.5 to generate a matrix of annotated genes with their corresponding raw counts. An average of 84.5% reads were successfully mapped to the reference genome. The count data were then analyzed to look for differential gene expression levels and statistical significance using DEseq2 (Love et al., 2014; Kimberly and Stephen, 2015).
The threshold to define differences in transcript levels was a statistical Log2 fold change. Genes were considered significantly differentially expressed when p-values were below 0.05.
RNA-Sequencing Data Registration Number
The sequence reads have been deposited in the GEO database under accession N°: GSE124468. The following secure token has been created to allow for the review of record GSE124468: glshocwuzvkprop.
Analysis of Gene Expression by Quantitative Real Time PCR
Quantitative real time-PCR experiments were performed to validate RNA-seq results. Total RNA was obtained by the TRI reagent® /BCP method (Ambion). One μg of RNA was retrotranscribed following the protocol provided in the manufacturer protocol (iScript BioRad, Alcobendas, Spain) and DNA sequences were amplified with a MX3005P real time PCR instrument (Stratagene) using the primers listed in Supplementary Table S3. The genes of interest were amplified by PCR using the Go Taq@qPCR Master Mix (Promega, Madison, WI, United States) as well as 1 μl of the cDNA template and the primers listed in Supplementary Table S3. Forty PCR cycles were conducted using an annealing temperature of 61°C. The cycle threshold values were normalized to that of the reference transcript, 16S RNA, and data were normalized to the control.
Generation of Mutants in Genes of the Genomic Island PA0643, PA0644, and PA0646
To generate the PA0643:Gm, PA0644:Gm, and PA0646:Gm mutants 656, 241, and 636 pb DNA fragments, respectively, covering the central part of the genes were amplified by PCR from P. aeruginosa PA01 genomic DNA. The resulting products were cloned into plasmid pMBL to yield plasmids pMBL:PA0643, pMBL:PA0644, and pMBL:PA0646. Subsequently, the resulting plasmids were digested with BamHI, which liberated the PA0643, PA0644, and PA0646 fragment. The plasmid pCHESI was also digested with BamHI, to liberate the gentamicin resistance gene (Gm) in order to ligate it with the three DNA fragments. The resulting chimeric DNA was cloned into pMBL digested with BamHI, to yield pMBL:PA0643ΩGm, pMBL:PA0644ΩGm and pMBL:PA0646ΩGm. The resulting plasmids were electroporated into P. aeruginosa PA01 for homologous double recombination. Mutant strains were selected on Gm plates and the correctness of the mutation was verified by Southern blotting (Sambrook et al., 1989; Molina-Fuentes et al., 2015).
Semi-Quantitative Determination of Biofilm Formation
Semi-quantitative determination of biofilm formation were performed as previously described (Christensen et al., 1985). P. aeruginosa PA0643, PA0644, and PA0646 mutants were tested in the biofilm-forming capacities in Minimum medium supplemented with 5 mM citrate. The determination of biofilm production was performed after 2, 4, and 6 h of growth by dissolving crystal violet from the biofilm with an ethanol-acetone mixture (70:30) and the absorbance measure at 590 nm.
Motility Assays
Assays were carried out to determine the effect of the PA0643, PA0644, and PA0646 deletion gene on swimming, twitching and swarming. For swimming assays, bacteria were placed with the help of a sterile tooth-pick at the center of plates containing a 5 mm layer of LB medium with 0.3% (w/v) Bacto agar, 0.2% casamino acids (w/v), and 30 mM glucose. Plates were incubated at 37°C for 24 h and the radial diffusion of bacteria, due to swimming, was inspected. To monitor twitching motility, bacteria were placed with a toothpick into a 2 mm thick layer containing 1.5% (w/v) Bacto agar, 0.2% (w/v) casamino acids, and 30 mM glucose. After incubation at 37°C for 24 h, the expansion of bacteria on the plate was observed. For swarming assays, 5 μl of an overnight culture of bacteria were placed in the center of swarm plates, which are made of 0.5% (w/v) Bacto agar supplemented with 0.2% (w/v) casamino acids and 30 mM glucose. Plates were incubated at 37°C for 24 h, followed by an inspection of the surface movement of the bacteria. All motility assays were performed in triplicate.
Statistical Analysis
All results are expressed as means from three cultures with the corresponding standard deviations. Data were analyzed for statistical significance using the one-way ANOVA analysis and a posteriori least significance test. All analyses were carried out with the SigmaStat 2.03 program (Jandel Corporation, San Rafael, CA, United States). Fitting of dose-response curves was done using Origin 7.0 (OriginLab Corporation, Northampton, MA, United States). Differences were considered significant at p < 0.05.
Results
FOS and Inulin Induce Differential Changes in Pseudomonas aeruginosa Transcript Levels
To understand the cellular response of P. aeruginosa PAO1 to FOS and inulin treatment, we conducted RNA-seq studies. Transcriptomic changes were determined in duplicate cultures grown in the absence and in the presence of either FOS or inulin at final concentrations of 20 mg/ml. Between 5,300,000 and up to 7,500,000 reads were obtained for inuline and FOS respectively, of which approximately 85% could be assigned to the 6,322 coding regions of the P. aeruginosa PAO1 reference genome (Table 1).
The heat map shown in Figure 1A illustrates genes with the most important alterations in transcript levels in the presence of FOS/inulin as compared to the control. For both compounds the number of genes with increased transcript levels were superior to those with decreased levels (see Figure 1B). FOS and inulin induced changes in the transcript level of 217 and 258 genes respectively, compared to the control to which no polysaccharide was added (Figure 2). Importantly, down changes in transcript levels of 57 and 83 genes were found to be specific for inulin or FOS, respectively (Figure 2A). Moreover, 201 and 134 genes showed an increase in transcript levels by inulin and FOS, respectively (Figure 2B) indicating that both compounds trigger different changes.
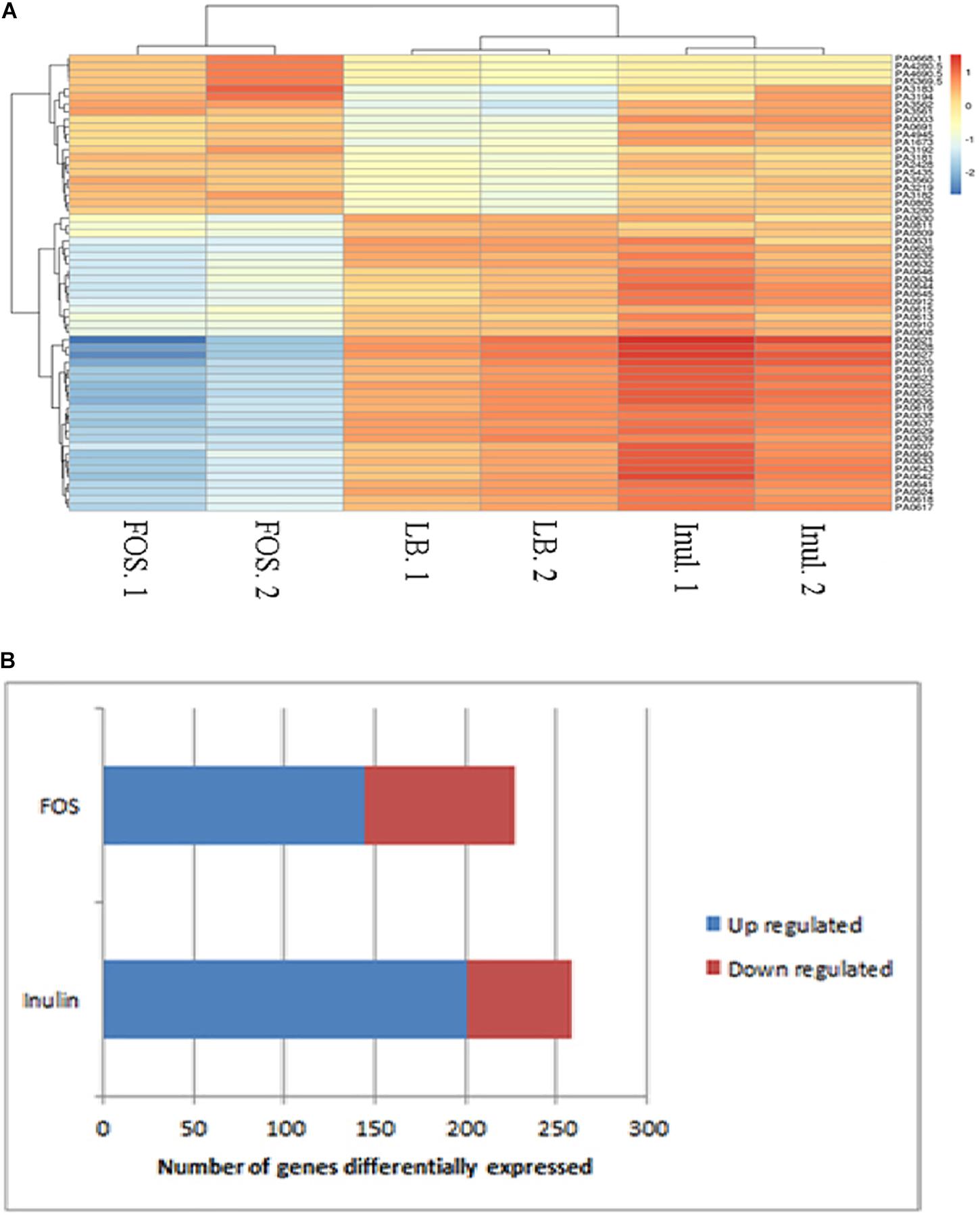
Figure 1. Heat-map (A) analysis and hierarchical cluster (B) of the genes that are differentially induced and repressed in the presence of FOS or inulin as compared to the untreated control (M9 minimal medium). Blue: genes with increased expression; red: genes with reduced expression.
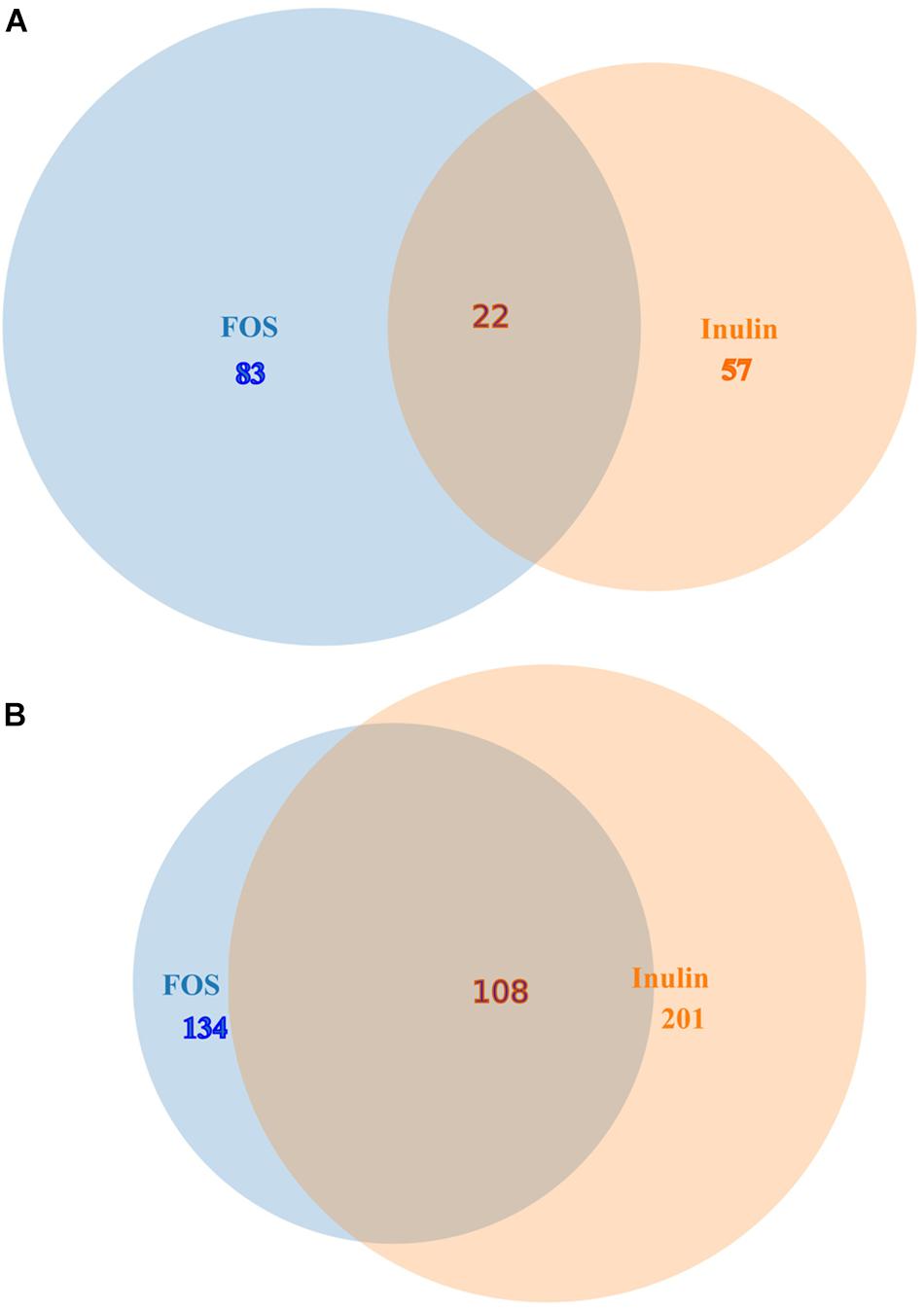
Figure 2. Venn diagrams comparing gene transcript levels in the presence of inulin and FOS. (A) Genes down regulated by inulin and FOS. (B) Genes up regulated by inulin and FOS.
Supplementary Tables S1 and S2 show the list of genes for which the expression level significantly increased or decreased in the presence of 20 mg/ml of inulin (Supplementary Table S1) and FOS (Supplementary Table S2). Analysis of the expression pattern showed that only 22 genes had decreased levels in the presence of both compounds (Figure 2A and Table 2). Among these genes the most prominent changes were observed for genes involved in: (1) Organic acid transport such as PA1342 (aatj), which encodes a C4-dicarboxylate transport protein and PA1183 (dctA), (2) Central metabolism, like a PA0795 (prpC), which regulates a citrate synthase, PA2008 (fahA) that controls a fumarylacetoacetase, and PA1585 (sucA) encoding a 2- oxoglutatate dehydrogenase, (3) Oxidative stress such as superoxide dismutase PA4366 and (4) Virulence system like the Type VI secretion ATPase (PA0090). These genes were outnumbered by the genes for which both compounds caused an increase in transcript levels (Figure 2 and Table 3).
A significant number of these genes appear to be involved in sensing (transcriptional regulators, sensor kinases, and chemotaxis transducers), motility, glucose metabolism as well as control of transcription and protein synthesis (Figure 2 and Table 3).
Functional Analysis of Pseudomonas aeruginosa Transcriptome Following Exposure to FOS and Inulin
GO terms (WEGO) enrichment analyses were conducted to characterize the DEG (Differentially expressed genes) profiles and K-means clustering was performed to further investigate their biological function. We found that the differentially expressed genes can be classified into 32 categories that belonged to three gene ontology (GO) categories, i.e., the biological process, the cellular component or the molecular function (Figure 3). There were more genes classified into biological processes than the other two categories and most genes were predicted to have a binding function, as these genes are primarily involved in protein metabolism (Figure 3).
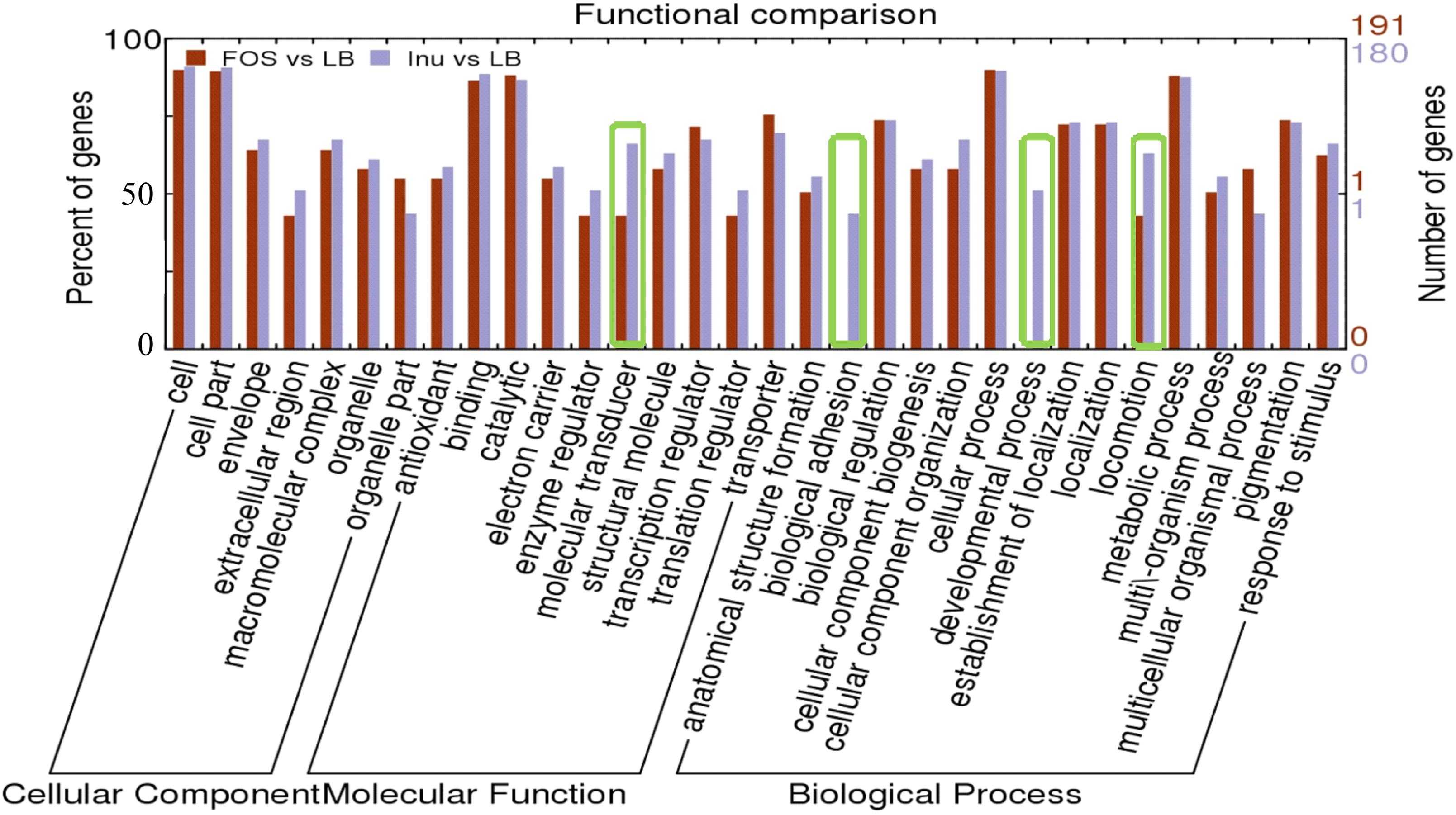
Figure 3. Functional comparison of differentially expressed genes in the presence of FOS and inulin as compared to the control. Functions are organized into three groups: Cellular components, Molecular Function, and Biological Process. The functional groups with the main differences between FOS and inulin are “Molecular transducer,” “Biological adhesion” “Developmental process” and “locomotion system.”
The over-expressed DEGs were assigned to 15 GO categories based on biological processes (Figure 3) and the results showed that the response to the stimulus, metabolic process, biological regulation, the establishment of localization and pigmentation were among the most highly represented groups in the biological process category in the presence of inulin or FOS. However, “biological adhesion” and “developmental process” showed a drastic decrease in the number of genes between inulin or FOS samples. While in the “locomotion process” most of the inhibited genes (43%) are annotated in FOS samples (Figure 3).
Furthermore, DEGs were assigned to seven GO categories based on cellular component and the result showed that “Cell” and “Cell part” are similar and highly represented groups for FOS and inulin samples (Figure 3). However, the “extracellular region” and the “organelle part” are lower and distinctly represented in the presence of inulin or FOS (Figure 3).
Genes with altered transcript levels could be grouped into 10 GO terms with different molecular functions of which the categories “antioxidant,” “binding,” “electron carrier,” “transcription regulator,” “structural molecular”, and “transcription regulator” were most populated (Figure 3). It is worth noting that, in the “molecular transducer” category a significantly higher number of genes were noted for inulin as compared to FOS. The individual genes that were classified into these different GO terms are provided in Supplementary Tables S1, S2.
Differential Gene Expression Pattern in the Presence of FOS
The differential expression analysis performed with DESeq2 in the presence of FOS showed that 83 gene transcript levels were reduced (Table 4), whereas 134 were increased (Figure 2 and Table 5). A large number of genes with reduced transcript levels (43%) were annotated as hypothetical proteins of uncharacterized functions (Table 4). We observed a decrease in the expression of genes related to (1) Metabolic pathways like citrate synthase (prpC), glutaminase-asparaginase (ansB), glutamylpolyamine synthetase (pauA4), and riboflavin synthase (ribC), (2) Transport systems such as PA0811 and PA1342 (aatj) (3) Translation cellular processes like several ribosomal proteins (rplQ, rpsD, rpsK, and rplK) and (4) Virulence such as PA0090 (clpV); PA0612 (ptrB), PA3866 (pyocin S4), and PA4370 (icmP) (see Table 4).
Surprisingly, in addition to increasing the expression of genes involved in different metabolic pathways necessary for bacterial growth, we found that transcript levels of many genes that are related to P. aeruginosa motility were increased in the presence of FOS (Table 5), exemplified by genes involved in twitching motility (pilG), components of the flagellar motor or chemoreceptors including CtpL that mediates specific taxis to inorganic phosphate – a key regulator of P. aeruginosa virulence (Zaborin et al., 2009; Bains et al., 2012). In the same manner, transcript levels of many genes associated within the glucose metabolism were also increased including genes eda, zwf, gapA, glk, edd, or gltR (Table 5) suggesting that P. aeruginosa can catabolize these compounds.
Effect of FOS on Virulence Related Gene Transcript Levels
All the DEGs were mapped to KO terms in the KEGG database to identify FOS modulated genes that play a role in bacterial virulence, motility, or sensitivity to antibiotics and the corresponding genes are provided in Table 6.
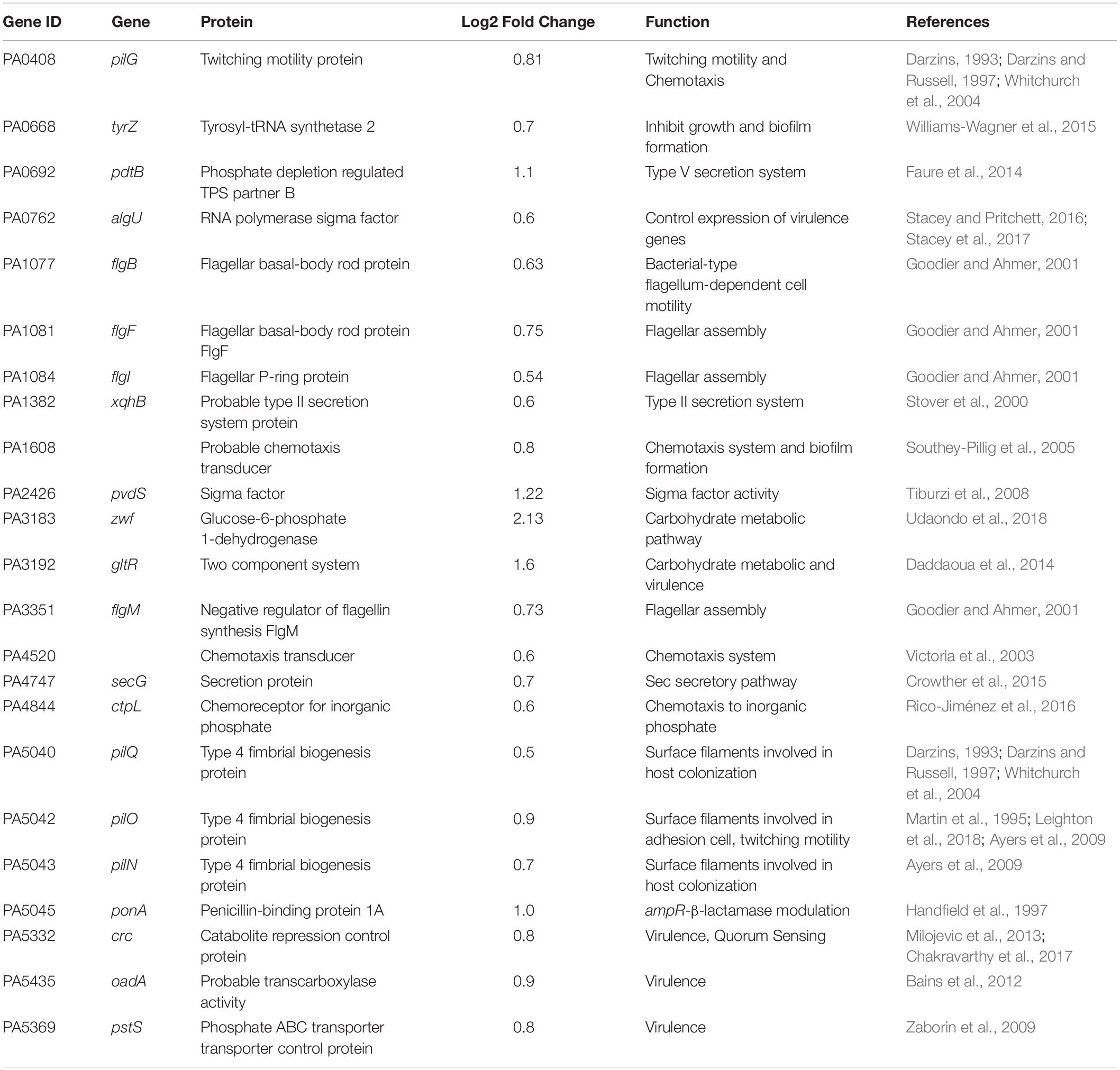
Table 6. Genes with increased transcript levels in response to FOS that are related to bacterial pathogenicity.
Noteworthy are the increases observed for transcript levels of PA0668 which encodes a TyrZ: Tyrosyl-tRNA synthetase2 that inhibits growth and biofilm formation (Williams-Wagner et al., 2015), genes of the pilMNOPQ operon, which are important for the pilus assembly system (T4P) and promote surface-associated attachment (Ayers et al., 2009; Tammam et al., 2013; McCallum et al., 2016) showed increased transcript levels like pilG (PA0408) (Log2 fold = 0.8); pilQ (PA5040) (0.5 Log2 fold); pilO (PA5042) (0.9 Log2 fold); pilN (PA5043) (0.7 Log2 fold); or different genes of the flg operon that encode proteins of the flagellar motor such as flgB (PA1077) (0.6 Log2 fold); flgF (PA1081) (0.7 Log2 fold); flgI (PA1084) (0.5 Log2 fold); and flgM (PA3351) (0.7 Log2 fold). Moreover, the transcript levels of three chemoreceptors were increased in the presence of FOS, of which two (PA1608, PA4520) are of unknown function, whereas PA4844 (ctpl) encodes a specific chemoreceptor for inorganic phosphate (Rico-Jiménez et al., 2016). In addition, the zwf1 gene encoding a glucose-6-phosphate dehydrogenase that is involved in P. aeruginosa virulence (Udaondo et al., 2018) showed much higher transcript levels (2.13 Log2 fold) in the presence of FOS. In the same manner, transcript levels of gltR encoding the response regulator of the GtrS/GltR two component system (TCS) were significantly increased (1.6 Log2 fold) (Table 6). This TCS was found to regulate the expression of toxA encoding the primary virulence factor exotoxin A (Udaondo et al., 2018).
However, the results (Table 7) show several virulence related genes with reduced transcript levels, such as PA0090 encoding the ClpV1 protein, involved in the type VI secretion system (Bönemann et al., 2009), or the PtrB component of the type III secretion system (TTSS) that coordinates TTSS repression and pyocin synthesis under DNA damage (Weihui and Shouguang, 2005). The latter observation agrees with the reduced transcript level of the gene (PA3866) encoding the pyocin S4 (Ameer et al., 2012).
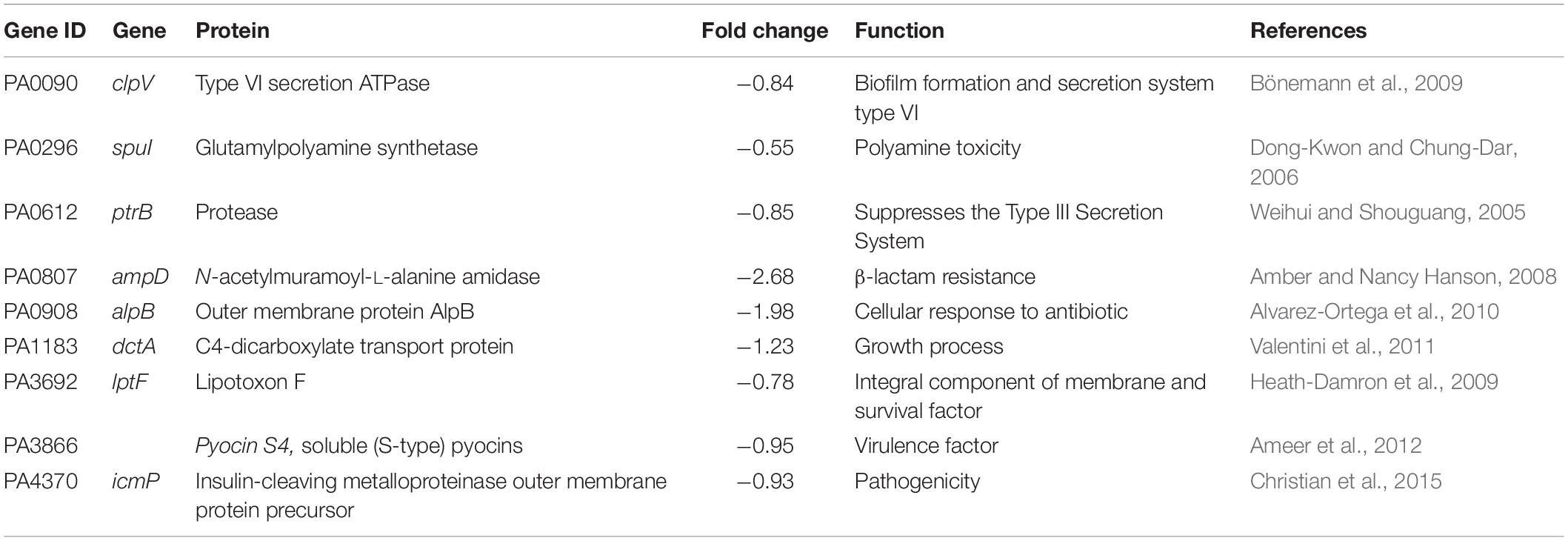
Table 7. Genes with decreased transcript levels in response to FOS that are related to bacterial pathogenicity.
In addition, the results shown in Table 7 reveal reduced transcript levels for PA3692 (lptF), encoding an outer membrane protein (alipotoxon), which plays a key role in P. aeruginosa survival under harsh environmental conditions, including lung colonization in patients with cystic fibrosis (Heath-Damron et al., 2009). Furthermore, the reduction in transcript levels for PA0807 (ampD) and PA0908 (alpB), encoding proteins involved in responses to antibiotics, or in PA0296, involved in polyamine toxicity, may indicate that FOS modulates sensitivity to antibiotics (Amber and Nancy Hanson, 2008; Shah and Swiatlo, 2008; Alvarez-Ortega et al., 2010) and polyamines (Dong-Kwon and Chung-Dar, 2006). These data have been confirmed by real time quantitative PCR (rt-qPCR) experiments (Table 8).
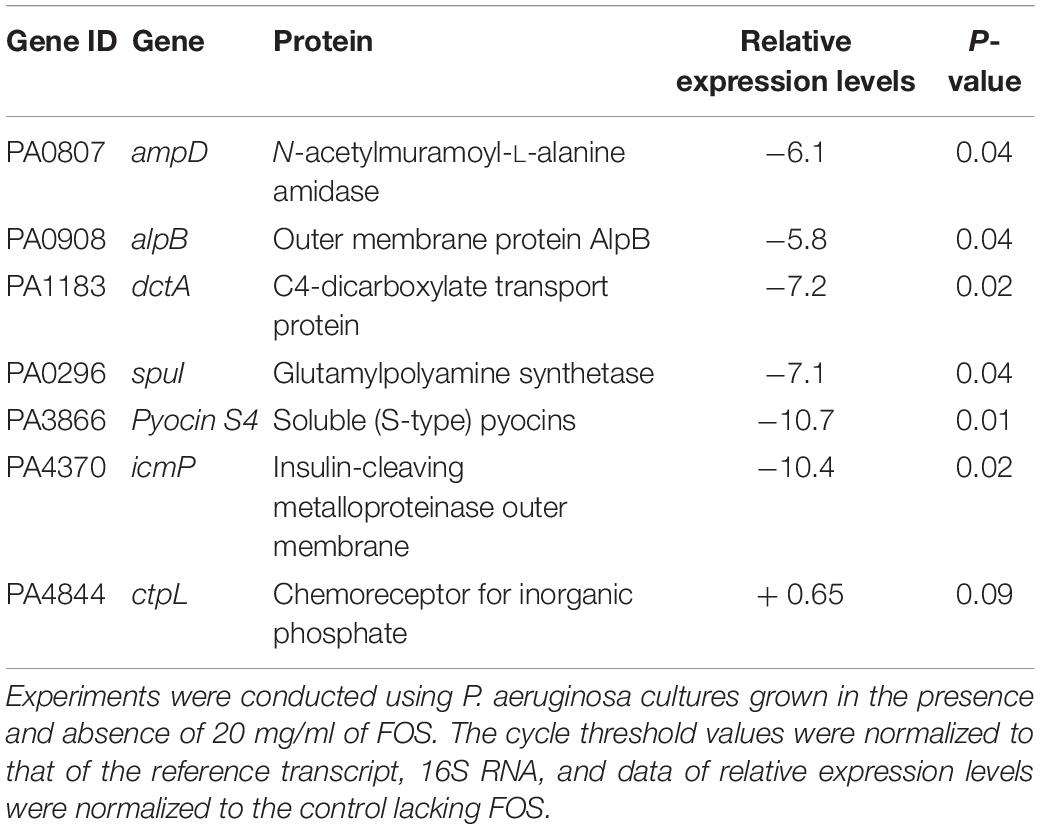
Table 8. Quantitative real time PCR experiments to quantify the effect of FOS on the transcript levels of genes that belong to the genomic island and that are related to bacterial pathogenicity in P. aeruginosa.
Moreover, FOS treatment resulted in lower transcript levels of the dctA gene (PA1183) which is associated with the normal growth of P. aeruginosa (Valentini et al., 2011) and of the icmP gene (PA4370) encoding an metalloproteinase outer membrane protein, which has been shown to degrade the plasminogen activator (Christian et al., 2015) and plays a key role in the Pseudomonas aeruginosa pathogenicity. These FOS mediated alterations in transcript levels have been confirmed by rt-qPCR studies (see Table 8). Altogether, data suggest that FOS acts as a signal molecule that modulates bacterial virulence through distinct signaling pathways.
Confirmation That FOS Reduces Expression of Structural Proteins of Secretion System III and VI
To confirm that FOS mediates changes in secretion system genes, we have conducted rt-qPCR experiments to determine the influence of FOS and inulin on the expression of 4 genes that encode proteins that are part of type III and VI secretion systems. The products of the pcrV and exsA genes control the activation of the type III secretion system (Lee et al., 2010), whereas the proteins encoded by hcp1 and vgrG1 are necessary for the type VI secretion system (Hachani et al., 2011).
We found that inulin caused a significant increase in pcrV and exsA transcript levels, whereas those of hcp1 and vgrG1 did not vary (Figure 4A). In contrast, the expression of exsA and hcp1 were dramatically down regulated by factors of approximately 20 and 7, respectively, in the presence of FOS (Figure 4B). FOS but not inulin down regulated the expression of two components of the type III and VI secretion system.
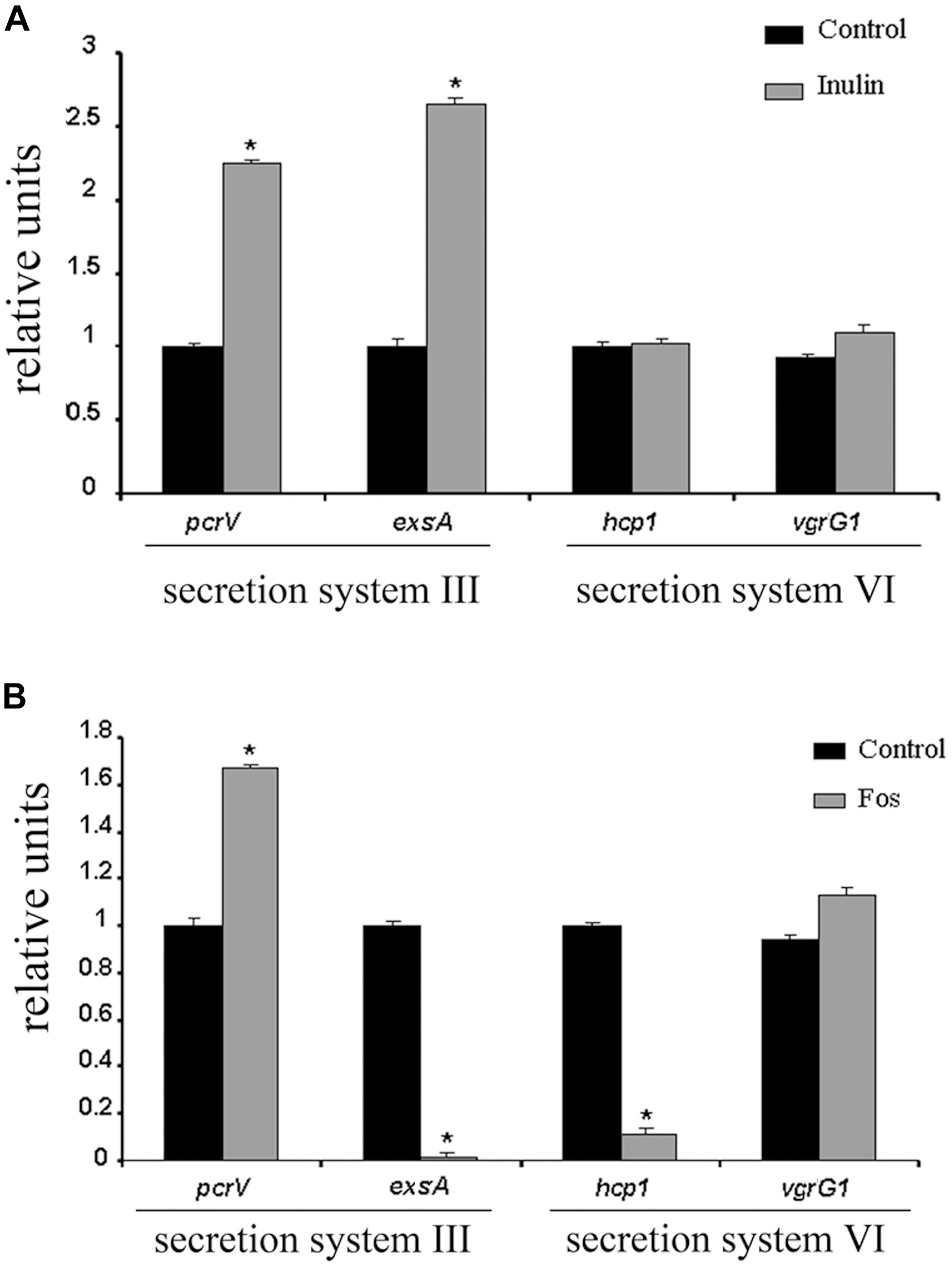
Figure 4. Quantitative RT-PCR validations of the effect of FOS and inulin on the expression of secretion system genes. rt-qPCR studies of P. aeruginosa of cultures grown in the presence and absence of 20 mg/ml inulin (A) or FOS (B). The expression of genes encoding proteins of secretion systems III and VI are shown. *P < 0.05 vs. Control.
Identification of Genomics Islands Widely Repressed by FOS-Treatment in Pseudomonas aeruginosa PAO1
Due to their relevance to human health, extensive efforts have been made to study genomic islands, which are large genetic elements acquired through horizontal transmission (Juhas et al., 2009; Stephen and Julian, 2015; Mao and Lu, 2016).
Our data, using RNAseq sequence analysis, indicates the presence of a genomic island in PAO1 of ∼17 kb comprising 15 genes that had been inserted into the 3′-end of the PA0639 gene (Figure 5) through a phage encoded R2/F2 pyocin (Chang et al., 2005). Most of its genes were clearly repressed in the presence of FOS. Unfortunately, most of these genes code for proteins with unknown functions. Furthermore, in order to determine the biological role of this genomic island, various isogenic mutants were constructed and submitted to a phenotypic analysis that investigates changes in growth, biofilm formation, and motility. Interestingly, PA0643, PA0644, and PA0646 mutants showed reduced growth inhibition compared to the wild-type strain (Figure 6). In addition, while the PA0643 mutant strain did not cause any significant changes, the PA0644 and PA0646 isogenic mutant demonstrated a reduction in biofilm formation at 4 and 6 h (Figure 7). Moreover, PA0643, PA0644, and PA0646 mutants were tested for their ability to swim, swarm, and twitch and the results showed that the mutants exhibited differences in all three types of motility (Figure 8). While PA0643, PA0644, and PA0646 mutants caused the same reduced change in swarming motility, it was found that PA0644 and PA0646 mutants significantly inhibited (at least 50–60% of WT) the swimming motility. Interestingly, the twitching motility has been drastically reduced in the case of the PA0646 mutant (Figure 8). Markedly, this is the first study which shows that the deletion of PA0643, PA0644, and PA0646 genes are able to block P. aeruginosa swarming, swimming, and twitching motility. However, further studies are required to elucidate the function of these proteins.
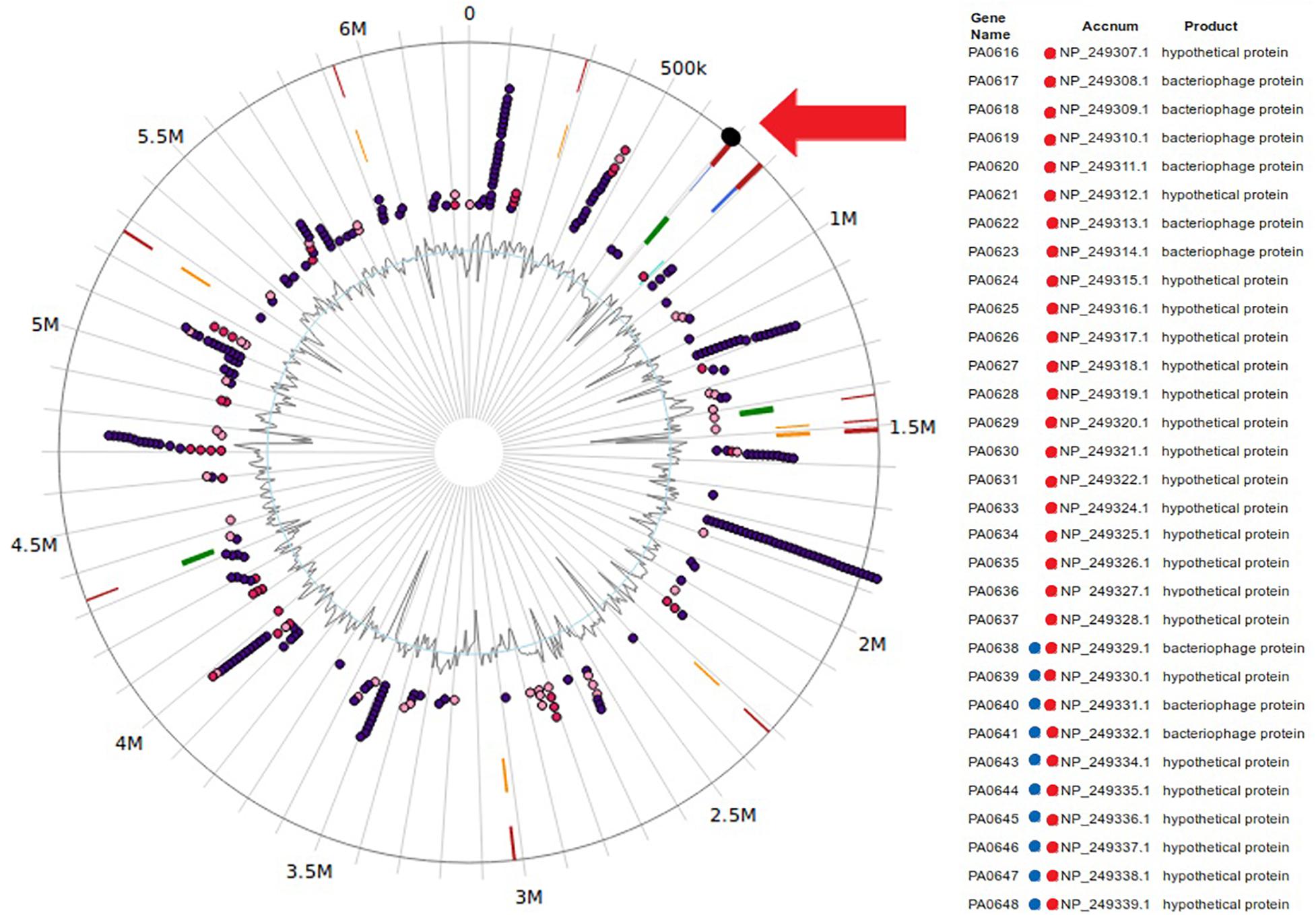
Figure 5. Location of the genomics island with genes widely repressed by FOS-treatment in Pseudomonas aeruginosa PAO1 genome. Figure were made using IslandViewer4 (Bertelli et al., 2017). Genes ids with a red dot represent genes repressed in presence of FOS treatment. Genes with blue dot represent genes identified in a genomic island in P. aeruginosa PAO1 genome.
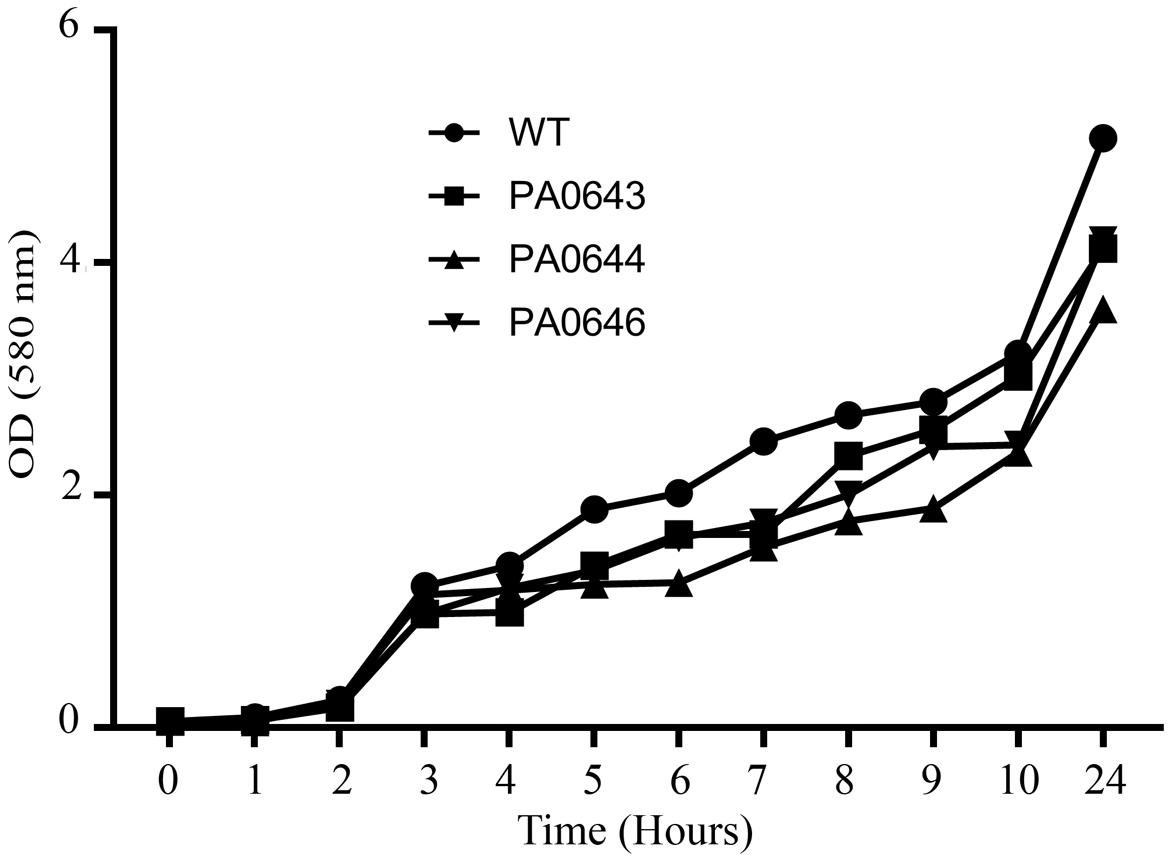
Figure 6. The effect of PA0643, PA0644, and PA0646 deletion on the growth of P. aeruginosa PAO1. Shown are results from growth experiments that were conducted in M9 minimum medium at 37°C for 24 h. Representative data from one of three independent experiments with similar results are shown.
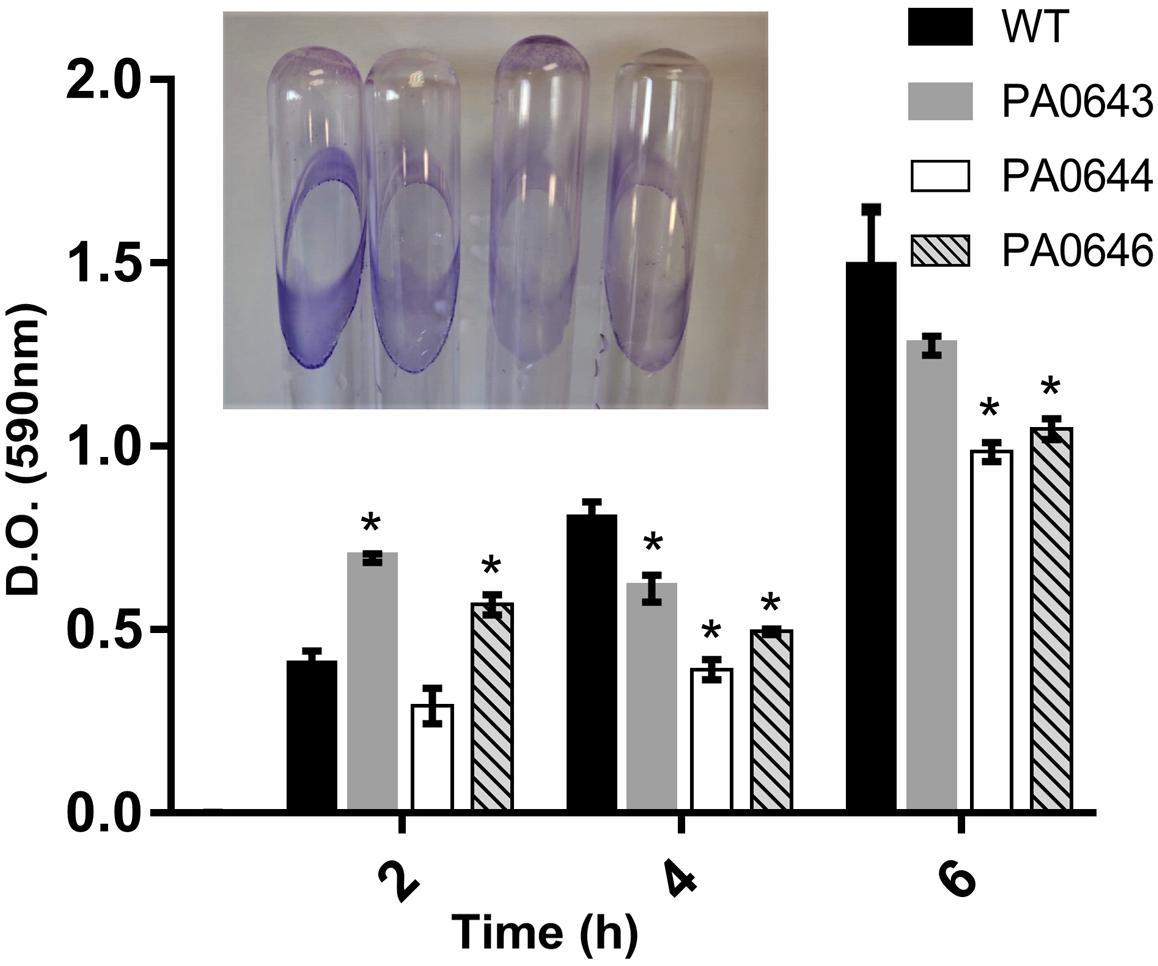
Figure 7. The effect of PA0643, PA0644, and PA0646 deletion on biofilm formation of P. aeruginosa PAO1. Biofilm formation was monitored in minimum medium supplemented with 5 mM of citrate and quantified after 2, 4, and 6 h. The OD at 590 nm of crystal violet (CV) stained and resuspended bacteria from biofilms was recorded. Shown are means and standard deviations with n = 3–6; *P < 0.05 vs. WT.
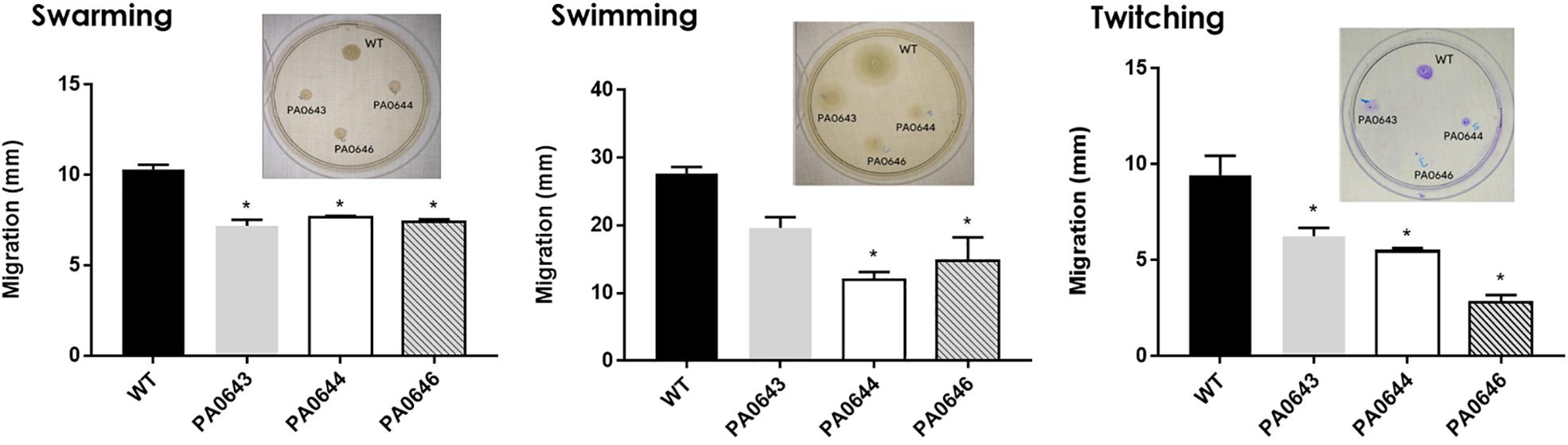
Figure 8. Effect of PA0643, PA0644, and PA0646 deletion on the motility of P. aeruginosa PAO1. Motility assays were carried out as described in section “Materials and Methods.” Shown are means and standard deviations with n = 3–6; *P < 0.05 vs. WT.
Discussion
Various factors appear to be implicated in the ability of P. aeruginosa to cause health problems. While surface structures, including pili and the polysaccharide, seem to favor biofilm formation and adhesion of P. aeruginosa to host cells (Pollack, 1984/1992; Prince, 1992) promoting colonization, its exotoxins in combination with various secretion systems deteriorate the host’s defenses. The identification of signal molecules and the study of their corresponding molecular mechanism, which is associated with these processes, are of utmost interest.
Prebiotics have been shown to exert beneficial effects on human health by altering the intestinal microbiota and also by inhibiting the progression of some pathogenic strains (Knol et al., 2005), due to an indirect effect caused by the selective growth of host friendly bacteria. To our knowledge, antimicrobial properties have been described for a number of oligosaccharides (Daddaoua et al., 2006) and in a previous study, it was shown that FOS as a prebiotic had specific effects on P. aeruginosa, since it reduced growth, limited the formation of biofilm, impaired motility, and reduced the inflammatory response (Ortega-González et al., 2014). Although, inulin and FOS are structurally related oligosaccharides, they differ in chain length and both compounds can be used as a carbon and energy source for P. aeruginosa growth.
The present study demonstrates that FOS induces a number of important changes in P. aeruginosa transcript levels some of which are related to bacterial survival (Figure 1) and provides an initial insight into the corresponding molecular mechanisms. As shown in Figure 2, in the 162 genes with reduced transcript levels by FOS and inulin, only 13% of them were affected by both compounds. Similarly, of the 443 genes with increased levels only 24% of gene transcript levels were altered by both compounds. Therefore, these compounds can be considered as signal molecules; however, their molecular mechanisms remain unknown.
RNA-Seq and rt-qPCR based comparative RNA profiling of P. aeruginosa PAO1 in the presence of FOS or inulin, not only highlighted known functions required for survival metabolism, but revealed a decrease in transcript levels of genes associated with carbohydrate metabolism and growth such as PA1183 (dctA) which has been shown to be associated previously with bacterial growth (Valentini et al., 2011). Thus, the growth reduction may be due to a reduction in transcript levels of genes associated with carboxylic acid transport, carboxylic acid metabolism, and reduction in ribosomal proteins.
Furthermore, these studies revealed a decrease of spuI gene transcript levels (PA0296), confirmed by rt-qPCR (Table 8), which control the expression of polyamine toxicity and of pyocins (PA3866), which are virulence factors that are induced by DNA-damaging agents, such as UV light and mitomycin C (Dean-Scholl and Martin, 2008).
The current analysis clearly shows that the pathogenesis of P. aeruginosa in the presence of FOS is compromised due to a decrease in several virulence-associated genes; i.e., alpB (PA0908), a holin-like protein that is required for lysis and the icmP gene (PA4370), which has been shown to degrade plasminogen activator (Christian et al., 2015) and may play a role in P. aeruginosa pathogenicity (Tables 5, 8).
Secretion of exotoxins through type III secretion systems (T3SSs) (Filloux, 2011) are related to acute infections in P. aeruginosa, while type VI secretion systems are often associated with chronic infections and biofilm formation (Silverman et al., 2012).
Here we show by rt-qPCR that FOS lowers the transcript levels of exsA encoding a transcriptional activator of the secretion system type (T3SS) as well as those of a key protein necessary for the secretion system type VI (T6SS) function, encoded by the hcp gene (Figure 4).
Interestingly, the RNA seq analysis data coincide with a report that demonstrated that the repression of the ptrB gene (PA0612) is implicated in the regulation of the T3SS under DNA damage stress conditions (Weihui and Shouguang, 2005). In addition, this report demonstrates that a repression of clpV (PA0090) regulates the biofilm formation and T6SS (Table 5). Altogether, our study is consistent with the notion that the FOS mediated reduction in pathogenicity is mediated by the secretion systems III and VI.
Further, we obtained initial data suggesting that FOS modulates bacterial resistance to antibiotics, since FOS reduced the expression of ampD (PA0807) transcription levels which leads to the constitutive hyperproduction of the beta-lactamase AmpC and consequently to an increase of the β-lactam resistance (Lindberg et al., 1987). Furthermore, the data shows that FOS lowers transcript levels of alpB (PA0908) a gene product which is related to cell responses to antibiotics (Alvarez-Ortega et al., 2010).
The RNA seq analysis demonstrated the presence of an island with different genes of unknown function, whose expression was completely inhibited in the presence of FOS (Figure 5 and Table 4). The deletion of the PA0643, PA0644, and PA0646 genes caused alterations in bacterial growth (Figure 6), biofilm formation (Figure 7) and motility (Figure 8). The alteration of the transcript levels of this island is thus another possible mechanism by which FOS reduces bacterial pathogenesis.
Conclusion
FOS containing supplements are currently being used to prevent gastrointestinal infections (Yasuda et al., 2012), suggesting that it is a valid strategy to combat Pseudomonas infection by potentially including FOS in antimicrobial cocktails. The present study is a contribution to close the gap of knowledge that exists in the corresponding molecular mechanisms.
Data Availability Statement
All datasets generated for this study are included in the article/Supplementary Material.
Author Contributions
JR-G, CS, and MG provided advice on experimental design. ZU and CS collected and assembled the data. AD designed the study, supervised and analyzed the data, and wrote the manuscript. TK and J-LR revised the manuscript. All authors reviewed and commented on the manuscript.
Funding
This work was supported by grants from the Spanish Ministry for Economy and Competitiveness (AGL2017-85270-R). CS is funded by the program Juan de la Cierva-Formación (FJCI-2015-23810).
Conflict of Interest
The authors declare that the research was conducted in the absence of any commercial or financial relationships that could be construed as a potential conflict of interest.
Supplementary Material
The Supplementary Material for this article can be found online at: https://www.frontiersin.org/articles/10.3389/fmicb.2020.00202/full#supplementary-material
References
Alvarez-Ortega, C., Wiegand, I., Olivares, J., Hancock, R. E., and Martínez, J. L. (2010). Genetic determinants involved in the susceptibility of Pseudomonas aeruginosa to beta lactam antibiotics. Antimicrob. Agents Chemother. 54, 4159–4167 doi: 10.1128/aac.00257-10
Amber, S. J., and Nancy Hanson, D. (2008). Role of ampD homologs in overproduction of AmpC in clinical isolates of Pseudomonas aeruginosa. Antimicrob. Agents Chemother. 52, 3922–3927. doi: 10.1128/AAC.00341-08
Ameer, E., Qing, W., and Pierre, C. (2012). The soluble pyocins S2 and S4 from Pseudomonas aeruginosa bind to the same FpvAI receptor. Microbiologyopen 1, 268–275. doi: 10.1002/mbo3.27
Amer, L. S., Bishop, B. M., and Van Hoek, M. L. (2010). Antimicrobial and antibiofilm activity of cathelicidins and short, synthetic peptides against Francisella. Biochem. Biophys. Res. Commun. 396, 246–251. doi: 10.1016/j.bbrc.2010.04.073
Andrews, S., and Fast, Q. C. (2010). A Quality Control Tool for High Throughput Sequence Data. Available at: http://www.bioinformatics.babraham.ac.uk/projects/fastqc/
Anyan, M. E., Amiri, A., Harvey, C. W., Tierra, G., Morales-Soto, N., Driscoll, C. M., et al. (2014). Type IV pili interactions promote intercellular association and moderate swarming of Pseudomonas aeruginosa. Proc. Natl. Acad. Sci. U.S.A. 50, 18013–18018. doi: 10.1073/pnas.1414661111
Ayers, M., Sampaleanu, L. M., Tammam, S., Koo, J., Harvey, H., Howell, P. L., et al. (2009). PilM/N/O/P proteins form an inner membrane complex that affects the stability of the Pseudomonas aeruginosa type IV pilus secretin. J. Mol. Biol. 394, 128–142. doi: 10.1016/j.jmb.2009.09.034
Bains, M., Fernandez, L., and Hancock, R. E. (2012). Phosphate starvation promotes swarming motility and cytotoxicity of Pseudomonas aeruginosa. Appl. Environ. Microbiol. 78, 6762–6768. doi: 10.1128/AEM.01015-12
Bertelli, C., Laird, M. R., Williams, K. P., Simon Fraser University Research Computing Group, Lau, B. Y., Hoad, G., et al. (2017). IslandViewer 4: expanded prediction of genomic islands for larger-scale datasets. Nucleic Acids Res. 45, W30–W35.
Bönemann, G., Pietrosiuk, A., Diemand, A., Zentgraf, H., and Mogk, A. (2009). Remodelling of VipA/VipB tubules by ClpV-mediated threading is crucial for type VI protein secretion. EMBO J. 28, 315–325. doi: 10.1038/emboj.2008.269
Bosscher, D., Van Loo, J., and Franck, A. (2006). Inulin and oligofructose as prebiotics in the prevention of intestinal infections and diseases. Nutr. Res. Rev. 19, 216–226. doi: 10.1017/S0954422407249686
Breidenstein, E. B., de la Fuente-Nunez, C., and Hancock, R. E. (2011). Pseudomonas aeruginosa: all roads lead to resistance. Trends Microbiol. 19, 419–426. doi: 10.1016/j.tim.2011.04.005
Buhl, M., Peter, S., and Willmann, M. (2015). Prevalence and risk factors associated with colonization and infection of extensively drug-resistant Pseudomonas aeruginosa: a systematic review. Expert Rev. Anti Infect. Ther. 13, 1159–1170. doi: 10.1586/14787210.2015.1064310
Cano, P. G., Santacruz, A., Trejo, F. M., and Sanz, Y. (2013). Bifidobacterium CECT 7765 improves metabolic and immunological alterations associated with obesity in high-fat diet-fed mice. Obesity (Silver Spring) 21, 2310–2321. doi: 10.1002/oby.20330
Capitán-Cañadas, F., Ocón, B., Aranda, C. J., Anzola, A., Suárez, M. D., Zarzuelo, A., et al. (2016). Fructooligosaccharides exert intestinal anti-inflammatory activity in the CD4+ CD62L+ T cell transfer model of colitis in C57BL/6J mice. Eur. J. Nutr. 55, 1445–1454. doi: 10.1007/s00394-015-0962-6
Capitán-Cañadas, F., Ortega-González, M., Guadix, E., Zarzuelo, A., Suárez, M. D., de Medina, F. S., et al. (2013). Prebiotic oligosaccharides directly modulate proinflammatory cytokine production in monocytes via activation of TLR4. Mol. Nutr. Food Res. 58, 1098–1110. doi: 10.1002/mnfr.201300497
Chakravarthy, S., Butcher, B. G., Liu, Y., D’Amico, K., Coster, M., and Filiatrault, M. J. (2017). Virulence of Pseudomonas syringae pv. tomato DC3000 is influenced by the catabolite repression control protein Crc. Mol. Plant Microbe Interact. 30, 283–294. doi: 10.1094/MPMI-09-16-0196-R
Chang, W., Small, D. A., Toghrol, F., and Bentley, W. E. (2005). Microarray analysis of Pseudomonas aeruginosa reveals induction of pyocin genes in response to hydrogen peroxide. BMC Genomics 6:115. doi: 10.1186/1471-2164-6-115
Christensen, G. D., Simpson, W. A., Younger, J. J., Baddour, L. M., Barrett, F. F., Melton, D. M., et al. (1985). Adherence of coagulase-negative staphylococci to plastic tissue culture plates: a quantitative model for the adherence of staphylococci to medical devices. J. Clin. Microbiol. 22, 996–1006. doi: 10.1128/jcm.22.6.996-1006.1985
Christian, L., Melanie, B., Chaves-Moreno, D., Andreas, O., Christian, H., Stephan, F., et al. (2015). Metaproteomics approach to elucidate host and pathogen protein expression during catheter-associated urinary tract infections (CAUTIs). Mol. Cell. Proteomics 14, 989–1008. doi: 10.1074/mcp.M114.043463
Crowther, G. J., Weller, S. M., Jones, J. C., Weaver, T., Fan, E., Van Voorhis, W. C., et al. (2015). The bacterial sec pathway of protein export: screening and follow-up. J. Biomol. Screen. 20, 921–926. doi: 10.1177/1087057115587458
Daddaoua, A., Molina-Santiago, C., de la Torre, J., Krell, T., and Ramos, J. L. (2014). GtrS and GltR form a two-component system: the central role of 2-ketogluconate in the expression of exotoxin A and glucose catabolic enzymes in Pseudomonas aeruginosa. Nucleic Acids Res. 42, 7654–7663. doi: 10.1093/nar/gku496
Daddaoua, A., Puerta, V., Requena, P., Martinez-Ferez, A., Guadix, E., de Medina, F. S., et al. (2006). Goat milk oligosaccharides are anti-inflammatory in rats with hapten-induced colitis. J. Nutr. 136, 672–676. doi: 10.1093/jn/136.3.672
Darzins, A. (1993). The pilG gene product, required for Pseudomonas aeruginosa pilus production and twitching motility, is homologous to the enteric, single-domain response regulator CheY. J. Bacteriol. 175, 5934–5944. doi: 10.1128/jb.175.18.5934-5944.1993
Darzins, A., and Russell, M. A. (1997). Molecular genetic analysis of type-4 pilus biogenesis and twitching motility using Pseudomonas aeruginosa as a model system–a review. Gene 192, 109–115. doi: 10.1016/s0378-1119(97)00037-1
Dean-Scholl, D., and Martin, D. W. Jr. (2008). Antibacterial efficacy of R-Type pyocins towards Pseudomonas aeruginosa in a murine peritonitis model. Antimicrob. Agents Chemother. 52, 1647–1652. doi: 10.1128/AAC.01479-07
Dong-Kwon, H., and Chung-Dar, L. (2006). Polyamines increase antibiotic susceptibility in Pseudomonas aeruginosa. Antimicrob. Agents Chemother. 50, 1623–1627 doi: 10.1128/aac.50.5.1623-1627.2006
Duseja, A., and Chawla, Y. K. (2014). Obesity and NAFLD: the role of bacteria and microbiota. Clin. Liver Dis. 18, 59–71
Faure, L. M., Garvis, S., De Bentzmann, S., and Bigot, S. (2014). Characterization of a novel two-partner secretion system implicated in the virulence of Pseudomonas aeruginosa. Microbiology 160, 1940–1952. doi: 10.1099/mic.0.079616-0
Filloux, A. (2011). Protein secretion systems in Pseudomonas aeruginosa: an essay on diversity, evolution. and function. Front. Microbiol. 2:155. doi: 10.3389/fmicb.2011.00155
Flemming, H. C., Neu, T. R., and Wozniak, D. J. (2007). The EPS matrix: the “house of biofilm cells”. J. Bacteriol. 189, 7945–7947. doi: 10.1128/jb.00858-07
Froebel, L. K., Jalukar, S., Lavergne, T. A., Lee, J. T., and Duong, T. (2019). Administration of dietary prebiotics improves growth performance and reduces pathogen colonization in broiler chickens. Poult. Sci. 98, 6668–6676. doi: 10.3382/ps/pez537
Gaines, J. M., Carty, N. L., Tiburzi, F., Davinic, M., Visca, P., Colmer-Hamood, J. A., et al. (2007). Regulation of the Pseudomonas aeruginosa toxA, regA and ptxR genes by the iron-starvation sigma factor PvdS under reduced levels of oxygen. Microbiology 153, 4219–4233. doi: 10.1099/mic.0.2007/011338-0
Gibson, G. R., Beatty, E. R., and Wang, X. (1995). Cummings JH Selective stimulation of bifidobacteria in the human colon by oligofructose and inulin. Gastroenterology 108, 975–982. doi: 10.1016/0016-5085(95)90192-2
Gómez-Lozano, M., Marvig, R. L., Tulstrup, M. V. L., and Molin, S. (2014). Expression of antisense small RNAs in response to stress in Pseudomonas aeruginosa. BMC Genomics 15:783. doi: 10.1186/1471-2164-15-783
Goodier, R. I., and Ahmer, B. M. (2001). SirA orthologs affect both motility and virulence. J. Bacteriol. 183, 2249–2258. doi: 10.1128/jb.183.7.2249-2258.2001
Hachani, A., Lossi, N. S., Hamilton, A., Jones, C., and Bleves, S. (2011). Type VI secretion system in Pseudomonas aeruginosa: secretion and multimerization of VgrG proteins. J. Biol. Chem. 286, 12317–12327. doi: 10.1074/jbc.M110.193045
Handfield, J. 1, Gagnon, L., Dargis, M., and Huletsky, A. (1997). Sequence of the ponA gene and characterization of the penicillin-binding protein 1A of Pseudomonas aeruginosa PAO1. Gene 199, 49–56. doi: 10.1016/s0378-1119(97)00345-4
Heath-Damron, F., Napper, J., Allison-Teter, M., and Hongwei, D. Yu. (2009). Lipotoxin F of Pseudomonas aeruginosa is an AlgU-dependent and alginate-independent outer membrane protein involved in resistance to oxidative stress and adhesion to A549 human lung epithelia. Microbiology 155, 1028–1038. doi: 10.1099/mic.0.025833-0
Hoiby, N., Ciofu, O., and Bjarnsholt, T. (2010). Pseudomonas aeruginosa biofilms in cystic fibrosis. Future Microbiol. 5, 1663–1674. doi: 10.2217/fmb.10.125
Juhas, M. (2015). Pseudomonas aeruginosa essentials: an update on investigation of essential genes. Microbiology 161, 2053–2060. doi: 10.1099/mic.0.000161
Juhas, M., Van der Meer, J. R., Gaillard, M., Harding, R. M., Hood, D. W., and Crook, D. W. (2009). FEMS genomic islands: tools of bacterial horizontal gene transfer and evolution. Microbiol. Rev. 33, 376–393. doi: 10.1111/j.1574-6976.2008.00136.x
Kimberly, R. K., and Stephen, M. B. (2015). RNA sequencing and analysis. Cold Spring Harb. Protoc. 11, 951–969.
Klausen, M., Heydorn, A., Ragas, P., Lambertsen, L., and Aaes-Jorgensen, A. (2003). Biofilm formation by Pseudomonas aeruginosa wild type, flagella and type IV pili mutants. Mol. Microbiol. 48, 1511–1524. doi: 10.1046/j.1365-2958.2003.03525.x
Knol, J., Boehm, G., Lidestri, M., Negretti, F., and Jelinek, J. (2005). Increase of faecal bifidobacteria due to dietary oligosaccharides induces a reduction of clinically relevant pathogen germs in the faeces of formula-fed preterm infants. Acta Paediatr. Suppl. 94, 31–33. doi: 10.1111/j.1651-2227.2005.tb02152.x
Lattimer, J. M., and Haub, M. D. (2010). Effects of dietary fiber and its components on metabolic health. Nutrients 2, 1266–1289. doi: 10.3390/nu2121266
Lee, P. C., Stopford, C. M., Svenson, A. G., and Rietsch, A. (2010). Control of effector export by the Pseudomonas aeruginosa type III secretion proteins PcrG and PcrV. Mol. Microbiol. 75, 924–941. doi: 10.1111/j.1365-2958.2009.07027.x
Leighton, T. L., Mok, M. C., Junop, M. S., Howell, P. L., and Burrows, L. L. (2018). Conserved, unstructured regions in Pseudomonas aeruginosa PilO are important for type IVa pilus function. Sci. Rep. 8:2600. doi: 10.1038/s41598-018-20925-w
Li, H., Handsaker, B., Wysoker, A., Fennell, T., Ruan, J., Homer, N., et al. (2009). The sequence alignment/map format and samtools. Bioinformatics 25, 2078–2079. doi: 10.1093/bioinformatics/btp352
Liao, Y., Smyth, G. K., and Shi, W. (2013). The Subread aligner: fast accurate and scalable read mapping by seed-and-vote. Nucleic Acids Res. 41:e108. doi: 10.1093/nar/gkt214
Liao, Y. G. K., Smyth, G. K., and Shi, W. (2014). Feature counts: an efficient general purpose program for assigning sequence reads to genomic features. Bioinformatics 30, 923–930. doi: 10.1093/bioinformatics/btt656
Lindberg, F., Lindquist, S., and Normark, S. (1987). Inactivation of the ampD gene causes semiconstitutive overproduction of the inducible Citrobacter freundii beta-lactamase. J. Bacteriol. 169, 1923–1928. doi: 10.1128/jb.169.5.1923-1928.1987
Love, M. I., Huber, W., and Anders, S. (2014). Moderated estimation of fold change and dispersion for RNA-seq data with DESeq2. Genome Biol. 15:550.
Lynch, J. P. III, Zhanel, G. G., and Clark, N. M. (2017). Emergence of antimicrobial resistance among Pseudomonas aeruginosa: implications for therapy. Semin. Respir. Crit. Care Med. 38, 326–345. doi: 10.1055/s-0037-1602583
Mah, T. F., Pitts, B., Pellock, B., and Walker, G. C. (2003). A genetic basis for Pseudomonas aeruginosa biofilm antibiotic resistance. Nature 426, 306–310. doi: 10.1038/nature02122
Mao, J., and Lu, T. (2016). Population-dynamic modeling of bacterial horizontal gene transfer by natural transformation. Biophys. J. 110, 258–268. doi: 10.1016/j.bpj.2015.11.033
Martin, P. R., Watson, A. A., McCaul, T. F., and Mattick, J. S. (1995). Characterization of a five-gene cluster required for the biogenesis of type 4 fimbriae in Pseudomonas aeruginosa. Mol. Microbiol. 16, 497–508. doi: 10.1111/j.1365-2958.1995.tb02414.x
McCallum, M., Tammam, S., Little, D. J., Robinson, H., Koo, J., Shah, M., et al. (2016). PilN binding modulates the structure and binding partners of the Pseudomonas aeruginosa type IVa pilus protein pilm. J. Biol. Chem. 291, 11003–11015. doi: 10.1074/jbc.M116.718353
Milojevic, T., Grishkovskaya, I., Sonnleitner, E., Djinovic-Carugo, K., and Bläsi, U. (2013). The Pseudomonas aeruginosa catabolite repression control protein Crc is devoid of RNA binding activity. PLoS One 8:e64609. doi: 10.1371/journal.pone.0064609
Miura, K., and Ohnishi, H. (2014). Role of gut microbiota and toll-like receptors in nonalcoholic fatty liver disease. World J. Gastroenterol. 20, 7381–7391. doi: 10.3748/wjg.v20.i23.7381
Miyoshi-Akiyama, T., Tada, T., Ohmagari, N., Viet Hung, N., Tharavichitkul, P., Pokhrel, B. M., et al. (2017). Emergence and spread of epidemic multidrug-resistant Pseudomonas aeruginosa. Genome Biol. Evol. 9, 3238–3245. doi: 10.1093/gbe/evx243
Molina-Fuentes, Á., Pacheco, D., Marín, P., Philipp, B., Schink, B., and Marqués, S. (2015). Identification of the gene cluster for the anaerobic degradation of 3,5-Dihydroxybenzoate (α-Resorcylate) in Thauera aromatica strain AR-1. Appl. Environ. Microbiol. 81, 7201–7214. doi: 10.1128/AEM.01698-15
Natividad, J. M., and Verdu, E. F. (2012). Modulation of intestinal barrier by intestinal microbiota: pathological and therapeutic implications. Pharmacol. Res. 69, 42–51. doi: 10.1016/j.phrs.2012.10.007
Ohara, T., and Itoh, K. (2003). Significance of Pseudomonas aeruginosa colonization of the gastrointestinal tract. Intern. Med. 42, 1072–1076. doi: 10.2169/internalmedicine.42.1072
Ortega-González, M., Sánchez de Medina, F., Molina-Santiago, C., López-Posadas, R., Pacheco, D., Krell, T., et al. (2014). Fructooligosacharides reduce Pseudomonas aeruginosa PAO1 pathogenicity through distinct mechanisms. PLoS One 22:e85772. doi: 10.1371/journal.pone.0085772
Ortiz-Castro, R., Pelagio-Flores, R., Méndez-Bravo, A., Ruiz-Herrera, L. F., Campos-García, J., and López-Bucio, J. (2014). Pyocyanin, a virulence factor produced by Pseudomonas aeruginosa, alters root development through reactive oxygen species and ethylene signaling in Arabidopsis. Mol. Plant Microbe Interact. 27, 364–378. doi: 10.1094/MPMI-08-13-0219-R
Pollack, M. (1984/1992). The virulence of Pseudomonas aeruginosa. Rev. Infect. Dis. 6(Suppl. 3), S617-S626.
Prince, A. (1992). Adhesins and receptors of Pseudomonas aeruginosa associated with infection of the respiratory tract. Microb. Pathog. 13, 251–260. doi: 10.1016/0882-4010(92)90035-m
Rico-Jiménez, M., Reyes-Darias, J.-A., Ortega, Á., Peña, A. I. D., Morel, B., and Tino, K. (2016). Two different mechanisms mediate chemotaxis to inorganic phosphate in Pseudomonas aeruginosa. Sci. Rep. 6:28967. doi: 10.1038/srep28967
Sambrook, J., Fritsch, E. F., and Maniatis, T. (1989). Molecular Cloning: A Laboratory Manual, 2nd Edn. Cold Spring Harbor, NY: Cold Spring Harbor Laboratory.
Sánchez de Medina, F., Ortega-González, M., González-Pérez, R., Capitán-Cañadas, F., and Martínez-Augustin, O. (2013). Host-microbe interactions: the difficult yet peaceful coexistence of the microbiota and the intestinal mucosa. Br. J. Nutr. 109, S12-S20
Shah, P., and Swiatlo, E. (2008). A multifaceted role for polyamines in bacterial pathogens. Mol. Microbiol. 68, 4–16. doi: 10.1111/j.1365-2958.2008.06126.x
Silverman, J. M., Brunet, Y. R., Cascales, E., and Mougous, J. D. (2012). Structure and regulation of the type VI secretion system. Annu. Rev. Microbiol. 66, 453–472. doi: 10.1146/annurev-micro-121809-151619
Southey-Pillig, C. J., Davies, D. G., and Sauer, K. (2005). Characterization of temporal protein production in Pseudomonas aeruginosa biofilms. J. Bacteriol. 187, 8114–8126. doi: 10.1128/jb.187.23.8114-8126.2005
Stacey, S. D., and Pritchett, C. L. (2016). Pseudomonas aeruginosa AlgU contributes to posttranscriptional activity by increasing rsmA expression in a mucA22 Strain. J. Bacteriol. 198, 1812–1826. doi: 10.1128/JB.00133-16
Stacey, S. D., Williams, D. A., and Pritchett, C. L. (2017). The Pseudomonas aeruginosa two-component regulator AlgR directly activates rsmA expression in a phosphorylation independent manner. J Bacteriol. 199:e00048-17. doi: 10.1128/JB.00048-17
Stephen, D. B., and Julian, P. (2015). Genomic perspectives on the evolution and spread of bacterial pathogens. Proc. Biol. Sci. 282:20150488. doi: 10.1098/rspb.2015.0488
Stoodley, P., Sauer, K., Davies, D. G., and Costerton, J. W. (2002). Biofilms as complex differentiated communities. Annu. Rev. Microbiol. 56, 187–209. doi: 10.1146/annurev.micro.56.012302.160705
Stover, C. K., Pham, X. Q., Erwin, A. L., Mizoguchi, S. D., Warrener, P., Hickey, M. J., et al. (2000). Complete genome sequence of Pseudomonas aeruginosa PAO1, an opportunistic pathogen. Nature 406, 959–964.
Tammam, S., Sampaleanu, L. M., Koo, J., Manoharan, K., Daubaras, M., Burrows, L. L., et al. (2013). PilMNOPQ from the Pseudomonas aeruginosa type IV pilus system form a transenvelope protein interaction network that interacts with PilA. J. Bacteriol. 195, 2126–2135. doi: 10.1128/JB.00032-13
Taylor, P. K., Yeung, A. T. Y., and Hancock, R. E. W. (2014). Antibiotic resistance in Pseudomonas aeruginosa biofilms: towards the development of novel anti-biofilm therapies. J. Biotechnol. 191, 121–130. doi: 10.1016/j.jbiotec.2014.09.003
Tiburzi, F., Imperi, F., and Visca, P. (2008). Intracellular levels and activity of PvdS, the major iron starvation sigma factor of Pseudomonas aeruginosa. Mol. Microbiol. 67, 213–227. doi: 10.1111/j.1365-2958.2007.06051.x
Udaondo, Z., Ramos, J., Segura, A., Krell, T., and Daddaoua, A. (2018). Regulation of carbohydrate degradation pathways in Pseudomonas involves a versatile set of transcriptional regulators. Microb. Biotechnol. 11, 442–454. doi: 10.1111/1751-7915.13263
Valentini, M., Storelli, N., and Lapouge, K. (2011). Identification of C4-Dicarboxylate transport systems in Pseudomonas aeruginosa PAO1. J. Bacteriol. 193, 4307–4316. doi: 10.1128/jb.05074-11
Victoria, E. W., Daniel, B., Luciano, P., Brooks, A. I., and Iglewski, B. H. (2003). Microarray analysis of Pseudomonas aeruginosa quorum-sensing regulons: effects of growth phase and environment. J. Bacteriol. 185, 2080–2095. doi: 10.1128/jb.185.7.2080-2095.2003
Von Klitzing, E., Bereswill, S., and Heimesaat, M. M. (2017). Multidrug-resistant Pseudomonas aeruginosa induce systemic pro-inflammatory immune responses in colonized mice. Eur. J. Microbiol. Immunol. 7, 200–209. doi: 10.1556/1886.2017.00022
Weihui, W., and Shouguang, J. (2005). PtrB of Pseudomonas aeruginosa suppresses the type III secretion system under the stress of DNA damage. J. Bacteriol. 187, 6058–6068. doi: 10.1128/jb.187.17.6058-6068.2005
Whitchurch, C. B., Leech, A. J., Young, M. D., Kennedy, D., Sargent, J. L., Bertrand, J. J., et al. (2004). Characterization of a complex chemosensory signal transduction system which controls twitching motility in Pseudomonas aeruginosa. Mol. Microbiol. 52, 873–893. doi: 10.1111/j.1365-2958.2004.04026.x
WHO (2017). Global Priority List of Antibiotic-Resistant Bacteria to Guide Research, Discovery and Development of New Antibiotics. Available at: http://www.who.int/medicines/publications/global-priority-list-antibiotic-resistant-bacteria/en/
Williams-Wagner, R. N., Grundy, F. J., Raina, M., Ibba, M., and Henkin, T. M. (2015). The Bacillus subtilis tyrZ gene encodes a highly selective tyrosyl-tRNA synthetase and is regulated by a MarR regulator and T box riboswitch. J. Bacteriol. 197, 1624–1631. doi: 10.1128/JB.00008-15
Yasuda, A., Inoue, K., Sanbongi, C., Yanagisawa, R., and Ichinose, T. (2012). Dietary supplementation with fructooligosaccharides attenuates allergic peritonitis in mice. Biochem. Biophys. Res. Commun. 422, 546–550. doi: 10.1016/j.bbrc.2012.05.007
Keywords: RNA sequencing, rt-qPCR, adhesion, developmental process, molecular transducer, pathogenicity
Citation: Rubio-Gómez JM, Santiago CM, Udaondo Z, Garitaonaindia MT, Krell T, Ramos J-L and Daddaoua A (2020) Full Transcriptomic Response of Pseudomonas aeruginosa to an Inulin-Derived Fructooligosaccharide. Front. Microbiol. 11:202. doi: 10.3389/fmicb.2020.00202
Received: 26 November 2019; Accepted: 28 January 2020;
Published: 20 February 2020.
Edited by:
Eric Houdeau, INRA UMR1331 Toxicologie Alimentaire, FranceReviewed by:
Jesús Muñoz-Rojas, Meritorious Autonomous University of Puebla, MexicoMariam Sahrawy Barragan, Spanish National Research Council (CSIC), Spain
Copyright © 2020 Rubio-Gómez, Santiago, Udaondo, Garitaonaindia, Krell, Ramos and Daddaoua. This is an open-access article distributed under the terms of the Creative Commons Attribution License (CC BY). The use, distribution or reproduction in other forums is permitted, provided the original author(s) and the copyright owner(s) are credited and that the original publication in this journal is cited, in accordance with accepted academic practice. No use, distribution or reproduction is permitted which does not comply with these terms.
*Correspondence: Abdelali Daddaoua, daddaoua@ugr.es
†These authors have contributed equally to this work