- 1Department of Bacteriology, University of Wisconsin-Madison, Madison, WI, United States
- 2Forest Products Laboratory, United States Forest Service, United States Department of Agriculture, Madison, WI, United States
Social animals are among the most successful organisms on the planet and derive many benefits from living in groups, including facilitating the evolution of agriculture. However, living in groups increases the risk of disease transmission in social animals themselves and the cultivated crops upon which they obligately depend. Social insects offer an interesting model to compare to human societies, in terms of how insects manage disease within their societies and with their agricultural symbionts. As living in large groups can help the spread of beneficial microbes as well as pathogens, we examine the role of defensive microbial symbionts in protecting the host from pathogens. We further explore how beneficial microbes may influence other pathogen defenses including behavioral and immune responses, and how we can use insect systems as models to inform on issues relating to human health and agriculture.
Introduction
Some of the most successful species on the planet in terms of number of species generated over time, ability to inhabit diverse ecosystems, and maintenance of high population densities are social animals (Wilson, 1987). Social lifestyles, however, come at the cost of increased exposure to pathogens. Both modeling and experimental results indicate that population size and density correlate with pathogen prevalence and diversity (Anderson and May, 1979, 1982; Altizer et al., 2003; Schmid-Hempel, 2017). The 10-fold expansion of the human population in the last 200 years with similar population density increases has caused concerns around the risk of spreading infectious diseases (Cohen, 2003). Social insects have faced the same challenges successfully, maintaining high population densities over millions of years and are simple models to gain a better understanding of how to mitigate pathogen burden and spread (Figure 1).
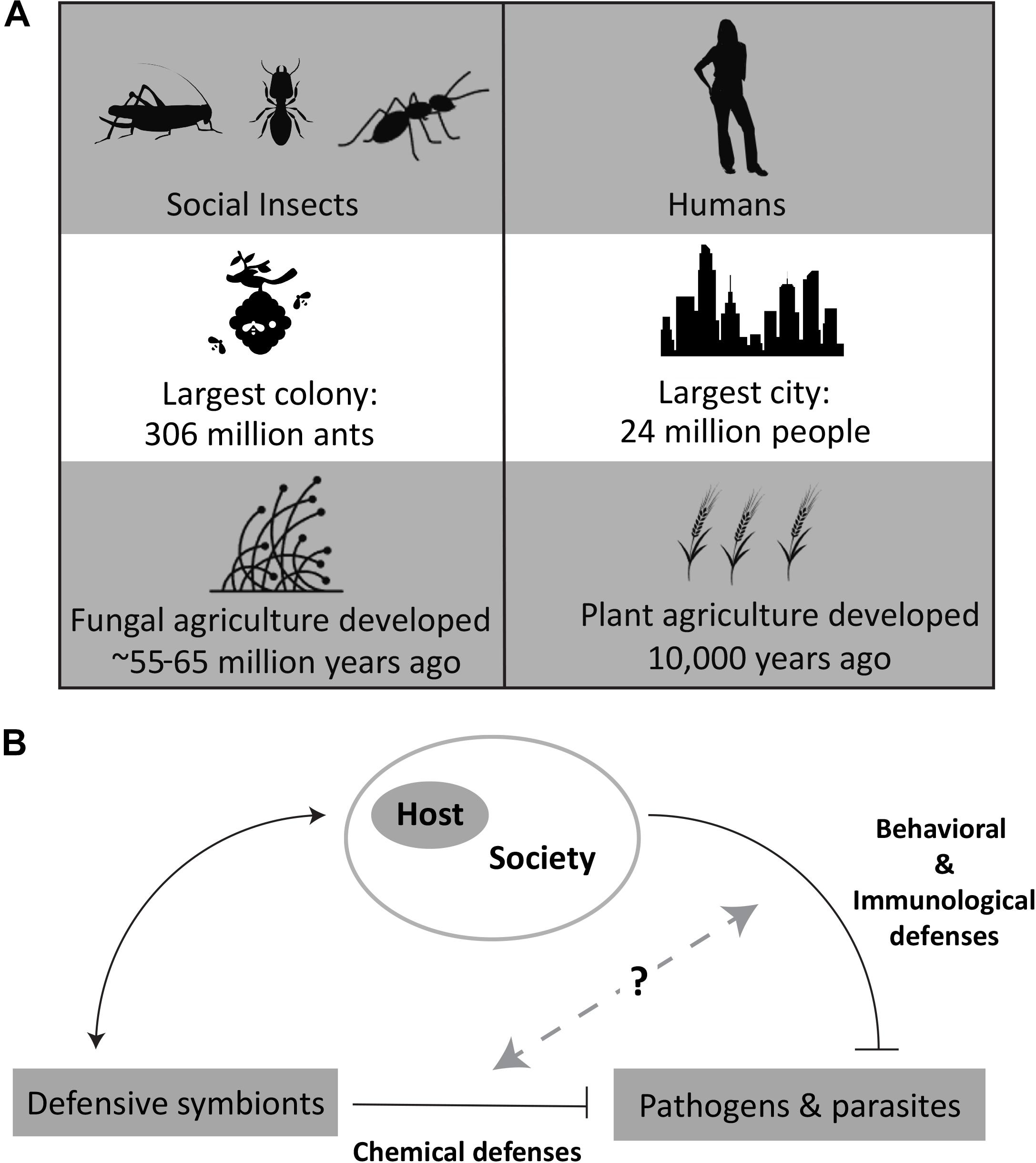
Figure 1. (A) Comparisons of human and insect societies, based on social grouping sizes (Burchill and Moreau, 2016; Sawe, 2018) and history with agriculture (Pringle, 1998; Schultz and Brady, 2008). (B) Overview of the relationship of defensive symbionts with host and pathogens. Specific image credit from the Noun Project (https://thenounproject.com/): Woman by Lluisa Iborra, Locust by OCHA Visual, Termite by Heberti Almeida, Ant by Jacob Eckert, City by sumhi_icon, Beehive by Juraj Sedlák, Barley by Nathan Stang, and Fungi by CombineDesign. All images used and modified under the Creative Commons License, Attribution 3.0.
While social living may enhance pathogen spread, social living also enables the spread of beneficial microbes (Biedermann and Rohlfs, 2017). For instance, after termites molt, they must replace their gut symbionts from other nest mates through trophallaxis and coprophagy. This “social gut” is suggested to contribute to nestmate recognition as well as development, nutrition, and defense (Breznak and Brune, 1994; Matsuura, 2001; Nakashima et al., 2002; Adams and Boopathy, 2005). Many microbes benefit the host by providing protection against predators, parasites, pathogens, or environmental stresses, also known as defensive symbiosis (White and Torres, 2009). In a mutualistic relationship, the host provides shelter and/or nutrients in exchange for defense. Understanding interactions between hosts, pathogens, and beneficial microbes can inform on the potential use of beneficial symbionts in systematically targeting certain pathogens.
In interactions between social animals, their microbial defensive symbionts and pathogens, many different selective pressures may be operating simultaneously. Pathogen pressures can impact host and symbiont (King and Bonsall, 2017; Engl et al., 2018). Beneficial symbionts may influence social behavior to facilitate their horizontal transmission, but core microbiota may be influenced by diet or other factors (Sherwin et al., 2019). The evolutionary and ecological dynamics of microbial symbiont relationships with social animals are not well understood. To deconvolute these interactions, social insects are interesting models to compare social and solitary relatives (e.g., bees, discussed below) or comparing changes in microbiota of species that alternate between gregarious and solitary lifestages may also be useful (Lavy et al., 2018).
In this review, we discuss the role of microbial defensive symbionts in pathogen mitigation within social communities and their associated agricultural systems. We also consider how defensive symbiosis intersects with immunological and behavioral defenses. We compare examples from insects with defensive symbionts in humans and highlight how insect models can advance understanding the social impacts of defensive symbionts.
Insect Defenses Against Pathogens
While defensive symbionts can benefit both social and solitary animals, social living may better enable sharing defensive symbionts than solitary lifestyles. For example, eusocial bees (e.g., Apis mellifera and Bombus spp.), have a consistent core microbiota that defends against the trypanosome gut parasite Crithidia bombi, whereas solitary bees do not have a consistent core community (Koch and Schmid-Hempel, 2011). Several core microbiome members, including Gilliamella apicola and Lactobacillus spp., correlate with decreased susceptibility to C. bombi (Cariveau et al., 2014; Mockler et al., 2018; Näpflin and Schmid-Hempel, 2018). Additionally, experiments disrupting the core bee microbiota support the hypothesis that the gut microbiota plays a role in protecting against opportunistic pathogens (Raymann et al., 2017) and another common parasite, Lotmaria passim (Schwarz et al., 2016). Biofilm formation by the core strains is the suggested protective mechanism against this pathogen, as indicated by fluorescent in situ hybridization (FISH) imaging (Martinson et al., 2012) and the enrichment of secretion systems and surface proteins in bee gut metagenomes (Engel et al., 2012). As biofilm formation and colonization resistance are broad defensive mechanisms, it is unclear whether solitary bees have microbes with similar functionality. Likewise, social bee gut microbes may confer other functions affecting fitness.
Social animals need to not only protect themselves from disease, but also their shared food sources. Three lineages of eusocial or subsocial insects demonstrate agricultural behavior: ants (Myrmicinae: Attini), termites (Macrotermitinae), and ambrosia beetles (Xyleborinae and others). All of these insects live in gregarious communities supporting the hypothesis that sociality allowed for evolution of insect agriculture (Mueller et al., 2005). Fungus farming termites cultivate basidiomycete fungi, Termitomyces spp. as a food source that are either vertically or horizontally acquired depending on termite species (Johnson and Hagen, 1981; Korb and Aanen, 2003). Some termites (Macrotermes natalensis) harbor Bacillus sp. that produce bacillaene which has antifungal activity and helps protect the fungal cultivar (Um et al., 2013). Xyleborine ambrosia beetles cultivate an assemblage of fungi, rather than a single fungal cultivar, which comprises mycelial fungi, yeasts, and bacteria (Norris, 1965; Hulcr and Stelinski, 2017). A cycloheximide-producing Streptomyces phylotype has been isolated from two species of ambrosia beetles as a possible defensive symbiont (Grubbs et al., 2019).
In the fungus-growing ants, microbial associations range from mutualistic to parasitic and are well-described. The ants grow a fungal cultivar as their primary food source in a monoculture, which makes it highly susceptible to the specialized fungal pathogen Escovopsis (Ascomycete; Hypocreales). To protect their food source, the ants evolved several defense mechanisms, including a mutualism with Pseudonocardia spp. (Currie et al., 1999b, 2003). Pseudonocardia produces antimicrobial molecules that are active against Escovopsis (Currie et al., 1999b, 2003; Poulsen et al., 2010). Growing Pseudonocardia and Escovopsis together reveals patterns of inhibition and resistance between the two organisms suggesting population and interaction dynamics at fine phylogenetic scales (Poulsen et al., 2010; Cafaro et al., 2011). Several of the antibiotics produced by Pseudonocardia have been characterized (Oh et al., 2009; Carr et al., 2012; Van Arnam et al., 2016) although the full diversity of antibiotics used is unknown.
Interactions of Defensive Symbionts With Host Defenses in Insects
Other methods of pathogen resistance, such as behavior and immunity, aid in disease resistance and can be influenced by microbes (Nyholm and Graf, 2012; Lizé et al., 2014; Flórez et al., 2015). Host and symbionts may adapt to each other in different ways: symbionts may avoid triggering immune function (Trappeniers et al., 2019); hosts may diversify immune pathways (Maire et al., 2019) or hosts may potentially reduce immune function (International Aphid Genomics Consortium, 2010; Douglas et al., 2011). Further examples of innate immunity in social insects can be found in the following review (Otani et al., 2016).
Social insects can coordinate defensive behaviors, some of which may be triggered or helped by beneficial microbes. Many of the defensive behaviors in social insects are aimed at maintaining sanitation of the nest as well as the individuals within the nest. This phenomenon of collective actions to mitigate pathogen spread/exposure is known as social immunity, which is defined as the control or elimination of potential pathogens by cooperation of individuals through behavioral, physiological, and/or organizational means (Cremer et al., 2007; Meunier, 2015). For example, subsocial aphid Nipponaphis monzeni soldiers respond to attacks on their colonies by swarming and exploding their abdomens. Their abdomens are swollen with hemocytes and tyrosine that seal and protect the colony. The endosymbiotic bacterium, Buchnera, regulated by aphid host genes, helps overproduce tyrosine (Kutsukake et al., 2019). This example highlights the complex interplay occurring between host, beneficial symbionts, immune system, and social structure of an organism. Other examples of social immunity include grooming, removing waste material and weeding nests and fungal gardens. Further experimentation using antibiotics or probiotics could explore the manner in which microbes may influence behavior and fitness (Alberoni et al., 2018).
Defensive behaviors can also be facilitated by the microbial production of chemical signals or chemical defenses. Social insects participate in extensive grooming behaviors categorized as autogrooming (i.e., self-grooming) and allogrooming (i.e., grooming among nestmates), which serve not only to remove foreign substances from the body surface, but can also provide lasting antimicrobial defenses (Zhukovskaya et al., 2013). In terms of using microbes for production of chemical defenses, many examples in the above defensive symbioses fit this description (e.g., antimicrobial phenols from locust symbionts, antibiotics from fungus-farming ant symbionts). Microbes are also capable of producing chemical signals, such as the intestinal microbes of subterranean termites (Reticulitermes speratus), which allow recognition of nestmates from non-nestmate intruders (Matsuura, 2001). The diversity of interactions between defensive microbes and host behavior remains an open area of exploration.
Human Defenses Against Pathogens
As in insects, the microbiota provides defense against various pathogens in humans, but is more complex than insect microbiomes. While different sites, such as the vagina and nasal cavity can support symbionts with abilities to produce defensive compounds (Donia et al., 2014; Zipperer et al., 2016), most of the potential defensive microbes described reside in the gut. Unlike many insect gut microbiotas, the human gut microbiota may contain hundreds of species (Qin et al., 2010). Adding further complication, whereas in bees and other hosts a core community is evident, a consistent core community has not been identified in humans, although a core functionality appears more conserved than particular strains (Turnbaugh and Gordon, 2009; Human Microbiome Project Consortium, 2012). Although humans lack an equivalent solitary lifestyle to insects, evidence suggests that humans in close social relationships may share a variety of bacteria with one another and have greater richness and diversity than humans living alone (Dill-McFarland et al., 2019).
Many different mechanisms for microbial defense exist and understanding the microbiota’s functions may lead to improved therapies. For example, fecal microbiota transplants for treating Clostridium difficile infections that are non-responsive to antibiotics have cure rates of 90% (Bakken et al., 2011; Youngster et al., 2016). Several mechanisms have been suggested including that the microbiota outcompete the pathogen for nutrients, microbially produced antibiotics target C. difficile, microbially produced secondary bile acids inhibit C. difficile, and microbial interactions with the immune system help repair the gut barrier (Khoruts and Sadowsky, 2016). Human gut microbes have also been linked to defense against Vibrio cholerae, where correlations have been found between microbiota taxa present in the gut and resistance to cholera (Hsiao et al., 2014; Midani et al., 2018). Likewise, human microbiota strains compete with Salmonella for nutrients and produce metabolites that potentially inhibit Salmonella (Antunes et al., 2014; Bratburd et al., 2018; Zhang et al., 2018). Although many interactions and correlations have been suggested between defensive symbiotic bacteria and pathogens in humans, the challenge remains to explore these symbionts on a society-wide scale to understand the benefits not only to individuals but to public health.
Although humans do not have ancient history (on an evolutionary time scale) with agriculture, many crops used by humans associate with defensive microbes against certain pathogens. One example of an agricultural defensive symbiont is Pseudomonas fluorescens, a bacterium that produces the antibiotic 2,4-diacetylphloroglucinol, which can inhibit the causative agent of take-all disease in wheat (Keel et al., 1992). This bacterium can be found naturally in soils and is a prominent example of suppressive soils, where soil harbors a community or certain strains that inhibit plant pathogens, analogous to the idea of colonization resistance in animals. Beneficial microbes may provide an environmentally sustainable alternative to chemical control of pathogens and vectors, but will require maintaining beneficial microbes in agricultural settings and consideration of microbial interactions in plant breeding beyond the host’s pathogen resistance (see the following review for more detail (Syed Ab Rahman et al., 2018).
Interactions of Defensive Symbionts With Host Defenses in Humans
The role of the immune system and behaviors is increasingly recognized as not only defending against harmful microbes, but also fostering the establishment and maintenance of bacterial symbionts. We direct the reader to other reviews for further exploration of the numerous interactions between the microbiota and the immune system (Belkaid and Harrison, 2017) and behavior (Vuong et al., 2017; Johnson and Foster, 2018).
Humans have been practicing their own social immunity with hygienic behaviors throughout history. This includes early ritualistic behaviors, quarantine and sanitation, and after the rise of the germ-theory of disease, water treatment, vaccinations, and vector control (Institute of Medicine (US) Committee for the Study of the Future of Public Health, 1988; Curtis, 2007). While humans have taken advantage of antimicrobial compounds from a variety of sources for hundreds of years (Aminov, 2010; Harrison et al., 2015), large scale antibiotic discovery, often microbially derived, took off in the 1900’s and enabled treating a wide variety of pathogens in people as well as in agriculture (Aminov, 2010). Unfortunately, broad-spectrum antibiotics can have lasting impacts on the microbiota affecting the many interactions discussed above (Jernberg et al., 2007). While efforts to eliminate pathogens have substantial impacts, most notably with vaccines eliminating smallpox and reducing other disease to 99% fewer cases (Orenstein and Ahmed, 2017), practices for sharing beneficial microbes could also be valuable for medicine and agriculture. These practices may include fecal microbiota transplants, probiotic and prebiotic supplementation (George Kerry et al., 2018; Sonnenburg and Sonnenburg, 2019), creating built environments that favor beneficial microbes (Kembel et al., 2012); however, besides perhaps fecal microbiota transplants for treating C. difficile, these practices currently lack substantial evidence of efficacy.
What Can We Learn From Insects?
Insects are useful models to address societal-wide impacts of defensive symbionts (Table 1). Given the vast complexity in the human gut, insects can be a simple model to dissect various mechanisms of microbial defenses since insects tend to have simplified microbiomes relative to humans. Comparisons between social and solitary insects (whether in different life stages as described above with locusts, or among related social and solitary members as described with bees) can shed light on what roles, if any, defensive symbionts have played in the evolution of sociality. Insect colonies are well-defined social units for replication, tend to have limited within colony genetic variation, and can be reared in controlled conditions. The insects themselves often have relatively fast life cycles, which is useful for examining fitness and intergeneration effects defensive microbes may have. Social insects also engage in behaviors of interest, like farming. In the most direct sense, natural products from insect symbioses may be useful as leads for new antibiotics themselves (Stow and Beattie, 2008; Ramadhar et al., 2014; Chevrette et al., 2019) and insects have inherent practical value as many species are important pollinators or pests; however, we also want to highlight using insect models to explore the societal impact of gaining or losing beneficial symbionts. We detailed many benefits of insect models above, but these models come with drawbacks. The simplicities of social insect models limit conclusions relevant for humans to basic ecological dynamics. Insect models lack many features that mediate host-microbe interactions in humans, including an adaptive immune system or complex nervous systems. While much microbiome research has focused on the impact to the individual host, social insects can be used to address basic ecological and evolutionary dynamics including (i) how resilient societies transmit beneficial microbes to other individuals; and (ii) the larger impact of beneficial microbes at the population level.
Social insect models can address how social animals maximize beneficial microbe transmission while minimizing pathogen spread. Disrupting transmission of beneficial microbes can render hosts more susceptible to disease (Bohnhoff et al., 1954; Currie et al., 1999a; Raymann et al., 2017). In some human societies, transmission and maintenance of microbes has changed dramatically with the introduction of antibiotics, hygiene practices, and diet changes (Bokulich et al., 2016; Vangay et al., 2018). Disruptions in microbiota transmission are hypothesized to have health impacts, including obesity (Principi and Esposito, 2016). In both social insects and humans we have limited understanding of how beneficial microbes are effectively transmitted. In the leaf-cutter ant system, we know that the defensive symbiont Pseudonocardia is generally vertically transmitted, acquired during a narrow time window (Marsh et al., 2014) and may use certain host structures (Li et al., 2018), but we do not know what limits bacterial acquisition to certain strains and microbial adaptations to the host. Analogously in humans, we know microbial acquisition begins at birth but the roles and extent of vertically versus horizontally acquired microbes is still debated (Ferretti et al., 2018; Korpela and de Vos, 2018; Moeller et al., 2018; Brito et al., 2019). One drawback of insect models is that specific mechanisms enabling transmission and colonization of beneficial microbes likely differ considerably between insects and humans (e.g., coprophagy is normal behavior for all termite colony members, while fecal microbiota transplant in humans is a medical procedure for the sick). Similarly, humans may travel further and interact with other communities introducing complicated interactions that may not be captured with insect models. However, the defined social structures of eusocial insects may be useful for understanding and manipulating microbial transmission later in life. Reproductive queens have limited contact with other adult workers, for instance, and understanding when and how they share microbes with other castes could illuminate the social elements of microbial transmission (Otani et al., 2019). Microbiomes of distinct nest structures provide an interesting comparison to the idea of built environments (Sharma and Gilbert, 2018).
Additionally, social insect models may address how environmental perturbations such as diet or temperature change the overall community response to pathogens and illuminate fitness effects in different contexts. For example, different substrates used in leafcutter ant fungal gardens impacts overall colony survivorship (Khadempour et al., 2016). While some leafcutter ants associate with defensive symbionts as described above, others rely on their own chemical defenses (Fernández-Marín et al., 2009). The leafcutting ant model could be used to explore how resilient different defensive strategies (chemical or biological control) are to perturbations such as the availability of different substrates. Fisher et al. (2019) predict how other social insect characteristics (including degree of specialization and nest architecture) may enhance susceptibility or resilience to various climate perturbations. The relative simplicity of insect models could help test and reveal basic principles to understand how microbial defenses change in different contexts.
Conclusion
How societies effectively address risk of pathogen exposure is of increasing concern, especially as the human population size and density rises. Social insects provide a window to explore disease management on a society-wide scale. Increasingly, defensive symbionts are recognized for their valuable role in mitigating pathogens, in insects as well as in humans. Social insects can act as useful models to address the role of defensive symbionts in societies and their interactions with physiological, chemical, and behavioral defenses. Examples from insects provide insight for microbiome-based therapies and agricultural products, as well as help address basic questions on how beneficial microbes are transmitted, maintained, and perturbed in social animals.
Author Contributions
JB, HH, and RA wrote the manuscript. All authors contributed to the manuscript revision and approved the submitted version.
Funding
Support for this project was provided through National Institutes of Health (NIH) U19 Al109673 and NIH U19 TW009872. Funding for JB provided through the University of Wisconsin-Madison Department of Bacteriology Michael and Winona Foster fellowship and NIH T32 AI55397. Funding for HH was provided through the Department of Energy Great Lakes Bioenergy Research Center, U.S. Department of Energy, Office of Science, Office of Biological and Environmental Research under award DE-SC0018409. Funding for RA was provided through the USDA Forest Products Laboratory.
Conflict of Interest
The authors declare that the research was conducted in the absence of any commercial or financial relationships that could be construed as a potential conflict of interest.
Acknowledgments
We thank Dr. Cameron Currie, Dr. Reed Stubbendieck, Dr. Margaret Thairu, and Dr. Marc Chevrette for their insightful comments and critical appraisal on the manuscript.
References
Adams, L., and Boopathy, R. (2005). Isolation and characterization of enteric bacteria from the hindgut of Formosan termite. Bioresour. Technol. 96, 1592–1598. doi: 10.1016/J.BIORTECH.2004.12.020
Alberoni, D., Baffoni, L., Gaggìa, F., Ryan, P. M., Murphy, K., Ross, P. R., et al. (2018). Impact of beneficial bacteria supplementation on the gut microbiota, colony development and productivity of Apis mellifera L. Benef. Microbes 9, 269–278. doi: 10.3920/BM2017.0061
Altizer, S., Nunn, C. L., Thrall, P. H., Gittleman, J. L., Antonovics, J., Cunningham, A. A., et al. (2003). Social organization and parasite risk in mammals: integrating theory and empirical studies. Annu. Rev. Ecol. Evol. Syst. 34, 517–547. doi: 10.1146/annurev.ecolsys.34.030102.151725
Aminov, R. I. (2010). A brief history of the antibiotic era: lessons learned and challenges for the future. Front. Microbiol. 1:134. doi: 10.3389/fmicb.2010.00134
Anderson, R. M., and May, R. M. (1979). Population biology of infectious diseases: part I. Nature 280, 361–367. doi: 10.1038/280361a0
Anderson, R. M., and May, R. M. (1982). Coevolution of hosts and parasites. Parasitology 85, 411–426. doi: 10.1017/S0031182000055360
Antunes, L. C. M., McDonald, J. A. K., Schroeter, K., Carlucci, C., Ferreira, R. B. R., Wang, M., et al. (2014). Antivirulence activity of the human gut metabolome. mBio 5, e1183–e1114. doi: 10.1128/mBio.01183-14
Bakken, J. S., Borody, T., Brandt, L. J., Brill, J. V., Demarco, D. C., Franzos, M. A., et al. (2011). Treating Clostridium difficile infection with fecal microbiota transplantation. Clin. Gastroenterol. Hepatol. 9, 1044–1049. doi: 10.1016/j.cgh.2011.08.014
Belkaid, Y., and Harrison, O. J. (2017). Homeostatic immunity and the microbiota. Immunity 46, 562–576. doi: 10.1016/j.immuni.2017.04.008
Biedermann, P. H., and Rohlfs, M. (2017). Evolutionary feedbacks between insect sociality and microbial management. Curr. Opin. Insect Sci. 22, 92–100. doi: 10.1016/j.cois.2017.06.003
Bohnhoff, M., Drake, B. L., and Miller, C. P. (1954). Effect of streptomycin on susceptibility of intestinal tract to experimental Salmonella infection. Proc. Soc. Exp. Biol. Med. 86, 132–137. doi: 10.3181/00379727-86-21030
Bokulich, N. A., Chung, J., Battaglia, T., Henderson, N., Jay, M., Li, H., et al. (2016). Antibiotics, birth mode, and diet shape microbiome maturation during early life. Sci. Transl. Med. 8:343ra82. doi: 10.1126/scitranslmed.aad7121
Bratburd, J. R., Keller, C., Vivas, E., Gemperline, E., Li, L., Rey, F. E., et al. (2018). Gut microbial and metabolic responses to Salmonella enterica Serovar Typhimurium and Candida albicans. mBio 9, e2032–e2018. doi: 10.1128/MBIO.02032-18
Breznak, J. A., and Brune, A. (1994). Role of microorganisms in the digestion of lignocellulose by termites. Annu. Rev. Entomol. 39, 453–487. doi: 10.1146/annurev.en.39.010194.002321
Brito, I. L., Gurry, T., Zhao, S., Huang, K., Young, S. K., Shea, T. P., et al. (2019). Transmission of human-associated microbiota along family and social networks. Nat. Microbiol. 4, 964–971. doi: 10.1038/s41564-019-0409-6
Burchill, A. T., and Moreau, C. S. (2016). Colony size evolution in ants: macroevolutionary trends. Insectes Soc. 63, 291–298. doi: 10.1007/s00040-016-0465-3
Cafaro, M. J., Poulsen, M., Little, A. E. F., Price, S. L., Gerardo, N. M., Wong, B., et al. (2011). Specificity in the symbiotic association between fungus-growing ants and protective Pseudonocardia bacteria. Proc. R. Soc. B Biol. Sci. 278, 1814–1822. doi: 10.1098/rspb.2010.2118
Cariveau, D. P., Elijah Powell, J., Koch, H., Winfree, R., and Moran, N. A. (2014). Variation in gut microbial communities and its association with pathogen infection in wild bumble bees (Bombus). ISME J. 8, 2369–2379. doi: 10.1038/ismej.2014.68
Carr, G., Derbyshire, E. R., Caldera, E., Currie, C. R., and Clardy, J. (2012). Antibiotic and antimalarial quinones from fungus-growing ant-associated Pseudonocardia sp. J. Nat. Prod. 75, 1806–1809. doi: 10.1021/np300380t
Chevrette, M. G., Carlson, C. M., Ortega, H. E., Thomas, C., Ananiev, G. E., Barns, K. J., et al. (2019). The antimicrobial potential of Streptomyces from insect microbiomes. Nat. Commun. 10:516. doi: 10.1038/s41467-019-08438-0
Cohen, J. E. (2003). Human population: the next half century. Science 302, 1172–1175. doi: 10.1126/science.1088665
Cremer, S., Armitage, S. A. O., and Schmid-Hempel, P. (2007). Social immunity. Curr. Biol. 17, R693–R702. doi: 10.1016/J.CUB.2007.06.008
Currie, C. R., Bot, A. N. M., and Boomsma, J. J. (2003). Experimental evidence of a tripartite mutualism: bacteria protect ant fungus gardens from specialized parasites. Oikos 101, 91–102. doi: 10.1034/j.1600-0706.2003.12036.x
Currie, C. R., Mueller, U. G., Malloch, D., Dowd, S. E., Hong, E., and Mueller, U. G. (1999a). The agricultural pathology of ant fungus gardens. Proc. Natl. Acad. Sci. U.S.A. 96, 7998–8002. doi: 10.1073/pnas.96.14.7998
Currie, C. R., Scott, J. A., Summerbell, R. C., and Malloch, D. (1999b). Fungus-growing ants use antibiotic-producing bacteria to control garden parasites. Nature 398, 701–704. doi: 10.1038/19519
Curtis, V. A. (2007). Dirt, disgust and disease: a natural history of hygiene. J. Epidemiol. Community Health 61, 660–664. doi: 10.1136/jech.2007.062380
Dill-McFarland, K. A., Tang, Z.-Z., Kemis, J. H., Kerby, R. L., Chen, G., Palloni, A., et al. (2019). Close social relationships correlate with human gut microbiota composition. Sci. Rep. 9:703. doi: 10.1038/s41598-018-37298-9
Donia, M. S., Cimermancic, P., Schulze, C. J., Wieland Brown, L. C., Martin, J., Mitreva, M., et al. (2014). A systematic analysis of biosynthetic gene clusters in the human microbiome reveals a common family of antibiotics. Cell 158, 1402–1414. doi: 10.1016/j.cell.2014.08.032
Douglas, A. E., Bouvaine, S., and Russell, R. R. (2011). How the insect immune system interacts with an obligate symbiotic bacterium. Proc. R. Soc. B Biol. Sci. 278, 333–338. doi: 10.1098/rspb.2010.1563
Engel, P., Martinson, V. G., and Moran, N. A. (2012). Functional diversity within the simple gut microbiota of the honey bee. Proc. Natl. Acad. Sci. U.S.A. 109, 11002–11007. doi: 10.1073/pnas.1202970109
Engl, T., Kroiss, J., Kai, M., Nechitaylo, T. Y., Svatoš, A., and Kaltenpoth, M. (2018). Evolutionary stability of antibiotic protection in a defensive symbiosis. Proc. Natl. Acad. Sci. U.S.A. 115, E2020–E2029. doi: 10.1073/pnas.1719797115
Fernández-Marín, H., Zimmerman, J. K., Nash, D. R., Boomsma, J. J., and Wcislo, W. T. (2009). Reduced biological control and enhanced chemical pest management in the evolution of fungus farming in ants. Proc. Biol. Sci. 276, 2263–2269. doi: 10.1098/rspb.2009.0184
Ferretti, P., Pasolli, E., Tett, A., Asnicar, F., Gorfer, V., Fedi, S., et al. (2018). Mother-to-infant microbial transmission from different body sites shapes the developing infant gut microbiome. Cell Host. Microbe 24, 133.e5–145.e.5 doi: 10.1016/J.CHOM.2018.06.005
Fisher, K., West, M., Lomeli, A. M., Woodard, S. H., and Purcell, J. (2019). Are societies resilient? Challenges faced by social insects in a changing world. Insectes Soc. 66, 5–13. doi: 10.1007/s00040-018-0663-2
Flórez, L. V., Biedermann, P. H. W., Engl, T., and Kaltenpoth, M. (2015). Defensive symbioses of animals with prokaryotic and eukaryotic microorganisms. Nat. Prod. Rep. 32, 904–936. doi: 10.1039/c5np00010f
George Kerry, R., Patra, J. K., Gouda, S., Park, Y., Shin, H. S., and Das, G. (2018). Benefaction of probiotics for human health: a review. J. Food Drug Anal. 26, 927–939. doi: 10.1016/j.jfda.2018.01.002
Grubbs, K. J., Surup, F., Biedermann, P. H. W., McDonald, B. R., Klassen, J., Carlson, C. M., et al. (2019). Cycloheximide-producing Streptomyces associated with Xyleborinus saxesenii and Xyleborus affinis fungus-farming ambrosia beetles. bioRxiv [preprint]. doi: 10.1101/511493
Harrison, F., Roberts, A. E. L., Gabrilska, R., Rumbaugh, K. P., Lee, C., and Diggle, S. P. (2015). A 1,000-Year-Old antimicrobial remedy with antistaphylococcal activity. MBio 6:e01129. doi: 10.1128/mBio.01129-15
Hsiao, A., Ahmed, A. M. S., Subramanian, S., Griffin, N. W., Drewry, L. L., Petri, W. A., et al. (2014). Members of the human gut microbiota involved in recovery from Vibrio cholerae infection. Nature 515, 423–426. doi: 10.1038/nature13738
Hulcr, J., and Stelinski, L. L. (2017). The ambrosia symbiosis: from evolutionary ecology to practical management. Annu. Rev. Entomol. 62, 285–303. doi: 10.1146/annurev-ento-031616-035105
Human Microbiome Project Consortium (2012). Structure, function and diversity of the healthy human microbiome. Nature 486, 207–214. doi: 10.1038/nature11234
Institute of Medicine (US) Committee for the Study of the Future of Public Health (1988). The Future of Public Health. Washington, D.C.: National Academies Press. doi: 10.17226/1091
International Aphid Genomics Consortium (2010). Genome sequence of the pea aphid Acyrthosiphon pisum. PLoS Biol. 8:e1000313. doi: 10.1371/journal.pbio.1000313
Jernberg, C., Löfmark, S., Edlund, C., and Jansson, J. K. (2007). Long-term ecological impacts of antibiotic administration on the human intestinal microbiota. ISME J. 1, 56–66. doi: 10.1038/ismej.2007.3
Johnson, J. B., and Hagen, K. S. (1981). A neuropterous larva uses an allomone to attack termites. Nature 289, 506–507. doi: 10.1038/289506a0
Johnson, K. V.-A., and Foster, K. R. (2018). Why does the microbiome affect behaviour? Nat. Rev. Microbiol. 16, 647–655. doi: 10.1038/s41579-018-0014-3
Keel, C., Schnider, U., Maurhofer, M., Voisard, C., Laville, J., Burger, U., et al. (1992). Suppression of root diseases by Pseudomonas fluorescens CHA0: importance of the bacterial secondary metabolite 2,4-Diacetylphloroglucinol. Mol. Plant-Microbe Interact. 5:4. doi: 10.1094/MPMI-5-004
Kembel, S. W., Jones, E., Kline, J., Northcutt, D., Stenson, J., Womack, A. M., et al. (2012). Architectural design influences the diversity and structure of the built environment microbiome. ISME J. 6, 1469–1479. doi: 10.1038/ismej.2011.211
Khadempour, L., Burnum-Johnson, K. E., Baker, E. S., Nicora, C. D., Webb-Robertson, B.-J. M., White, R. A., et al. (2016). The fungal cultivar of leaf-cutter ants produces specific enzymes in response to different plant substrates. Mol. Ecol. 25, 5795–5805. doi: 10.1111/mec.13872
Khoruts, A., and Sadowsky, M. J. (2016). Understanding the mechanisms of faecal microbiota transplantation. Nat. Rev. Gastroenterol. Hepatol. 13, 508–516. doi: 10.1038/nrgastro.2016.98
King, K. C., and Bonsall, M. B. (2017). The evolutionary and coevolutionary consequences of defensive microbes for host-parasite interactions. BMC Evol. Biol. 17:190. doi: 10.1186/s12862-017-1030-z
Koch, H., and Schmid-Hempel, P. (2011). Socially transmitted gut microbiota protect bumble bees against an intestinal parasite. Proc. Natl. Acad. Sci. U.S.A. 108, 19288–19292. doi: 10.1073/pnas.1110474108
Korb, J., and Aanen, D. K. (2003). The evolution of uniparental transmission of fungal symbionts in fungus-growing termites (Macrotermitinae). Behav. Ecol. Sociobiol. 53, 65–71. doi: 10.1007/s00265-002-0559-y
Korpela, K., and de Vos, W. M. (2018). Early life colonization of the human gut: microbes matter everywhere. Curr. Opin. Microbiol. 44, 70–78. doi: 10.1016/J.MIB.2018.06.003
Kutsukake, M., Moriyama, M., Shigenobu, S., Meng, X.-Y., Nikoh, N., Noda, C., et al. (2019). Exaggeration and cooption of innate immunity for social defense. Proc. Natl. Acad. Sci. U.S.A. 116, 8950–8959. doi: 10.1073/PNAS.1900917116
Lavy, O., Gophna, U., Gefen, E., and Ayali, A. (2018). The effect of density-dependent phase on the locust gut bacterial composition. Front. Microbiol. 9:3020. doi: 10.3389/fmicb.2018.03020
Li, H., Sosa-Calvo, J., Horn, H. A., Pupo, M. T., Clardy, J., Rabeling, C., et al. (2018). Convergent evolution of complex structures for ant-bacterial defensive symbiosis in fungus-farming ants. Proc. Natl. Acad. Sci. U.S.A. 115, 10720–10725. doi: 10.1073/pnas.1809332115
Lizé, A., McKay, R., and Lewis, Z. (2014). Kin recognition in Drosophila: the importance of ecology and gut microbiota. ISME J. 8, 469–477. doi: 10.1038/ismej.2013.157
Maire, J., Vincent-Monégat, C., Balmand, S., Vallier, A., Hervé, M., Masson, F., et al. (2019). Weevil pgrp-lb prevents endosymbiont TCT dissemination and chronic host systemic immune activation. Proc. Natl. Acad. Sci. U.S.A. 116, 5623–5632. doi: 10.1073/pnas.1821806116
Marsh, S. E., Poulsen, M., Pinto-Tomás, A., and Currie, C. R. (2014). Interaction between workers during a short time window is required for bacterial symbiont transmission in Acromyrmex leaf-cutting ants. PLoS One 9:e103269. doi: 10.1371/journal.pone.0103269
Martinson, V. G., Moy, J., and Moran, N. A. (2012). Establishment of characteristic gut bacteria during development of the honeybee worker. Appl. Environ. Microbiol. 78, 2830–2840. doi: 10.1128/AEM.07810-11
Matsuura, K. (2001). Nestmate recognition mediated by intestinal bacteria in a termite, Reticulitermes speratus. Oikos 92, 20–26. doi: 10.1034/j.1600-0706.2001.920103.x
Meunier, J. (2015). Social immunity and the evolution of group living in insects. Philos. Trans. R. Soc. B Biol. Sci. 370, 20140102–20140102. doi: 10.1098/rstb.2014.0102
Midani, F. S., Weil, A. A., Chowdhury, F., Begum, Y. A., Khan, A. I., Debela, M. D., et al. (2018). Human gut microbiota predicts susceptibility to Vibrio cholerae infection. J. Infect. Dis. 218, 645–653. doi: 10.1093/infdis/jiy192
Mockler, B. K., Kwong, W. K., Moran, N. A., and Koch, H. (2018). Microbiome Structure Influences Infection by the Parasite Crithidia bombi in Bumble Bees. Appl. Environ. Microbiol. 84, e2335–e2317. doi: 10.1128/AEM.02335-17
Moeller, A. H., Suzuki, T. A., Phifer-Rixey, M., and Nachman, M. W. (2018). Transmission modes of the mammalian gut microbiota. Science 362, 453–457. doi: 10.1126/science.aat7164
Mueller, U. G., Gerardo, N. M., Aanen, D. K., Six, D. L., and Schultz, T. R. (2005). The evolution of agriculture in insects. Annu. Rev. Ecol. Evol. Syst. 36, 563–595. doi: 10.1146/annurev.ecolsys.36.102003.152626
Nakashima, K., Watanabe, H., and Azuma, J.-I. (2002). Cellulase genes from the parabasalian symbiont Pseudotrichonympha grassii in the hindgut of the wood-feeding termite Coptotermes formosanus. Cell. Mol. Life Sci. 59, 1554–1560. doi: 10.1007/s00018-002-8528-1
Näpflin, K., and Schmid-Hempel, P. (2018). High gut microbiota diversity provides lower resistance against infection by an intestinal parasite in bumblebees. Am. Nat. 192, 131–141. doi: 10.1086/698013
Norris, D. (1965). The complex of fungi essential to growth and development of Xyleborus sharpi in wood. Mater. Org. Beih 1, 523–529.
Nyholm, S. V., and Graf, J. (2012). Knowing your friends: invertebrate innate immunity fosters beneficial bacterial symbioses. Nat. Rev. Microbiol. 10, 815–827. doi: 10.1038/nrmicro2894
Oh, D.-C., Poulsen, M., Currie, C. R., and Clardy, J. (2009). Dentigerumycin: a bacterial mediator of an ant-fungus symbiosis. Nat. Chem. Biol. 5, 391–393. doi: 10.1038/nchembio.159
Orenstein, W. A., and Ahmed, R. (2017). Simply put: vaccination saves lives. Proc. Natl. Acad. Sci. U.S.A. 114, 4031–4033. doi: 10.1073/pnas.1704507114
Otani, S., Bos, N., and Yek, S. H. (2016). Transitional complexity of social insect immunity. Front. Ecol. Evol. 4:69. doi: 10.3389/fevo.2016.00069
Otani, S., Zhukova, M., Koné, N. A., da Costa, R. R., Mikaelyan, A., Sapountzis, P., et al. (2019). Gut microbial compositions mirror caste-specific diets in a major lineage of social insects. Environ. Microbiol. Rep. 11, 196–205. doi: 10.1111/1758-2229.12728
Poulsen, M., Cafaro, M. J., Erhardt, D. P., Little, A. E. F., Gerardo, N. M., Tebbets, B., et al. (2010). Variation in Pseudonocardia antibiotic defence helps govern parasite-induced morbidity in Acromyrmex leaf-cutting ants. Environ. Microbiol. Rep. 2, 534–540. doi: 10.1111/j.1758-2229.2009.00098.x
Principi, N., and Esposito, S. (2016). Antibiotic administration and the development of obesity in children. Int. J. Antimicrob. Agents 47, 171–177. doi: 10.1016/J.IJANTIMICAG.2015.12.017
Pringle, H. (1998). The slow birth of agriculture. Science 282, 1446–1446. doi: 10.1126/SCIENCE.282.5393.1446
Qin, J., Li, R., Raes, J., Arumugam, M., Burgdorf, K. S., Manichanh, C., et al. (2010). A human gut microbial gene catalogue established by metagenomic sequencing. Nature 464, 59–65. doi: 10.1038/nature08821
Ramadhar, T. R., Beemelmanns, C., Currie, C. R., and Clardy, J. (2014). Bacterial symbionts in agricultural systems provide a strategic source for antibiotic discovery. J. Antibiot. 67, 53–58. doi: 10.1038/ja.2013.77
Raymann, K., Shaffer, Z., and Moran, N. A. (2017). Antibiotic exposure perturbs the gut microbiota and elevates mortality in honeybees. PLoS Biol. 15:e2001861. doi: 10.1371/journal.pbio.2001861
Sawe, B. E. (2018). The 10 Largest Cities in the World. Available at: https://www.worldatlas.com/articles/the-10-largest-cities-in-the-world.html (accessed August 26, 2019).
Schmid-Hempel, P. (2017). Parasites and their social hosts. Trends Parasitol. 33, 453–462. doi: 10.1016/J.PT.2017.01.003
Schultz, T. R., and Brady, S. G. (2008). Major evolutionary transitions in ant agriculture. Proc. Natl. Acad. Sci. U.S.A. 105, 5435–5440. doi: 10.1073/pnas.0711024105
Schwarz, R. S., Moran, N. A., and Evans, J. D. (2016). Early gut colonizers shape parasite susceptibility and microbiota composition in honey bee workers. Proc. Natl. Acad. Sci. U.S.A. 113, 9345–9350. doi: 10.1073/pnas.1606631113
Sharma, A., and Gilbert, J. A. (2018). Microbial exposure and human health. Curr. Opin. Microbiol. 44, 79–87. doi: 10.1016/J.MIB.2018.08.003
Sherwin, E., Bordenstein, S. R., Quinn, J. L., Dinan, T. G., and Cryan, J. F. (2019). Microbiota and the social brain. Science 366:eaar2016. doi: 10.1126/science.aar2016
Sonnenburg, J. L., and Sonnenburg, E. D. (2019). Vulnerability of the industrialized microbiota. Science 366:eaaw9255. doi: 10.1126/science.aaw9255
Stow, A., and Beattie, A. (2008). Chemical and genetic defenses against disease in insect societies. Brain. Behav. Immun. 22, 1009–1013. doi: 10.1016/j.bbi.2008.03.008
Syed Ab Rahman, S. F., Singh, E., Pieterse, C. M. J., and Schenk, P. M. (2018). Emerging microbial biocontrol strategies for plant pathogens. Plant Sci. 267, 102–111. doi: 10.1016/J.PLANTSCI.2017.11.012
Trappeniers, K., Matetovici, I., Van Den Abbeele, J., and De Vooght, L. (2019). The tsetse fly displays an attenuated immune response to its secondary symbiont, Sodalis glossinidius. Front. Microbiol. 10:1650. doi: 10.3389/fmicb.2019.01650
Turnbaugh, P. J., and Gordon, J. I. (2009). The core gut microbiome, energy balance and obesity. J. f Physiol. 587(Pt 17), 4153–4158. doi: 10.1113/jphysiol.2009.174136
Um, S., Fraimout, A., Sapountzis, P., Oh, D.-C., and Poulsen, M. (2013). The fungus-growing termite Macrotermes natalensis harbors bacillaene-producing Bacillus sp. that inhibit potentially antagonistic fungi. Sci. Rep. 3:3250. doi: 10.1038/srep03250
Van Arnam, E. B., Ruzzini, A. C., Sit, C. S., Horn, H., Pinto-Tomás, A. A., Currie, C. R., et al. (2016). Selvamicin, an atypical antifungal polyene from two alternative genomic contexts. Proc. Natl. Acad. Sci. U.S.A. 113, 12940–12945. doi: 10.1073/pnas.1613285113
Vangay, P., Johnson, A. J., Ward, T. L., Al-Ghalith, G. A., Shields-Cutler, R. R., Hillmann, B. M., et al. (2018). US Immigration westernizes the human gut microbiome. Cell 175, 962.e10–972.e10. doi: 10.1016/j.cell.2018.10.029
Vuong, H. E., Yano, J. M., Fung, T. C., and Hsiao, E. Y. (2017). The microbiome and host behavior. Annu. Rev. Neurosci. 40, 21–49. doi: 10.1146/annurev-neuro-072116-031347
White, J. F., and Torres, M. S. (2009). Defensive Mutualism in Microbial Symbiosis. Boca Raton, FL: CRC.
Wilson, E. O. (1987). Causes of ecological success: the case of the ants. J. Anim. Ecol. 56:1. doi: 10.2307/4795
Youngster, I., Mahabamunuge, J., Systrom, H. K., Sauk, J., Khalili, H., Levin, J., et al. (2016). Oral, frozen fecal microbiota transplant (FMT) capsules for recurrent Clostridium difficile infection. BMC Med. 14:134. doi: 10.1186/s12916-016-0680-9
Zhang, Y., Brady, A., Jones, C., Song, Y., Darton, T. C., Jones, C., et al. (2018). Compositional and functional differences in the human gut microbiome correlate with clinical outcome following infection with wild-type Salmonella enterica serovar Typhi. MBio 9, e686–e618. doi: 10.1128/mBio.00686-18
Zhukovskaya, M., Yanagawa, A., and Forschler, B. (2013). Grooming behavior as a mechanism of insect disease defense. Insects 4, 609–630. doi: 10.3390/insects4040609
Keywords: defensive symbiosis, social insects and humans, gut microbiome, colonization resistance, model systems, social immunity, insect agriculture
Citation: Bratburd JR, Arango RA and Horn HA (2020) Defensive Symbioses in Social Insects Can Inform Human Health and Agriculture. Front. Microbiol. 11:76. doi: 10.3389/fmicb.2020.00076
Received: 02 November 2019; Accepted: 14 January 2020;
Published: 07 February 2020.
Edited by:
Marko Rohlfs, University of Bremen, GermanyReviewed by:
Florent Masson, Swiss Federal Institute of Technology Lausanne, SwitzerlandRosario Gil, University of Valencia, Spain
Copyright © 2020 Bratburd, Arango and Horn. This is an open-access article distributed under the terms of the Creative Commons Attribution License (CC BY). The use, distribution or reproduction in other forums is permitted, provided the original author(s) and the copyright owner(s) are credited and that the original publication in this journal is cited, in accordance with accepted academic practice. No use, distribution or reproduction is permitted which does not comply with these terms.
*Correspondence: Jennifer R. Bratburd, YnJhdGJ1cmRAd2lzYy5lZHU=; YnJhdGJ1cmRqQGdtYWlsLmNvbQ==