- 1State Key Laboratory of Microbial Technology, Marine Biotechnology Research Center, Shandong University, Qingdao, China
- 2Department of Hematology, Qilu Hospital, Shandong University, Jinan, China
- 3College of Marine Life Sciences, Institute for Advanced Ocean Study, Ocean University of China, Qingdao, China
- 4Laboratory for Marine Biology and Biotechnology, Pilot National Laboratory for Marine Science and Technology (Qingdao), Qingdao, China
Monoacylglycerol lipases (MGLs) are present in all domains of life. However, reports on bacterial MGLs are still limited. Until now, reported bacterial MGLs are all thermophilic/mesophilic enzymes from warm terrestrial environments or deep-sea hydrothermal vent, and none of them originates from marine environments vastly subject to low temperature, high salts, and oligotrophy. Here, we characterized a novel MGL, GnMgl, from the marine cold-adapted and halophilic bacterium Glaciecola nitratireducens FR1064T. GnMgl shares quite low sequence similarities with characterized MGLs (lower than 31%). GnMgl and most of its bacterial homologs harbor a catalytic Ser residue located in the conserved C(A/S)HSMG motif rather than in the typical GxSxG motif reported on other MGLs, suggesting that GnMgl-like enzymes might be different from reported MGLs in catalysis. Phylogenetic analysis suggested that GnMgl and its bacterial homologs are clustered as a separate group in the monoglyceridelipase_lysophospholipase family of the Hydrolase_4 superfamily. Recombinant GnMgl has no lysophospholipase activity but could hydrolyze saturated (C12:0-C16:0) and unsaturated (C18:1 and C18:2) MGs and short-chain triacylglycerols, displaying distinct substrate selectivity from those of reported bacterial MGLs. The substrate preference of GnMgl, predicted to be a membrane protein, correlates to the most abundant fatty acids within the strain FR1064T, suggesting the role of GnMgl in the lipid catabolism in this marine bacterium. In addition, different from known bacterial MGLs that are all thermostable enzymes, GnMgl is a cold-adapted enzyme, with the maximum activity at 30°C and retaining 30% activity at 0°C. GnMgl is also a halotolerant enzyme with full activity in 3.5M NaCl. The cold-adapted and salt-tolerant characteristics of GnMgl may help its source strain FR1064T adapt to the cold and saline marine environment. Moreover, homologs to GnMgl are found to be abundant in various marine bacteria, implying their important physiological role in these marine bacteria. Our results on GnMgl shed light on marine MGLs.
Introduction
Lipases (EC 3.1.1.3) are a group of α/β hydrolases that catalyze the hydrolysis of ester bonds in water-insoluble long-chain acylglycerols. Monoacylglycerol lipases (MGLs) (EC 3.1.1.23) are a subclass of lipases, which specifically catalyze the hydrolysis of monoacylglycerol with low stereo-selectivity. MGLs are widely distributed in bacteria, yeast, plant, and mammals. In the ESTHER (ESTerases and alpha/beta-Hydrolase Enzymes and Relatives) database (Lenfant et al., 2013), MGLs are classified into (super)families Pancreatic_lipase, α,β-hydrolase domain-containing protein 6 (ABHD6), ABHD16, Duf_676, and Hydrolase_4 based on amino acid sequence similarity.
Most reported MGLs are from mammals and their physiological roles in mammals are well-studied. Mammalian MGLs play an essential role in energy homeostasis by catalyzing the last step of intracellular phospholipid and triacylglycerol degradation (Fredrikson et al., 1986; Taschler et al., 2011). MGLs also participate in mediating endocannabinoid-based signaling in mammalian brains to regulate processes such as pain, inflammation, memory, and cancer (Hohmann et al., 2005; Nomura et al., 2010; Micale et al., 2013), thus making them important pharmacological targets (Gil-Ordóñez et al., 2018).
In contrast to the extensive study of MGLs in mammals, only several bacterial MGLs have been biologically characterized in detail, including a MGL from soil bacterium Pseudomonas sp. LP7315 (Sakiyama et al., 2001) (named as pMGL in this study), bMGL from moderately thermophilic soil bacterium Bacillus sp. H257 (Imamura and Kitaura, 2000; Kitaura et al., 2001), LipS from a soil metagenomic library (Chow et al., 2012; Riegler-Berket et al., 2018), GMGL from Geobacillus sp. 12AMOR1 isolated from a deep-sea hydrothermal vent (Tang et al., 2019), MSMEG_0220 from human non-pathogenic Mycobacterium smegmatis (Dhouib et al., 2010) and Rv0183 from human pathogenic Mycobacterium tuberculosis H37Rv (Cotes et al., 2007), which all belong to the Hydrolase_4 superfamily except for pMGL which sequence is still unknown. These bacterial MGLs showed different substrate specificities toward MGs, di- and tri-acylglycerols, and p-nitrophenyl (pNP)-acylesters. bMGL and LipS had the highest hydrolytic activity toward monolauroylglycerol (C12:0), pMGL and MSMEG_0220 preferred monomyristoylglycerol (C14:0), Rv0183 preferred both monodecanoylglycerol (C10:0) and monolauroylglycerol (C12:0), and GMGL preferred monostearoylglycerol (C18:0). Among the reported bacterial MGLs, only Rv0183 could hydrolyze both di- and tri-acylglycerols (Cotes et al., 2007). For the synthetic pNP-acylester substrates, bMGL and GMGL showed the highest activity toward pNPC4 (Kitaura et al., 2001; Tang et al., 2019), whereas LipS preferred pNPC8 (Riegler-Berket et al., 2018). All reported bacterial MGLs are thermostable enzymes. Among them, while Rv0183 and MSMEG_0220 are mesophilic enzymes functioning at human body temperature (37°C), the others are all thermophilic enzymes with an optimum temperature for activity at 60–75°C, consistent with their origin from warm terrestrial/marine environments.
Monoacylglycerol lipases from non-pathogenic bacteria play an important role in lipid catabolism and also function in detoxification processes by degrading short-chain MGs (especially C10:0 and C12:0) that are highly toxic to these organisms (Conley and Kabara, 1973; Kabara and Vrable, 1977; Rengachari et al., 2013). In addition, MSMEG_0220, secreted by non-pathogenic M. smegmatis, was also found to participate in bacterial cell interaction (Dhouib et al., 2010). The extracellular Rv0183 from pathogenic M. tuberculosis H37Rv, an ortholog of MSMEG_0220, was thought to be involved in the catabolism of host membrane lipids to enhance pathogenicity (Cotes et al., 2007). Until now, nearly all reported bacterial MGLs but GMGL are from terrestrial environments. Reports on marine MGLs are still limited.
Marine environments cover more than two-thirds of the earth surface, which are mostly under low temperature, high salts, and oligotrophy. Marine microorganisms have been reported to produce a variety of enzymes with habitat-specific characteristics to adapt to the unique marine environments. However, reported bacterial MGLs are all from warm terrestrial environments or deep-sea hydrothermal vent, which are either thermophilic or mesophilic enzymes, and none of them originates from the vast cold marine environments. Whether these marine MGLs display habitat-specific characteristics is also unknown.
Glaciecola nitratireducens FR1064T, a cold-adapted and halophilic bacterium, was isolated from a surface seawater sample collected off Jeju Island, South Korea (Baik et al., 2006; Qin et al., 2014). The genome sequence of this strain was reported by our research group in Bian et al. (2011). In this study, we identified a gene encoding a novel MGL (GnMgl, accession number WP_014107521) from the genome sequence of strain FR1064T, which was previously annotated as an α/β fold hydrolase. GnMgl shares quite low sequence similarities with characterized MGLs (lower than 31%). GnMgl was over-expressed in Escherichia coli and purified. Recombinant GnMgl could hydrolyze saturated or unsaturated long-chain MGs. Similar to its source bacterium, GnMgl is also cold-adapted and halotolerant, which may help the adaption of the strain FR1064T to the cold and saline marine environment. Additionally, homologs to GnMgl are found to be abundant in various marine bacterial species. Our study on GnMgl will offer a better understanding on marine bacterial MGLs.
Materials and Methods
Gene Cloning and Mutagenesis
Gene GnMgl (accession number WP_014107521) was amplified from the genomic DNA of G. nitratireducens FR1064T using gene-specific primers (Table 1). The amplified fragment was seamlessly ligated with the vector pET22b to construct the recombinant plasmid pET22b-GnMgl. Using plasmid pET22b-GnMgl as the template, mutants S156A, D290A, and H318A were generated by a modified QuikChange site-directed mutagenesis method (Xia et al., 2015) with partially overlapping primers containing mutations (Table 1). All the recombinant plasmids were verified by sequencing.
Protein Expression and Purification
Wild-type GnMgl protein and its mutants were expressed in E. coli BL21 (DE3) cells and induced by the addition of 1 mM isopropyl-β-D-thiogalactopyranoside (IPTG) at 18°C for 16 h. Cells were disrupted by a JN-02C French press (JNBIO, China) in 50 mM Tris-HCl buffer (pH 8.0) containing 100 mM NaCl and 5 mM imidazole and were centrifuged at 12,000 rpm for 1 h at 4°C. The supernatant was first purified by Ni affinity chromatography (Qiagen, United States) and then by gel filtration chromatography on a Superdex 200 column (GE Healthcare, Sweden) with 10 mM Tris-HCl buffer (pH 8.0) containing 100 mM NaCl. The target protein was collected and the protein concentration was measured by the Pierce BCA Protein Assay Kit (Thermo Scientific, United States) using BSA as a standard.
Esterase Activity Assay
Esterase activity was measured as described by Li et al. (2014). The standard assay system contained 0.02 ml of 10 mM p-nitrophenyl-acylesters (pNP-acylesters) dissolved in high-performance liquid chromatography (HPLC)-grade 2-propanol, 0.02 ml enzyme and 50 mM Tris-HCl buffer (pH 8.0) in a final volume of 1 ml. After incubation at 30°C for 5 min, the reaction was terminated by the addition of 0.1 ml 20% (w/v) SDS. The absorbance of the reaction mixture at 405 nm was measured using a SpectraMax Plus384 microplate spectrophotometer (Molecular Devices, United States). All experiments were corrected for substrate autohydrolysis. One unit of enzyme activity (U) was determined as the amount of enzyme required to release 1 μmol of p-nitrophenol per minute by hydrolyzing pNP-acylesters. Substrate specificity assays were performed with pNP-acylesters different in acyl chain length (C2-C16) (Sigma, United States).
Lysophospholipase Activity Assay
Lysophospholipase activity was measured as described by Kovacic et al. (2013) with some modifications. Lysophospholipid substrates 1-palmitoyl-glycerophosphocholine (C16-GPC) and 1-stearoyl-glycerophosphocholine (C18-GPC) (Sigma, United States) were prepared as 10 mM stocks in HPLC-grade 2-propanol, respectively. The reaction mixture of 1 ml containing 0.2 mM substrate, 1.5 mM NaN3, 0.25% (v/v) Triton X-100, 20 mM Tris-HCl (pH 8.0) and 14 μg of enzyme was incubated at 30°C for 1 h. The reaction was terminated by the addition of 0.1 ml chloroform. The same reaction system without enzyme was used as the blank control. Released fatty acids were quantified spectrophotometrically using the NEFA-HR(2) Kit (Wako Life Sciences, United States) according to the manufacturer’s instructions. One unit of enzyme activity (U) was determined as the amount of enzyme required to release 1 μmol of free fatty acid per minute by hydrolyzing lysophospholipid substrates.
MGL Activity Assay
Monoacylglycerol lipase activity was determined as described by Aschauer et al. (2016) with some modifications. 1(3)-rac MGs were used because MGLs are not regioselective. MG substrates with different chain lengths and saturations were purchased from Sigma (United States) and prepared as 20 mM stocks in HPLC-grade 2-propanol, including 1-monolauroyl-rac-glycerol (C12:0), 1-monomyristoyl-rac-glycerol (C14:0), 3-monopalmitoyl-sn-glycerol (C16:0), 1-monostearoyl-rac-glycerol (C18:0), 1-monooleoyl-rac-glycerol (C18:1) and 1-monolinoleoyl-rac-glycerol (C18:2). The reaction mixture contained 0.1 ml of 20 mM MG substrate, 0.1 ml of 50 mM 3-[(3-cholamidopropyl)dimethylammonio]-1-propanesulfonate (CHAPS), 0.02 ml enzyme and 100 mM Tris-HCl buffer (pH 8.0) in a final volume of 1 ml. The same reaction system with 0.02 ml of buffer instead of enzyme was used as the blank control. After incubation at 30°C for 1 h, the reaction was stopped by the addition of 0.1 ml chloroform. Released fatty acids were quantified spectrophotometrically using the NEFA-HR(2) Kit (Wako Life Sciences, United States). One unit of enzyme activity (U) was determined as the amount of enzyme required to release 1 μmol of free fatty acid per minute by hydrolyzing MG substrates.
Triacylglycerol Hydrolase Activity Assay
Triacylglycerol hydrolase activity was determined by the titration method using triacetate, tributyrin, tricaprylin, and trilaurin as substrates. The triacylglycerol emulsions were prepared in 2.5 mM Tris-HCl (pH 7.0) containing 100 mM NaCl and 1% (w/v) gum arabic by mechanical stirring. Before starting the reaction, the emulsion pH was adjusted to 7.00 by adding 10 mM NaOH. The reaction mixture contained 2 ml triacylglycerol emulsion and 0.05 ml enzyme. The same reaction system with 0.05 ml of buffer instead of enzyme was used as the blank control. After incubation at 30°C for 30 min with mechanical stirring at 180 rpm, reaction was terminated by the addition of 0.5 ml ethanol. The release of fatty acids was measured by a pH titrator (Mettler Toledo, Switzerland). One unit of enzyme (U) was defined as the amount of enzyme required to release 1 μmol of fatty acid per minute by hydrolyzing triacylglycerol substrates.
Biochemical Characterization
By using p-nitrophenyl hexanoate (pNPC6) as substrate, the biochemical characteristics of GnMgl were studied. The optimal temperature was determined in a range from 0 to 60°C. For thermostability assay, the enzyme was incubated at 30, 35, and 40°C for different periods, and the residual activity was measured at 30°C. The optimal pH was determined with Britton-Robinson buffer in a range from pH 6.0 to 12.0 at 30°C. For pH stability assay, the enzyme was incubated in a range of pH 3.0 to 12.0 at 0°C for 1 h, and the residual activity was measured at pH 8.0 and 30°C. The effect of NaCl on enzyme activity was determined at different NaCl concentrations in a range from 0 to 4.825M at 30°C. For salt-tolerance assay, the enzyme was incubated in buffers with different NaCl concentrations ranging from 0 to 4.5M at 4°C for 24 h, and the residual activity was measured at pH 8.0 and 30°C.
The effects of metal ions (K+, Li+, Na+, Ba2+, Ca2+, Co2+, Cu2+, Mg2+, Mn2+, Ni2+, and Zn2+) and potential inhibitors (β-mercaptoethanol, DTT, Thiourea, Urea, EDTA, PMSF, JZL184, and N-arachidonoylmaleimide) on enzyme activity were tested at a final concentration of 1 or 10 mM. The effects of Tween 20, Tween 80, and Triton X-100 on enzyme activity were measured at a final concentration of 0.001, 0.01, or 0.1% (v/v). The effect of SDS on enzyme activity was examined in a final concentration of 0.001, 0.01, or 0.1% (w/v). The effects of organic solvents including methanol, ethanol, 2-propanol, dimethylsulfoxide (DMSO), dimethylformamide (DMF), acetonitrile, and acetone were tested in a final concentration of 10 or 20% (v/v).
Circular dichroism (CD) spectra of wild-type GnMgl and its mutants were recorded at 25°C on a J-810 spectropolarimeter (JASCO, Japan). All the spectra were collected from 200 to 250 nm at a scanning rate of 200 nm/min with a path length of 0.1 cm. Proteins for CD spectroscopy assays were at a concentration of 0.1 mg/ml in 50 mM Tris-HCl buffer (pH 8.0).
Sequence Analysis
Multiple-sequence alignment was carried out using MUSCLE (Edgar, 2004). Phylogenetic analysis of GnMgl and its homologous sequences was performed using the MEGA 7.0 (Kumar et al., 2016). SignalP 4.0 (Petersen et al., 2011) was used to identify potential signal peptide sequences. PSORTb 3.0 (Yu et al., 2010) was used to predict the location of GnMgl in bacterial cells. The putative secondary structures of GnMgl was predicted by psipred (Buchan et al., 2013).
Abundance Analysis of GnMgl in Marine Bacteria
Bacterial genomes from defined marine environments were identified from NCBI GenBank, and a total of 900 marine bacterial genomes were downloaded. By BLAST analysis against marine bacterial genomes using GnMgl as the query sequence, hits with E-values ≤ 1e–50, ≥ 30% sequence identity and ≥ 50% coverage in length were probed.
Results
Sequence Analysis of the α/β Hydrolase GnMgl From Marine Bacterium Glaciecola nitratireducens FR1064T
A gene encoding an α/β hydrolase (accession number WP_014107521), designated GnMgl, was identified from the genome sequence of marine bacterium G. nitratireducens FR1064T. GnMgl is 1,026 bp in length, encoding a putative lipolytic enzyme of 341 amino acid residues with a calculated molecular mass of 38.9 kDa and a theoretical isoelectric point of 6.54. GnMgl contains a Hydrolase_4 domain based on BLAST analysis against the Conserved Domains Database (CDD) and Pfam protein domain database. GnMgl may lack an N-terminal signal peptide sequence according to the SignalP 4.0 prediction. In addition, GnMgl is predicted to be a membrane protein by PSORTb 3.0.
GnMgl shows the highest sequence identity to an α/β hydrolase from Glaciecola sp. 33A (WP_101182205, 79% identity) and an uncharacterized lysophospholipase from Glaciecola pallidula (WP_006011195, 79% identity). Among the characterized enzymes, GnMgl was most closely related to the human monoacylglycerol lipase (3HJU) (Labar et al., 2010) with a sequence identity of only 31%, covering 45% of GnMgl sequence.
Phylogenetic Analysis of GnMgl
Homology searches against the ESTHER database suggested that GnMgl is affiliated with the monoglyceridelipase_lysophospholipase family of the Hydrolase_4 superfamily. To reveal the phylogenetic position of GnMgl in the monoglyceridelipase_lysophospholipase family, a phylogenetic tree was constructed with sequences of GnMgl and its homologs from other bacteria, fungi, invertebrates, and vertebrates (Figure 1). In this tree, GnMgl and its bacterial homologs are clustered as a separate group in the monoglyceridelipase_lysophospholipase family. As shown in Figure 1, bacterial homologs, fungal homologs, and invertebrate/vertebrate homologs are grouped into three different branches in the phylogenetic tree, suggesting that enzymes from this family possibly differentiate in these evolutionarily distant organisms. Notably, the two human-associated mycobacterial MGLs, Rv0183 and MSMEG_0220, are clustered within the invertebrate/vertebrate branch (Figure 1).
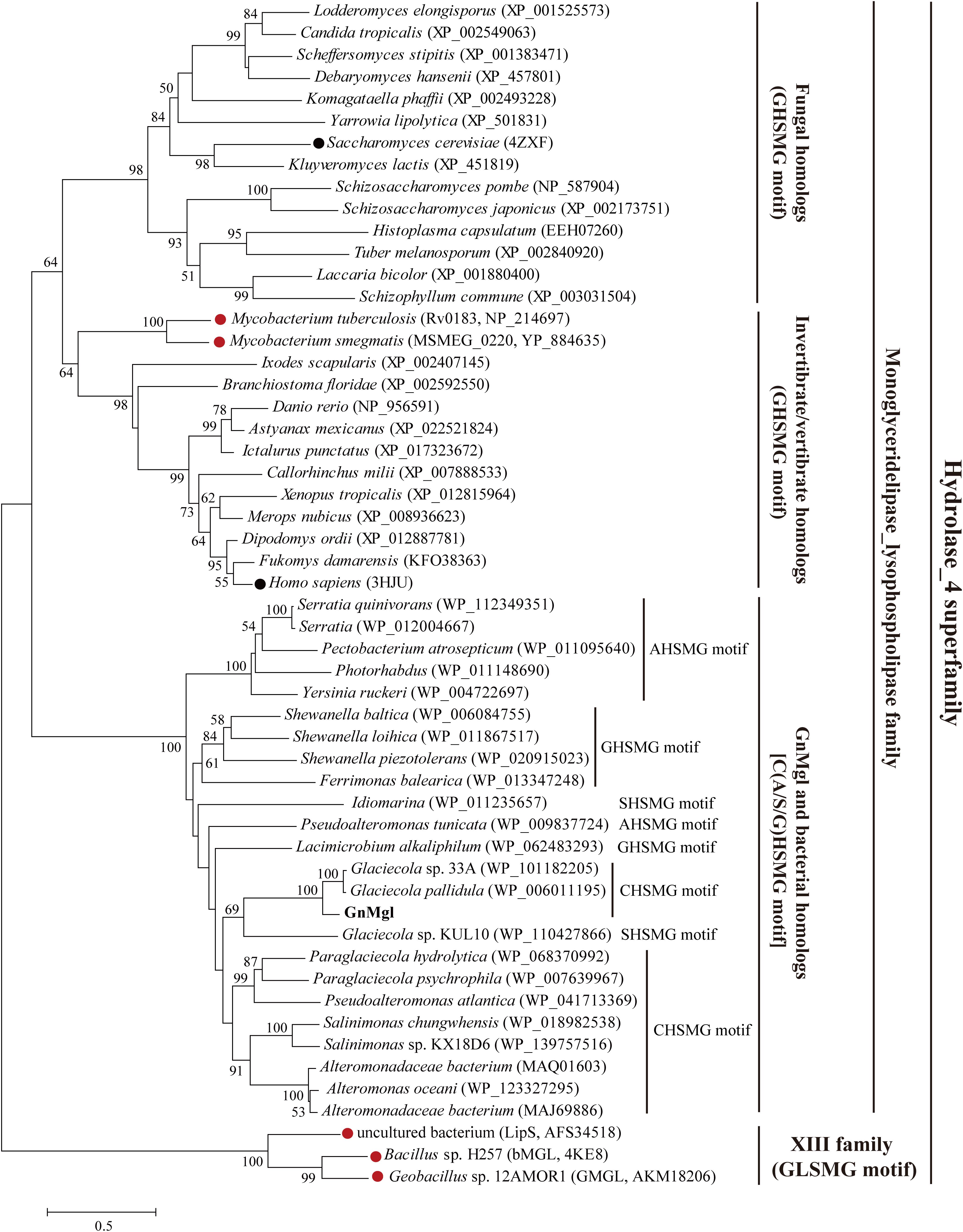
Figure 1. Phylogenetic analysis of GnMgl and its bacterial and eukaryotic homologs. The tree was built by the Neighbor Joining method with a JTT-matrix-based model using 209 amino acid positions. Bootstrap analysis of 1,000 replicates was conducted and values above 50% are shown. The scale for branch length is shown below the tree. Characterized monoacylglycerol lipases (MGLs) from eukaryotes and bacteria are indicated by black and red circles, respectively. The conserved pentapeptide motif containing the catalytic Ser residue is also shown for each sequence.
Multiple sequence alignment suggested that GnMgl has a catalytic triad possibly formed by Ser156, Asp290, and His318 (Figure 2). The potential catalytic Ser156 of GnMgl is located in the CHSMG motif, different from the typical GxSxG motif in other reported MGLs (Figures 1, 2). The oxyanion hole of GnMgl is likely composed of residues Arg58 and Met157 (Figure 2). Multiple sequence alignment combined with psipred prediction suggested that the hydrophobic helix α4 in the cap domain of human MGL is conserved in GnMgl, in spite of its low homology with mammalian MGLs (Figure 2). In human MGL, residues Cys201 and Cys208 in the cap domain and Cys242 near the catalytic site are reported to regulate the enzymatic activity (Scalvini et al., 2016). However, the counterparts of these regulatory cysteine residues are all replaced by non-cysteine residues in GnMgl (Figure 2), implying that GnMgl may be different from mammalian MGLs in catalysis.
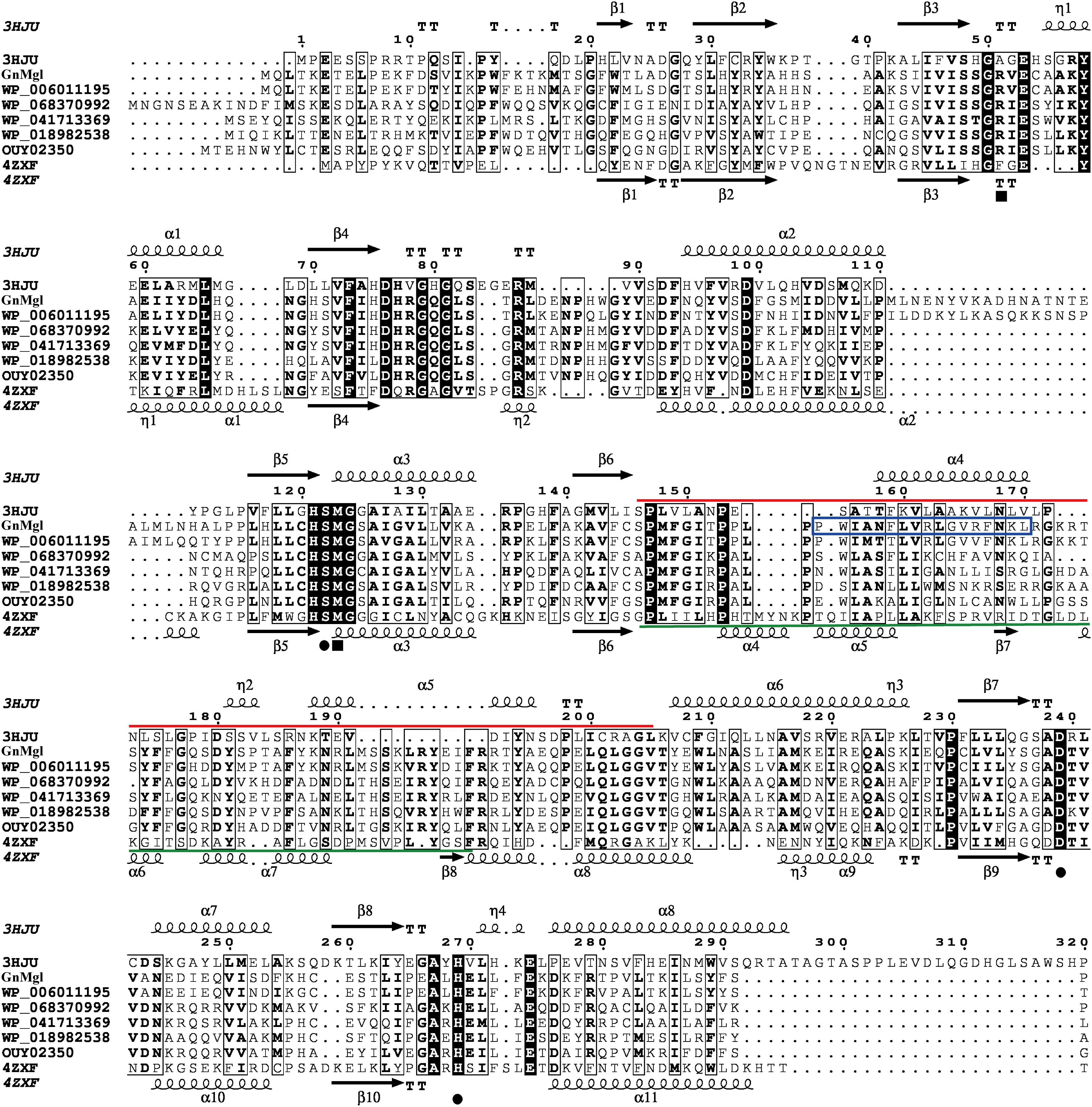
Figure 2. Multiple-sequence alignment of GnMgl with its bacterial and eukaryotic homologs. The two reported MGLs with structures are from human (PDB code 3HJU) and yeast (PDB code 4ZXF), respectively. Using ESPript, secondary structures of human MGL are shown above alignment and secondary structures of yeast MGL under alignment. Helices are indicated by springs, strands by arrows, turns by TT letters, and 310-helices by η letters. Identical residues are shown in white on a black background, and similar residues are in bold black. Solid circles represent catalytic residues, and solid squares represent oxyanion hole residues. The cap domain of human MGL is labeled by a red line, and the cap domain of yeast MGL by a green line. The putative helix in GnMgl corresponding to the lipophilic helix α4 of human MGL is boxed in blue.
Expression and Purification of GnMgl
GnMgl was over-expressed in E. coli BL21 (DE3), and the resulting recombinant protein was first purified by Ni affinity chromatography and then eluted by gel filtration chromatography. Sodium dodecyl sulfate polyacrylamide gel electrophoresis (SDS-PAGE) showed that the purified GnMgl exhibits an apparent molecular mass of approximately 39 kDa (Figure 3A), consistent with the calculated molecular mass of GnMgl. Gel filtration analysis suggested that, like the human MGL (residues 1–303) (Schalk-Hihi et al., 2011), the purified GnMgl tends to form aggregates in solution even in the presence of the detergents such as DDM (Figure 3B).
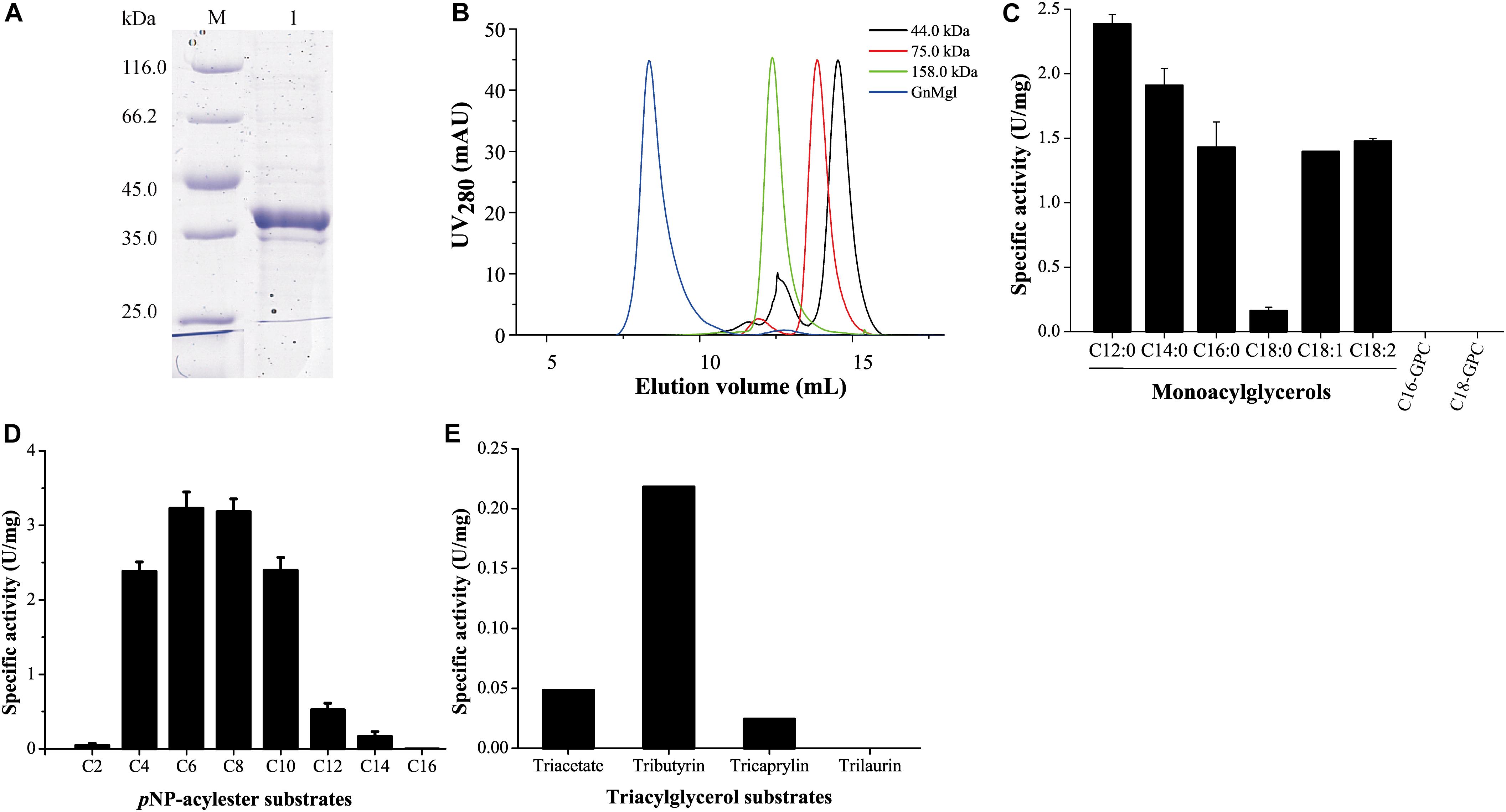
Figure 3. Substrate specificity of GnMgl. (A) SDS-PAGE analysis of purified GnMgl. Lane M, protein mass markers; lane 1, purified GnMgl. (B) Gel filtration analysis of GnMgl. GnMgl monomer has a calculated molecular mass of 38.9 kDa. The three protein size markers are ovalbumin (44.0 kDa), conalbumin (75.0 kDa), and aldolase (158.0 kDa). (C) GnMgl activity against monoacylglycerols and lysophospholipid substrates. (D) GnMgl activity against pNP-acylesters. (E) GnMgl activity against triacylglycerols. The graphs in (C–E) show data from triplicate experiments (mean ± SD).
GnMgl Functions as a Monoacylglycerol Lipase
Phylogenetic analysis showed that GnMgl belongs to the monoglyceridelipase_lysophospholipase family (Figure 1), suggesting that GnMgl may have MGL and/or lysophospholipase activity. Therefore, the enzymatic activity of GnMgl was assayed against monoacylglycerols, lysophospholipids, pNP-acylesters, and triacylglycerols (Figure 3). GnMgl had no detectable activity toward 1-palmitoyl-glycerophosphocholine (C16-GPC) and 1-stearoyl-glycerophosphocholine (C18-GPC) (Figure 3C), typical substrates for lysophospholipases, indicating that GnMgl is not a lysophospholipase. However, GnMgl could hydrolyze saturated MGs with chain length of 12–16 carbon atoms and unsaturated monostearoylglycerol (C18:0) containing one or two double bonds (Figure 3C), indicating that GnMgl acts as a MGL. Among the tested MG substrates, GnMgl showed the maximum activity toward monolauroylglycerol (C12:0) with a specific activity of 2.4 U/mg (Figure 3C). Like other MGLs (Riegler-Berket et al., 2018; Tang et al., 2019), GnMgl could hydrolyze pNP-acylesters (pNPC4-pNPC14), with the highest activity toward pNPC6 and pNPC8 (∼3.2 U/mg) (Figure 3D). In addition, GnMgl showed weak hydrolytic activity toward short-chain triacylglycerols (0.02–0.22 U/mg) (Figure 3E).
GnMgl Is a Cold-Active and Alkaline Enzyme
The optimum temperature for GnMgl activity was 30°C (Figure 4A). At 0°C, GnMgl was still active, retaining 30% of the maximum activity. GnMgl activity was sharply decreased at temperatures over 35°C and completely lost at 50°C. For thermostability, GnMgl retained more than 80% of its highest activity after 1 h incubation at 30°C (Figure 4B). GnMgl became unstable at temperatures over 30°C, losing a half of its activity after 30 min incubation at 35°C and over 80% activity after 10 min incubation at 40°C (Figure 4B). Our results above suggested that GnMgl is a cold-adapted enzyme and stable at temperatures lower than 30°C.
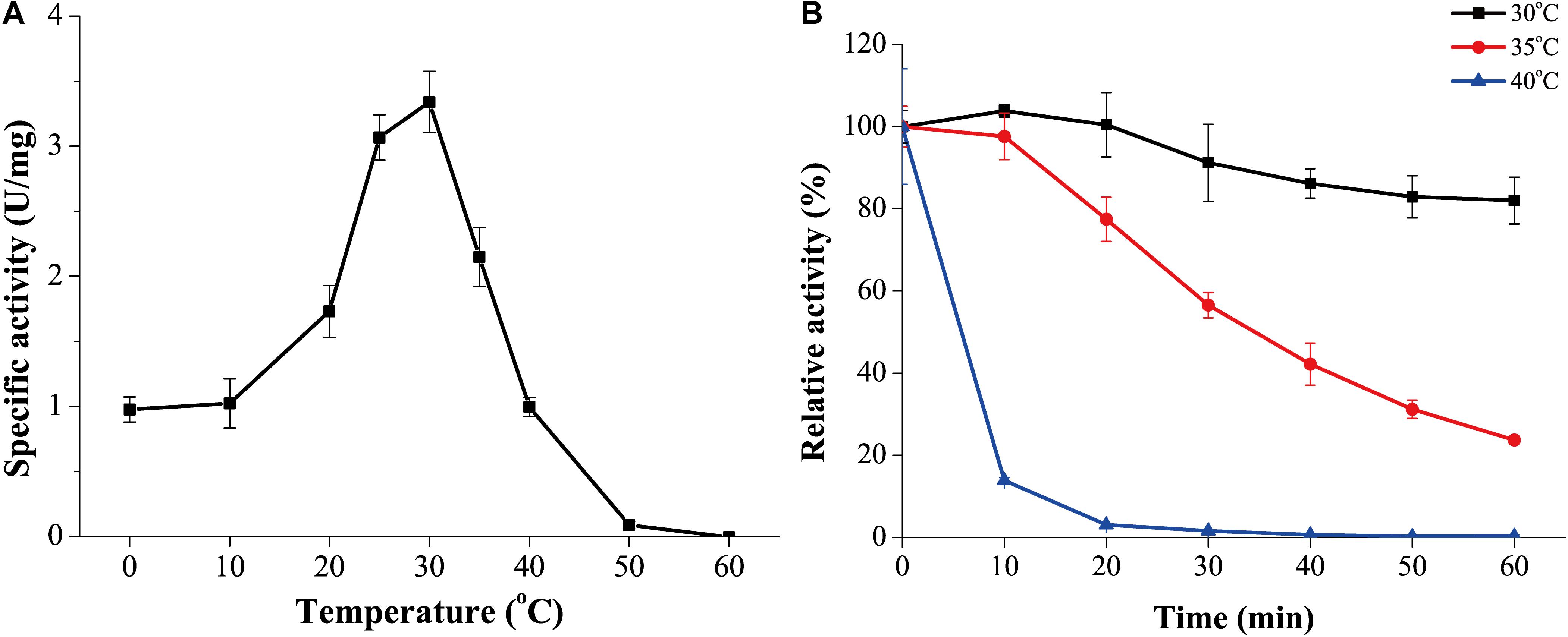
Figure 4. Effect of temperature on the activity and stability of GnMgl. (A) Effect of temperature on GnMgl activity. (B) Effect of temperature on the stability of GnMgl. The residual activity of GnMgl was measured after incubation at different time and temperatures. The activity of GnMgl at 0°C (3.10 ± 0.14 U/mg) was defined as 100%. The graphs show data from triplicate experiments (mean ± SD).
GnMgl was active at pH values between 7.0 and 11.0, and the optimum pH was 9.0 (Figure 5A), suggesting that GnMgl acts as an alkaline enzyme, consistent with its marine origin. GnMgl was stable in a wide pH range of 5.0 to 11.0 (Figure 5B). After incubation for 1 h, GnMgl retained over 66% activity in the pH range of 5.0 to 11.0.
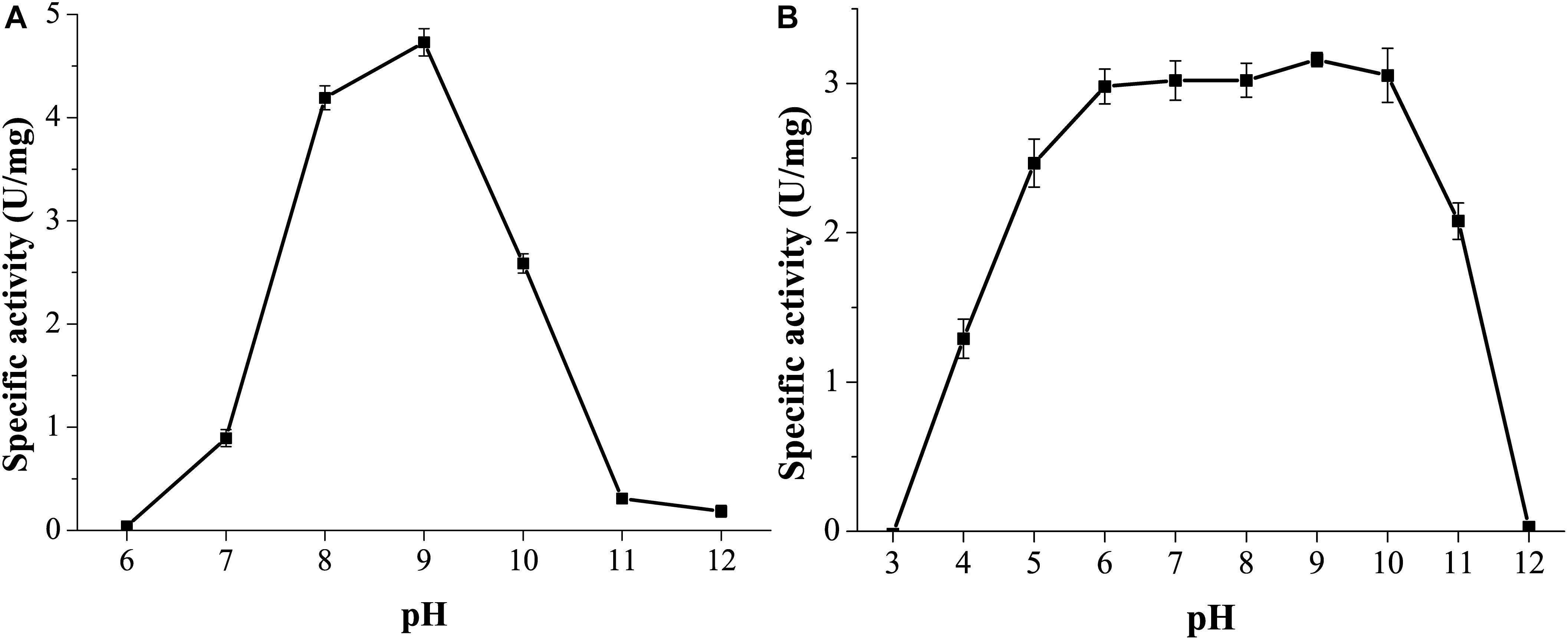
Figure 5. Effect of pH on the activity and stability of GnMgl. (A) Effect of pH on GnMgl activity. (B) Effect of pH on the stability of GnMgl. The residual activity was measured at pH 8.0 and 30°C after incubation at different pH for 1 h. The graphs show data from triplicate experiments (mean ± SD).
GnMgl Is a Halotolerant Enzyme
Marine environments usually contain ∼3.5% (w/v) NaCl. Moreover, the marine strain G. nitratireducens FR1064T where GnMgl comes from was found to be halophilic, requiring 2–9% (w/v) sea salts (optimum of 4–7%) for growth (Baik et al., 2006). Therefore, the effect of NaCl on the activity and stability of GnMgl was investigated. GnMgl still had full activity in NaCl at a concentration of as high as 3.5M (Figure 6A), indicating that GnMgl has high salt tolerance. Furthermore, after 24 h incubation in 4.5M NaCl, GnMgl still retained 75% activity (Figure 6B). These results show that GnMgl is a halotolerant enzyme.
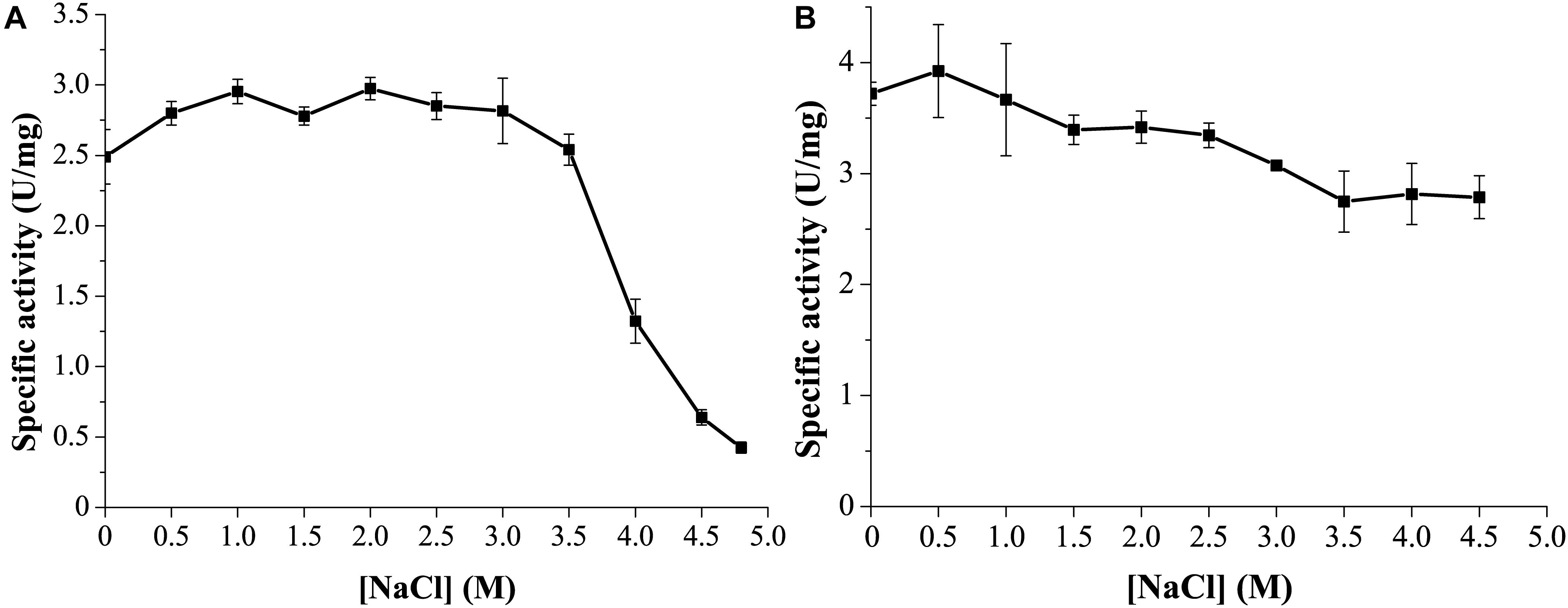
Figure 6. Effect of NaCl on the activity and stability of GnMgl. (A) Effect of NaCl on GnMgl activity. (B) Effect of NaCl on the stability of GnMgl. The residual activity was measured after incubation at different concentrations of NaCl and 4°C for 24 h. The graphs show data from triplicate experiments (mean ± SD).
GnMgl Is Tolerant to Metal Ions, Detergents, and Organic Solvents
Among all the tested metal ions, only 10 mM of Cu2+ or Zn2+ severely inhibited GnMgl activity, whereas the other metal ions almost had no effect on GnMgl activity (Table 2). EDTA had no effect on GnMgl activity, indicating that the catalysis mediated by GnMgl may not require metal ions (Table 3). Like other MGLs, GnMgl was strongly inhibited by 10 mM PMSF, suggesting that GnMgl is possibly a serine hydrolase. GnMgl was also partially inhibited by 1 mM JZL184, a selective MGL inhibitor, by approximately 25% activity. Different from human MGL which is inhibited by N-arachidonoylmaleimide (NAM), a selective sulfhydryl-reactive MAGL inhibitor, with nanomolar level by targeting Cys201 and Cys242 (Zvonok et al., 2008; King et al., 2009; Laitinen et al., 2014), the MGL activity of GnMgl is hardly impacted by NAM with a concentration as high as 1 mM. The inhibition results combined with sequence analysis (Figure 2) suggest that the role of cysteine residues in the catalysis by GnMgl may be different from those of mammalian MGLs. GnMgl showed high resistance to reductants DTT and β-mercaptoethanol, chaotropic agents urea and thiourea, and detergents Tween 20 and Triton X-100 (Tables 3, 4). However, GnMgl was completely inactivated by 0.01% (w/v) SDS (Table 4). In addition, GnMgl showed good tolerance to all the tested organic solvents at 10% (v/v) concentration except for acetonitrile (Table 5).
Identification of the Catalytic Triad of GnMgl Based on Mutational Analysis
Multiple sequence alignment suggested that the catalytic triad of GnMgl is most likely composed of residues Ser156, Asp290, and His318, and that the catalytic Ser156 is located in the CHSMG motif rather than in the typical GxSxG motif (Figures 1, 2). To confirm this, site-directed mutagenesis on these residues was carried out. Mutation of any of these residues to Ala led to the loss of the full activity (Figure 7A), thus confirming the key role of residues Ser156, Asp290, and His318 in catalysis by GnMgl. CD spectroscopy analysis showed that the secondary structures of the mutants exhibited little deviation from that of the wild-type GnMgl, indicating that the changes in the enzymatic activity of the mutants are caused by residue substitution rather than structural changes in GnMgl (Figure 7B).
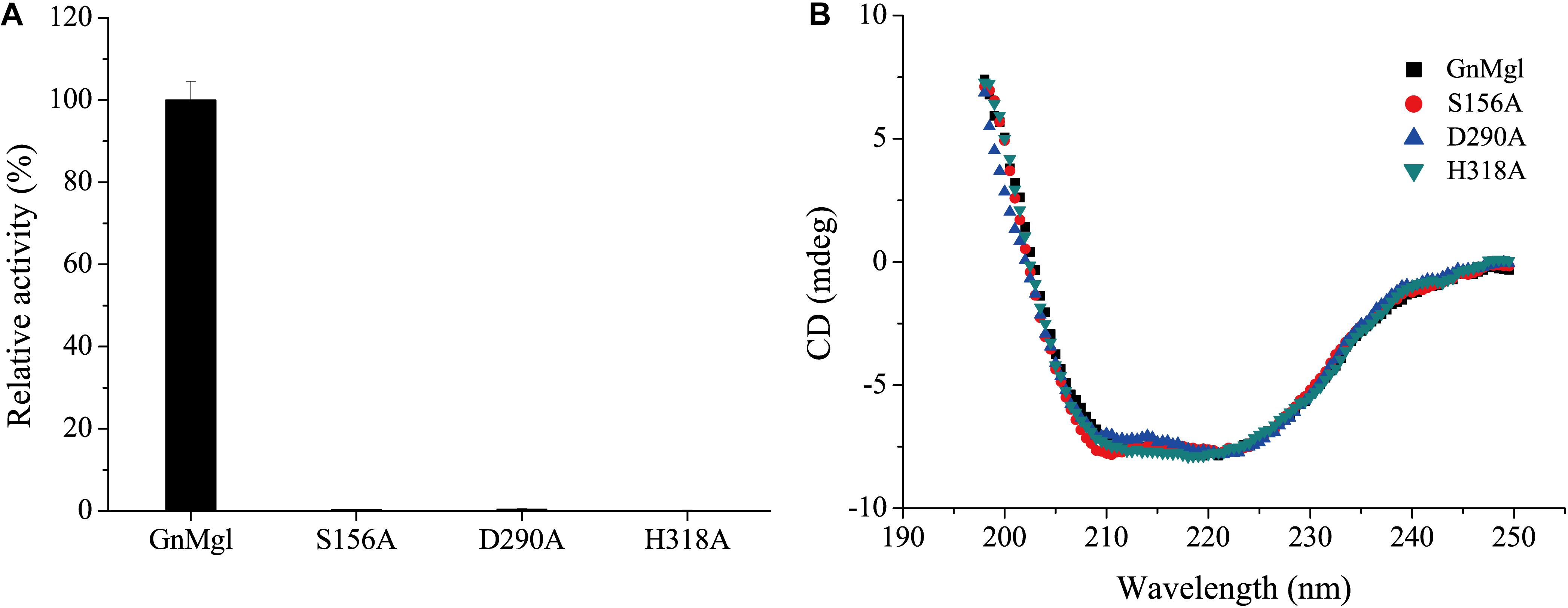
Figure 7. Enzymatic activities (A) and CD spectra (B) of wild-type GnMgl and its mutants. In (A), reactions were conducted in triplicate in 100 mM Tris-HCl buffer (pH 8.0) containing 50 mM CHAPS at 30°C, and the concentrations of the enzyme and substrate monolauroylglycerol (C12:0) used were 0.15–0.29 μM and 2 mM, respectively. The activity of wild-type GnMgl (1.40 ± 0.01 U/mg) was defined as 100%.
GnMgl Homologs Are Widespread in Marine Bacteria
To reveal the distribution of GnMgl-like sequences in marine bacteria, a total of 900 bacterial genomes with defined marine origins including seawater, marine sediment, sea ice, algae, fish, and other marine animals were downloaded from NCBI GenBank, which were affiliated with 107 genera. BLAST analysis revealed that GnMgl homologs are found in 347 marine bacterial genomes, covering 38.5% of marine bacteria. GnMgl homologs are distributed in 35 genera of Gammaproteobacteria, Alphaproteobacteria, Deltaproteobacteria, Bacteroidetes, Firmicutes, and Cyanobacteria (Figure 8), covering 32.7% of marine bacterial genera. These results show the abundance of GnMgl homologs in marine bacteria. Marine bacteria containing GnMgl-like sequences are dominated by Alphaproteobacteria belonging to the genera Pseudoalteromonas (34.6%), Shewanella (11.5%), Alteromonas (11.2%), and Photobacterium (9.2%) (Figure 8). Like GnMgl, most of its marine bacterial homologs contain a potential catalytic Ser residue located in unusual C(A/S)HSMG motifs (Table 6).
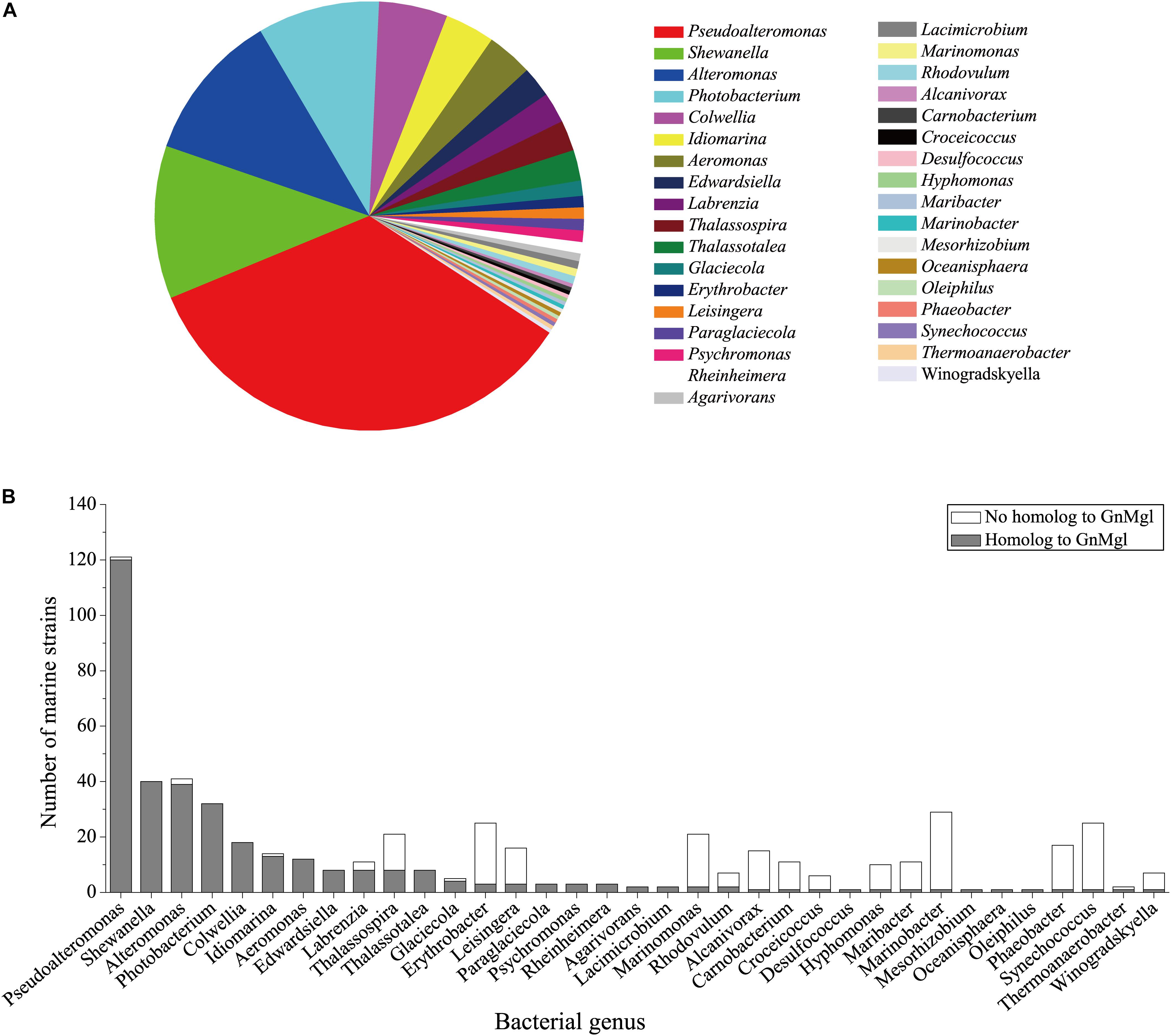
Figure 8. Distribution of GnMgl-like sequences in marine bacteria (A) and abundance of GnMgl-like sequences in each marine bacterial genus (B).
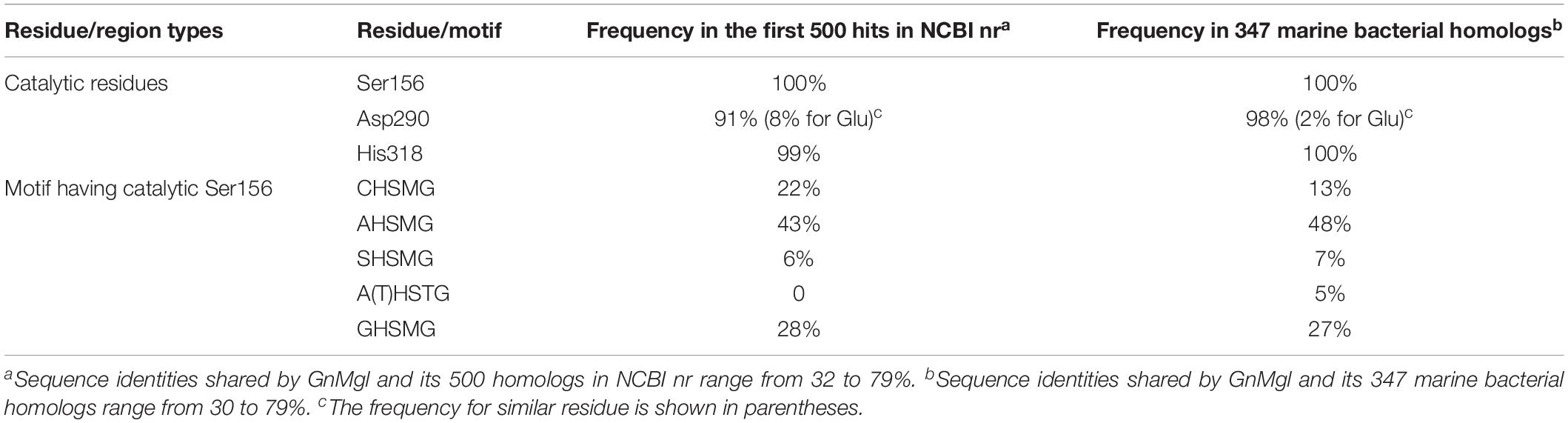
Table 6. Frequencies of key residues and motifs of GnMgl in the first 500 hits in NCBI nr or 347 marine bacterial homologs using BLASTP.
Discussion
Monoacylglycerol lipases are widely distributed among organisms ranging from bacteria to mammals. However, reports on bacterial MGLs are still limited and none of them is from marine environments vastly subject to low temperature, high salts, and oligotrophy. In this study, a novel MGL, GnMgl, from the marine bacterial strain G. nitratireducens FR1064T was characterized. GnMgl is distantly related to characterized MGLs in sequence with similarity lower than 31%. Phylogenetic analysis also suggested that GnMgl and its bacterial homologs are clustered as a separate group in the monoglyceridelipase_lysophospholipase family of the Hydrolase_4 superfamily (Figure 1). In addition, different from reported MGLs with a conserved GH(L)SMG catalytic motif, GnMgl and most of its bacterial homologs harbor a catalytic Ser residue located in an atypical GxSxG motif, the conserved C(A/S)HSMG motif (Figure 1 and Table 6), suggesting that GnMgl-like enzymes might be different from reported MGLs in catalysis. Thus, GnMgl and its bacterial homologs represent a new group of MGLs.
Biochemical analysis revealed that recombinant GnMgl had no lysophospholipase activity but could hydrolyze saturated (C12:0-C16:0) and unsaturated (C18:1 and C18:2) MGs (Figure 3 and Table 7). For MG substrates, GnMgl displayed similar substrate preference to that of bMGL from Bacillus sp. H257 (Imamura and Kitaura, 2000; Kitaura et al., 2001). Both GnMgl and bMGL showed limited hydrolytic activity toward saturated monostearoylglycerol (C18:0), whereas other bacterial MGLs such as LipS (Riegler-Berket et al., 2018), Rv0183 (Cotes et al., 2007), MSMEG_0220 (Dhouib et al., 2010), and pMGL (Sakiyama et al., 2001) all could strongly degrade it. Furthermore, the maximum specific activity of GnMgl (2.4 U/mg) toward MGs was found to be about two orders of magnitude lower than those of other bacterial MGLs (80–620 U/mg) (Table 7). For triacylglycerol substrates, most reported bacterial MGLs showed no activity. However, GnMgl was capable of hydrolyzing short-chain triacylglycerols, which was also observed in the case of Rv0183 (Cotes et al., 2007). In conclusion, marine GnMgl is distinct from reported bacterial MGLs in both substrate selectivity and enzymatic activity.
The marine strain FR1064T where GnMgl comes from was reported to be cold-adapted and halophilic, growing at temperatures between 4 and 30°C (optimum of 25°C) within 2–9% (w/v) sea salts (optimum of 4–7%) (Baik et al., 2006; Qin et al., 2014). Biochemical characterization revealed that GnMgl displays similar characteristics to its source strain. GnMgl had the maximum activity at 30°C and retained 30% activity at 0°C. GnMgl is a cold-adapted enzyme, different from reported bacterial MGLs which are all thermophilic/mesophilic enzymes (Table 7). GnMgl is also a halotolerant enzyme, whose activity was not affected by 3.5M NaCl. The cold-adapted and salt-tolerant characteristics of GnMgl may help the adaption of the strain FR1064T to the cold and saline marine environment.
PSORTb prediction suggests that GnMgl is most likely a membrane protein. The hydrophobic helix α4 in the cap domain was proposed to allow human MGL to anchor in the membrane in order to recruit its lipid substrates (Labar et al., 2010). Interestingly, the counterpart of this lipophilic helix is also found in GnMgl based on multiple sequence alignment and psipred prediction (Figure 2), which might play similar role in GnMgl as in human MGL. Moreover, the substrate preference of GnMgl correlates to the most abundant fatty acids (Baik et al., 2006) within the strain FR1064T. Thus, GnMgl is thought to play roles in the lipid catabolism and membrane lipid remodeling in this marine bacterium. GnMgl showed the maximum activity toward monolauroylglycerol (C12:0) which is highly toxic to bacteria, suggesting that GnMgl may also function in bacterial detoxification process. Furthermore, homologs to GnMgl are found to be abundant in various marine bacteria, suggesting that they may play an important physiological role in these marine bacteria. Therefore, our study will shed light on marine MGLs and their physiological roles in marine bacteria, and additional studies are required to further illustrate the function of GnMgl-like proteins in lipid catabolism and/or detoxification of their source strains through gene overexpression or deletion/reduction.
Data Availability Statement
The protein sequence of GnMgl can be found in the GenBank WP_014107521.
Author Contributions
Y-ZZ and X-YS designed the research. X-LC and P-YL directed the research. P-YL, Y-QZ, YZ, and W-XJ performed the experiments. L-SZ, Z-ZS, Y-JW, Y-SZ, and C-YL helped in data analysis. P-YL and Y-QZ wrote the manuscript. X-LC and MS edited the manuscript.
Funding
The work was supported by the Pilot National Laboratory for Marine Science and Technology (Qingdao) (QNLM2016ORP0310), National Natural Science Foundation of China (grants 91751101, 41676180, 31870052, 31670497, and 41906195), the National Key R&D Program of China (grants 2018YFC0310704 and 2018YFC1406700), the AoShan Talents Cultivation Program Supported by Pilot National Laboratory for Marine Science and Technology (Qingdao) (2017ASTCP-OS14), the program of Preparation of Active Marine Protein Feed Additives and Construction of Industrial Chain from Development of Marine Economy Demonstration City Program during the 13th Five- Year Plan Period, Taishan Scholars Program of Shandong Province (tspd20181203), and the Young Scholars Program of Shandong University (2017WLJH57).
Conflict of Interest
The authors declare that the research was conducted in the absence of any commercial or financial relationships that could be construed as a potential conflict of interest.
References
Aschauer, P., Rengachari, S., Lichtenegger, J., Schittmayer, M., Das, K. M., Mayer, N., et al. (2016). Crystal structure of the Saccharomyces cerevisiae monoglyceride lipase Yju3p. Biochim. Biophys. Acta 1861, 462–470. doi: 10.1016/j.bbalip.2016.02.005
Baik, K. S., Park, Y. D., Seong, C. N., Kim, E. M., Bae, K. S., and Chun, J. (2006). Glaciecola nitratireducens sp. nov., isolated from seawater. Int. J. Syst. Evol. Microbiol. 56(Pt 9), 2185–2188. doi: 10.1099/ijs.0.64330-0
Bian, F., Qin, Q. L., Xie, B. B., Shu, Y. L., Zhang, X. Y., Yu, Y., et al. (2011). Complete genome sequence of seawater bacterium Glaciecola nitratireducens FR1064(T). J. Bacteriol. 193, 7006–7007. doi: 10.1128/JB.06296-11
Buchan, D. W., Minneci, F., Nugent, T. C., Bryson, K., and Jones, D. T. (2013). Scalable web services for the PSIPRED protein analysis workbench. Nucleic Acids Res. 41, W349–W357. doi: 10.1093/nar/gkt381
Chow, J., Kovacic, F., Dall Antonia, Y., Krauss, U., Fersini, F., Schmeisser, C., et al. (2012). The metagenome-derived enzymes LipS and LipT increase the diversity of known lipases. PLoS One 7:e47665. doi: 10.1371/journal.pone.0047665
Conley, A. J., and Kabara, J. J. (1973). Antimicrobial action of esters of polyhydric alcohols. Antimicrob. Agents Chemother. 4, 501–506. doi: 10.1128/aac.4.5.501
Cotes, K., Dhouib, R., Douchet, I., Chahinian, H., de Caro, A., Carriere, F., et al. (2007). Characterization of an exported monoglyceride lipase from Mycobacterium tuberculosis possibly involved in the metabolism of host cell membrane lipids. Biochem. J. 408, 417–427. doi: 10.1042/BJ20070745
Dhouib, R., Laval, F., Carriere, F., Daffe, M., and Canaan, S. (2010). A monoacylglycerol lipase from Mycobacterium smegmatis Involved in bacterial cell interaction. J. Bacteriol. 192, 4776–4785. doi: 10.1128/JB.00261-10
Edgar, R. C. (2004). MUSCLE: multiple sequence alignment with high accuracy and high throughput. Nucleic Acids Res. 32, 1792–1797. doi: 10.1093/nar/gkh340
Fredrikson, G., Tornqvist, H., and Belfrage, P. (1986). Hormone-sensitive lipase and monoacylglycerol lipase are both required for complete degradation of adipocyte triacylglycerol. Biochim. Biophys. Acta 876, 288–293. doi: 10.1016/0005-2760(86)90286-9
Gil-Ordóñez, A., Martín-Fontecha, M., Ortega-Gutiérrez, S., and López-Rodríguez, M. L. (2018). Monoacylglycerol lipase (MAGL) as a promising therapeutic target. Biochem. Pharmacol. 157, 18–32. doi: 10.1016/j.bcp.2018.07.036
Hohmann, A. G., Suplita, R. L., Bolton, N. M., Neely, M. H., Fegley, D., Mangieri, R., et al. (2005). An endocannabinoid mechanism for stress-induced analgesia. Nature 435, 1108–1112. doi: 10.1038/nature03658
Imamura, S., and Kitaura, S. (2000). Purification and characterization of a monoacylglycerol lipase from the moderately thermophilic Bacillus sp. H-257. J. Biochem. 127, 419–425. doi: 10.1093/oxfordjournals.jbchem.a022623
Kabara, J. J., and Vrable, R. (1977). Antimicrobial lipids: natural and synthetic fatty acids and monoglycerides. Lipids 12, 753–759. doi: 10.1007/bf02570908
King, A. R., Lodola, A., Carmi, C., Fu, J., Mor, M., and Piomelli, D. (2009). A critical cysteine residue in monoacylglycerol lipase is targeted by a new class of isothiazolinone-based enzyme inhibitors. Br. J. Pharmacol. 157, 974–983. doi: 10.1111/j.1476-5381.2009.00276.x
Kitaura, S., Suzuki, K., and Imamura, S. (2001). Monoacylglycerol lipase from moderately thermophilic Bacillus sp. strain H-257: molecular cloning, sequencing, and expression in Escherichia coli of the gene. J. Biochem. 129, 397–402. doi: 10.1093/oxfordjournals.jbchem.a002870
Kovacic, F., Granzin, J., Wilhelm, S., Kojic-Prodic, B., Batra-Safferling, R., and Jaeger, K. E. (2013). Structural and functional characterisation of TesA - a novel lysophospholipase A from Pseudomonas aeruginosa. PLoS One 8:e69125. doi: 10.1371/journal.pone.0069125
Kumar, S., Stecher, G., and Tamura, K. (2016). MEGA7: molecular evolutionary genetics analysis version 7.0 for bigger datasets. Mol. Biol. Evol. 33, 1870–1874. doi: 10.1093/molbev/msw054
Labar, G., Bauvois, C., Borel, F., Ferrer, J. L., Wouters, J., and Lambert, D. M. (2010). Crystal structure of the human monoacylglycerol lipase, a key actor in endocannabinoid signaling. Chembiochem 11, 218–227. doi: 10.1002/cbic.200900621
Laitinen, T., Navia-Paldanius, D., Rytilahti, R., Marjamaa, J. J., Karizkova, J., Parkkari, T., et al. (2014). Mutation of Cys242 of human monoacylglycerol lipase disrupts balanced hydrolysis of 1- and 2-monoacylglycerols and selectively impairs inhibitor potency. Mol. Pharmacol. 85, 510–519. doi: 10.1124/mol.113.090795
Lenfant, N., Hotelier, T., Velluet, E., Bourne, Y., Marchot, P., and Chatonnet, A. (2013). ESTHER, the database of the alpha/beta-hydrolase fold superfamily of proteins: tools to explore diversity of functions. Nucleic Acids Res. 41, D423–D429. doi: 10.1093/nar/gks1154
Li, P. Y., Ji, P., Li, C. Y., Zhang, Y., Wang, G. L., Zhang, X. Y., et al. (2014). Structural basis for dimerization and catalysis of a novel esterase from the GTSAG motif subfamily of the bacterial hormone-sensitive lipase family. J. Biol. Chem. 289, 19031–19041. doi: 10.1074/jbc.M114.574913
Micale, V., Di Marzo, V., Sulcova, A., Wotjak, C. T., and Drago, F. (2013). Endocannabinoid system and mood disorders: priming a target for new therapies. Pharmacol. Ther. 138, 18–37. doi: 10.1016/j.pharmthera.2012.12.002
Nomura, D. K., Long, J. Z., Niessen, S., Hoover, H. S., Ng, S. W., and Cravatt, B. F. (2010). Monoacylglycerol lipase regulates a fatty acid network that promotes cancer pathogenesis. Cell 140, 49–61. doi: 10.1016/j.cell.2009.11.027
Petersen, T. N., Brunak, S., von Heijne, G., and Nielsen, H. (2011). SignalP 4.0: discriminating signal peptides from transmembrane regions. Nat. Methods 8, 785–786. doi: 10.1038/nmeth.1701
Qin, Q. L., Xie, B. B., Yu, Y., Shu, Y. L., Rong, J. C., Zhang, Y. J., et al. (2014). Comparative genomics of the marine bacterial genus Glaciecola reveals the high degree of genomic diversity and genomic characteristic for cold adaptation. Environ. Microbiol. 16, 1642–1653. doi: 10.1111/1462-2920.12318
Rengachari, S., Aschauer, P., Schittmayer, M., Mayer, N., Gruber, K., Breinbauer, R., et al. (2013). Conformational plasticity and ligand binding of bacterial monoacylglycerol lipase. J. Biol. Chem. 288, 31093–31104. doi: 10.1074/jbc.M113.491415
Riegler-Berket, L., Leitmeier, A., Aschauer, P., Dreveny, I., and Oberer, M. (2018). Identification of lipases with activity towards monoacylglycerol by criterion of conserved cap architectures. Biochim. Biophys. Acta 1863, 679–687. doi: 10.1016/j.bbalip.2018.03.009
Sakiyama, T., Yoshimi, T., Miyake, A., Umeoka, M., Tanaka, A., Ozaki, S., et al. (2001). Purification and characterization of a monoacylglycerol lipase from Pseudomonas sp. LP7315. J. Biosci. Bioeng. 91, 27–32.
Scalvini, L., Piomelli, D., and Mor, M. (2016). Monoglyceride lipase: structure and inhibitors. Chem. Phys. Lipids 197, 13–24. doi: 10.1016/j.chemphyslip.2015.07.011
Schalk-Hihi, C., Schubert, C., Alexander, R., Bayoumy, S., Clemente, J. C., Deckman, I., et al. (2011). Crystal structure of a soluble form of human monoglyceride lipase in complex with an inhibitor at 1.35 A resolution. Protein Sci. 20, 670–683. doi: 10.1002/pro.596
Tang, W., Lan, D., Zhao, Z., Li, S., Li, X., and Wang, Y. (2019). A thermostable monoacylglycerol lipase from marine Geobacillus sp. 12AMOR1: biochemical characterization and mutagenesis study. Int. J. Mol. Sci. 20:E780. doi: 10.3390/ijms20030780
Taschler, U., Radner, F. P., Heier, C., Schreiber, R., Schweiger, M., Schoiswohl, G., et al. (2011). Monoglyceride lipase deficiency in mice impairs lipolysis and attenuates diet-induced insulin resistance. J. Biol. Chem. 286, 17467–17477. doi: 10.1074/jbc.M110.215434
Xia, Y., Chu, W., Qi, Q., and Xun, L. (2015). New insights into the QuikChange process guide the use of Phusion DNA polymerase for site-directed mutagenesis. Nucleic Acids Res. 43:e12. doi: 10.1093/nar/gku1189
Yu, N. Y., Wagner, J. R., Laird, M. R., Melli, G., Rey, S., Lo, R., et al. (2010). PSORTb 3.0: improved protein subcellular localization prediction with refined localization subcategories and predictive capabilities for all prokaryotes. Bioinformatics 26, 1608–1615. doi: 10.1093/bioinformatics/btq249
Zvonok, N., Pandarinathan, L., Williams, J., Johnston, M., Karageorgos, I., Janero, D. R., et al. (2008). Covalent inhibitors of human monoacylglycerol lipase: ligand-assisted characterization of the catalytic site by mass spectrometry and mutational analysis. Chem. Biol. 15, 854–862. doi: 10.1016/j.chembiol.2008.06.008
Keywords: α/β hydrolase, monoacylglycerol lipase, marine bacterium, cold-adapted enzyme, halotolerance
Citation: Li P-Y, Zhang Y-Q, Zhang Y, Jiang W-X, Wang Y-J, Zhang Y-S, Sun Z-Z, Li C-Y, Zhang Y-Z, Shi M, Song X-Y, Zhao L-S and Chen X-L (2020) Study on a Novel Cold-Active and Halotolerant Monoacylglycerol Lipase Widespread in Marine Bacteria Reveals a New Group of Bacterial Monoacylglycerol Lipases Containing Unusual C(A/S)HSMG Catalytic Motifs. Front. Microbiol. 11:9. doi: 10.3389/fmicb.2020.00009
Received: 23 October 2019; Accepted: 06 January 2020;
Published: 23 January 2020.
Edited by:
Philippe M. Oger, UMR5240 Microbiologie, Adaptation et Pathogenie (MAP), FranceReviewed by:
Kwang-Mook Jung, University of California, Irvine, United StatesLada Petrovskaya, Institute of Bioorganic Chemistry (RAS), Russia
Copyright © 2020 Li, Zhang, Zhang, Jiang, Wang, Zhang, Sun, Li, Zhang, Shi, Song, Zhao and Chen. This is an open-access article distributed under the terms of the Creative Commons Attribution License (CC BY). The use, distribution or reproduction in other forums is permitted, provided the original author(s) and the copyright owner(s) are credited and that the original publication in this journal is cited, in accordance with accepted academic practice. No use, distribution or reproduction is permitted which does not comply with these terms.
*Correspondence: Long-Sheng Zhao, cmVhbHdvYWlsdW9AMTYzLmNvbQ==; Xiu-Lan Chen, Y3hsMDQyM0BzZHUuZWR1LmNu
†These authors have contributed equally to this work