- International Cooperative Laboratory of Traditional Chinese Medicine Modernization and Innovative Drug Development of Chinese Ministry of Education (MOE), College of Pharmacy, Jinan University, Guangzhou, China
3′,5′ Cyclic diguanylic acid (c-di-GMP) has been shown to play a central role in the regulation of bacterial physiological processes such as biofilm formation and virulence production, and is regarded as a potential target for the development of anti-infective drugs. A method for the facile detection of the bacterial level of cellular c-di-GMP is required to explore the details of c-di-GMP signaling and design drugs on the basis of this pathway. Current methods of c-di-GMP detection have limited sensitivity or difficultly in probe preparation. Herein a new fluorescent probe is reported for the detection of c-di-GMP at concentrations as low as 500 nM. The probe was developed on the basis of the G-quadruplex formation of c-di-GMP induced by aromatic molecules. When used on crude bacterial cell lysates, it can effectively distinguish between the low c-di-GMP levels of bacteria in plankton and the high c-di-GMP levels in biofilm. The method described here is simple, inexpensive, sensitive, and suitable for practical applications involving the rapid detection of cellular c-di-GMP levels in vitro after simple bacterial lysis and filtration.
Introduction
First discovered in 1987 (Ross et al., 1987), 3′,5′-cyclic diguanylic acid (c-di-GMP) is a ubiquitous second messenger that has been shown to play a central role in the regulation of bacterial biofilm formation (Tsiry et al., 2015). The level of c-di-GMP in bacteria has a direct impact on the formation of bacterial biofilm and is closely linked to clinical bacterial biofilm infection (Hall and Lee, 2017). Bacteria within a biofilm are several orders of magnitude more resistant to antibiotics than planktonic bacteria, and more than 80% of malignant infections are associated with bacterial biofilm resistance (Rabin et al., 2015). Consequently, targeting c-di-GMP signaling to inhibit the formation of bacterial biofilm is a new potential antibiofilm method that may overcome antibiotic resistance. As an important intracellular signal, c-di-GMP has also been confirmed to affect many other biological phenotypes of bacteria, including motility, extracellular polysaccharide, eDNA, and virulence factor production (Alina et al., 2008; Ueda and Wood, 2010; Jenal et al., 2017).
Exploration of the details of the physiological processes affected by c-di-GMP signaling is a basis for implementing regulation and drug design using this pathway. Thus, a simple and rapid method for the detection of the cellular c-di-GMP levels in different clinical strains that is suitable for practical application is urgently required to decipher more details of the relationship between c-di-GMP signaling and bacterial pathogenicity and toxicity as well as drug resistance. A readily available detection method for the determination of c-di-GMP content in bacteria is also necessary to quickly evaluate the efficacy of antibiofilm infection drugs targeting c-di-GMP. However, quantitative and selective detection of cellular c-di-GMP in complex mixed metabolites is difficult. Much effort has been invested in developing c-di-GMP detection methods, many of which can now detect c-di-GMP levels in vivo, but methods for quick in vitro overall cellular c-di-GMP level detection remain limited by poor sensitivity, complicated operation, or difficulty in probe preparation. For example, the commonly used c-di-GMP detection method is tandem HPLC-MS (Spangler et al., 2010), which offers a relatively low limit of detection but involves tedious separation and purification steps. Methods using protein-based fluorescent biosensors can be successfully applied to fluorescence imaging in vivo for single bacteria (Rybtke et al., 2012), but if they are used in vitro for quantitative detection of c-di-GMP levels, rigorous separation and extraction of the expressed proteins from bacterial cells and complicated purification to remove the prebinding of c-di-GMP are necessary. The chemiluminescent biosensor recently established by the Hammond lab for in vitro studies of cyclic di-GMP signaling in cell lysates (Dippel et al., 2018) also requires proteins to be expressed and purified from bacteria and minimal c-di-GMP to remain bound. RNA-based biosensor detection methods have developed rapidly in recent years; some biosensors with high sensitivity can offer real-time detection of c-di-GMP levels in living cells (Wang et al., 2016; Yeo et al., 2017, 2018), although some RNA-based biosensors have been synthesized by in vitro transcription for c-di-GMP detection in vitro (Nakayama et al., 2012; Kellenberger et al., 2015; Tsuji and Sintim, 2016), the complexity and high cost of in vitro transcription limit the access of detection probes in most laboratories, making it difficult for such biosensors to be widely used for in vitro detection. Therefore, the development of a simple yet highly sensitive in vitro detection method is still necessary.
C-di-GMP has been reported to form G-quadruplex in the presence of monovalent cations such as potassium ions or certain aromatic small molecular inducers, whereas other nucleic acid metabolites, such as GTP, GMP, cGMP, and c-di-AMP, lack this characteristic. Nakayama et al. demonstrated that the fluorescence of the c-di-GMP G-quadruplex inducer thiazole orange (TO) is enhanced in a c-di-GMP/TO complex (Zhang et al., 2006; Nakayama et al., 2011a,b), which can be used to establish a simple method for c-di-GMP detection in vitro. This method, based on an illuminating fluorescent probe, is easier to operate and the probe is easy to obtain. However, the sensitivity of the current fluorescent probe TO could limit the applicability of this method. The reported fluorescent probe TO with a detection limit of 5 μM has a relatively low sensitivity; the c-di-GMP level of bacteria is often lower than this value—typically less than 2 μM or even less than 50 nM (Hengge, 2009; Simm et al., 2009; Ho et al., 2013). Thus, a relatively large volume of bacterial culture must be processed to detect the c-di-GMP–induced fluorescent signal in the lysate. Its low sensitivity limits its application for simple and rapid detection with a large number of different bacterial samples.
A small aromatic molecule, A18 ((E)-2-(2-(1H-indol-3-yl) vinyl) -3-methyl-3λ4-benzo [d] thiazole iodide), has been designed and synthesized. It is useful for c-di-GMP detection because of its G-quadruplex formation property and offers effective c-di-GMP G-quadruplex induction (Nakayama et al., 2011a,b; Kelsey et al., 2012), enabling the detection limit for c-di-GMP to be reduced to roughly 500 nM. A18 can also distinguish c-di-GMP from other small nucleotides, such as GMP and cGMP. This capability is useful in in vitro assays of bacterial lysate samples in which other macromolecules such as DNA and RNA can be separated from the nucleotides through a simple filtration process. The improvement of sensitivity and selectivity makes the immediate detection and quantification of cellular c-di-GMP levels possible through simple lysis and filtration of bacterial samples, without the need for prior HPLC purification.
Materials and Methods
Materials and Instruments
Fluorescence experiments were performed on a Perkin-Elmer-LS 55 Fluorescence Spectrometer with 1 cm path length cuvette. Absorbance spectra were obtained on a ND2000C spectrophotometer with 1 cm path length cuvette. CD studies were performed by Chirascan Circular Dichroism with 1 mm path length cuvette. Synthetic materials were purchased from Bidepharm. C-di-GMP was purchased from Sigma-Innochem. TO was purchased from Sigma-Aldrich. rGTP, rATP, rCTP, and rUTP were purchased from Promega. dATP, dGTP, dCTP, and dTTP were purchased from Biomiga. GMP and cGMP (guanosine 3′,5′-cyclic monophosphate) were purchased from Biorbyt. 1H NMR spectra (Supplementary Figure S1) and 13C NMR spectra (Supplementary Figure S2) were recorded using a Bruker AM-400 spectrometer or a Bruker AM-300 spectrometer with DMSO-d6 as the solvent and TMS as the internal standard. Electrospray Ionization-Mass Spectrometer (ESI-MS) spectra (Supplementary Figure S3) were recorded with a TRACE METM spectrometer.
Synthesis and Characterization of Fluorescent Probe A18
3-Methyl-3λ4-Benzo[d]thiazole Iodide (2)
2-Methyl benzothiazole (0.76 ml, 6 mmol) was dissolved in MeCN (10 ml). Methyl iodide (0.94 ml, 15 mmol) was added to the solution. Then, the mixture was refluxed for 24 h under N2. After cooling, the resulting precipitate was filtered, then washed with MeCN and Et2O to give a white solid 1.42 g. yield 83%. 1H NMR (400 MHz, DMSO- d6) δ 8.49 (d, J = 8.1 Hz, 1H), 8.28 (d, J = 8.4 Hz, 1H), 7.85 (t, J = 7.8 Hz, 1H), 7.77 (t, J = 7.7 Hz, 1H), 4.23 (s, 3H), 3.23 (s, 3H); 13C NMR (100 MHz, DMSO- d6) δ 177.45, 141.98, 129.70, 129.07, 128.45, 125.02, 117.26, 37.29, 18.40. ESI-MS (m/z):164[M-I]+.
(E)-2-(2-(1H-Indol-3-Yl)Vinyl)-3-Methyl-3λ4-Benzo[d]thiazole Iodide (A18)
A mixture of 2,3-dimethylbenzothiazolium iodide (150 mg, 0.52 mmol) and indole-3-carbaldehyde (90 mg, 0.62 mmol) in MeOH was refluxed for 12 h in the presence of pyridine (21 μl, 0.26 mmol). The reaction mixture was allowed to cool slowly to room temperature, and a dark solid was filtered off, washed with cold MeOH and Et2O, then dried to afford an orange solid A18, yield 74%. 1H NMR (300 MHz, DMSO-d6) δ 12.50 (s, 1H), 8.51-8.38 (m, 2H), 8.33 (d, J = 7.7 Hz, 1H), 8.29-8.23 (m, 1H), 8.13 (d, J = 8.3 Hz, 1H), 7.79 (t, J = 7.3 Hz, 1H), 7.69 (t, J = 7.5 Hz, 1H), 7.61-7.54 (m, 1H), 7.50 (d, J = 15.4 Hz, 1H), 7.38-7.30 (m, 2H), 4.27 (s, 3H); 13C NMR (75 MHz, DMSO-d6) δ 172.30, 144.64, 142.31, 138.31, 138.00, 129.27, 127.79, 127.03, 125.19, 124.36, 124.26, 122.89, 121.43, 116.34, 114.69, 113.55, 106.14, 36.16. ESI-MS (m/z):291.1[M-I]+.
General Preparation of Sample Before Measurements Assay
C-di-GMP, 10 mM Tris–HCl buffer solution (pH = 7.5), and salt solutions were mixed, heated to 95°C, and kept at 95°C for 5 min, and then slowly cooled back to rt. and kept at room temperature for 10 min. A18 was then added to the mixture and incubated in the refrigerator at −20°C overnight (about 12 h); unless otherwise stated, the reaction temperature is −20°C, the optimum temperature.
Measurement of Fluorescence
The fluorescence instrument settings were chosen as follows: λex = 485 nm (slit 6 nm), λem = 500–700 nm (slit 6 nm). The measurements were carried out at 15°C.
Measurement of Ultraviolet Spectra
The UV instrument settings were chosen as follows: wavelength = 400–600 nm, temperature was 15°C. The concentration of c-di-GMP was 20 μM, A18 was 10 μM, and buffer was 10 mM Tris–HCl (pH 7.5) containing 1 M salt (KCl, NaCl, or NH4Oac) or no salt was added.
Circular Dichroism Experiments
The concentration of c-di-GMP was 70 μM, [A18] or [TO] was 30 μM, and buffer was 10 mM Tris–HCl (pH 7.5) containing 250 mM KCl. The CD instrument settings were chosen as follows: Data pitch, 1 nm; scan speed, 50 nm/min; response, 8 s; bandwidth, 1 nm.
Preparation of Cell Lysate
PAO1-ΔwspF were grown up at 37°C in Luria-Bertani (LB) medium (50 ml) with static (biofilm state) or shaking (planktonic state) for 24 h, final OD (600 nm) was about 0.6, cells were pelleted by centrifugation and resuspended with 2 ml of 10 mM Tris–HCl (pH 7.5), and 200 μl of 50 mg/ml lysozyme was then added to each resuspension. The resuspension was sonicated (10/10 s, 30 min) to disrupt membranes and allow lysozyme to reach cell walls. The crude cell lysate was boiled for 10min. Each sample was centrifuged at 13,000g for 10min. The collected supernatant was then filtered by 0.2-μm filter and 3 kD exclusion columns.
Results and Discussion
Discovery of the New 3′,5′ Cyclic Diguanylic Acid G-Quadruplex Inducer A18
Inducing c-di-GMP to form G-quadruplex in the presence of monovalent cations and thus enhancing the fluorescence of the aromatic inducer is a promising method for the detection of cellular c-di-GMP. Because the reported inducer (TO) has low sensitivity and fluorescence intensity is closely related to the ability of a small molecule to induce the formation of a G-quadruplex, a new aromatic c-di-GMP G-quadruplex inducer (A18) that can be used as a more sensitive c-di-GMP fluorescent probe for rapid and efficient detection of cellular c-di-GMP in bacterial lysate samples was designed and synthesized in accordance with the structure of TO. Electron-rich S atoms are thought to be capable of interacting with the phosphate of c-di-GMP and enhancing the mutual interaction between the probe and the G-quadruplex. Thus, the benzothiazole moiety in TO with a positive charge was preserved as the basic nucleus in the newly developed probe. However, our experience in designing G-quadruplex ligands suggests that the introduction of groups capable of forming hydrogen bonds at the end of the molecule is extremely favorable for interaction. Accordingly, an indole group was introduced onto the benzothiazole ring to replace the quinoline part of TO. To expose the indole amino group, namely the hydrogen bond donor, the indole group was connected to the benzothiazole at the end of the molecule. Because aromaticity is a basic feature of an inducer, the benzothiazole and the indole were linked in a conjugated double bond to produce the new probe molecule A18 ((E)-2-(2-(1H-indol-3-yl) vinyl)-3-methyl-3λ4 -benzo [d] thiazole iodide). This molecule was obtained using the simple two-step reaction shown in Scheme 1.
Circular dichroism (CD) is a powerful tool with which to identify the aggregation state of c-di-GMP. When G-quadruplex is present in a solution, a positive CD peak occurs at approximately 300 nm and a negative CD peak occurs at approximately 262 nm (Zhang et al., 2006; Stelitano et al., 2013). The induction ability of synthesized A18 was first evaluated through CD, with TO serving as a positive control. As shown in Figure 1A, the A18/c-di-GMP complex exhibited similar CD features as the reported TO/c-di-GMP complex, indicating that A18 is also capable of inducing c-di-GMP to form G-quadruplex as TO.
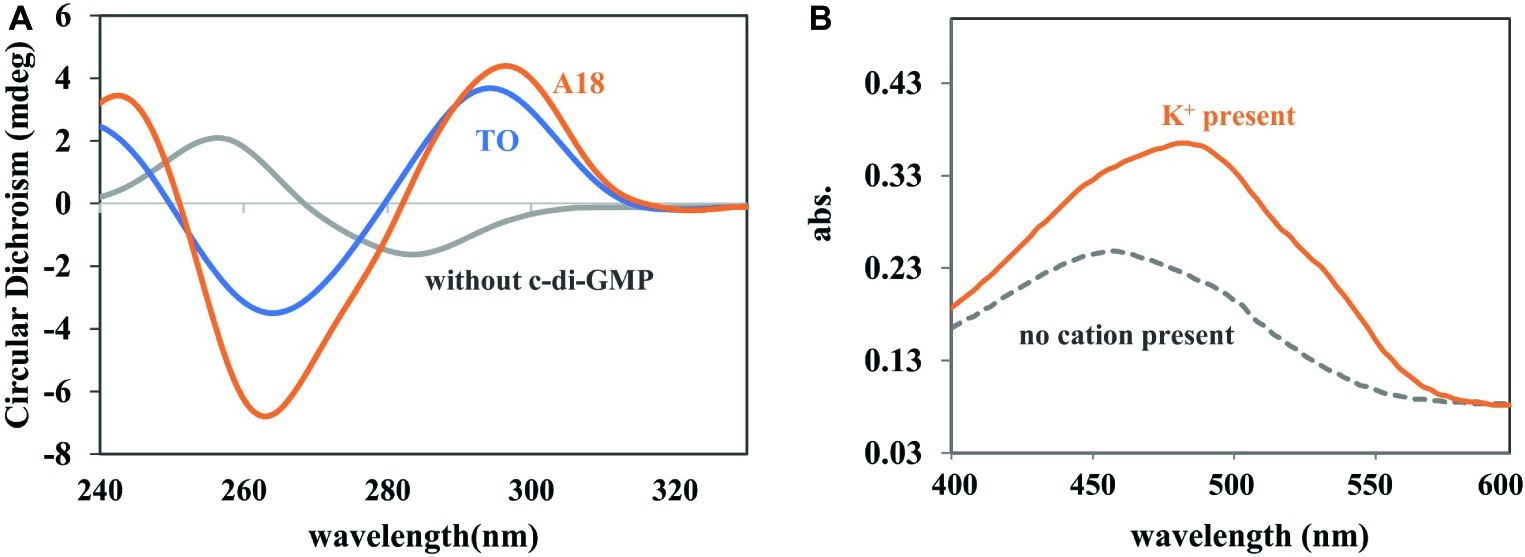
Figure 1. (A) CD spectra of c-di-GMP, c-di-GMP-A18, and c-di-GMP-TO complexes. [c-di-GMP] = 70 μM; [A18] or [TO] = 30 μM. Buffer: 10 mM Tris–HCl (pH 7.5) containing 250 mM KCl. Reaction temperature: 4°C. (B) UV spectra of A18 + c-di-GMP in different buffers. Addition of K+ (1 M) to the buffer (10 mM Tris–HCl, pH 7.5) caused a red shift in the absorption spectrum. [c-di-GMP] = 20 μM; [A18] = 10 μM. Reaction temperature: 4°C.
Fluorescence Enhancement Effect Upon Formation of an A18-G-Quadruplex Complex
The fluorescent molecule A18 can bind to G-quadruplex through π–π interactions in the form of intercalation or end-stacking (Lubitz et al., 2007; Seo et al., 2008; Dutta et al., 2018), and c-di-GMP has been demonstrated to form G-quadruplex in the presence of A18. We hypothesized that A18 is likely to intercalate between the two planes of the G-quadruplex formed by c-di-GMP, and because the rotation of A18 in G-quadruplex is restricted, the nonradiative channel in excited A18 would be closed, and the quantum yield (Φ) of the confined A18 would subsequently increase, leading to fluorescence enhancement of A18 (Figure 2). As expected, A18 has a low fluorescence quantum yield in an aqueous solution (Φ = 0.011), and Φ increases to 0.211 when A18 is in the confined cavities of c-di-GMP G-quadruplex complexes. This result was calculated as described in the Supporting Information using the data listed in Supplementary Table S1 (Chen et al., 2008; He et al., 2009). Further UV analysis indicated a π–π interaction between the G-quadruplex complex of c-di-GMP and A18, which is reflected by a red shift in the absorption spectrum when A18 induces c-di-GMP to form the G-quadruplex in the presence of K+ (Figure 1B; Jin et al., 2018).
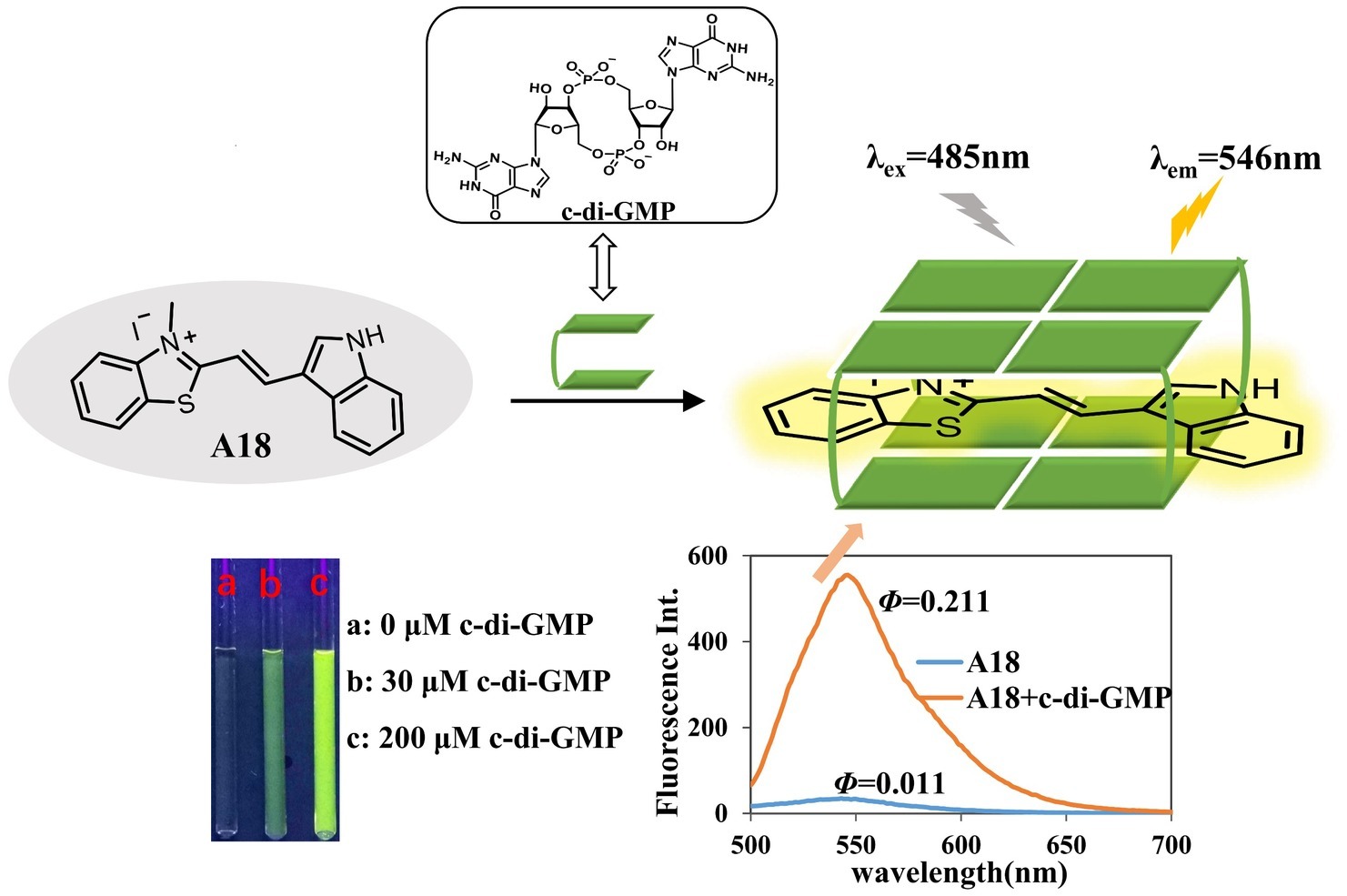
Figure 2. A18 was predicted to interact with c-di-GMP to yield an A18–G-quadruplex complex. We hypothesized that A18 would intercalate between the two planes of the G-quadruplex formed by c-di-GMP and that the fluorescence of A18 would be enhanced by the restriction of its rotation.
Optimal Ion Conditions for Detection
Because the process of fluorescence change requires the participation of the G-quadruplex, the formation of which depends on the existence of monovalent cations, the type and concentration of monovalent cations should have a large impact on fluorescence enhancement (Chen et al., 2008; Shim et al., 2009). As shown in Figure 3, the detection of c-di-GMP with A18 was more sensitive in the presence of K+ than in the presence of Na+ or NH4+, and Na+ did not seem to affect the fluorescence change. UV analysis yielded similar results. In the presence of both K+ and NH4+, the absorption spectra exhibited a significant red shift, indicative of a π–π interaction between the c-di-GMP G-quadruplex and A18, but no significant change in the absorption spectra was observed when Na+ was present (Supplementary Figure S4). K+ promotes more stable G-quadruplexes (Sen and Gilbert, 1990; Olsen et al., 2006). Jones et al. demonstrated that K+ and NH4+ favor the formation of one or more guanine quartet complexes, whereas Na+ favors a bimolecular self-intercalated structure, which is consistent with our results (Zhang et al., 2006).
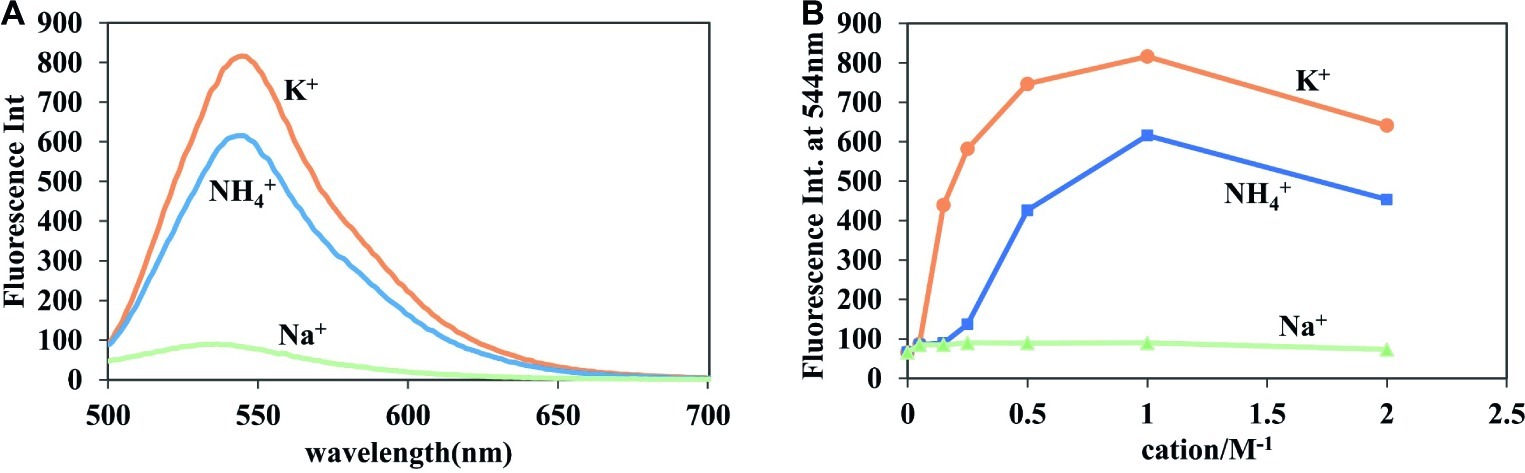
Figure 3. Effect of different ions on the fluorescent properties of A18. (A) Fluorescence of the A18-c-di-GMP complex in the presence of 1 M NaCl, KCl, and NH4OAc. (B) Fluorescence of the A18-c-di-GMP complex in the presence of different salt concentrations (0 mM, 50 mM, 150 mM, 250 mM, 500 mM, 1 M, and 2 M). [c-di-GMP] = 20 μM; [A18] = 10 μM. Ex. 485 nm, em. 500–700 nm. Buffer: 10 mM Tris–HCl (pH 7.5) containing different salts.
Dose Response of Fluorescence on A18 and 3′,5′ Cyclic Diguanylic Acid Concentration
To optimize the assay conditions and investigate the detection limit of this method, the fluorescent properties of A18 with different concentrations of c-di-GMP and A18 were investigated using fluorescence titration. As shown in Figures 4, 5, when the concentration of c-di-GMP or A18 increased, the fluorescence intensity was gradually enhanced. Nevertheless, obvious fluorescence enhancement was observed when the concentration of A18 was as low as 1 μM. This is significantly stronger than the fluorescence produced by TO at 30 μM under the same conditions, and when the concentration of A18 was increased to 10 μM, the fluorescence intensity was almost seven times stronger than that of 30 μM TO (Figure 4). These results reflect a significantly enhanced sensitivity of A18 over TO. When the concentration of A18 was >10 μM, the fluorescence intensity no longer increased (Figure 4). This indicated that A18 has a strong ability to induce c-di-GMP aggregation and that a 10 μM concentration of A18 may result in complete induction.
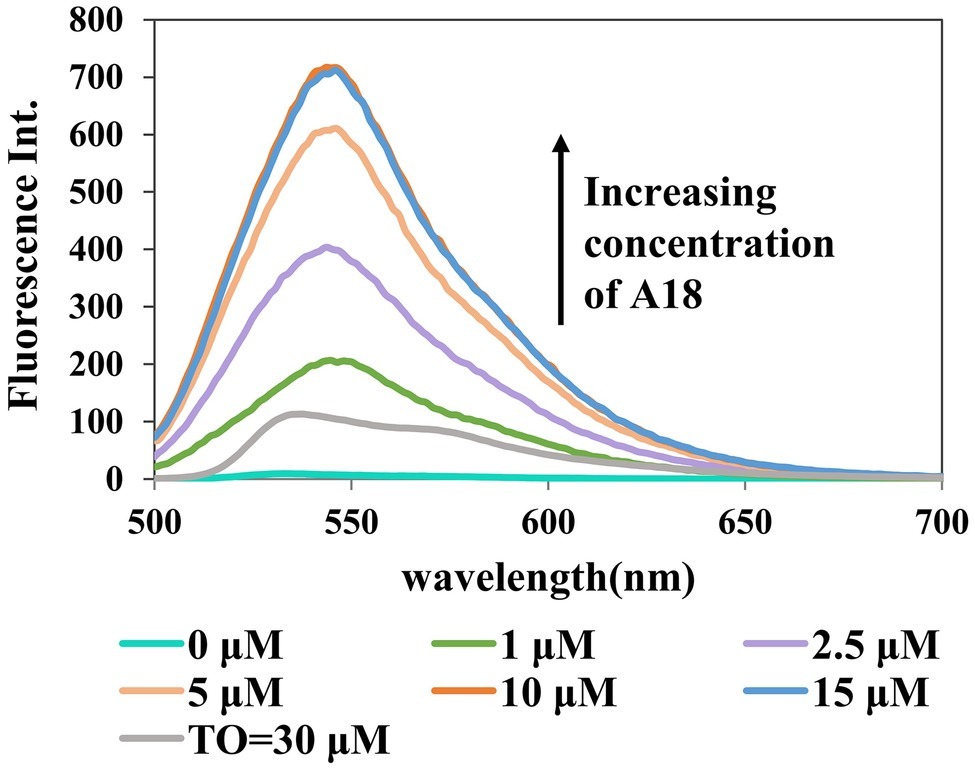
Figure 4. Dose response for the A18–c-di-GMP interaction. [c-di-GMP] = 20 μM. Buffer: 10 mM Tris–HCl (pH 7.5) containing 500 mM KCl. Ex. 485 nm, em. 500–700 nm.
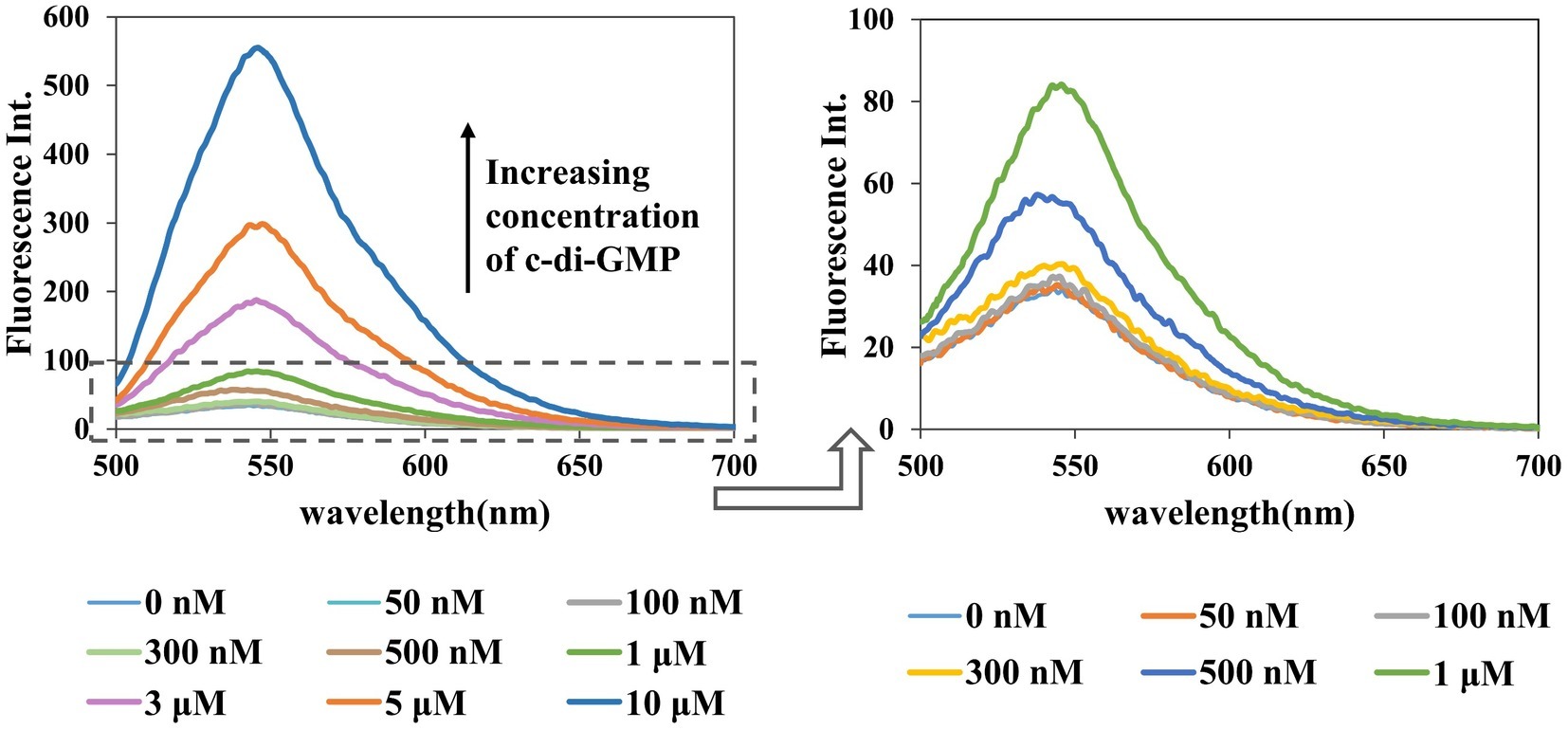
Figure 5. Dose response for the A18–c-di-GMP interaction. [A18] = 2.5 μM, buffer: 10 mM Tris–HCl (pH 7.5) containing 1 M KCl. Ex. 485 nm, em. 500–700 nm.
Similarly, in the presence of an increasing concentration of c-di-GMP, fluorescence intensity also increased gradually, and the detection limit for this assay was as low as 500 nM (Figure 5). Among the detection methods reported to date, using an assay to detect c-di-GMP in vitro with small molecules is the simplest method, but its general application is limited by its low sensitivity (Nakayama et al., 2011a,b). Furthermore, c-di-GMP, with a cellular concentration range of 0–10 μM, plays a central role in several processes that control bacterial phenotypes (Simm et al., 2009; Ho et al., 2013), and nanomolar fluorescence detection is critical to deciphering the molecular details of c-di-GMP signaling. Our research represents an advance in the detection of c-di-GMP with small molecules.
Selective Detection of 3′,5′ Cyclic Diguanylic Acid by A18
Having established the superiority of A18 in the detection of c-di-GMP, we proceeded to investigate the selectivity of the method. The concentration of several other nucleotides such as cGMP, dGTP, and ATP is significantly higher than that of c-di-GMP in bacterial cells (Wang and Sintim, 2019). Therefore, it should be ascertained that the detection method can specifically detect c-di-GMP without interference from other nucleotides. It was found that c-di-GMP or a mixture containing c-di-GMP and other nucleotides (GMP, cGMP, dNTP, and rNTP) can be detected by A18, but a mixture of only the other nucleotides could not be detected with A18. The fluorescent signal of a sample that contained several other nucleotides and c-di-GMP was similar to that containing only c-di-GMP (Figure 6A). This illustrates the specificity of A18 for fluorescence detection of c-di-GMP.
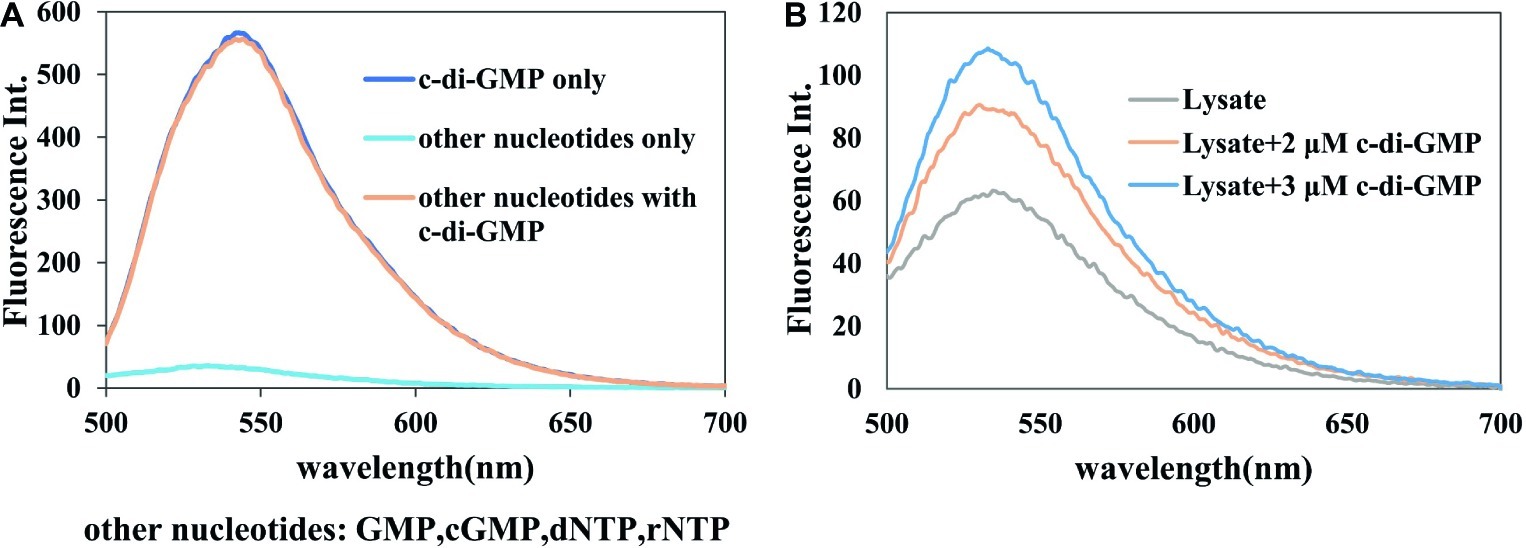
Figure 6. Selectivity of the detection system. Buffer: 10 mM Tris–HCl (pH7.5) containing 1 M KCl. Ex. 485 nm. em. 500–700 nm. (A) [c-di-GMP] = 10 μM, each [nucleotide] = 10 μM, [A18] = 2.5 μM. (B) Lysate + c-di-GMP, [A18] = 2.5 μM.
To further verify the specificity of this assay and prove the application of this assay in detection in bacterial samples, the specificity of the assay was also verified in crude bacterial lysate. As shown in Figure 6B, it can be seen that the selective detection of c-di-GMP proceeded well on crude cell lysate, without any prior separation step. After the addition of a standard sample of c-di-GMP, the magnitude of fluorescence enhancement was almost identical to that in the in vitro experiment, and it was apparently not affected by other components in the lysate. This also showed that our method could distinguish different concentrations of c-di-GMP in bacterial lysates, and indicates the feasibility of detection of different bacterial samples using this probe.
Applied Research on the Detection of 3′,5′ Cyclic Diguanylic Acid in Bacterial Crude Cell Lysates
The goal in this research was to develop a sensitive in vitro assay to quickly and easily determine the cellular levels of c-di-GMP in various bacterial samples without relying on complex steps to prepare probes or culturing large volumes of bacterial samples. The c-di-GMP detection assay using A18 was performed on crude cell lysates with as few 50-ml bacterial samples as possible. For TO, 3 L of lysate was necessary. Compared with the bacteria in the planktonic state, bacteria in the static state that contributed to the formation of biofilm had a higher level of c-di-GMP (Barraud et al., 2009). Accordingly, we determined the c-di-GMP levels of PAO1-Δwspf (a mutant PAO1 with high expression of c-di-GMP; the construction method was as reported in Hickman et al., 2005) in planktonic and biofilm states, which have different levels of cellular c-di-GMP, by using our fluorescent probe A18. HPLC was also used to verify the identity of c-di-GMP and quantify the cellular c-di-GMP concentrations of the same sample (Supplementary Figure S5). We found that the peak corresponding to the c-di-GMP molecule was not obvious in the HPLC spectrum (Supplementary Figure S5), and it was difficult to quantify the c-di-GMP level even in the static state, which has high c-di-GMP expression. This may have been caused by the low level of c-di-GMP derived from the 50-ml sample and the interference of other metabolite peaks. The sample amount required for HPLC detection is generally 500 ml. Our method could distinguish well between the different c-di-GMP levels in the two culture states (Figure 7). We can infer that compared with the c-di-GMP standard addition HPLC spectrum (Supplementary Figure S5), the c-di-GMP content in the lysate was less than 1 μmol, indicating that our method can readily distinguish between the two culture states even at low micromolar concentrations. The detection method described here sensitively reflects the c-di-GMP level through fluorescence in bacterial samples, even at low micromolar concentrations, whereas HPLC and other methods are unable to detect low concentrations in vitro because of low sensitivity or interference from other metabolites.
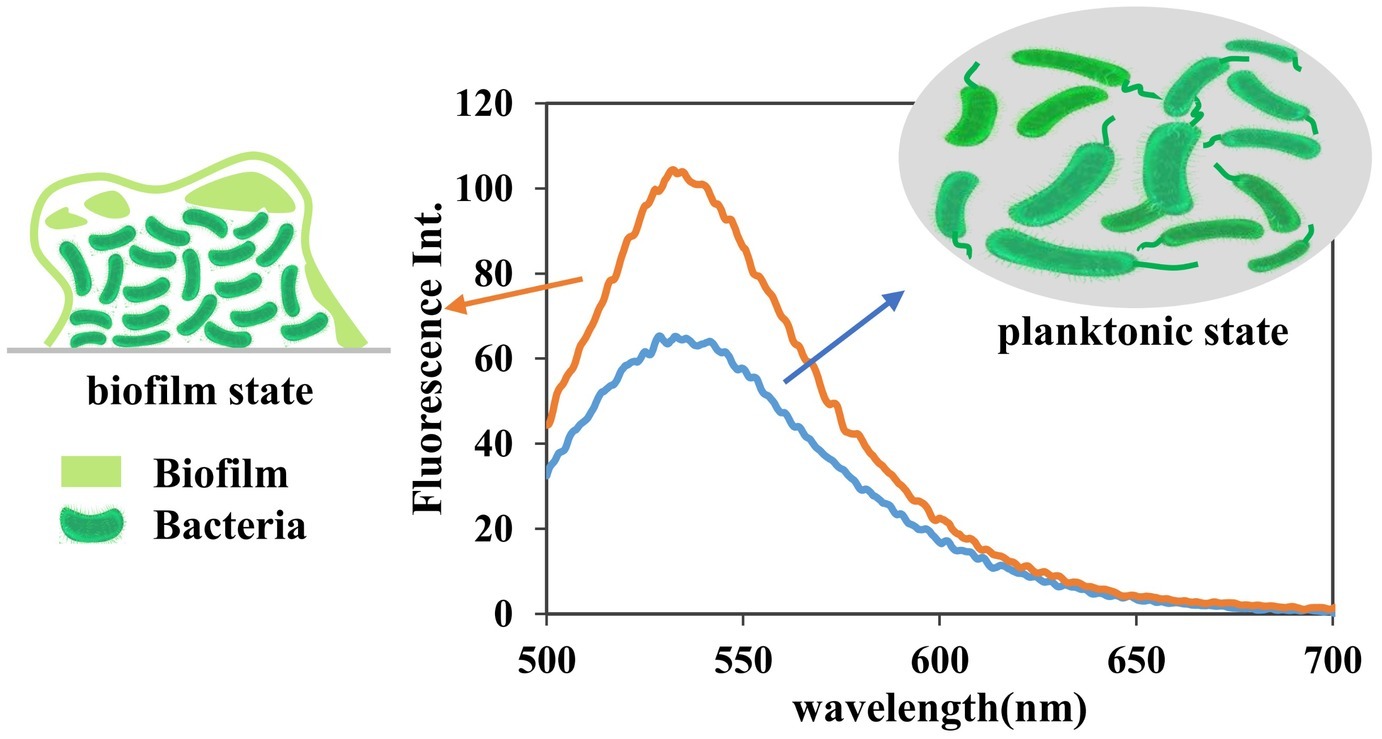
Figure 7. Fluorescence detection of c-di-GMP in PAO1-Δwspf lysate. Detection conditions: [A18] = 2.5 μM, buffer, 10 mM Tris–HCl (pH 7.5) containing 1 M KCl. In line with expectations, our method can reliably distinguish the differences between the two culture states even at low micromolar concentrations. Samples: 50 ml of bacterial crude lysate was concentrated to 2 ml.
Conclusions
In summary, a new fluorescent molecule A18 was developed for c-di-GMP detection using the property of aromatic molecule-induced G-quadruplex formation of c-di-GMP. This method is capable of detecting c-di-GMP at concentrations as low as 500 nM in a simple buffer system and has good selectivity to c-di-GMP over other small nucleotides. The method can be used for the facile and rapid detection of cellular c-di-GMP levels in bacterial lysate samples that can be obtained using a simple cell lysis and filtration process without HPLC purification. This method will contribute to the quick evaluation of the efficacy of drugs that inhibit c-di-GMP signaling as well as other explorations of this signaling.
Data Availability Statement
All datasets generated for this study are included in the article/Supplementary Material.
Author Contributions
T-FX, JLin, and W-MC conceived the research idea and designed the experiments. T-FX performed the experiments and wrote the manuscript. T-FX, Z-QW, JLiu, JLin, and W-MC participated in the analysis of the study and helped to revise the manuscript. All authors read and approved the final manuscript.
Funding
This work was supported by the Pearl River S&T Nova Program of Guangzhou (201806010116), the Natural Science Foundation of Guangdong Province (2019A1515011801), and the Fundamental Research Funds for the Central University (21617478).
Conflict of Interest
The authors declare that the research was conducted in the absence of any commercial or financial relationships that could be construed as a potential conflict of interest.
Acknowledgments
The authors sincerely thank Dr. Liang Yang from the Singapore Centre for Environmental Life Sciences Engineering, Nanyang Technological University for gifting the PAO1-Δwspf strain.
Supplementary Material
The Supplementary Material for this article can be found online at: https://www.frontiersin.org/articles/10.3389/fmicb.2019.03163/full#supplementary-material
References
Alina, N., Caroline, W., and Rowe-Magnus, D. A. (2008). Cyclic-di-GMP regulates extracellular polysaccharide production, biofilm formation, and rugose colony development by vibrio vulnificus. Appl. Environ. Microbiol. 74, 4199–4209. doi: 10.1128/AEM.00176-08
Barraud, N., Schleheck, D., Klebensberger, J., Webb, J. S., Hassett, D. J., Rice, S. A., et al. (2009). Nitric oxide signaling in Pseudomonas aeruginosa biofilms mediates phosphodiesterase activity, decreased cyclic Di-GMP levels, and enhanced dispersal. J. Bacteriol. 191, 7333–7342. doi: 10.1128/JB.00975-09
Chen, S. Y., Tang, C. Y., Chuang, W. T., Lee, J. J., Tsai, Y. L., Chan, J. C. C., et al. (2008). A facile route to synthesizing functionalized mesoporous SBA-15 materials with platelet morphology and short mesochannels. Chem. Mater. 20, 3906–3916. doi: 10.1021/cm703500c
Dippel, A. B., Anderson, W. A., Evans, R. S., Deutsch, S., and Hammond, M. C. (2018). Chemiluminescent biosensors for detection of second messenger cyclic di-GMP. ACS Chem. Biol. 13, 1872–1879. doi: 10.1021/acschembio.7b01019
Dutta, D., Debnath, M., Diana, M., Paul, R., and Dash, J. (2018). Cell penetrating thiazole peptides inhibit c-MYC expression via site-specific targeting of c-MYC G-quadruplex. Nucleic Acids Res. 46, 5355–5365. doi: 10.1093/nar/gky385
Hall, C. L., and Lee, V. T. (2017). Cyclic-di-GMP regulation of virulence in bacterial pathogens. Wiley Interdiscip. Rev. RNA. 9:e1454. doi: 10.1002/wrna.1454
He, Q., Shi, J., Cui, X., Zhao, J., Chen, Y., and Zhou, J. (2009). Rhodamine B-co-condensed spherical SBA-15 nanoparticles: facile co-condensation synthesis and excellent fluorescence features. J. Mater. Chem. 19, 3395–3405. doi: 10.1039/b900357f
Hengge, R. (2009). Principles of c-di-GMP signalling in bacteria. Nat. Rev. Microbiol. 7, 263–273. doi: 10.1038/nrmicro2109
Hickman, J. W., Tifrea, D. F., and Harwood, C. S. (2005). A chemosensory system that regulates biofilm formation through modulation of cyclic diguanylate levels. Proc. Natl. Acad. Sci. USA 102, 14422–14427. doi: 10.1073/pnas.0507170102
Ho, C. L., Chong, K. S. J., Oppong, J. A., Chuah, M. L. C., Tan, S. M., and Liang, Z. X. (2013). Visualizing the perturbation of cellular cyclic di-GMP levels in bacterial cells. J. Am. Chem. Soc. 135, 566–569. doi: 10.1021/ja310497x
Jenal, U., Reinders, A., and Lori, C. (2017). Cyclic di-GMP: second messenger extraordinaire. Nat. Rev. Microbiol. 15, 271–284. doi: 10.1038/nrmicro.2016.190
Jin, M., Liu, X. J., Zhang, X., Wang, L. L., Bing, T., Zhang, N., et al. (2018). Thiazole orange modified carbon dots for ratiometric fluorescence detection of G-quadruplex and double-stranded DNA. ACS Appl. Mater. Interfaces 10, 25166–25173. doi: 10.1021/acsami.8b07869
Kellenberger, C. A., Sales-Lee, J., Pan, Y., Gassaway, M. M., Herr, A. E., and Hammond, M. C. (2015). A minimalist biosensor: quantitation of cyclic di-GMP using the conformational change of a riboswitch aptamer. RNA Biol. 12, 1189–1197. doi: 10.1080/15476286.2015.1062970
Kelsey, I., Nakayama, S., and Sintim, H. O. (2012). Diamidinium and iminium aromatics as new aggregators of the bacterial signaling molecule, c-di-GMP. Bioorg. Med. Chem. Lett. 22, 881–885. doi: 10.1016/j.bmcl.2011.12.039
Lubitz, I., Borovok, N., and Kotlyar, A. (2007). Interaction of monomolecular G4-DNA nanowires with TMPyP: evidence for intercalation. Biochemistry 46, 12925–12929. doi: 10.1021/bi701301u
Nakayama, S., Kelsey, I., Wang, J., Roelofs, K., and Sintim, H. O. (2011a). Thiazole orange-induced c-di-GMP quadruplex formation facilitates a simple fluorescent detection of this ubiquitous biofilm regulating molecule. J. Am. Chem. Soc. 133, 4856–4864. doi: 10.1021/ja1091062
Nakayama, S., Kelsey, I., Wang, J., and Sintim, H. O. (2011b). C-di-GMP can form remarkably stable G-quadruplexes at physiological conditions in the presence of some planar intercalators. Chem. Commun. 47, 4766–4768. doi: 10.1039/c0cc05432a
Nakayama, S., Luo, Y. L., Zhou, J., Dayie, T. K., and Sintim, H. O. (2012). Nanomolar fluorescent detection of c-di-GMP using a modular aptamer strategy. Chem. Commun. 48, 9059–9061. doi: 10.1039/c2cc34379g
Olsen, C. M., Gmeiner, W. H., and Marky, L. A. (2006). Unfolding of G-Quadruplexes: energetic, and ion and water contributions of G-quartet stacking. J. Phys. Chem. B 110, 6962–6969. doi: 10.1021/jp0574697
Rabin, N., Zheng, Y., Opoku-Temeng, C., Du, Y., Bonsu, E., and Sintim, H. O. (2015). Biofilm formation mechanisms and targets for developing antibiofilm agents. Future Med. Chem. 7, 493–512. doi: 10.4155/fmc.15.6
Ross, P., Weinhouse, H., Aloni, Y., Michaeli, D., Weinberger-Ohana, P., Mayer, R., et al. (1987). Regulation of cellulose synthesis in acetobacter xylinum by cyclic diguanylic acid. Nature 325, 279–281. doi: 10.1038/325279a0
Rybtke, M. T., Borlee, B. R., Murakami, K., Irie, Y., Hentzer, M., Nielsen, T. E., et al. (2012). Fluorescence-based reporter for gauging cyclic di-GMP levels in Pseudomonas aeruginosa. Appl. Environ. Microbiol. 78, 5060–5069. doi: 10.1128/AEM.00414-12
Sen, D., and Gilbert, W. (1990). A sodium-potassium switch in the formation of four-stranded G4-DNA. Nature 344, 410–414. doi: 10.1038/344410a0
Seo, Y. J., Lee, I. J., and Kim, B. H. (2008). Detection of structure-switching in G-quadruplexes using end-stacking ability. Bioorg. Med. Chem. Lett. 18, 3910–3913. doi: 10.1016/j.bmcl.2008.06.033
Shim, J. W., Tan, Q., and Gu, L. Q. (2009). Single-molecule detection of folding and unfolding of the g-quadruplex aptamer in a nanopore nanocavity. Nucleic Acids Res. 37, 972–982. doi: 10.1093/nar/gkn968
Simm, R., Morr, M., Remminghorst, U., Andersson, M., and Romling, U. (2009). Quantitative determination of cyclic diguanosine monophosphate concentrations in nucleotide extracts of bacteria by matrix-assisted laser desorption/ionization-time-of-flight mass spectrometry. Anal. Biochem. 386, 53–58. doi: 10.1016/j.ab.2008.12.013
Spangler, C., Bohm, A., Jenal, U., Seifert, R., and Kaever, V. (2010). A liquid chromatography-coupled tandem mass spectrometry method for quantitation of cyclic di-guanosine monophosphate. J. Microbiol. Methods 81, 226–231. doi: 10.1016/j.mimet.2010.03.020
Stelitano, V., Brandt, A., Fernicola, S., Franceschini, S., Giardina, G., Pica, A., et al. (2013). Probing the activity of diguanylate cyclases and c-di-GMP phosphodiesterases in real-time by CD spectroscopy. Nucleic Acids Res. 41:e79. doi: 10.1093/nar/gkt028
Tsiry, R., Quentin, L., Pierre, D., and Mondher, E. J. (2015). The formation of biofilms by Pseudomonas aeruginosa: a review of the natural and synthetic compounds interfering with control mechanisms. Biomed. Res. Int. 2015, 1–17. doi: 10.1155/2015/759348
Tsuji, G., and Sintim, H. O. (2016). Cyclic dinucleotide detection with riboswitch-G-quadruplex hybrid. Mol. BioSyst. 12, 773–777. doi: 10.1039/c5mb00751h
Ueda, A., and Wood, T. K. (2010). Tyrosine phosphatase TpbA of Pseudomonas aeruginosa controls extracellular DNA via cyclic diguanylic acid concentrations. Environ. Microbiol. Rep. 2, 449–455. doi: 10.1111/j.1758-2229.2010.00171.x
Wang, M., and Sintim, H. O. (2019). Discriminating cyclic from linear nucleotides - CRISPR/Cas-related cyclic hexaadenosine monophosphate as a case study. Anal. Biochem. 567, 21–26. doi: 10.1016/j.ab.2018.11.022
Wang, X. C., Wilson, S. C., and Hammond, M. C. (2016). Next-generation RNA-based fluorescent biosensors enable anaerobic detection of cyclic di-GMP. Nucleic Acids Res. 44:e139. doi: 10.1093/nar/gkw580
Yeo, J., Dippel, A. B., Wang, X. C., and Hammond, M. C. (2018). In vivo biochemistry: single-cell dynamics of cyclic Di-GMP in Escherichia coli in response to zinc overload. Biochemistry 57, 108–116. doi: 10.1021/acs.biochem.7b00696
Yeo, J., Wang, X. C., and Hammond, M. C. (2017). Live flow cytometry analysis of c-di-GMP levels in single cell populations. Methods Mol. Biol. 1657, 111–130. doi: 10.1007/978-1-4939-7240-1_10
Keywords: c-di-GMP, fluorescent, detection, quadruplex, biofilm
Citation: Xuan T-F, Liu J, Wang Z-Q, Chen W-M and Lin J (2020) Fluorescent Detection of the Ubiquitous Bacterial Messenger 3′,5′ Cyclic Diguanylic Acid by Using a Small Aromatic Molecule. Front. Microbiol. 10:3163. doi: 10.3389/fmicb.2019.03163
Edited by:
Carlos Alberto Moreira-Filho, University of São Paulo, BrazilReviewed by:
Michael Y. Galperin, National Center for Biotechnology Information (NLM), United StatesHerman Sintim, Purdue University, United States
Copyright © 2020 Xuan, Liu, Wang, Chen and Lin. This is an open-access article distributed under the terms of the Creative Commons Attribution License (CC BY). The use, distribution or reproduction in other forums is permitted, provided the original author(s) and the copyright owner(s) are credited and that the original publication in this journal is cited, in accordance with accepted academic practice. No use, distribution or reproduction is permitted which does not comply with these terms.
*Correspondence: Wei-Min Chen, dHdtY2hlbkBqbnUuZWR1LmNu; Jing Lin, bGluamluZ19qbnVAMTYzLmNvbQ==