- 1AgroSup Dijon, PAM UMR A 02.102, Université Bourgogne Franche-Comté, Dijon, France
- 2AgroSup Dijon, Service d’Appui à la Recherche, Dijon, France
- 3Synchrotron SOLEIL, Saint-Aubin, France
Bacterial spores are extremely resistant life-forms that play an important role in food spoilage and foodborne disease. The return of spores to a vegetative cell state is a three-step process, these being activation, germination, and emergence. High-pressure (HP) processing is known to induce germination in part of the spore population and even to inactivate a high number of Bacillus spores when combined with other mild treatments such as the addition of nisin. The aim of the present work was to investigate the mechanisms involved in the sensitization of spores to nisin following HP treatment at ambient temperature or with moderate heating leading to a heterogeneous spore response. Bacillus subtilis spores were subjected to HP treatment at 500 MPa at 20 and 50°C. The physiological state of different subpopulations was characterized. Then Fourier transform infrared (FTIR) microspectroscopy coupled to a synchrotron infrared source was used to explore the heterogeneity of the biochemical signatures of the spores after the same HP treatments. Our results confirm that HP at 50°C induces the germination of a large proportion of the spore population. HP treatment at 20°C generated a subpopulation of ungerminated spores reversibly sensitized to the presence of nisin in their growth medium. Regarding infrared spectra of individual spores, spores treated by HP at 50°C and germinated spores had similar spectral signatures involving the same structural properties. However, after HP was performed at 20°C, two groups of spores were distinguished; one of these groups was clearly identified as germinated spores. The second group displayed a unique spectral signature, with shifts in the spectral bands corresponding to changes in membrane fluidity. Besides, spores spectra in the amide region could be divided into several groups close to spectral properties of dormant, germinated, or inactivated spores. The part of the spectra corresponding to α-helix and β-sheet-structures contribute mainly to the spectral variation between spores treated by HP at 20°C and other populations. These changes in the lipid and amide regions could be the signature of reversible changes linked to spore activation.
Introduction
High pressure (HP) is the most widely used non-thermal method of processing to stabilize commercial foods. By reducing the microbial load, this process extends the product shelf-life while meeting consumer demand for freshly prepared foods with high sensory and nutritional qualities (Huang et al., 2017). Industrial HP machines are currently designed to treat foods at a pressure of up to 700 MPa at ambient or refrigerated temperatures, thus ensuring complete inactivation of foodborne pathogens, spoilage microorganisms, and deteriorative enzymes (Metrick et al., 1989; Patterson et al., 1995). However, the persistence of bacterial spores is the Achilles heel of this process because spores of Clostridium botulinum, Bacillus cereus, and other pathogenic and alteration species easily resist HP treatments and can then readily return to active growth (Nguyen Thi Minh et al., 2010).
When subjected to an HP process with moderate heating at about 50°C, two different processes induce the germination of Bacillus sp. bacterial spores depending on the pressure applied. Moderate HP (<400 MPa) induces spore germination by triggering the germinant receptors (GRs) located in the inner spore membrane, whereas HP above 500 MPa induces GR-independent germination by triggering the release of dipicolinic acid (DPA) from the spore core (Paidhungat et al., 2002; Doona et al., 2014; Setlow, 2014). Spores lose their inherent resistance once germinated and can then be inactivated by other inactivation processes (Black et al., 2005; Nguyen Thi Minh et al., 2010). HP has been shown to act synergistically with nisin to inactivate various species of spore-forming bacteria (Black et al., 2008; Gao et al., 2011; Aouadhi et al., 2013). Furthermore, the generally accepted hypothesis is that HP-germinated spores are sensitive to nisin and thus inactivated by the latter. This has not, however, been clearly demonstrated. Indeed, spore subpopulations respond differently to mild stress such as HP. Some spores may begin the germination process while others remain dormant, rendering the exact mechanism of the synergy difficult to evidence.
The aim of the present work was to investigate the mechanisms involved in the sensitization of spores to further stresses such as the addition of nisin following HP treatment at ambient temperature or with moderate heating leading to a heterogeneous spore response. For this purpose, we characterized the physiological state of Bacillus subtilis spore subpopulations after HP treatment at 500 MPa at 20 and 50°C. Then we used Fourier transform infrared (FTIR) microspectroscopy coupled to a synchrotron light as a source of infrared (IR) photons to explore the heterogeneity of the biochemical signatures of the spores after the same HP treatments. FTIR spectroscopy is a powerful analytical method to characterize cell metabolism and biochemical properties of biomolecules such as lipids, proteins, and nucleic acids. Some authors have already used this method with spores, which has provided new knowledge about species-related biochemical compositions and the effect of HP processes on the structural properties of spores (Valentine et al., 2006; Georget et al., 2014). However, these studies were conducted on bulk samples, providing a single average spectrum characterizing millions of spores. In this study, we used synchrotron attenuated total reflectance (ATR)–FTIR microspectroscopy to measure the spectra of individual spores and assess the heterogeneity of their response to the HP treatment.
Materials and Methods
Bacterial Strains, Growth, and Sporulation Conditions
Bacillus subtilis PS533 and the mutant strain B. subtilis PS4150 (provided by the Department of Molecular, Microbial, and Structural Biology, University of Connecticut Health Center, United States) were used in this study. Strain PS4150 has a defective spore coat due to the deletion of most of the cotE and gerE coding sequences (Ghosh et al., 2008).
For sporulation, cultures were obtained by inoculating two or three fresh colonies in 50 ml of complex medium (CM): 10 g/L of meat extract (Biokar, France), 2 g/L of yeast extract (Biokar, France), and 0.04 g/L of MnSO4 (Sigma-Aldrich, France) (André et al., 2013). After incubation for about 24 h (until 0.4 < OD600 < 0.6), 0.4 ml of the culture was spread on CM agar (CMA) in Petri dishes (CM supplemented with 15 g/L of agar, Biokar, France). Sporulation was monitored using phase contrast microscopy. When more than 95% of phase-bright spores were observed, spores were harvested by flooding the agar plates with cold sterile distilled water and scraping the agar surface with a sterile cell spreader. Spore suspensions were then centrifuged (3,600 g, 15 min, 4°C), and the spores were suspended in 0.2 μm of filtrated 70% ethanol (filter: cellulose acetate, Sartorius, France; ethanol: Elvetec, France) for 1 h to inactivate vegetative cells (Koransky et al., 1978). Spores were then washed three times by successive centrifugation (3,600 g, 15 min, 4°C) and suspension in sterile distilled water at 4°C. Spores were enumerated [in colony-forming unit (CFU)/ml] on Luria broth (LB) medium (Sigma-Aldrich, France). Spore suspensions were standardized to obtain a final concentration of 108 CFU/ml and stored at 4°C. For the synchrotron FTIR microspectroscopy experiments, the final spore suspensions were standardized to obtain a final concentration of 109 CFU/ml, frozen overnight at −80°C, and freeze-dried (FreeZone 18, Labconco, Kansas City, United States). Spores were stored at 4°C for a maximum of 1 month until use.
HP Treatment and Control Samples
High-pressure tests were performed in a Top Industrie 700-MPa vessel (France) with a 7-cm3 metal pressure chamber (working temperature: −120°C/120°C). Water was used as the pressure-transmission fluid. The pressure chamber was immersed in a water bath (Minisat 240, Huber, Germany) to control the internal temperature and to ensure that adiabatic heating during compression did not increase the temperature by more than 5°C above the desired set point. The compression rate was set to 3 MPa/s, and decompression was almost instantaneous (<3 s).
Effect of HP on Inactivation, Germination, and Nisin Sensitivity of B. subtilis Spores
The initial spore suspensions were diluted (1:100) in 0.11 mol/L of 2-(N-morpholino)ethane sulfonic acid (MES) buffer at pH 6.1 (Sigma-Aldrich, France) to reach a final concentration of approximately 107 CFU/ml. MES buffer was chosen because its pH varies only slightly with temperature and pressure (ΔpKa/°C = −0.011; pH varies from 5.5 to 6.5 between 10 and 1,000 MPa at 25°C) (Bruins et al., 2007). Then, 0.5 ml of each spore suspension in MES was heat-sealed in polyethylene pouches (polyethylene transfer pipette, Dominique Dutscher). For each strain, zero-time samples were taken to determine the initial spore concentration before treatment. The pouches were placed in the HP vessel 5 min before treatment to allow the temperature to equilibrate. Samples were treated at 500 MPa for 10 min at 20°C or 50°C and then immediately immersed in iced water.
Immediately after treatment, the samples were divided into three equal volumes. The first volume was serially diluted and plated on an LB medium (15 g/L, Sigma-Aldrich, France; bacteriological agar type E, 15 g/L, Biokar, Beauvais, France) to determine bacterial cell numbers in colony-forming units per milliliter. The second volume was treated at 80°C for 10 min in a water bath to inactivate germinated spores and then serially diluted and plated on LB Petri dishes for enumeration. The fraction of HP-induced germinated spores was calculated as the difference between the logarithmic counts of pressure- and heat-treated (NHPT) and pressure-treated (NHP) portions of the samples [log10(NHPT) - log10(NHP)].
The third volume was plated on an LB medium supplemented with 50 IU/ml of nisin (Sigma-Aldrich, France) to assess the sensitization of spores to nisin. To evaluate the effect of nisin alone, control samples of non-HP-treated spores were plated on an LB medium supplemented with nisin. Furthermore, to assess the reversibility of nisin sensitization after HP treatment, spore suspensions were stored for 2, 4, 6, or 24 h after HP treatment before being plated on an LB medium supplemented with nisin for enumeration (in CFU/ml). As controls, HP-treated spores were stored at 4°C for 2, 4, or 6 h and plated on an LB medium without nisin.
Synchrotron FTIR Microspectroscopy of Bacterial Spores
Sample Preparation
The lyophilized spore stock was suspended in MES buffer to obtain a final concentration of approximately 107 CFU/ml and treated at 500 MPa for 10 min at 20°C or 50°C, as explained above. After treatment, the samples were centrifuged at 11,200 g for 5 min at 4°C, washed twice in deionized water to remove the MES buffer, and suspended in distilled water.
As a control, germinated spores were obtained by suspending lyophilized spores in an LB medium (15 g/l, Sigma-Aldrich, France) at a final concentration of 107 CFU/ml. Spore samples in LB were incubated for 45 min at 37°C and then washed twice by successive centrifugation (11,200 g, 5 min, 4°C) and suspension in phosphate buffer saline (PBS).
To obtain inactivated spores, lyophilized spores were suspended in sterile distilled water at a final concentration of 107 CFU/ml and treated in a dry bath (BSH1001-E, Benchmark Scientific, Edison, NJ, United States) at 121°C for 30 min. Inactivated spore samples were then centrifuged (11,200 g, 5 min, 4°C) and suspended in sterile distilled water.
All the samples were stored at 4°C for a maximum of 30 min until analysis.
Sample Analysis Using Synchrotron FTIR Microspectroscopy
Synchrotron IR microscopy was performed on the SMIS beamline at the SOLEIL Synchrotron Facility (session 20160780, Saint Aubin, France), as previously described by Passot et al. (2015). Briefly, spectra were recorded on a continuum microscope (Thermo Fisher Scientific, Villebon-sur-Yvette, France) coupled to a Nicolet 5700 FTIR spectrometer (Thermo Fisher Scientific). The microscope includes a motorized sample stage and a liquid nitrogen-cooled mercury cadmium telluride (MCT-A) detector. It operates in confocal mode with a dual-path single aperture and uses an x32 infinity-corrected Schwarzschild-type objective and a matching x32 condenser. Individual spectra were obtained at a spectral resolution of 4 cm–1, co-adding 256 scans over the mid-IR spectral range from 4,000 to 400 cm–1.
Two microliters of the HP-treated spore suspension was deposited on the base of an IR transparent ZnSe ATR crystal with a diameter of 4 mm hemispherical (refractive index: n = 2.4; ISP Optics Corp., Latvia) (Passot et al., 2015). The sample drop was dried at room temperature for 5 min under a gentle airflow. The hemisphere was then placed under the microscope, its base on a glass slide. Single bacterial spores were located using the visible objective. For each sample treated by HP, 50 spores were selected, and their IR spectra were recorded. For control samples, 25 spectra were recorded. Moreover, three spore-free zones were located and selected for the background IR signal.
The ATR mode was chosen to improve the spatial resolution to focus on single spores. Synchrotron light was shone through a 10 × 10-μm2 aperture on the ZnSe ATR crystal, resulting in beam spot of about 4.2 × 4.2 μm2 at the surface of the hemisphere due to the high refractive index of the crystal, allowing single spores to be measured. This high resolution was obtained without compromising the signal-to-noise ratio of the IR spectra thanks to the brightness of the synchrotron IR radiation (Passot et al., 2015).
Chemometric Tools
Preprocessing
Mathematical pretreatments are necessary to eliminate experimental bias in spectral data. Classical preprocessing techniques include baseline correction, normalization, derivatives, and smoothing (Beebe et al., 1998; Wehrens, 2011).
A combination of these techniques was computed as follows: baseline correction (to reduce systematic variation from background signal), Savitzky–Golay (SG) filters (to reduce the amount of random variation such as noise), and normalization (to remove systematic variation associated with the size of the individual spores). With SG pretreatment, each point is replaced by a smoothed estimate obtained from a local polynomial regression. This pretreatment can be applied to the raw data, as well as to derivative spectra (first or second). The choice of two parameters is required: w corresponding to the window size in polynomial smoothers and p the polynomial order. In this study, the optimal window size and polynomial order were w = 13 and p = 3, respectively.
All the analyses were computed using R 3.3.2 (R Core Team and R Development Core Team, 2016). The “prospectr” package (Stevens, 2015) was used for the SG filters, and the “hyperSpec” package (Beleites and Sergo, 2015) was used for the other pretreatments.
Data Reduction
A single FTIR spectrum is represented by 1,867 variables (IR region from 650 to 4,000 cm–1). The two most informative regions were from 2,800 to 3,050 cm–1 and from 1,500 to 1,800 cm–1, which correspond to the lipid and the protein domains, respectively. To retain only useful information, these regions were pretreated separately.
Principal Component Analysis
Principal component analysis (PCA) is a widely used exploratory chemometric tool. It reduces the number of variables while projecting the data in a new space that explains the variance in the data set. The new axes, called principal components (PCs), are obtained from linear combinations of the original variables, and the variation along the first PCs is maximal. Here, the variables are the different wavelengths. They are consequently numerous and can be ordered. In contrast with traditional PCA – where loadings can be represented by a correlation circle – the loadings here are represented by a curve presenting the value of the PC loading (ordinate) according to the wavelength (abscissa). Thus, each PC is related to a curve that can be interpreted as a spectrum (Cordella, 2012). The positive and negative peaks observed on these spectra correspond to the spectral domains involved in the discrimination of the spectra. PCA was performed using R 3.3.2 (prcomp function in the stats package). The best data separation was obtained using baseline correction followed by SG (w = 13, p = 3), second derivative, and normalization.
Principal component analysis was performed on the spectra of spores treated by HP at 50°C or 20°C and on inactivated, dormant, and germinated spores (control samples). The lipid (3,050–2,800 cm–1) and the amide regions (1,800–15,00 cm–1) were processed. Some of the bands correspond to well-known IR absorption peaks associated with the spectral features of the spore clusters considered. These IR absorption peaks identified as indicators of specific protein and lipid structures are summarized in Table 1.
Results
Effect of HP on B. subtilis PS533 Spore Inactivation–Germination and Sensitivity to Nisin
The inactivation, germination, and sensitivity to nisin of B. subtilis spores were evaluated after HP treatment at 500 MPa for 10 min at 20°C or 50°C. Figure 1 presents the HP-inactivated spore fraction (dark gray bars), the HP-germinated spore fraction (light gray bars), and total spore inhibition resulting from the addition of nisin to the plating medium (black bars). The cumulated inactivation (dark and light gray bars) represents the inactivation of germinated spores achieved after HP treatment followed immediately by heat treatment at 80°C. The effect of nisin alone on spores (striped bars) represents the inhibition of spores plated only on the medium supplemented with nisin (50 IU/ml).
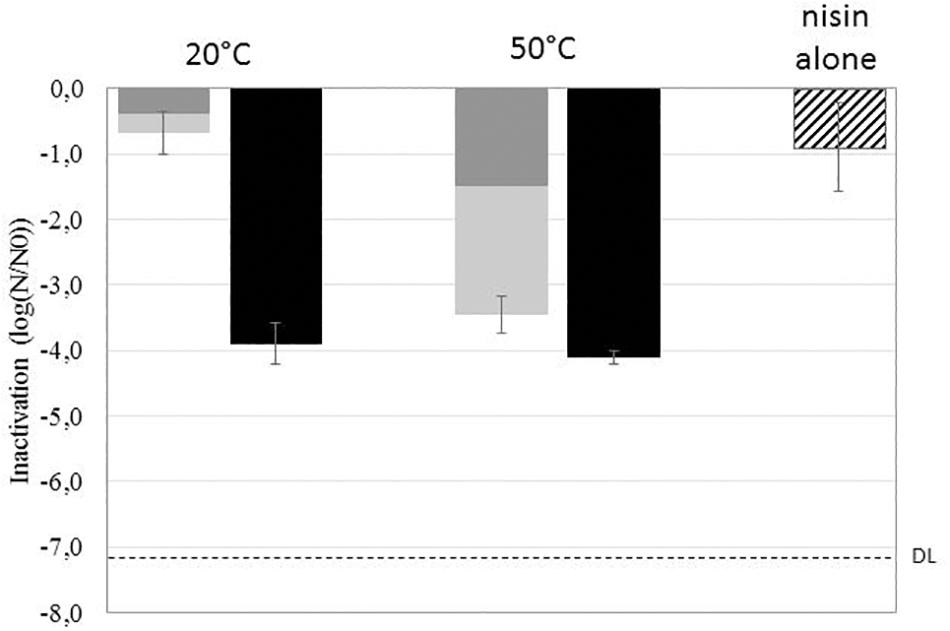
Figure 1. Effect of high pressure (HP) (500 MPa, 10 min, 20°C or 50°C) on the inactivation (dark gray), germination (light gray), and sensitization to nisin (black) of Bacillus subtilis PS533 spores. The striped bar represents the effect of nisin alone on spore inactivation. DL, detection limit.
The effect of HP alone on spores is strongly related to the treatment temperature. HP treatment at 20°C resulted in less than 1-log inactivation and germination of spores, whereas HP treatment at 50°C resulted in 1.5-log inactivation and 3.5-log cumulated inactivation and germination of spores.
The addition of nisin to the plating medium of untreated spores induced a 1-log reduction in B. subtilis spore growth. HP treatment (whether at 50°C or 20°C) followed by plating on nisin-supplemented medium induced a 4-log reduction in spore outgrowth.
Effect of Storage Time After HP Treatment on the Sensitivity of B. subtilis PS533 Spores to Nisin
Bacillus subtilis PS533 spores suspended in MES were treated by HP at 500 MPa for 10 min at 50°C or 20°C; stored at 4°C for 0, 2, 4, 6, or 24 h; and plated on LB medium supplemented or not with 50 IU/ml of nisin. The results are shown in Figure 2. Storage at 4°C did not impact B. subtilis spore growth after HP at 20°C (Figure 2A) and 50°C (Figure 2B) when plated on an LB medium without nisin.
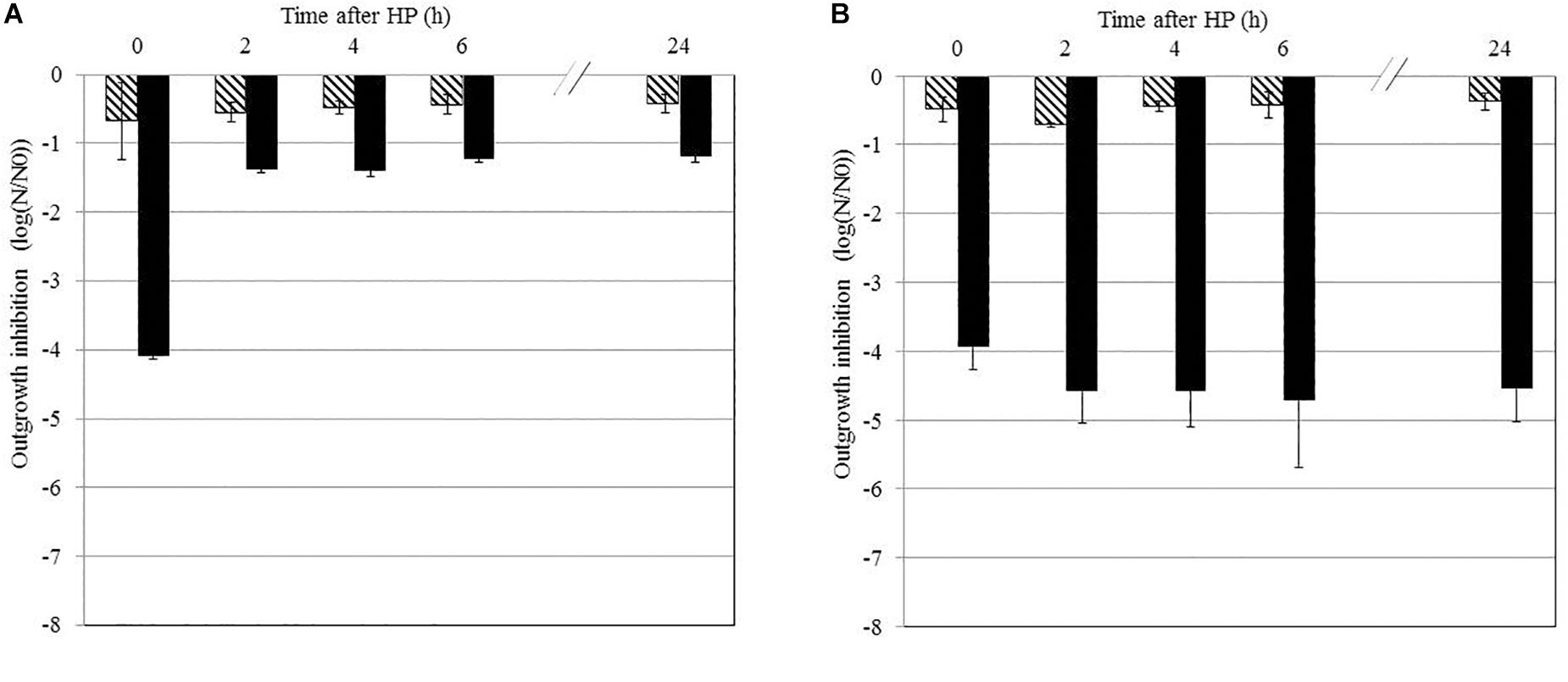
Figure 2. Effect of the storage time after high-pressure (HP) treatment on the sensitivity of Bacillus subtilis PS533 spores to nisin. Reduction of HP-treated spores after storage at 4°C for 0, 2, 4, 6, or 24 h measured by plating on Luria broth (LB) medium (striped bars) or LB medium supplemented with 50 IU/ml of nisin (black bars). (A) HP processed at 20°C; (B) HP processed at 50°C. Error bars represent SD calculated from triplicates.
Plating on an LB medium supplemented with nisin immediately after HP at 50°C and 20°C (storage for 0 h) induced a 4-log reduction in B. subtilis spore outgrowth. Spores treated by HP at 50°C remained sensitive to nisin for up to 24 h after treatment, as the inactivation remained stable (approximately 4 log). However, this sensitization seems reversible after HP treatment at 20°C, as inactivation decreased to 1 log after 2 h of storage.
Influence of the Coat in the Sensitivity of B. subtilis Spores to Nisin
Bacillus subtilis PS533 and B. subtilis PS4150 (ΔcotE; ΔgerE) were plated on LB Petri dishes supplemented with nisin concentrations varying from 50 to 200 IU/ml. The effect of nisin concentrations on growth inhibition is presented in Figure 3.
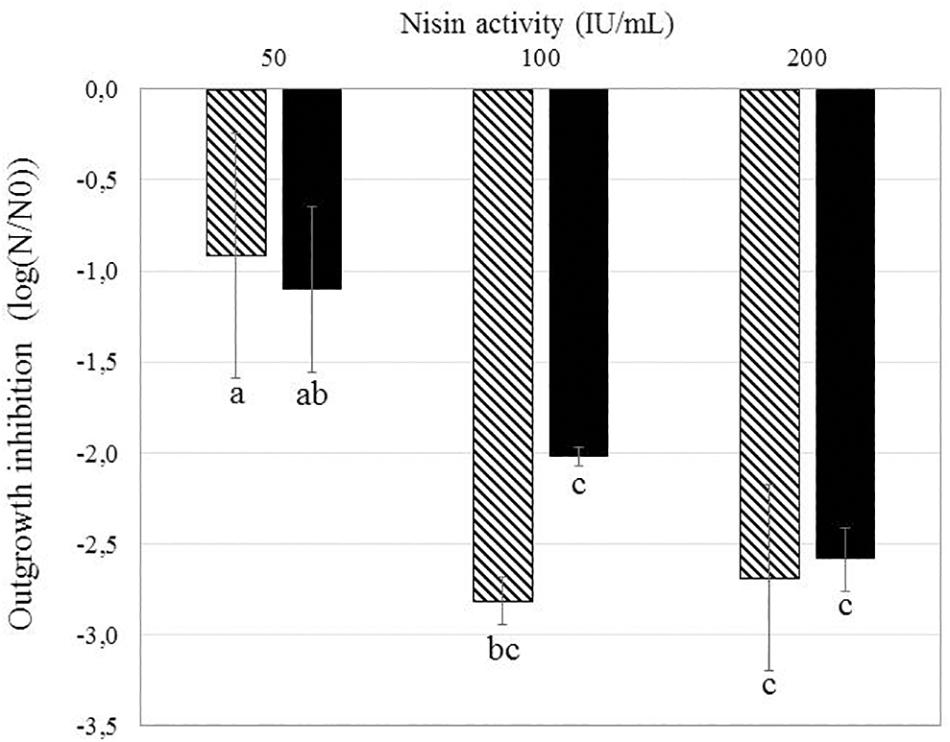
Figure 3. Effect of different nisin concentrations on the reduction of Bacillus subtilis PS533 and B. subtilis PS4150 (ΔcotE; ΔgerE) spore outgrowth. Striped bars: inhibition of B. subtilis PS533 spore outgrowth. Black bars: inhibition of B. subtilis PS4150 (ΔcotE; ΔgerE) spore outgrowth. Error bars represent SD calculated from independent triplicates. The letters represent a significant difference (p < 0.05) obtained with Tukey’s honest significant difference (HSD) test.
Non-significant differences in growth inhibition between the two strains were detectable at the three concentrations tested, which would suggest that the spore coat is not a key barrier to preventing nisin access to the inner membrane (IM).
Analysis of the Lipid Domain by FTIR Microscopy
The PCA of the lipid region is presented in Figure 4A. First, regardless of the control samples, the PCA score plot shows clear cluster separation between the spores treated by HP at 50°C (HP50, red dots) and those treated at 20°C (HP20, blue dots). The HP50 and germinated spore clusters (green dots) are clearly overlapping, showing high similarity between these two groups. Conversely, three distinctive HP20 spore groups with unique, heterogeneous properties can be observed: Gr1, Gr2, and Gr3. One part of the population (Gr3) is close to the germinated spores, and another part (Gr1) has some similarities with dormant spores (pink dots).
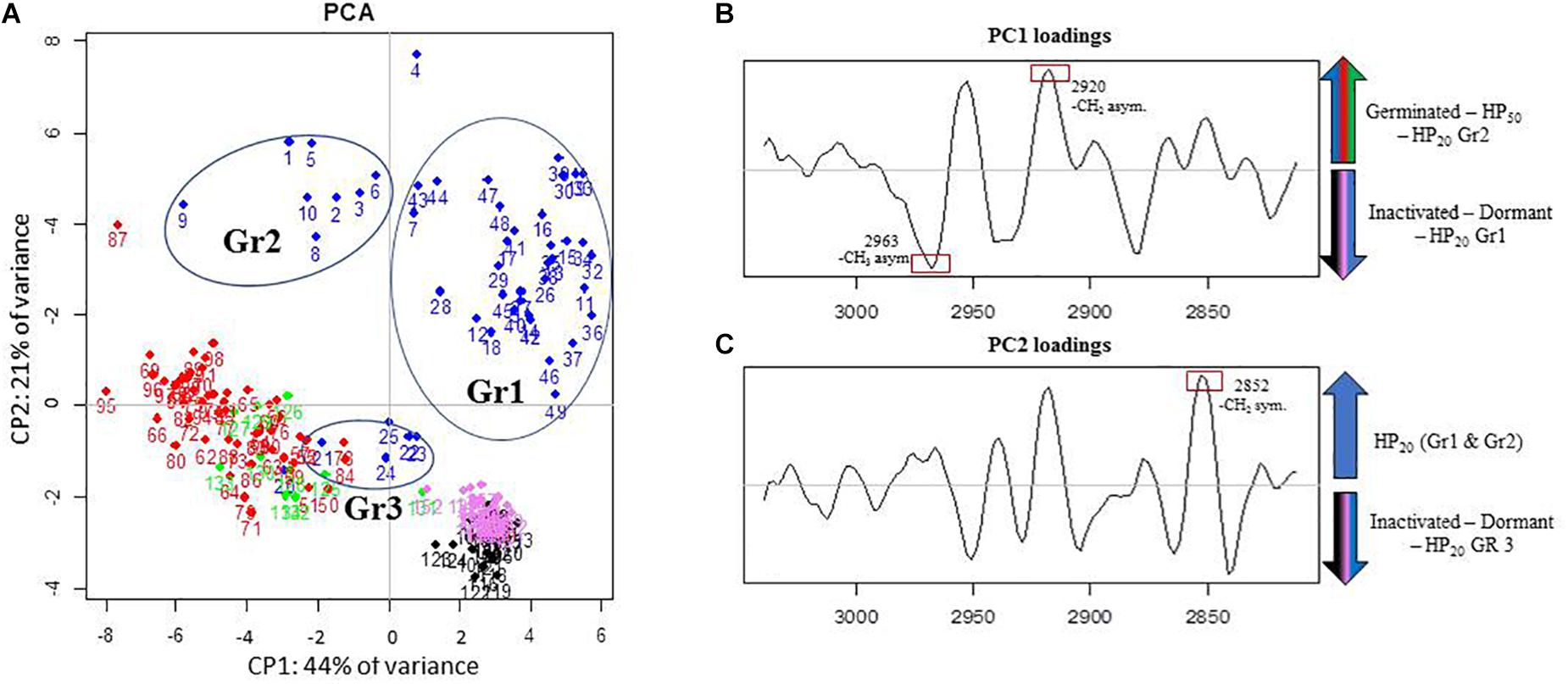
Figure 4. Principal component analysis (PCA) of infrared spectra obtained on control spore samples (germinated: green dots; dead: black dots; dormant: pink dots) and spores treated by high pressure (HP) at 500 MPa for 10 min at 50°C (red dots) or 20°C (blue dots) in the spectral region of lipids (3,050–2,800 cm–1). (A) Principal component 1 (PC1) vs. principal component 2 (PC2) score plots in the spectral regions of lipids (3,050–2,800 cm–1); (B) PC1 loadings plot; (C) PC2 loadings plot.
The PC1 loadings plot (Figure 4B) distinguishes HP20-Gr2, HP50, and germinated spore clusters from HP20-Gr1, inactivated, and dormant spores. The C–H asymmetric stretching bands of CH3 (2,963 cm–1) and CH2 (2,920 cm–1) contributed significantly to the spectral variation between these groups. Regarding the second derivative spectra of the CH3 stretching band (Figure 5A), a marked downshift of 3 cm–1 was observed for the spectra of HP20-Gr2, HP50, and germinated spores.
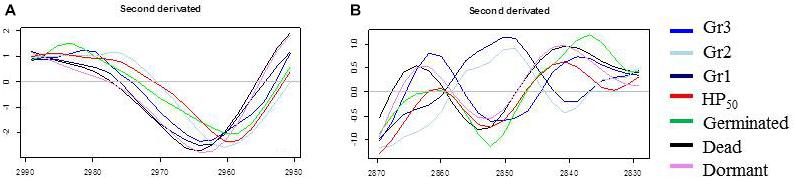
Figure 5. Second derivative of the mean infrared spectra of control spore samples (germinated; dead; dormant) and spores treated by high pressure (HP) at 500 MPa for 10 min at 50°C or 20°C. (A) Second derivative spectra in the spectral domain 2,950–2,990 cm–1; (B) Second derivative spectra in the spectral domain 2,870–2,830 cm–1.
The PC2 loadings plot (Figure 4C) distinguishes Gr1 and Gr2 from the other samples and reveals that Gr1 and Gr2 are both correlated to the C–H symmetric stretching band of the CH2 alkyl group (2,852 cm–1) (Movasaghi et al., 2008). The second derivative of the mean IR spectra of the CH2 alkyl group spectral bands contributing to the spectral variation observed between HP20-Gr1 and HP20-Gr2 and the other groups is presented in Figure 5. A negative peak at 2,850 cm–1 is visible on the spectra of all the groups except for HP20-Gr1 and HP20-Gr2 for which positive and negative peaks are present at 2,852 and 2,840 cm–1, respectively. This derivative shape around 2,850 cm–1 is the typical signature of a peak shift from above 2,850 to below 2,850 cm–1 as captured by PCA.
The ambiguous position of the Gr3 cluster, relatively close to the origins of both the PC1 and PC2 score plots, provides little information about its spectral characteristics. Nonetheless, the PC2 loadings plot indicates that Gr3 spectra displayed spectral contributions similar to those of germinated and HP50 spore clusters.
Analysis of the Amide Domain Using FTIR Microscopy
The three groups of HP20 spores identified in the lipid domain were found again for the PCA performed in the amide domain (1,800–1,500 cm–1) presented in Figure 6. Regarding the PC1 and PC2 loadings plots (Figures 6B,C), the bands corresponding to α-helix (1,660 cm–1) and β-sheet (1,620 cm–1) structures contributed significantly to the spectral variation between germinated, HP50, and HP20-Gr2 spores and the other groups.
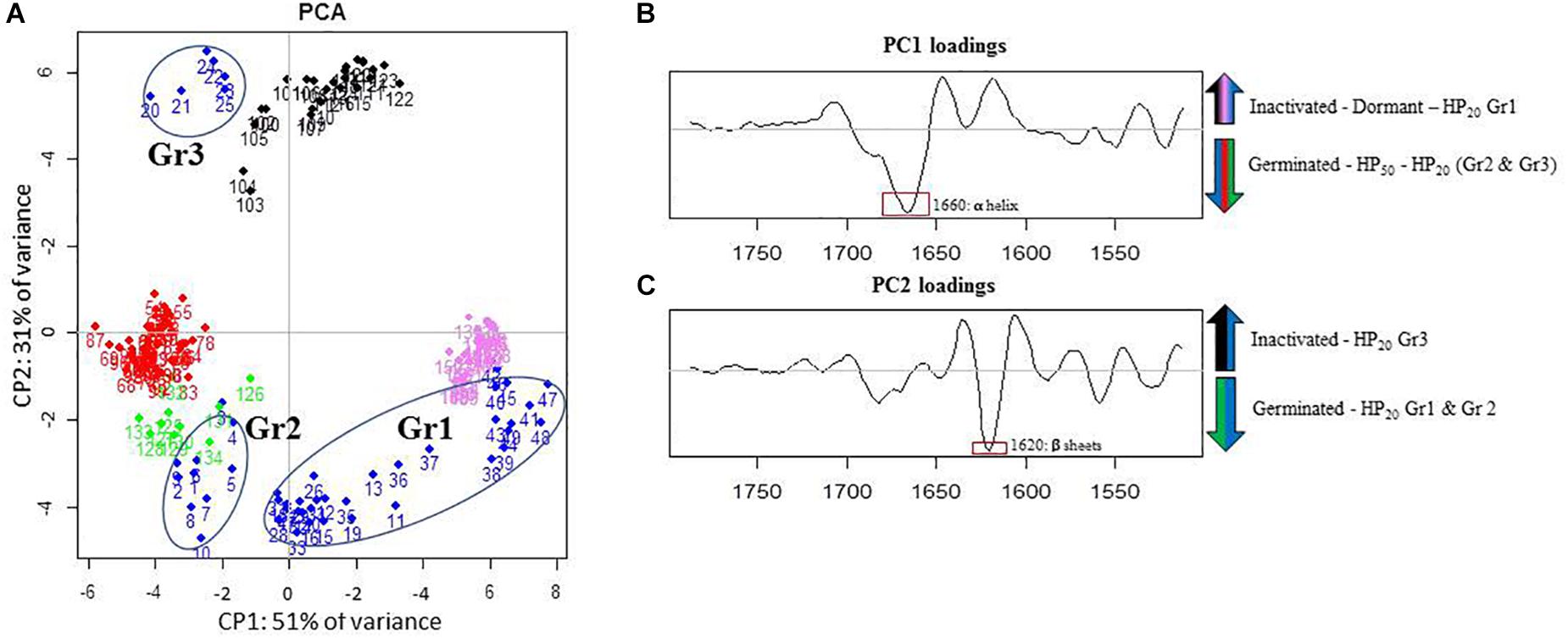
Figure 6. Principal component analysis (PCA) of infrared spectra obtained on control spore samples (germinated: green dots; dead: black dots; dormant: pink dots) and spores treated by high pressure (HP) at 500 MPa for 10 min at 50°C (red dots) or 20°C (blue dots) in the amide I and II spectral regions (1,800–1,500 cm–1). (A) Principal component 1 (PC1) vs. principal component 2 (PC2) score plots in the amide I and II spectral regions (1,800–1,500 cm–1); (B) loading plot of PC1; (C) loading plot of PC2.
The analysis of Figures 6A,B indicates high similarities between the amide properties of the HP50, HP20-Gr2, and germinated spores. Conversely, higher cell dispersion was observed within the HP20-Gr1 group: one part displayed features similar to those of dormant spores, and the other one features similar to those of germinated spores. According to the PC2 loadings plot, spores in group 3 (Gr3) seem to display amide properties similar to those of dead spores.
The amide domains of the second derivative spectra of the control samples (germinated, inactivated, dormant spores) and the three HP20 groups are presented in Figure 7. While the band corresponding to α-helix is centered around 1,654 cm–1 in spectra of germinated, HP50, and HP20-Gr2 and HP20-Gr3 spores, a shift toward 1,660 cm–1 is observed in the spectra of dormant and HP20-Gr1 spores. The band located at 1,620 cm–1 also shows that the spectra of Gr3 and inactivated spores display similar features, as a shift of the β-sheet contribution toward 1,620–1,630 cm–1 is observed for these two populations.
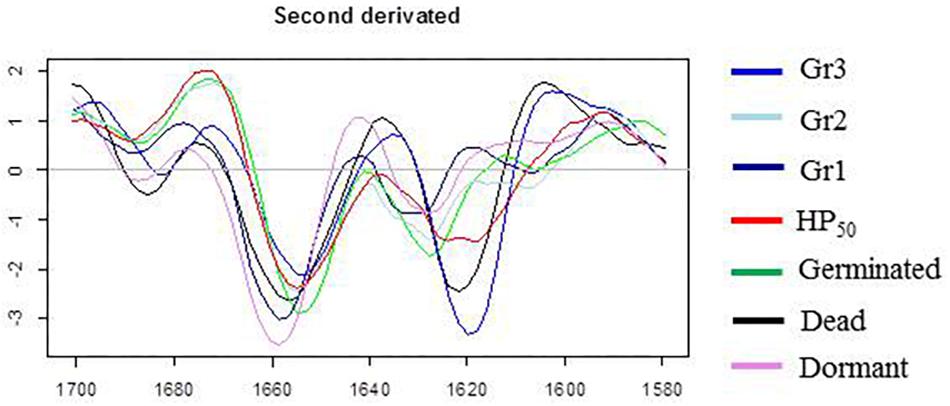
Figure 7. Second derivative of the mean infrared spectra (1,700–1,580 cm–1) of control spore samples (germinated; dead; dormant) and spores treated by high pressure (HP) at 500 MPa for 10 min at 50°C or 20°C.
Discussion
High-pressure is known to induce Bacillus spore germination. It is thus thought that the synergy between HP and other mild treatments, such as the presence of nisin following HP-induced germination, results in the inactivation of a greater number of spores (Black et al., 2008; Gao et al., 2011; Aouadhi et al., 2013). In the case of nisin, it is generally accepted that it can only have a bactericidal effect on germinated spores (Gut et al., 2008; Egan et al., 2016), thus reinforcing the aforementioned assumption. Nisin prevents spores from becoming metabolically active by disrupting the establishment of their membrane potential (Gut et al., 2008). Nisin binds to lipid II in both spores and vegetative cells. However, this is insufficient to induce membrane pore formation in spores and thus to inhibit growth recovery (Gut et al., 2011). The formation of pores could also be due to nisin binding to the thiol groups of inner proteins that only become accessible at the time of spore germination (Morris et al., 1984).
The present study first investigated the link between the occurrence of HP-induced germination of B. subtilis spores and their sensitization to nisin. Bacillus spore germination occurs in two phases. In the first stage of germination, spores release DPA and become sensitive to heat. In the second stage, the external layers are degraded, allowing the rapid swelling of spores and their subsequent sensitization to other stresses (Nguyen Thi Minh et al., 2008; Setlow, 2014). After HP treatment, spores in the first stage of germination were evaluated by heat treatment at 80°C for 10 min. This allowed us to distinguish two subpopulations after HP treatment: HP-inactivated spores and spores that were at least in the first stage of germination. The results presented in Figure 1 indicate that a large part of the spore population was germinated after HP at 50°C. The number of spores inactivated or germinated by HP at 50°C was similar to the number of spores inhibited by the nisin present in the recovery medium. We can thus assume that HP-germinated spores are sensitized to nisin in this case. The fact that the sensitization to nisin was irreversible for at least 24 h after HP treatment at 50°C (Figure 2) reinforces this hypothesis. However, after HP treatment at 20°C, a large fraction of B. subtilis spores was not in the first stage of germination before subsequent inhibition by nisin. Thus, the inhibition of spore growth by nisin after HP at 20°C is not only due to spore germination, as one subpopulation was sensitized to nisin but not germinated. Additionally, the results show this sensitization to nisin was largely reversible after HP treatment at 20°C (Figure 2). HP acts on non-covalent binding of proteins, leading to the reversible or irreversible modification of secondary to quaternary protein structures depending on the treatment parameters. Moreover, the effect of HP on proteins strongly depends on the treatment temperature, as the HP–temperature stability diagram of proteins is generally elliptical (Heremans and Smeller, 1998). Hence, our results regarding the reversibility of nisin sensitization are consistent with a mechanism involving protein denaturation.
The subpopulation of ungerminated spores sensitized to nisin could have been modified by HP in a way that promotes access of nisin to the IM. Rao et al. (2016) found similar results on B. subtilis spores treated by HP CO2 (HPCD) combined with nisin. The authors suggest that HPCD promotes the penetration of nisin into the spores by damaging their coat and cortex. This hypothesis means that nisin is unable to act on dormant spores, as the outer layers (coat and cortex) block its access to the IM. Currently, little is known regarding how nisin gets to its sites of action. The spore coat is a self-assembled proteinaceous structure consisting of at least 70 proteins. It constitutes an initial barrier to large molecules, such as peptidoglycan lytic enzymes, which would otherwise have access to the spore cortex (Leggett et al., 2012). The spore coat has also been identified as playing a critical role in resistance to many chemicals, especially oxidizing agents such as nitrogen peroxide, ozone, chlorine, and hypochlorite. In contrast, smaller molecules, such as spore germination, must presumably pass through this barrier. Nisin is a cationic peptide with a molecular weight of 3,300 Da, and its potential interaction with the spore coat is unknown. Our results showed that the spore coat may not be a key barrier in preventing nisin access to the IM of spores, as the mutant B. subtilis strain with a reduced coat is as sensitive to nisin as the wild strain (Figure 4). We therefore assumed that potential damage to the spore coat could not explain the inhibition of spore outgrowth following the use of nisin after HP at 20°C.
To identify the biochemical modifications of spores treated by HP at spore level, spores were analyzed using IR microspectroscopy immediately after HP treatment (500 MPa) at 50°C or 20°C. The biochemical modifications could be accurately identified by tracking individual spores using this technique. Instead of observing a whole spore population using “traditional” FTIR spectroscopy methods, the structural properties of single spores could be observed, and PCA analyses identified several spore subpopulations with different biochemical signatures.
As expected, the changes induced by HP at 50°C were linked to the induction of spore germination. Indeed, PCA could not discriminate the HP50 group from the germinated control group in either the amide or lipid domain. The similarities between these two populations were also confirmed by the shift in the α-helix spectral band (1,665 cm–1), previously identified as a marker of spore germination (Cheung et al., 1999). Therefore, HP treatment at 50°C induced rapid spore germination with a fast impact on the membrane and protein structure and characteristics. These results are consistent with other studies showing a rapid induction of spore germination when Bacillus spores are subjected to HP treatment at temperatures over 40°C (Nguyen Thi Minh et al., 2010; Georget et al., 2014).
Concerning spores treated by HP at 20°C, heterogeneous spectral characteristics were observed in both the lipid and amide regions. Three spore populations, Gr1, Gr2, and Gr3, were discriminated in the lipid region, mainly due to a shift in the vibration of the CH3 groups for Gr2 spores and the CH2 methylene groups toward higher wavenumbers for Gr1 and Gr2 spores compared to dormant spores. The shift in the vibration of the CH3 and CH2 groups is commonly linked to modifications in the packing of lipid molecules in biological membranes (Kapoor et al., 2011). In particular, the shift in the vibration of CH2 groups toward higher wavenumbers was previously linked to an increase in membrane fluidity (Laroche et al., 2005; Alvarez-Ordonez et al., 2010; Passot et al., 2015). Gr3 spectra displayed spectral contributions similar to those of germinated spores in the lipid region. We can draw a parallel between these three groups and the three spore subpopulations identified after HP treatment at 20°C, namely, inactivated, germinated, and sensitized to nisin. Although there are two spore subpopulations after HP treatment at 50°C (inactivated and germinated spores), the spores only form one group with respect to their spectral signature in the lipid region, which is similar to the germinated spores group. In the same way, Gr3 spores include germinated spores and spores inactivated by HP treatment at 20°C. Gr1 and Gr2 spores could thus display the signature of transitory changes arising in the inner or outer membrane of spores after HP at 20°C. The link between such changes occurring in spore membranes and the reversible sensitization of spores to nisin needs to be further investigated.
The same groups of spores were shown in the PCA chart obtained for the amide region. The bands corresponding to α-helix (1,650–1,700 cm–1) and β-sheet (1,620–1,630 cm–1) structures contributed significantly to the spectral variation between HP20 spores and the other populations (Georget et al., 2014). Gr1 spores were divided into two subgroups displaying a spectral signature similar to germinated or dormant spores, whereas Gr2 spores presented all the spectral characteristics of germinated spores. Thus, the spectral characteristics of Gr1 and Gr2 spores seem to represent a transient state that could evolve toward the spectral characteristics of germinated spores. The return of spores to a vegetative cell state is a three-step process: activation, germination, and emergence. External agents like specific pH or temperature conditions trigger the activation step. A mechanical stimulus like abrasion can also activate the spores (Keynan et al., 1964). Even though it is still not well known, a change in the conformation of a receptor protein might lead to this reversible phenomenon (Zhang et al., 2009). That way, spores get ready to wake up. It has never been shown that HP can activate bacterial spores. These changes in the lipid and amide regions could be the signature of the reversible change in the conformation of a receptor protein linked to spore activation. Generally, HP acts simply by promoting the unfolded form of proteins, which is less voluminous than the native form. HP between 100 and 500 MPa has been shown to increase the number and the reactivity of sulfhydryl groups by unfolding the protein structure and exposing the interior sulfhydryl groups (Funtenberger et al., 1997; Zhang et al., 2015).
Conclusion
In conclusion, our main hypothesis is that the inhibition of spores by nisin after HP is due to the modification of the properties of some IM proteins resulting in better binding of nisin to sulfhydryl groups. Further experiments focusing on the identification of the structures involved in this activation could potentially allow a more subtle physiological characterization of these spores.
Data Availability Statement
The raw data supporting the conclusions of this article will be made available by the authors, without undue reservation, to any qualified researcher.
Author Contributions
CM, HS, and J-MP-C contributed to the conception and design of the study. CS and FC organized the data acquisition and contributed to the data analysis. CP and LD performed the statistical analysis. CM wrote the first draft of the manuscript. CP and HS wrote sections of the manuscript. All authors contributed to the manuscript revision and read and approved the submitted version.
Funding
This work is a part of the ANR-14-CE20-0004 project funded by the French National Research Agency (ANR). This work is also supported by the Bourgogne-Franche-Comté Regional Council and the European Regional Development Fund (FEDER).
Conflict of Interest
The authors declare that the research was conducted in the absence of any commercial or financial relationships that could be construed as a potential conflict of interest.
Acknowledgments
We acknowledge SOLEIL for the provision of synchrotron radiation facilities (beamline SMIS, proposal no. 20160780).
References
Alvarez-Ordonez, A., Halisch, J., and Prieto, M. (2010). Changes in Fourier transform infrared spectra of Salmonella enterica serovars Typhimurium and Enteritidis after adaptation to stressful growth conditions. Int. J. Food Microbiol. 142, 97–105. doi: 10.1016/j.ijfoodmicro.2010.06.008
André, S., Zuber, F., and Remize, F. (2013). Thermophilic spore-forming bacteria isolated from spoiled canned food and their heat resistance. Results of a French ten-year survey. Int. J. Food Microbiol. 165, 134–143. doi: 10.1016/j.ijfoodmicro.2013.04.019
Aouadhi, C., Simonin, H., Mejri, S., and Maaroufi, A. (2013). The combined effect of nisin, moderate heating and high hydrostatic pressure on the inactivation of Bacillus sporothermodurans spores. J. Appl. Microbiol. 115, 147–155. doi: 10.1111/jam.12220
Beebe, K. R., Pell, R. J., and Seasholtz, M. B. (1998). Chemometrics: A Practical Guide. New York, NY: Wiley.
Beleites, C., and Sergo, V. (2015). Hyperspec: A Package to Handle Hyperspectral Data sets in R. In: R Package Version 0.99-20171005.
Black, E. P., Kelly, A. L., and Fitzgerald, G. F. (2005). The combined effect of high pressure and nisin on inactivation of microorganisms in milk. Innov. Food Sci. Emerg. Technol. 6, 286–292. doi: 10.1016/j.ifset.2005.04.005
Black, E. P., Linton, M., McCall, R. D., Curran, W., Fitzgerald, G. F., Kelly, A. L., et al. (2008). The combined effects of high pressure and nisin on germination and inactivation of Bacillus spores in milk. J. Appl. Microbiol. 105, 78–87. doi: 10.1111/j.1365-2672.2007.03722.x
Bruins, M. E., Matser, A. M., Janssen, A. E. M., and Boom, R. M. (2007). Buffer selection for high pressure treatment of biomaterials and its consequences for enzyme inactivation studies. High Press. Res. 27, 101–107. doi: 10.1080/08957950601082573
Cheung, H., Cui, J., and Sunt, S. (1999). Real-time monitoring of Bacillus subtilis endospore components by attenuated total reflection Fourier-transform infrared spectroscopy during germination. Microbiology 145, 1043–1048. doi: 10.1099/13500872-145-5-1043
Cordella, C. B. Y. (2012). “PCA: the basic building block of chemometrics,” in Analytical Chemistry, ed. I. S. Krul, (London: Cordella).
Doona, C. J., Ghosh, S., Feeherry, F. F., Ramirez-Peralta, A., Huang, Y., Chen, H., et al. (2014). High pressure germination of Bacillus subtilis spores with alterations in levels and types of germination proteins. J. Appl. Microbiol. 117, 711–720. doi: 10.1111/jam.12557
Egan, K., Field, D., Rea, M. C., Ross, R. P., Hill, C., and Cotter, P. D. (2016). Bacteriocins: novel solutions to age old spore-related problems? Front. Microbiol 7:461. doi: 10.3389/fmicb.2016.00461
Fang, J., Lyon, D. Y., Wiesner, M. R., Dong, J., and Alvarez, P. J. J. (2007). Effect of a fullerene water suspension on bacterial phospholipids and membrane phase behavior. Environ. Sci. Technol. 41, 2636–2642. doi: 10.1021/es062181w
Funtenberger, S., Dumay, E., and Cheftel, J. C. (1997). High Pressure Promotes Lactoglobulin Aggregation through SH/S-S Interchange Reactions. J. Agric. Food Chem. 45, 912–921. doi: 10.1021/jf960383d
Gao, Y., Qiu, W., Wu, D., and Fu, Q. (2011). Assessment of clostridium perfringens spore response to high hydrostatic pressure and heat with nisin. Appl. Biochem. Biotechnol. 164, 1083–1095. doi: 10.1007/s12010-011-9196-0
Georget, E., Kapoor, S., Winter, R., Reineke, K., Song, Y., Callanan, M., et al. (2014). In situ investigation of Geobacillus stearothermophilus spore germination and inactivation mechanisms under moderate high pressure. Food Microbiol. 41, 8–18. doi: 10.1016/j.fm.2014.01.007
Ghosh, S., Setlow, B., Wahome, P. G., Cowan, A. E., Plomp, M., Malkin, A. J., et al. (2008). Characterization of spores of Bacillus subtilis that lack most coat layers. J. Bacteriol. 190, 6741–6748. doi: 10.1128/JB.00896-08
Gut, I. M., Blanke, S. R., and Van Der Donk, W. A. (2011). Mechanism of inhibition of Bacillus anthracis spore outgrowth by the lantibiotic nisin. ACS Chem. Biol 6, 744–752. doi: 10.1021/cb1004178
Gut, I. M., Prouty, A. M., Ballard, J. D., van der Donk, W. A., and Blanke, S. R. (2008). Inhibition of Bacillus anthracis spore outgrowth by nisin. Antimicrob. Agents Chemother. 52, 4281–4288. doi: 10.1128/AAC.00625-08
Heremans, K., and Smeller, L. (1998). Protein structure and dynamics at high pressure. Biochim. Biophys. Acta 1386, 353–370. doi: 10.1016/S0167-4838(98)00102-2
Huang, H. W., Wu, S. J., Lu, J. K., Shyu, Y. T., and Wang, C. Y. (2017). Current status and future trends of high-pressure processing in food industry. Food Control 72(pt A), 1–8. doi: 10.1016/j.foodcont.2016.07.019
Kapoor, S., Werkmüller, A., Denter, C., Zhai, Y., Markgraf, J., Weise, K., et al. (2011). Temperature-pressure phase diagram of a heterogeneous anionic model biomembrane system: results from a combined calorimetry, spectroscopy and microscopy study. Biochim. Biophys. Acta - Biomembr. 1808, 1187–1195. doi: 10.1016/j.bbamem.2011.01.011
Keynan, A., Evenchik, Z., Halvorson, H. O., and Hastings, J. W. (1964). Activation of bacterial endospores. J. Bacteriol. 88, 313–318.
Koransky, J. R., Allen, S. D., and Dowell, V. R. (1978). Use of ethanol for selective isolation of sporeforming microorganisms. Appl. Environ. Microbiol. 35, 762–765.
Laroche, C., Simonin, H., Beney, L., and Gervais, P. (2005). Phase transitions as a function of osmotic pressure in Saccharomyces cerevisiae whole cells, membrane extracts and phospholipid mixtures. Biochim. Biophys. Acta - Biomembr. 1669, 8–16. doi: 10.1016/j.bbamem.2005.01.007
Leggett, M., McDonnell, G., Denyer, S. P., Setlow, P., and Maillard, J.-Y. (2012). Bacterial spore structures and their protective role in biocide resistance. J. Appl. Microbiol. 113, 485–498. doi: 10.1111/j.1365-2672.2012.05336.x
Metrick, C., Hoover, G., and Farkas, D. F. (1989). Effects of High Hydrostatic pressure on heat-resistant heat-sensitive strains of Salmonella. J. Food Sci. 54, 1547–1550. doi: 10.1111/j.1365-2621.1989.tb05156.x
Morris, S. L., Walsh, R. C., and Hansen, J. N. (1984). Identification and characterization of some bacterial membrane sulfhydryl groups which are targets of bacteriostatic and antibiotic action. J. Biol. Chem. 259, 13590–13594.
Movasaghi, Z., Rehman, S., and Rehman, I. (2008). Fourier transform infrared (FTIR) spectroscopy of biological tissues. Appl. Spectrosc. Rev. 4928, 134–179. doi: 10.1080/05704920701829043
Nguyen Thi Minh, H., Dantigny, P., Perrier-Cornet, J., and Gervais, P. (2010). Germination and inactivation of Bacillus subtilis spores induced by moderate hydrostatic pressure. Biotechnol. Bioeng. 107, 876–883. doi: 10.1002/bit.22849
Nguyen Thi Minh, H., Perrier-Cornet, J.-M., and Gervais, P. (2008). Effect of the osmotic conditions during sporulation on the subsequent resistance of bacterial spores. Appl. Microbiol. Biotechnol. 80, 107–114. doi: 10.1007/s00253-008-1519-x
Paidhungat, M., Setlow, B., Daniels, W. B., Hoover, D., Papafragkou, E., and Setlow, P. (2002). Mechanisms of induction of germination of Bacillus subtilis spores by high pressure. Appl. Environ. Microbiol. 68, 3172–3175. doi: 10.1128/AEM.68.6.3172-3175.2002
Passot, S., Gautier, J., Jamme, F., Cenard, S., Dumas, P., and Fernanda, F. (2015). Understanding the cryotolerance of lactic acid bacteria using combined synchrotron infrared and fluorescence microscopies. Analyst 140, 5920–5928. doi: 10.1039/c5an00654f
Patterson, M. F., Quinn, M., Simpson, R., and Gilmour, A. (1995). Sensitivity of vegetative pathogens to high hydrostatic pressure treatment in phosphate-buffered saline and foods. J. Food Prot. 58, 524–529. doi: 10.4315/0362-028X-58.5.524
R Core Team and R Development Core Team (2016). R: A Language and Environment for Statistical Computing. Vienna: R Core Team.
Rao, L., Wang, Y., Chen, F., and Liao, X. (2016). the synergistic effect of high pressure CO2 and nisin on inactivation of Bacillus subtilis spores in aqueous solutions. Front. Microbiol. 7:1507. doi: 10.3389/fmicb.2016.01507
Setlow, P. (2014). Germination of spores of Bacillus species: what we know and do not know. J. Bacteriol. 196, 1297–1305. doi: 10.1128/JB.01455-13
Stevens, M. A. (2015). Package ‘ Prospectr. Available at: https://github.com/antoinestevens/prospectr.
Valentine, N. B., Johnson, T. J., Su, Y., and Forrester, J. B. (2006). FTIR Spectroscopy for Bacterial Spore Identification and Classification. Proc. SPIE 6378, Chem. Biol. Sensors Ind. Environ. Monit. II 6378, 1–10. doi: 10.1117/12.686232
Wehrens, R. (2011). “Chemometrics with R,” in Multivariate Data Analysis in the Natural Sciences and Life Sciences. Use R!, eds R. Gentleman, K. Hornik, and G. Parmigiani, (London: Springer).
Wolkers, W. F., and Hoekstra, F. A. (1995). Aging of dry desiccation-tolerant pollen does not affect protein secondary structure. Plant Physiol. 109, 907–915. doi: 10.1104/pp.109.3.907
Zhang, P., Setlow, P., and Li, Y. (2009). Characterization of single heat-activated Bacillus spores using laser tweezers Raman spectroscopy. Opt. Express 17, 445–448. doi: 10.1364/OE.17.016480
Keywords: Bacillus subtilis, nisin, germination, activation, mild treatments
Citation: Modugno C, Peltier C, Simonin H, Dujourdy L, Capitani F, Sandt C and Perrier-Cornet J-M (2020) Understanding the Effects of High Pressure on Bacterial Spores Using Synchrotron Infrared Spectroscopy. Front. Microbiol. 10:3122. doi: 10.3389/fmicb.2019.03122
Received: 14 February 2019; Accepted: 24 December 2019;
Published: 31 January 2020.
Edited by:
Marta López, Spanish National Research Council (CSIC), SpainReviewed by:
Marcela Claudia Pagano, Federal University of Minas Gerais, BrazilStella Maris Reginensi Rivera, Universidad de la República, Uruguay
Peter Setlow, University of Connecticut Health Center, United States
Copyright © 2020 Modugno, Peltier, Simonin, Dujourdy, Capitani, Sandt and Perrier-Cornet. This is an open-access article distributed under the terms of the Creative Commons Attribution License (CC BY). The use, distribution or reproduction in other forums is permitted, provided the original author(s) and the copyright owner(s) are credited and that the original publication in this journal is cited, in accordance with accepted academic practice. No use, distribution or reproduction is permitted which does not comply with these terms.
*Correspondence: Hélène Simonin, SGVsZW5lLnNpbW9uaW5AYWdyb3N1cGRpam9uLmZy; Francesco Capitani, ZnJhbmNlc2NvLmNhcGl0YW5pQHN5bmNocm90cm9uLXNvbGVpbC5mcg==