- 1Interdepartmental Microbiology Graduate Program, Iowa State University, Ames, IA, United States
- 2Department of Animal Science, Iowa State University, Ames, IA, United States
Listeria monocytogenes is a well-characterized pathogen that represents a major threat to food safety. In this study, we examine the chromosomal and plasmid transcriptomes of two different L. monocytogenes strains, 6179 [belonging to sequence type (ST) 121] and R479a (ST8), in response to 30 min exposure to oxidative (0.01% hydrogen peroxide) and acid (1% lactic acid, pH 3.4) stress. The exposure to oxidative stress resulted in 102 and 9 differentially expressed (DE) genes in the chromosomal transcriptomes of 6179 and R479a, respectively. In contrast, 2280 and 2151 DE genes were observed in the respective chromosomal transcriptomes of 6179 and R479a in response to lactic acid stress. During lactic acid stress, we observed upregulation of numerous genes known to be involved in the L. monocytogenes stress response, including multiple members of the σB regulon, many of which have not been functionally characterized. Among these genes, homologs of lmo2230 were highly upregulated in both strains. Most notably, the σB-dependent non-coding RNA Rli47 was by far the most highly expressed gene in both 6179 and R479a, accounting for an average of 28 and 38% of all mapped reads in the respective chromosomal transcriptomes. In response to oxidative stress, one DE gene was identified in the 6179 plasmid transcriptome, and no DE genes were observed in the transcriptome of the R479a plasmid. However, lactic acid exposure resulted in upregulation of the stress response gene clpL, among others, on the 6179 plasmid. In R479a, a number of uncharacterized plasmid genes were upregulated, indicating a potential role in stress response. Furthermore, an average of 65% of all mapped transcriptome reads for the R479a plasmid following acid stress were mapped to an intergenic region bearing similarity to riboswitches involved in transition metal resistance. The results of this study support the conclusion that members of the σB regulon, particularly lmo2230 and the non-coding RNA Rli47, play an integral role in the response of L. monocytogenes to acid stress. Furthermore, we report the first global transcriptome sequencing analysis of L. monocytogenes plasmid gene expression and identify a putative, plasmid-encoded riboswitch with potential involvement in response to acid exposure.
Introduction
Food-borne pathogens present a major concern to public health, causing diseases ranging from gastroenteritis to meningitis. In some cases, these infections are severe enough to result in hospitalization or death. Therefore, preventing food-borne pathogens from contaminating foods and food production environments (FPEs) is essential. To mitigate contamination, the food production and processing industries utilize a variety of methods including strict hygiene plans, cleaning agents, disinfectants, and food additives to prevent bacterial growth. As a result, food-borne pathogens are routinely exposed to a variety of stress conditions such as high salinity and extremes in both pH and temperature. Cleaning routines and disinfecting agents can expose food-borne pathogens to heavy metals or reactive oxygen species, and the presence of preservatives such as salts and organic acids, produced by fermentation or added intentionally, present additional environmental stressors for microorganisms.
Despite these efforts, the food-borne pathogen Listeria monocytogenes readily persists in FPEs (Carpentier and Cerf, 2011; Ferreira et al., 2014). L. monocytogenes is the causative agent of listeriosis, a disease that has the potential to cause severe conditions such as meningitis, sepsis, and spontaneous abortions in susceptible individuals. Although listeriosis has a low morbidity rate, the high rates of hospitalization and mortality associated with this disease are of significant concern (Allerberger and Wagner, 2010; Scallan et al., 2011). Furthermore, the economic consequences of product recalls caused by L. monocytogenes contamination have been estimated to be 1.2–2.4 billion United States dollars annually (Ivanek et al., 2004), and in 2008, a single outbreak of 57 cases of listeriosis in Canada was estimated to have cost a total of 242 million Canadian dollars (Thomas et al., 2015). L. monocytogenes is also concerning because it is considerably more resilient to the numerous stress conditions used in FPEs to mitigate contamination than many other food-borne pathogens. As a result, L. monocytogenes is able to colonize niche environments in FPEs such as drainage and hard-to-clean surfaces, and therefore the complete eradication of L. monocytogenes from a FPE is a difficult task (Ferreira et al., 2014). In addition to persistence in FPEs themselves, the tolerance of L. monocytogenes to food preservatives provides the organism with a competitive advantage when growing in certain types of foods, particularly in “ready-to-eat” foods that are highly processed and have a long shelf life.
Upon exposure to environmental stress conditions found in food and FPEs, L. monocytogenes responds by activating a wide variety of mechanisms that confer stress tolerance (Dutta et al., 2013; Müller et al., 2013; Harter et al., 2017; Bucur et al., 2018; Hingston et al., 2019). In addition to functional characterization, multiple gene expression analyses have implicated many genes in the response of L. monocytogenes to temperature, oxidative, disinfectant, pH, and osmotic stresses (Oliver et al., 2009; Soni et al., 2011; Tessema et al., 2012; Casey et al., 2014; Tang et al., 2015; Hingston et al., 2017b). Until now, transcriptomic research on L. monocytogenes has been conducted almost exclusively on strains that lack plasmids. Previous research from our group has demonstrated that the plasmids of L. monocytogenes strains 6179 and R479a contribute to survival under heat, acid, salt, and oxidative stress (Naditz et al., 2019). Therefore, transcriptomic analysis may provide valuable insight into the effect of plasmids on stress tolerance and may also identify novel candidate chromosomal features permitting L. monocytogenes persistence in food and FPEs. Thus, this study conducted transcriptome sequencing of the plasmid-carrying strains 6179 and R479a to analyze their chromosomal and plasmid gene expression patterns after short-term exposure to oxidative and acid stress.
Materials and Methods
Bacterial Strains Used in This Study
Listeria monocytogenes 6179 is a persistent strain that belongs to ST121 and was isolated from Irish cheese in 2000 (Fox E. et al., 2011; Fox E. M. et al., 2011). It possesses a 3.01 Mbp genome and a 62.2 kbp plasmid, designated pLM6179 (Schmitz-Esser et al., 2015b). Notably, the stress survival characteristics of L. monocytogenes 6179 have been extensively characterized (Müller et al., 2013, 2014; Casey et al., 2014; Makariti et al., 2015; Rychli et al., 2016; Harter et al., 2017; Naditz et al., 2019). L. monocytogenes strain R479a is an ST8 strain isolated from smoked salmon in Denmark in 1996 where it persisted for more than 2 years in the same FPE (Fonnesbech Vogel et al., 2001; Schmitz-Esser et al., 2015a). L. monocytogenes R479a possesses a 2.94 Mbp genome and a 86.6 kbp plasmid, pLMR479a. Similarly to 6179, L. monocytogenes R479a has been subject to multiple stress survival analyses (Müller et al., 2013; Fagerlund et al., 2016; Rychli et al., 2016; Naditz et al., 2019).
Experimental Stress Conditions
Recently, we demonstrated that plasmids pLM6179 and pLMR479a contribute to the survival of L. monocytogenes strains 6179 and R479a during acid (1% (vol/vol) lactic acid, pH 3.4) and oxidative stress (0.01% (vol/vol) hydrogen peroxide) (Naditz et al., 2019). To elucidate plasmid genes with potential involvement in stress response, we sequenced the transcriptomes of 6179 and R479a under the same stress conditions as applied in Naditz et al. (2019). Overnight cultures of 6179 and R479a were made by inoculating a loop of colonies into 25 mL of tryptic soy broth (TSB; Becton, Dickinson and Company) and incubating at 20°C with 200 rpm shaking for 22 h. 7.5 mL of 6179 culture and 15 mL of R479a culture were harvested and spun down at 4696 × g at 20°C for 10 min before pouring off the supernatants, and the 6179 and R479a pellets were then resuspended in 5 and 7.5 mL sterile 1× PBS, respectively. OD600 values were measured using a spectrophotometer (SmartSpec 3000, Bio-Rad Laboratories), and the cultures were adjusted to an OD600 of 3.5 ± 0.2. Next, 0.5 mL of adjusted overnight culture was then inoculated into 4.5 mL TSB with either a final concentration of 0.01% (vol/vol) hydrogen peroxide or 1% (vol/vol) lactic acid (pH 3.4). These tubes were then incubated at 20°C with 200 rpm shaking. To reduce the chance of significant RNA degradation, stress exposure was conducted for 30 min rather than 2 h as previously conducted (Naditz et al., 2019). Controls for both strains were created in a similar manner by inoculating 0.5 mL of adjusted overnight culture to 4.5 mL of TSB without the addition of hydrogen peroxide or lactic acid. Each condition, including the controls, was conducted in biologically independent triplicates. For the triplicates, three tubes for each condition were combined to ensure sufficiently high RNA concentrations for transcriptome sequencing.
RNA Extraction, Library Preparation, and Sequencing
Following stress exposure for 30 min, the three tubes for each condition were combined and centrifuged at 4696 × g at 20°C for 3 min. Supernatants were poured off, and the pellets were each resuspended in 600 μL of Invitrogen Purelink RNA Mini Kit lysis buffer containing 1% β-mercaptoethanol. The protocol for the Invitrogen Purelink RNA Mini Kit was then followed for RNA extraction, and chemical lysis was complemented with mechanical lysis using a bead-beater (Lysing Matrix E, MP Biomedicals; Bead Mill 24 Homogenizer, Fisher Scientific). 1 μL Superase RNase inhibitor (Invitrogen) was added to each sample, and any remaining DNA was removed using the Turbo DNA-Free kit (Invitrogen) following the instructions of the manufacturer.
A PCR targeting the prfA gene using the primers Lip1 (5′-GAT ACA GAA ACA TCG GTT GGC-3′) and Lip2 (5′-GTG TAA TCT TGA TGC CAT CAG G-3′) (Rossmanith et al., 2006) yielded no amplified DNA, confirming the absence of L. monocytogenes DNA in the extracted RNA samples. PCR was conducted using the Platinum Taq DNA Polymerase system (Invitrogen) according to the manufacturer’s specifications. PCR cycle conditions were as follows: initial denaturation at 94°C (4 min), 35 cycles of denaturation at 94°C (30 s), annealing at 64°C (30 s), elongation at 72°C (30 s), and final elongation at 72°C (5 min). PCR products were then confirmed with agarose gel electrophoresis.
The RNA integrity of all samples was then measured using an RNA 6000 Nano chip via an Agilent 2100 Bioanalyzer (Prokaryote Total RNA Nano assay). All RNA samples had RNA integrity numbers (RIN) of at least 9.9 (Supplementary Figure S1). Samples were then sent to Microsynth (Balgach, Switzerland) for ribosomal RNA depletion using the Ribo-Zero rRNA Removal Kit (Gram-Positive Bacteria, Illumina) and stranded TruSeq library preparation. Triplicate samples were sequenced for all experimental conditions, resulting in a total of 18 samples. Single-read, 75 bp reads were generated by Microsynth using Illumina NextSeq, and read-trimming and demultiplexing were also performed by Microsynth.
Sequence Analysis
Reads were mapped to either the L. monocytogenes 6179 or R479a chromosomes (HG813249.1 and HG813247.1, respectively) and plasmids (HG813250.1 and HG813248.1, respectively) using the Burrows-Wheeler aligner mem algorithm (Li and Durbin, 2010). The resulting BAM files were then imported into ReadXplorer (Hilker et al., 2014), and statistical analysis was performed using the DeSeq2 package included in ReadXplorer. DeSeq2 utilizes the Benjamini and Hochberg method for correction for multiple-testing to generate adjusted p-values, also called Q-values (Benjamini and Hochberg, 1995); Q-values lower than 0.05 were considered significant. Principal component analysis (PCA) was performed using R statistical software v3.5.1 (R Development Core Team, 2018) and the packages DeSeq2 v1.22.2 (Love et al., 2014) and ggplot2 v3.1.0 (Hadley, 2016). Nucleotide and amino acid alignments were done with MAFFT (Katoh et al., 2019), and shading of conserved amino acid residues was performed with Boxshade available at: https://embnet.vital-it.ch/software/BOX_form.html. RNA secondary structure predictions were generated using the RNAfold WebServer (Hofacker, 2003).
BLAST Methods
NCBI BLAST (Wheeler et al., 2008) was used to determine homologs between the locus_tags of the 6179 and R479a chromosomes (HG813249.1 and HG813247.1, respectively) as well as homologs between the chromosomal locus_tags of the aforementioned strains and EGD-e locus_tags (NC_003210.1). Protein BLAST searches were carried out using the module NCBI-blast (2.4.0). Only those matches with 90% amino acid identity or greater were considered homologs between strains.
Results and Discussion
Chromosomal Gene Expression
Average chromosome coverage for each condition ranged from 165× to 232× with an average of 192× coverage for all 18 samples. On average, 97.3 and 96.4% of the total reads per sample mapped to the 6179 and R479a chromosomes, respectively (Table 1).
Principal component analysis conducted between the replicates demonstrated that for a given strain the replicates of each condition clustered closely together, indicating that there were no major disparities between replicates (Figure 1). In general, changes in the chromosomal transcription patterns of 6179 and R479a were less pronounced in response to 30 min exposure to 0.01% hydrogen peroxide than in response to 1% lactic acid. The replicates for both lactic acid exposure experiments clustered far from the control, whereas the replicates for the hydrogen peroxide experiments fell closer to the controls (Figure 1). Based on a Q-value of <0.05, 102 DE genes were identified in 6179 in the hydrogen peroxide treatment, and nine DE genes were identified in R479a (Table 2 and Supplementary Tables S1, S2). In contrast, lactic acid exposure resulted in differential expression of 2280 genes in 6179 and 2151 genes in R479a (Table 2 and Supplementary Tables S1, S2).
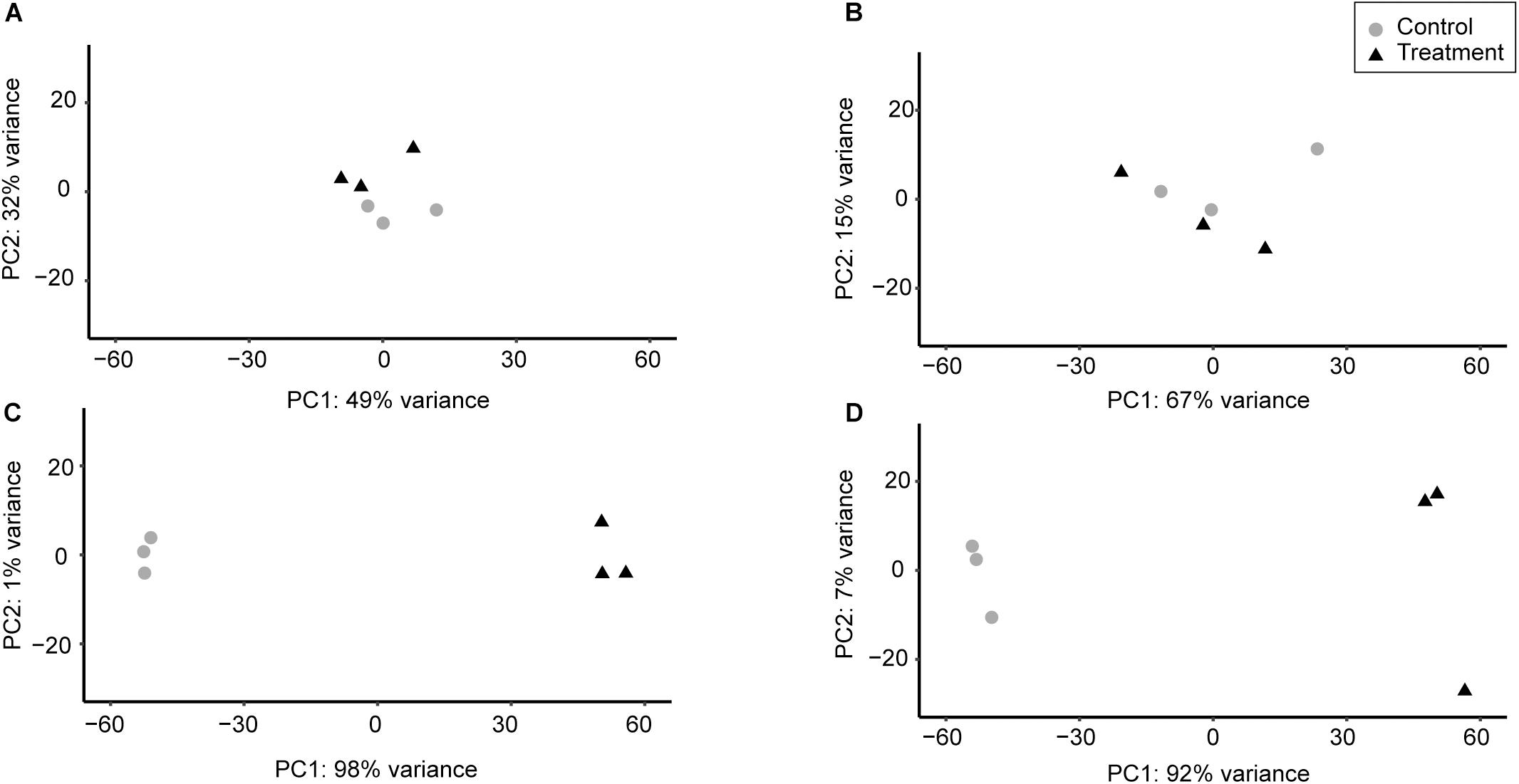
Figure 1. Differences between transcriptome replicates of L. monocytogenes strains 6179 and R479a under oxidative and acid stress conditions based on principal component analysis. For each condition, the three control replicates for each strain were compared to the three treatment replicates. (A) 6179 control vs. 6179 exposed to 0.01% H2O2, (B) R479a control vs. R479a exposed to 0.01% H2O2, (C) 6179 control vs. 6179 exposed to 1% lactic acid, (D) R479a control vs. R479a exposed to 1% lactic acid.
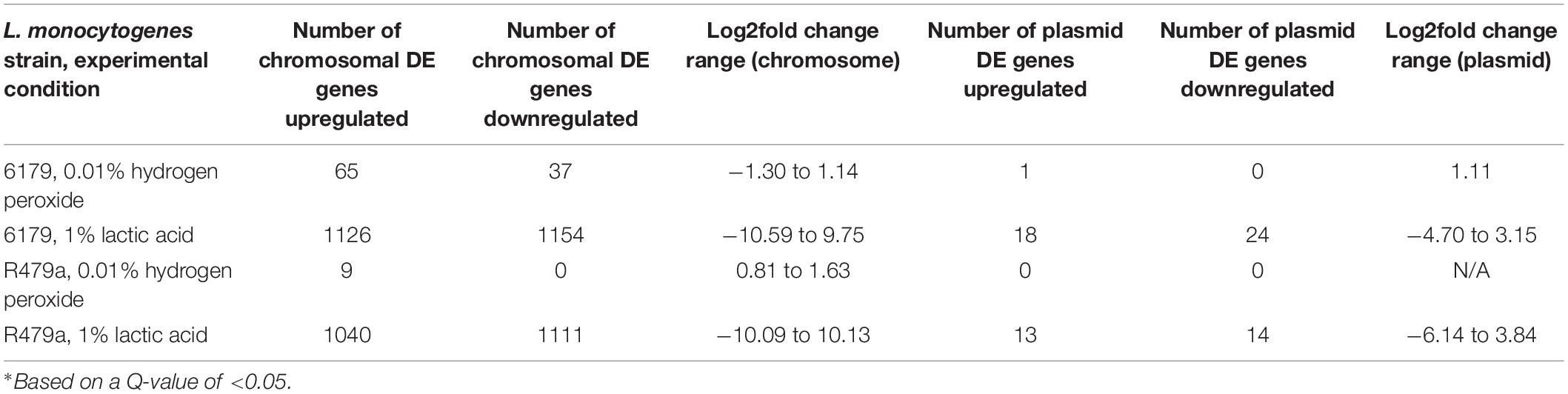
Table 2. Number of differentially expressed (DE) chromosomal and plasmid genes∗ in response to 1% lactic acid and 0.01% hydrogen peroxide.
Further demonstrating the difference in the stress response between the two conditions, the log2fold changes of DE genes ranged from −1.30 to 1.14 in the 6179 hydrogen peroxide treatment and from 0.81 to 1.63 in the R479a hydrogen peroxide treatment. Under lactic acid stress exposure, the log2fold change ranges of 6179 and R479a were −10.59 to 9.75 and -10.09 to 10.13, respectively (Table 2 and Supplementary Tables S1, S2).
Chromosomal Gene Expression Changes in Response to Hydrogen Peroxide Treatment
Although other studies observed pronounced transcriptional shifts (Pleitner et al., 2014) and increased expression of a functionally characterized oxidative stress survival islet (Harter et al., 2017) following 15 and 10 min exposures to oxidative stress, respectively, our study observed relatively minor transcriptomic differences even after 30 min of oxidative stress exposure. Only nine DE genes were seen in the R479a hydrogen peroxide treatment (Supplementary Table S3), although seven of these were also among the 50 DE genes of 6179 with the highest log2fold changes (Supplementary Table S4). Two of these shared DE genes are annotated as ohrA and ohrR, encoding a peroxiredoxin and its transcription factor which are involved in peroxide resistance in Bacillus subtilis (Fuangthong et al., 2001). In 6179, ohrA and ohrR were upregulated by log2fold changes of 1.06 and 1.04, respectively, and in R479a by respective log2fold changes of 1.02 and 0.99. In L. monocytogenes, transposon mutagenesis of ohrA resulted in decreased tolerance to hydrogen peroxide, diamide, and cumene hydroperoxide (Reniere et al., 2016). Additionally, exposure to chlorine dioxide resulted in increased levels of ohrA expression (Pleitner et al., 2014). Genes annotated as enzymes involved in certain steps of the citric acid cycle (citZ – citrate synthase, citB – aconitase, and citC – isocitrate dehydrogenase) were also upregulated in both 6179 and R479a following exposure to hydrogen peroxide. Two of these genes, citC and citZ, are part of an operon (Mittal et al., 2009) along with the upstream gene (a lmo1568 homolog), which was upregulated as well. However, the relevance of these three citric acid cycle genes to stress response is currently unknown.
In 6179, the DE gene with the highest log2fold change (1.14) was bilEA, a gene involved in bile resistance (Sleator et al., 2005). However, bilEA was not differentially expressed in R479a. Another DE gene unique to the 6179 transcriptome in response to hydrogen peroxide exposure was LM6179_3010, a gene encoding a 141 amino acid protein upregulated by a log2fold change of 1.11. LM6179_3010 shares 100% nucleotide identity with the L. monocytogenes EGD-e gene lmo2230, a known member of the σB regulon, the primary stress response regulon in L. monocytogenes (Raengpradub et al., 2008). LM6179_3010 and lmo2230 show 28% amino acid identity to ArsC, an arsenate reductase from B. subtilis (Sato and Kobayashi, 1998). However, essential cysteine amino acid residues required for function as an arsenate reductase are not conserved (Supplementary Figure S2; Bennett et al., 2001), suggesting that Lmo2230 and homologs do not function as arsenate reductases. Lmo2230 and its homologs are discussed in more detail in the section covering the lactic acid stress response below.
Also unique to the 6719 hydrogen peroxide response was the upregulation of two oxidative stress protection genes: sodA (LM6179_2184), a superoxide dismutase upregulated by a log2fold change of 0.79, and LM6179_1300, annotated as a glutathione peroxidase and upregulated by a log2fold change of 0.56 (Chatterjee et al., 2006; Hingston et al., 2015). The importance of the superoxide dismutase in L. monocytogenes stress response has been documented before (Soni et al., 2011; Bucur et al., 2018), and upregulation of the EGD-e homolog of LM6179_1300, lmo0983, was observed following exposure to chlorine dioxide (Pleitner et al., 2014). However, stress survival islet 2 (SSI-2), an insert of two genes homologous to the Listeria innocua genes lin0464 and lin0465 (LM6179_0748 and LM6179_0749), was not differentially expressed in 6179 despite previous implication in oxidative stress (Harter et al., 2017). The lack of significant upregulation of the SSI-2 genes under the conditions applied in our study may be explained by the differences in oxidative stress induction between the two studies. Here, 0.01% hydrogen peroxide was used to induce oxidative stress, whereas Harter et al. (2017) used 10 mM cumene hydroperoxide. The lower number of DE genes in response to hydrogen peroxide treatment in R479a compared to 6179 suggests that R479a, which belongs to a different ST than 6179, may be inherently more tolerant to hydrogen peroxide-induced oxidative stress than 6179. However, it is also possible that the 30 min oxidative stress exposure period or the concentration of hydrogen peroxide applied in this study may have been too short to induce broader changes in gene expression.
Chromosomal Gene Expression Changes in Response to Lactic Acid Treatment
As a whole, the 6179 and R479a responses to lactic acid stress were quite similar, as was expected based on their chromosomal similarity (Naditz et al., 2019). Of the genes with homologs in both strains, 1742 were differentially expressed in the transcriptomes of both 6179 and R479a (Figure 2). Only 24 of these 1742 shared DE genes had inverse expressions (upregulated in 6179 and downregulated in R479a or vice versa), thus demonstrating that the overall lactic acid stress response is highly similar between both strains. The remaining DE genes, 538 in 6179 and 409 in R479a, were unique to the response of each strain. Due to the number of DE genes, we focused further analysis on those DE genes previously implicated in acid stress response and/or with log2fold changes > 2.0.
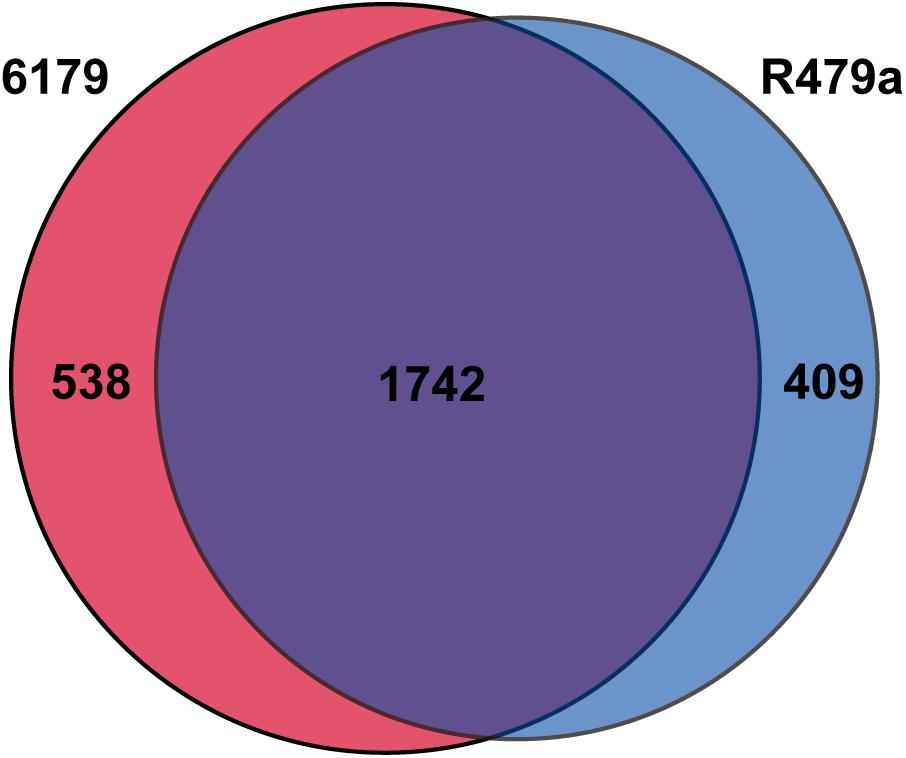
Figure 2. Number of unique and shared DE genes in L. monocytogenes strains 6179 and R479a exposed to 1% lactic acid.
A few notable patterns of downregulated DE genes were observed in both 6179 and R479a following lactic acid stress. Of these, the most apparent was that a large proportion of the 50 DE genes with the most negative log2fold changes encoded ribosomal proteins (23/50 in 6179 and 24/50 in R479a, Supplementary Tables S5, S6). Additional common patterns included the downregulation of genes encoding tRNAs, flagellar and motility components, chemotaxis proteins, and genes involved in replication and fatty acid biosynthesis. The downregulation of these genes suggests a decrease in general metabolic activity and motility in response to the stress conditions. In terms of general stress response, lmo2748, encoding the general stress protein 26 (GSP26), was upregulated by a log2fold change of 7.96 in 6179 and 7.59 in R479a. Although a role for GSP26 in osmotic stress response has been determined in L. monocytogenes, the same study demonstrated that a deletion of lmo2748 does not result in deficiencies in survival under acid stress (Abram et al., 2008). Indeed, in B. subtilis, GSP26 is induced in response to glucose and oxygen limitation as well as to heat, osmotic, and, to a lesser degree, oxidative stress (Volker et al., 1994).
The general stress response of L. monocytogenes is connected to the organism’s pathogenic lifestyle by direct interactions with virulence factors. Notably, σB directly regulates the transcription of two internalins involved in initial host cell invasion, inlA and inlB (Chaturongakul et al., 2008), and a complex regulatory interaction between the σB and PrfA regulons is necessary for virulence (Chaturongakul et al., 2008; Gaballa et al., 2019). In this study, inlA (which possesses a premature stop codon in 6179 and is therefore annotated as two separate genes) was upregulated by log2fold changes of 7.07 and 6.95 in 6179 and 8.43 in R479a. To a lesser degree, inlB was also upregulated in both 6179 and R479a with log2fold changes of 4.16 and 4.18, respectively. Other notable upregulated virulence genes include prfA (log2fold changes of 3.70 in 6179 and 3.72 in R479a) and hly (log2fold changes of 1.92 in 6179 and 4.58 in R479a). These results demonstrate that even brief exposure to lactic acid at low pH is capable of inducing the expression of virulence genes in L. monocytogenes.
Previous studies have demonstrated that the presence of lysogenic prophages in a genome can confer beneficial effects (Edlin et al., 1977; Burns et al., 2015) including tolerance to acid stress (Wang et al., 2010). In L. monocytogenes, an induction of prophage gene expression has recently been reported during intracellular replication (Hain et al., 2012; Schultze et al., 2015) and for the L. monocytogenes 10403S A118 prophage after acid stress exposure (Ivy et al., 2012). The tRNA-Arg-TCT prophage is found in both 6179 and R479a. This prophage contained 20 DE genes in 6179 (19 upregulated with log2fold changes ranging from 0.79 to 2.98) and 11 DE genes in R479a (all upregulated ranging in log2fold change from 0.62 to 4.39). 6179 harbors two additional prophages integrated into the tRNA-Arg-CCG and tRNA-Thr-GGT loci. These had 47 DE genes (45 upregulated with log2fold changes ranging from 0.73 to 2.37) and 31 DE genes (29 upregulated with log2fold changes ranging from 1.45 to 3.29), respectively. Notably, a role in biofilm formation for the comK prophage found in certain L. monocytogenes strains including R479a has previously been supported (Verghese et al., 2011), and in this study, 29 of the 68 comK prophage genes in R479a were differentially expressed (28 upregulated with log2fold changes ranging from 1.10 to 4.12). However, it remains unknown whether the expression of prophage genes under stress conditions merely results in the formation of lytic phage particles or actually increases stress tolerance. Although not conclusive, the results of this study raise the possibility that the induction of these prophage genes under lactic acid stress confers beneficial effects on 6179 and R479a. However, verifying this hypothesis would require further experimentation.
Additionally, both strains analyzed in this study possess different stress survival islets (SSIs) inserted between the homologs of lmo0443 and lmo0449. As mentioned above, 6179 possesses SSI-2, a two-gene insert with homology to the L. innocua genes lin0464 and lin0465, a transcriptional regulator and protease involved in oxidative and alkaline stress (Harter et al., 2017). Corroborating the conclusion of Harter et al. (2017) that SSI-2 is not involved in acid stress, the transcriptional response of 6179 to lactic acid stress showed a significant decrease in the transcription of the lin0464 homolog (−1.66 log2fold change) and no differential expression of the lin0465 homolog. R479a possesses SSI-1, a five-gene insert homologous to lmo0444–lmo0448. Previous results have shown that when all five genes of SSI-1 were deleted, L. monocytogenes growth in pH 4.8 media was significantly decreased (Ryan et al., 2010). However, only the homologs of lmo0444 and lmo0445 were differentially expressed in the R479a transcriptome following lactic acid stress with log2fold changes of 0.76 and 3.76, respectively.
Two other important acid stress response systems in L. monocytogenes are the glutamate decarboxylase (GAD) and arginine deiminase systems (Soni et al., 2011; Bucur et al., 2018). Three genes encoding GADs (lmo0447, lmo2363, and lmo2434) and two genes encoding antiporters (lmo0448 and lmo2362), are involved in the GAD pathway of EGD-e (Cotter et al., 2005). Notably, because 6179 lacks SSI-1, it lacks the homologs to lmo0447 and lmo0448 found in R479a. However, the homologs of lmo2363, lmo2434, and lmo2362 present in both 6179 and R479a were upregulated in both strains, with lmo2434 being upregulated by a log2fold change of 7.28 in 6179 and 8.08 in R479a. The results from this study are in line with the observation that lmo2363 and lmo2362 play a greater role than lmo0447 in acid-stress survival (Cotter et al., 2001; Ryan et al., 2009). The genes encoding the arginine deiminase operon ArcABC (lmo0036, lmo0037, lmo0039, and lmo0043), as well as the transcriptional regulator (lmo1367) (Ryan et al., 2009), were all significantly upregulated in both 6179 and in R479a. Thus, as expected, two of the well-defined acid stress response mechanisms were upregulated in response to 1% lactic acid exposure.
In both 6179 and R479a, high upregulation of mntABC (lmo1847, lmo1849, lmo1848), a set of genes encoding a putative manganese transporter, was observed; the log2fold expression change ranged from 7.93 to 8.32 in 6179 and from 6.65 to 9.89 in R479a (Supplementary Tables S7, S8). Functional characterization in EGD-e has demonstrated that mntA is involved in host-cell penetration and macrophage survival (Reglier-Poupet et al., 2003; Bierne and Cossart, 2007). Furthermore, another gene encoding an additional putative manganese transporter, mntH (lmo1424), was highly upregulated in both strains with log2fold changes of 5.65 in 6179 and 4.32 in R479a. The high upregulation of the manganese transporters mntABC and mntH suggest that manganese transporters may also be important for L. monocytogenes acid stress response in addition to their described contribution to oxidative stress response and virulence.
σB Genes
The sigma factor σB is an important regulator of stress and virulence genes in L. monocytogenes. Using data from three studies (Raengpradub et al., 2008; Oliver et al., 2009; Liu et al., 2019), we created a panel of 387 genes representing the σB regulon (Supplementary Table S9); of these genes, 6179 and R479a possessed homologs to 380 and 369, respectively (Figure 3). Following the exposure to lactic acid, each strain exhibited differential expression of 311 of the σB genes found in that strain. In contrast, far fewer σB genes were differentially expressed upon exposure of either strain to hydrogen peroxide. In the case of 6179 exposed to 0.01% hydrogen peroxide, only 29 σB genes were differentially expressed, and no DE genes in the R479a oxidative stress condition were members of the σB regulon (Figure 3). It should be noted that induction of a select number of σB-dependent genes was observed in L. monocytogenes following centrifugation before cold shock stress exposure (Chan et al., 2007). However, it is currently unknown how such an induction might affect the L. monocytogenes σB-dependent stress response as a whole or if this induction would also occur during acid or oxidative stress. Regardless, in the case of this study, a pre-induction of σB and genes in the σB regulon during centrifugation could have potentially “primed” L. monocytogenes for stress exposure. While the possibility of a pre-induction of σB following the initial centrifugation step cannot be ruled out, both the control and experimental treatment samples underwent this same centrifugation step, and therefore the differences between the control and treatment transcriptomes should largely be due to stress exposure. Thus, the overall pattern of σB regulon induction suggests that the immediate response of L. monocytogenes strains 6179 and R479a to lactic acid exposure is at least in part mediated by σB. In line with this, an upregulation of 45% of the genes from the σB regulon was recently described in a proteome study analyzing acid stress response in the L. monocytogenes strain Scott A (Bowman et al., 2012).
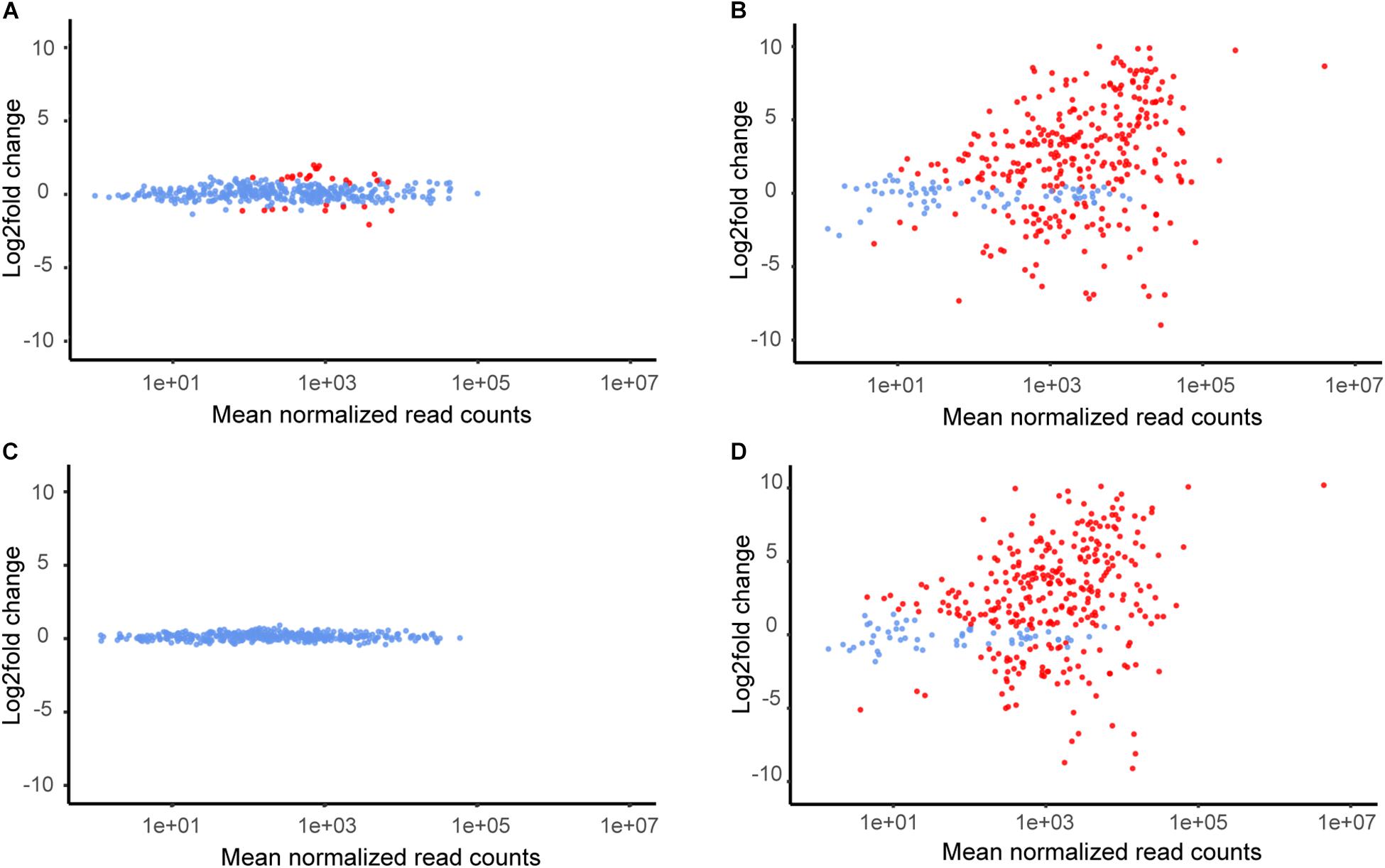
Figure 3. Mean of normalized read counts of all σB-regulated genes plotted against log2fold expression value changes for each gene. DE genes are colored red. (A) L. monocytogenes 6179 during H2O2 stress, (B) L. monocytogenes 6179 during lactic acid stress, (C) L. monocytogenes R479a during H2O2 stress, (D) L. monocytogenes R479a during lactic acid stress.
Members of the σB regulon also include a number of conserved hypothetical proteins which were differentially expressed in the experimental conditions. Additionally, many of these genes were also heavily upregulated. Following lactic acid stress, members of the σB regulon accounted for 108 and 122 DE genes encoding conserved hypothetical proteins in 6179 and R479a, respectively (the 50 most upregulated are shown in Supplementary Tables S10, S11). One of these genes, lmo2269 (LM6179_3049 and LMR479a_2383 in 6179 and R479a, respectively), was the DE gene with the third highest log2fold change in 6179 (9.60) and the sixth highest log2fold change in R479a (9.89). lmo2269 has been shown to be upregulated under heat stress in EGD-e (van der Veen et al., 2007). The numerous σB genes encoding conserved hypothetical proteins, their upregulation, and their high expression levels suggest that additional, uncharacterized σB-controlled genes may be important in the L. monocytogenes stress response. This is in line with the observation by Oliver et al. (2010) that over 30% of the σB-dependent genes shared by all strains in their study were uncharacterized, and many of them were upregulated in response to oxidative and acid stress.
Notably, the putative arsenate reductase lmo2230 (LM6179_3010 and LMR479a_2344 in 6179 and R479a, respectively) was highly upregulated in both strains during lactic acid stress. This gene was the fourth highest expressed gene in 6179 and the seventh highest expressed in R479a with log2fold increases of 9.50 in 6179 and 9.85 in R479a. Multiple studies have demonstrated that lmo2230 is a member of the σB regulon (Raengpradub et al., 2008; Oliver et al., 2009; Toledo-Arana et al., 2009; Utratna et al., 2011). These studies also showed that lmo2230 is upregulated during early stationary phase (Raengpradub et al., 2008; Oliver et al., 2009), within the murine intestinal lumen (Toledo-Arana et al., 2009) and in response to osmotic and acid stress (Raengpradub et al., 2008; Utratna et al., 2011; Horlbog et al., 2019). Heat and cold stress have also been shown to induce lmo2230 expression (van der Veen et al., 2007; Hingston et al., 2017a), and lmo2230 is also upregulated after chlorine dioxide exposure (Pleitner et al., 2014).
However, in contrast to the results from the lactic acid experiments in this study, lmo2230 expression was downregulated or only very weakly upregulated after exposure to the organic acid salts sodium diacetate and potassium lactate as well as to a combination of the two (Stasiewicz et al., 2011). Additionally, lmo2230 was differentially expressed in response to acid stress in brain-heart infusion broth adjusted to pH 5, but the log2fold changes were much lower (1.3–2.5) than seen in this study (Tessema et al., 2012). In addition to differences in experimental conditions, the differences in upregulation of lmo2230 could be explained by different timepoints, different L. monocytogenes strains, and/or the usage of a microarray compared to RNA sequencing. Although upregulation of lmo2230 in response to stress has been observed in numerous studies (van der Veen et al., 2007; Raengpradub et al., 2008; Oliver et al., 2009; Toledo-Arana et al., 2009; Utratna et al., 2011; Pleitner et al., 2014; Hingston et al., 2017a; Horlbog et al., 2019), its function in L. monocytogenes remains uncharacterized. The weak amino acid identity of Lmo2230 to the arsenate reductase ArsC from B. subtilis might suggest a possible role in heavy metal detoxification. However, it remains true that Lmo2230 lacks conserved amino acid residues essential for function as an arsenate reductase (Supplementary Figure S2), and it is upregulated under the aforementioned stress conditions which do not involve heavy metal (arsenate or arsenic) toxicity. Taken together, these factors indicate that Lmo2230 may have a broader, yet unknown, function in L. monocytogenes stress response.
Interestingly, an extremely high percentage of all transcriptome reads from the lactic acid replicates (averaging 28 and 38% for 6179 and R479a, respectively) mapped to the non-coding RNA rli47 (sbrE), a member of the σB regulon (Figure 4) (Oliver et al., 2009; Toledo-Arana et al., 2009; Mujahid et al., 2012; Liu et al., 2017). Expression of rli47 significantly increased during exposure to lactic acid, with a log2fold change of 8.47 in 6179 and 9.97 in R479a. Recently, Marinho et al. (2019) determined that rli47 transcripts hinder cell growth under stress conditions by suppressing translation of ilvA, an enzyme involved in isoleucine biosynthesis. Other studies also support the notion that rli47 is important for response to various stressors including stationary phase (Oliver et al., 2009; Toledo-Arana et al., 2009; Mujahid et al., 2012), intracellar survival in macrophages (Mraheil et al., 2011), and oxidative stress (Mujahid et al., 2012). Taken together, our data and published gene expression and functional characterization data indicate that rli47 likely plays a vital role in global stress response.
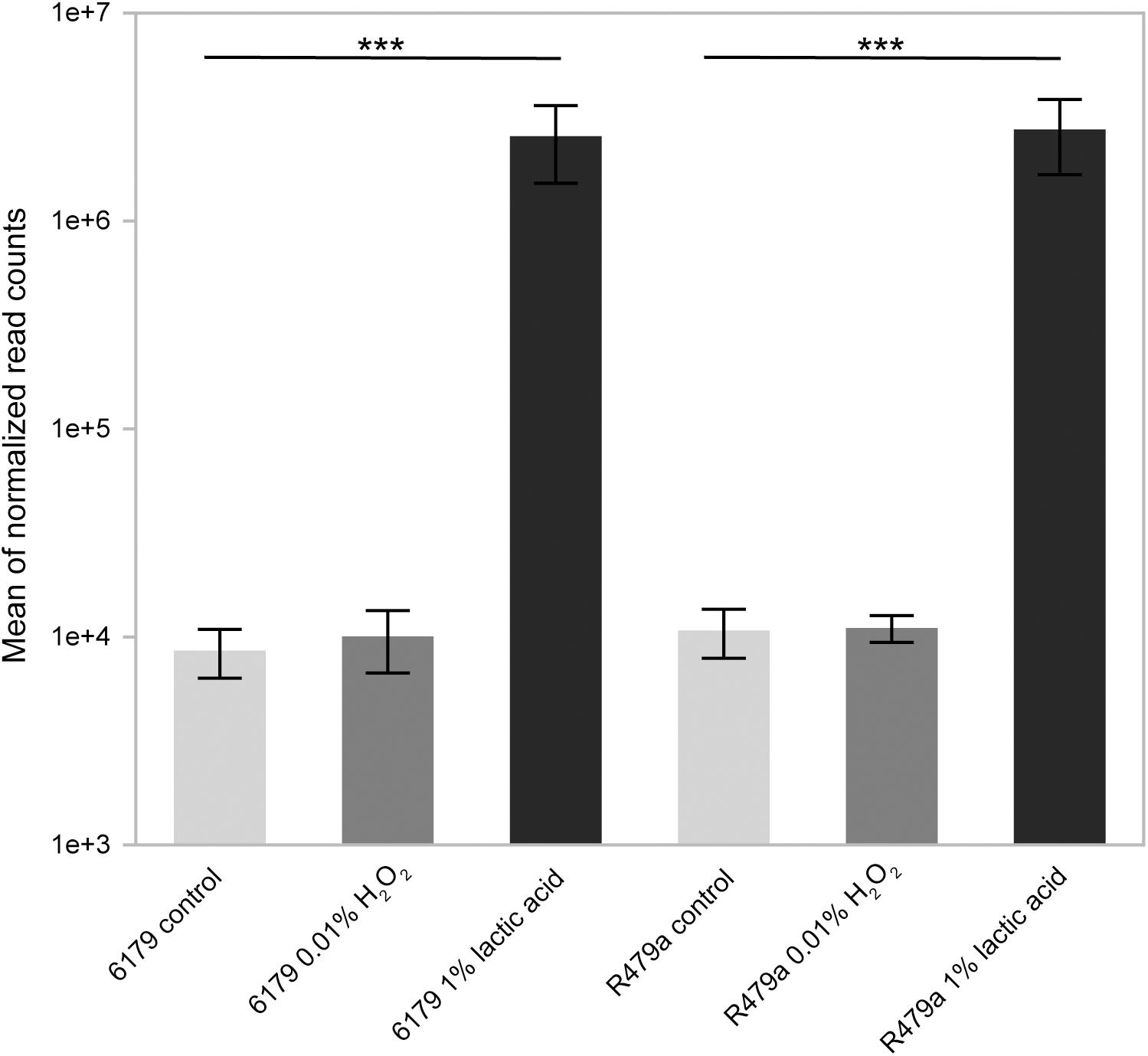
Figure 4. Average mean of normalized transcriptome sequencing reads mapped to the non-coding RNA Rli47 in L. monocytogenes strains 6179 and R479a exposed to control conditions, acid (1% lactic acid, pH 3.4), and oxidative stress (0.01% H2O2). Q-values (<0.001) are indicated by asterisks.
Here, we also observe a potential link between Rli47 and the Listeria adhesion protein (LAP, lmo1634). LAP is a bifunctional, highly conserved alcohol-acetaldehyde dehydrogenase which is essential in promoting the systemic spread of L. monocytogenes during infection (Pandiripally et al., 1999; Drolia et al., 2018). Previous research has demonstrated lap upregulation during nutrient starvation and low glucose (Jaradat and Bhunia, 2002), heme stress (Dos Santos et al., 2018), and oxygen limited environments (Burkholder et al., 2009). In our data, lap was significantly downregulated in both 6179 and R479a with log2fold changes of -5.10 and -2.99, respectively. Interestingly, differential expression analyses, including the one conducted in this study, consistently show a pattern of rli47 upregulation coupled with the concurrent downregulation of lap. This pattern is present during stationary phase (Oliver et al., 2009; Toledo-Arana et al., 2009; Mujahid et al., 2012), the overexpression of σB (Liu et al., 2017), multiplication in the gut (Toledo-Arana et al., 2009), and in macrophages (Chatterjee et al., 2006; Mraheil et al., 2011). Furthermore, lap was the highest upregulated gene (29.9-fold) in an rli47 deletion mutant (Marinho et al., 2019), suggesting that Rli47 is somehow involved in lap expression. The aforementioned evidence seems to implicate a relationship between LAP and Rli47 in L. monocytogenes, specifically that Rli47 is involved in the regulation of lap expression during stress conditions.
Plasmid Gene Expression
In addition to analyzing chromosomal gene expression patterns, we sought to provide first global insights into the differential expression of L. monocytogenes plasmid genes under stress conditions. Although the plasmids of 6179 and R479a share 46 genes, pLM6179 and pLMR479a harbor a number of strain-specific plasmid genes, some of which are predicted to be involved in stress response (Naditz et al., 2019). Total plasmid coverage for all 18 samples ranged from 41× to 170× with an average of 71× coverage. The average of total reads per sample mapped to pLM6179 and pLMR479a were 74,509 and 60,499, respectively (Table 1).
For both strains, there were far fewer transcriptional changes following the 30 min exposure to 0.01% hydrogen peroxide than to 1% lactic acid. After hydrogen peroxide treatment, analysis identified a single DE gene in the pLM6179 plasmid transcriptome, whereas no differential expression of any pLMR479a gene was found. In contrast, the 1% lactic acid treatment resulted in 42 DE genes for pLM6179 and 27 DE genes for pLMR479a. Of these, 18 and 13 were upregulated in the 6179 and R479a plasmid transcriptomes, respectively (Table 2 and Supplementary Tables S1, S2, S12, S13). For the lactic acid treatment, log2fold changes ranged from −4.70 to 3.15 for pLM6179 and from −6.14 to 3.84 for pLMR479a (Table 2 and Supplementary Tables S1, S2, S12, S13).
Plasmid Gene Expression Changes in Response to Hydrogen Peroxide Treatment
Interestingly, 0.01% hydrogen peroxide exposure resulted in no significant differences in plasmid gene expression between treatment and the control for R479a. In 6179, only one DE gene following 30 min exposure to 0.01% hydrogen peroxide was observed. This gene, LM6179_RS15385, was upregulated by a log2fold change of 1.11 and is annotated as encoding a conserved protein of unknown function. The minimal differences between the plasmid transcriptomes of the control and hydrogen peroxide treatments is in line with our chromosomal data and indicates either that 0.01% hydrogen peroxide exposure is not extremely stressful to 6179 or R479a or that the time of exposure to the stressor was insufficient to elicit major differential gene expression. It is interesting to note that our previous study showed a significant contribution of pLM6179 and pLMR479a to survival under 0.01% hydrogen peroxide, but the exposure to the stress was longer (2 h) in our previous study (Naditz et al., 2019). While not significant, the heat shock protein-encoding clpL gene of pLM6179 (LM6179_RS15400) was highly expressed in terms of read counts. An average of 47% of all reads mapped to pLM6179 mapped to clpL under hydrogen peroxide exposure.
Plasmid Gene Expression Changes in Response to 1% Lactic Acid Treatment
Although pLM6179 and pLMR479a are largely distinct, they nevertheless share 46 genes, and we observed some shared expression patterns between these two plasmids in response to lactic acid exposure. First, a membrane protein of unknown function (locus_tags: LM6179_RS15475, LMR479A_RS14845) was upregulated on both plasmids. Next, two genes found on the transposon Tn5422, cadA, and cadC, were downregulated in 6179 and R479a. These two genes encode a cadmium-transporting protein and accessory protein, respectively (Supplementary Tables S12, S13) (Lebrun et al., 1994). The gene encoding the putative plasmid replication protein repA was also downregulated, indicating a decrease in plasmid replication in response to the stress conditions.
In terms of DE genes unique to each strain, in pLM6179, the most highly upregulated and expressed DE gene was clpL, a gene found on pLM6179 but absent from pLMR479a. clpL was upregulated by a log2fold change of 3.15 (Supplementary Table S12). The ClpL protein is a member of the HSP100 subgroup of heat shock proteins, and the pLM6179 ClpL protein is identical to a functionally characterized ClpL protein harbored by the L. monocytogenes plasmid pLM58 that functions in heat stress response (Pöntinen et al., 2017). In other studies, ClpL proteins which share more than 67% amino acid identity to the protein encoded by the pLM6179 clpL gene have been shown to be involved in acid stress response, tolerance to detergents and penicillin, and long-term survival in different Streptococcus species (Kajfasz et al., 2009; Tran et al., 2011). A ClpL homolog in Lactobacillus reuteri sharing 67% amino acid identity to the pLM6179 ClpL was shown to be upregulated in response to acid stress (pH 2.7) (Wall et al., 2007). This, combined with the high expression level and high upregulation of clpL under lactic acid stress seen in this study, further supports the hypothesis that plasmid-borne clpL genes in L. monocytogenes play a role in acid stress response. Furthermore, if clpL is involved in environmental stress response, then this would at least partially explain the observed trend that plasmid-cured L. monocytogenes 6179 is less tolerant toward lactic acid, salt, and oxidative stress (Naditz et al., 2019). Further supporting the putative role for clpL in L. monocytogenes stress response, the upregulation of plasmid-encoded clpL during salt and acid stress was reported recently for two L. monocytogenes plasmids, pLMG1-12 and pLMG1-9 (Hingston et al., 2019). Other upregulated pLM6179 DE genes included mainly uncharacterized genes, a pair of putative toxin-antitoxin genes (LM6179_RS15455 and LM6179_RS15450), and a gene (LM6179_RS15380) found on many L. monocytogenes plasmids that is putatively involved in UV protection or DNA repair (Kuenne et al., 2010).
In response to 1% lactic acid treatment, the upregulated pLMR479a DE genes primarily consisted of genes encoding uncharacterized proteins for which no potential function can be inferred based on sequence similarity (Supplementary Table S13). However, a putative multicopper oxidase gene (LMR479a_RS15145) was also upregulated. The protein encoded by LMR479a_RS15145 shows more than 95% amino acid identity to a homolog in Staphylococcus aureus ATCC12600 which has been demonstrated to be involved in copper resistance and oxidative stress response (Sitthisak et al., 2005). However, the putative multicopper oxidases on pLMR479a and on other Listeria plasmids encode 386 amino acid proteins, which are shorter than the S. aureus homologs (462 amino acids). Recently, an identical homolog of the putative multicopper oxidase was also found to be upregulated under mild acid stress (pH 5) in the L. monocytogenes plasmid pLMG1-12 (Hingston et al., 2019).
Of significant interest was that the majority (65% on average) of the pLMR479a reads during lactic acid stress were mapped to an intergenic region (position 81657 to 81765) between locus_tags LMR479a_RS15240 and LMR479a_RS15245 (Supplementary Figure S3). Additionally, expression of this intergenic region was significantly upregulated by a log2fold change of 3.27. This region shows 56% nucleotide identity to the RFAM family RF02683, a recently described family of nickel–cobalt (NiCo) riboswitches known to be involved in transition metal tolerance (Furukawa et al., 2015). In Firmicutes, such as Clostridiales and Erysipelotrichaceae, these riboswitches bind to ions such as zinc, copper, nickel, and cobalt, and this binding activity is utilized in the detection of and response to toxic concentrations of transition metals (Furukawa et al., 2015). The putative riboswitch identified on pLMR479a shares 70% nucleotide identity and 90% percent coverage with both the functionally characterized Erysipelotrichaceae bacterium 3_1_53 NiCo riboswitch and the Clostridium scindens ATCC 35704 NiCo riboswitch (Furukawa et al., 2015; Supplementary Figures S4, S5). RNA secondary structural predictions revealed that the putative riboswitch from pLMR479a possessed a similar secondary structure to those of the functionally characterized NiCo riboswitches showing the four base-paired regions described by Furukawa et al. (2015) (Supplementary Figure S6).
Furukawa et al. (2015) also identified homologs of these NiCo riboswitches in Listeria. Interestingly, all homologs in Listeria identified by Furukawa et al. (2015) were found on plasmids, such as the homolog harbored on L. monocytogenes strain 08-5578 plasmid pLM5578. In contrast, the riboswitch homologs in Erysipelotrichaceae bacterium 3_1_53 and C. scindens are located on the chromosome. Notably, while the putative pLMR479a riboswitch shows high similarity to the characterized riboswitch homologs in Erysipelotrichaceae bacterium 3_1_53 and C. scindens, the transporter genes located upstream of these riboswitches show no similarity. All of the characterized NiCo riboswitches identified by Furukawa et al. (2015), including the putative Listeria riboswitches, are located upstream of putative heavy or transition metal transporters that correspond with the transition metal ions bound by the associated riboswitch. Similarly, the characterized riboswitches in Erysipelotrichaceae bacterium 3_1_53 and C. scindens are associated with a NiCo transporter. In pLMR479a, the putative riboswitch is located upstream of a putative zinc-transporter (LMR479a_RS15240), showing 41% amino acid identity to ZosA from B. subtilis, a zinc transporter important in oxidative stress response (Gaballa and Helmann, 2002). However, it should be noted that the zosA-like gene was not a DE gene under lactic acid stress applied here. The pLMR479a ZosA-like transporter is a 627 amino acid protein and belongs to the P-type ATPase superfamily 3.A.3 of the transporter classification database (Saier et al., 2016), whereas the transporters downstream of Erysipelotrichaceae bacterium 3_1_53 and C. scindens riboswitches are 308 amino acid residues in length and belong to the cation diffusion facilitator (CDF) family 2.A.4. This suggests that although transition metal-binding riboswitches may be similar, their associated transition metal transporters can be highly variable. BlastN searches against NCBI Genbank using the putative pLMR479a riboswitch as query revealed 44 identical homologs in different L. monocytogenes plasmids (Supplementary Table S14), suggesting that homologs of these putative riboswitches are found on a number of L. monocytogenes plasmids. Here, we provide the first gene expression data for this putative, plasmid-borne riboswitch in L. monocytogenes. This data shows that transcription of this riboswitch accounts for up to 73% of the transcripts of pLMR479a during 1% lactic acid stress. In the future, the potential function of this putative riboswitch in acid stress response and its regulation of the upstream ZosA-like transporter will need to be verified.
Conclusion
Here, we provide detailed insights into the short-term stress response of two L. monocytogenes strains, 6179 and R479a, to oxidative and acid stress. In particular, the lactic acid exposure resulted in striking changes in chromosomal gene expression. Our results provide more evidence for the importance of σB-regulated genes in stress response, including lmo2230 and its homologs, and show massive transcription of the non-coding RNA rli47. Additionally, our results demonstrate that the overall chromosomal responses to the aforementioned stress conditions are highly similar between 6179 and R479a. This study also provides the first in-depth analyses of plasmid gene expression in L. monocytogenes. Our results from two L. monocytogenes strains carrying distinct plasmids show that known and putative plasmid-encoded stress response genes such as clpL and the multi-copper oxidase are highly expressed and upregulated in response to stress conditions. We also reveal evidence in support of an important role of a putative, uncharacterized riboswitch in lactic acid stress response in pLMR479a based on its high expression level and significant upregulation during acid stress.
Data Availability Statement
The raw sequencing data were deposited at the NCBI Sequence Read Archive under Bioproject No. PRJNA563702.
Author Contributions
SS-E, BC, and AN were involved in study design and conceived the experiments. BC and AN performed the experiments. BC, AN, JA, and SS-E analyzed the data, wrote the manuscript, and approved the final manuscript.
Funding
SS-E, JA, and AN were supported by the USDA National Institute of Food and Agriculture Hatch Projects Nos. 1011114 and 1018898 and by the USDA National Institute of Food and Agriculture, Agricultural and Food Research Initiative Competitive Program, Grant Number: 2019-67017-29687. BC was supported by the National Science Foundation Graduate Research Fellowship Program. This material is based upon the work supported by the National Science Foundation Graduate Research Fellowship Program under Grant No. 1744592. Any opinions, findings, and conclusions or recommendations expressed in this material are those of the authors and do not necessarily reflect the views of the National Science Foundation.
Conflict of Interest
The authors declare that the research was conducted in the absence of any commercial or financial relationships that could be construed as a potential conflict of interest.
Supplementary Material
The Supplementary Material for this article can be found online at: https://www.frontiersin.org/articles/10.3389/fmicb.2019.03110/full#supplementary-material
References
Abram, F., Starr, E., Karatzas, K. A., Matlawska-Wasowska, K., Boyd, A., Wiedmann, M., et al. (2008). Identification of components of the sigma B regulon in Listeria monocytogenes that contribute to acid and salt tolerance. Appl. Environ. Microbiol. 74, 6848–6858. doi: 10.1128/AEM.00442-08
Allerberger, F., and Wagner, M. (2010). Listeriosis: a resurgent foodborne infection. Clin. Microbiol. Infect. 16, 16–23. doi: 10.1111/j.1469-0691.2009.03109.x
Benjamini, Y., and Hochberg, Y. (1995). Controlling the false discovery rate - a practical and powerful approach to multiple testing. J. R. Stat. Soc. Ser. B Stat. Methodol. 57, 289–300. doi: 10.1111/j.2517-6161.1995.tb02031.x
Bennett, M. S., Guan, Z., Laurberg, M., and Su, X. D. (2001). Bacillus subtilis arsenate reductase is structurally and functionally similar to low molecular weight protein tyrosine phosphatases. Proc. Natl. Acad. Sci. U.S.A. 98, 13577–13582. doi: 10.1073/pnas.241397198
Bierne, H., and Cossart, P. (2007). Listeria monocytogenes surface proteins: from genome predictions to function. Microbiol. Mol. Biol. Rev. 71, 377–397. doi: 10.1128/mmbr.00039-06
Bowman, J. P., Hages, E., Nilsson, R. E., Kocharunchitt, C., and Ross, T. (2012). Investigation of the Listeria monocytogenes Scott A acid tolerance response and associated physiological and phenotypic features via whole proteome analysis. J. Proteome Res. 11, 2409–2426. doi: 10.1021/pr201137c
Bucur, F. I., Grigore-Gurgu, L., Crauwels, P., Riedel, C. U., and Nicolau, A. I. (2018). Resistance of Listeria monocytogenes to stress conditions encountered in food and food processing environments. Front. Microbiol. 9:2700. doi: 10.3389/fmicb.2018.02700
Burkholder, K. M., Kim, K. P., Mishra, K. K., Medina, S., Hahm, B. K., Kim, H., et al. (2009). Expression of LAP, a SecA2-dependent secretory protein, is induced under anaerobic environment. Microb. Infect. 11, 859–867. doi: 10.1016/j.micinf.2009.05.006
Burns, N., James, C. E., and Harrison, E. (2015). Polylysogeny magnifies competitiveness of a bacterial pathogen in vivo. Evol. Appl. 8, 346–351. doi: 10.1111/eva.12243
Carpentier, B., and Cerf, O. (2011). Review - Persistence of Listeria monocytogenes in food industry equipment and premises. Int. J. Food Microbiol. 145, 1–8. doi: 10.1016/j.ijfoodmicro.2011.01.005
Casey, A., Fox, E. M., Schmitz-Esser, S., Coffey, A., Mcauliffe, O., and Jordan, K. (2014). Transcriptome analysis of Listeria monocytogenes exposed to biocide stress reveals a multi-system response involving cell wall synthesis, sugar uptake, and motility. Front. Microbiol. 5:68. doi: 10.3389/fmicb.2014.00068
Chan, Y. C., Boor, K. J., and Wiedmann, M. (2007). SigmaB-dependent and sigmaB-independent mechanisms contribute to transcription of Listeria monocytogenes cold stress genes during cold shock and cold growth. Appl. Environ. Microbiol. 73, 6019–6029. doi: 10.1128/aem.00714-07
Chatterjee, S. S., Hossain, H., Otten, S., Kuenne, C., Kuchmina, K., Machata, S., et al. (2006). Intracellular gene expression profile of Listeria monocytogenes. Infect. Immun. 74, 1323–1338. doi: 10.1128/iai.74.2.1323-1338.2006
Chaturongakul, S., Raengpradub, S., Wiedmann, M., and Boor, K. J. (2008). Modulation of stress and virulence in Listeria monocytogenes. Trends Microbiol. 16, 388–396. doi: 10.1016/j.tim.2008.05.006
Cotter, P. D., Gahan, C. G., and Hill, C. (2001). A glutamate decarboxylase system protects Listeria monocytogenes in gastric fluid. Mol. Microbiol. 40, 465–475. doi: 10.1046/j.1365-2958.2001.02398.x
Cotter, P. D., Ryan, S., Gahan, C. G., and Hill, C. (2005). Presence of GadD1 glutamate decarboxylase in selected Listeria monocytogenes strains is associated with an ability to grow at low pH. Appl. Environ. Microbiol. 71, 2832–2839. doi: 10.1128/aem.71.6.2832-2839.2005
Dos Santos, P. T., Larsen, P. T., Menendez-Gil, P., Lillebaek, E. M. S., and Kallipolitis, B. H. (2018). Listeria monocytogenes relies on the Heme-regulated transporter hrtAB to resist Heme toxicity and uses heme as a signal to induce Transcription of lmo1634, encoding Listeria Adhesion protein. Front. Microbiol. 9:3090. doi: 10.3389/fmicb.2018.03090
Drolia, R., Tenguria, S., Durkes, A. C., Turner, J. R., and Bhunia, A. K. (2018). Listeria adhesion protein induces intestinal epithelial barrier dysfunction for bacterial translocation. Cell Host. Microbe 23:470-484.e7.
Dutta, V., Elhanafi, D., and Kathariou, S. (2013). Conservation and distribution of the benzalkonium chloride resistance cassette bcrABC in Listeria monocytogenes. Appl. Environ. Microbiol. 79, 6067–6074. doi: 10.1128/AEM.01751-13
Edlin, G., Lin, L., and Bitner, R. (1977). Reproductive fitness of P1, P2, and Mu lysogens of Escherichia coli. J. Virol. 21, 560–564.
Fagerlund, A., Langsrud, S., Schirmer, B. C. T., Møretrø, T., and Heir, E. (2016). Genome analysis of Listeria monocytogenes sequence type 8 strains persisting in salmon and poultry processing environments and comparison with related strains. PLoS One 11:e0151117. doi: 10.1371/journal.pone.0151117
Ferreira, V., Wiedmann, M., Teixeira, P., and Stasiewicz, M. J. (2014). Listeria monocytogenes persistence in food-associated environments: epidemiology, strain characteristics, and implications for public health. J. Food Prot. 77, 150–170. doi: 10.4315/0362-028X.JFP-13-150
Fonnesbech Vogel, B., Huss, H. H., Ojeniyi, B., Ahrens, P., and Gram, L. (2001). Elucidation of Listeria monocytogenes contamination routes in cold-smoked salmon processing plants detected by DNA-based typing methods. Appl. Environ. Microbiol. 67, 2586–2595. doi: 10.1128/aem.67.6.2586-2595.2001
Fox, E., Hunt, K., O’brien, M., and Jordan, K. (2011). Listeria monocytogenes in Irish farmhouse cheese processing environments. Int. J. Food Microbiol. 145(Suppl. 1), S39–S45. doi: 10.1016/j.ijfoodmicro.2010.10.012
Fox, E. M., Leonard, N., and Jordan, K. (2011). Physiological and transcriptional characterization of persistent and nonpersistent Listeria monocytogenes isolates. Appl. Environ. Microbiol. 77, 6559–6569. doi: 10.1128/AEM.05529-11
Fuangthong, M., Atichartpongkul, S., Mongkolsuk, S., and Helmann, J. D. (2001). OhrR is a repressor of ohrA, a key organic hydroperoxide resistance determinant in Bacillus subtilis. J. Bacteriol. 183, 4134–4141. doi: 10.1128/jb.183.14.4134-4141.2001
Furukawa, K., Ramesh, A., Zhou, Z., Weinberg, Z., Vallery, T., Winkler, W. C., et al. (2015). Bacterial riboswitches cooperatively bind Ni(2+) or Co(2+) ions and control expression of heavy metal transporters. Mol. Cell 57, 1088–1098. doi: 10.1016/j.molcel.2015.02.009
Gaballa, A., Guariglia-Oropeza, V., Wiedmann, M., and Boor, K. J. (2019). Cross talk between SigB and PrfA in Listeria monocytogenes facilitates transitions between extra- and intracellular environments. Microbiol. Mol. Biol. Rev. 83:e00034-19.
Gaballa, A., and Helmann, J. D. (2002). A peroxide-induced zinc uptake system plays an important role in protection against oxidative stress in Bacillus subtilis. Mol. Microbiol. 45, 997–1005. doi: 10.1046/j.1365-2958.2002.03068.x
Hain, T., Ghai, R., Billion, A., Kuenne, C. T., Steinweg, C., Izar, B., et al. (2012). Comparative genomics and transcriptomics of lineages I. II, and III strains of Listeria monocytogenes. BMC Genomics 13:144. doi: 10.1186/1471-2164-13-144
Harter, E., Wagner, E. M., Zaiser, A., Halecker, S., Wagner, M., and Rychli, K. (2017). Stress survival islet 2, predominantly present in Listeria monocytogenes strains of sequence type 121, is involved in the alkaline and oxidative stress responses. Appl. Environ. Microbiol. 83:e00827-17. doi: 10.1128/AEM.00827-17
Hilker, R., Stadermann, K. B., Doppmeier, D., Kalinowski, J., Stoye, J., Straube, J., et al. (2014). ReadXplorer–visualization and analysis of mapped sequences. Bioinformatics 30, 2247–2254. doi: 10.1093/bioinformatics/btu205
Hingston, P., Brenner, T., Truelstrup Hansen, L., and Wang, S. (2019). Comparative analysis of Listeria monocytogenes plasmids and expression levels of plasmid-encoded genes during growth under salt and acid stress conditions. Toxins 11:426. doi: 10.3390/toxins11070426
Hingston, P., Chen, J., Allen, K., Truelstrup Hansen, L., and Wang, S. (2017a). Strand specific RNA-sequencing and membrane lipid profiling reveals growth phase-dependent cold stress response mechanisms in Listeria monocytogenes. PLoS One 12:e0180123. doi: 10.1371/journal.pone.0180123
Hingston, P., Chen, J., Dhillon, B. K., Laing, C., Bertelli, C., Gannon, V., et al. (2017b). Genotypes associated with Listeria monocytogenes isolates displaying impaired or enhanced tolerances to cold, salt, acid, or Desiccation stress. Front. Microbiol. 8:369. doi: 10.3389/fmicb.2017.00369
Hingston, P. A., Piercey, M. J., and Truelstrup Hansen, L. (2015). Genes associated with desiccation and Osmotic stress in Listeria monocytogenes as revealed by insertional Mutagenesis. Appl. Environ. Microbiol. 81, 5350–5362. doi: 10.1128/AEM.01134-15
Hofacker, I. L. (2003). Vienna RNA secondary structure server. Nucleic Acids Res. 31, 3429–3431. doi: 10.1093/nar/gkg599
Horlbog, J. A., Stevens, M. J. A., Stephan, R., and Guldimann, C. (2019). Global transcriptional response of three highly acid-tolerant field strains of Listeria monocytogenes to HCl stress. Microorganisms 7:455. doi: 10.3390/microorganisms7100455
Ivanek, R., Grohn, Y. T., and Wiedmann, M. (2004). The cost and benefit of Listeria monocytogenes food safety measures. Crit. Rev. Food Sci. Nutr. 44, 513–523.
Ivy, R. A., Wiedmann, M., and Boor, K. J. (2012). Listeria monocytogenes grown at 7 degrees C shows reduced acid survival and an altered transcriptional response to acid shock compared to L. monocytogenes grown at 37 degrees C. Appl. Environ. Microbiol. 78, 3824–3836. doi: 10.1128/AEM.00051-12
Jaradat, Z. W., and Bhunia, A. K. (2002). Glucose and nutrient concentrations affect the expression of a 104-kilodalton Listeria adhesion protein in Listeria monocytogenes. Appl. Environ. Microbiol. 68, 4876–4883. doi: 10.1128/aem.68.10.4876-4883.2002
Kajfasz, J. K., Martinez, A. R., Rivera-Ramos, I., Abranches, J., Koo, H., and Quivey, R. G. Jr., et al. (2009). Role of Clp proteins in expression of virulence properties of Streptococcus mutans. J. Bacteriol. 191, 2060–2068. doi: 10.1128/JB.01609-08
Katoh, K., Rozewicki, J., and Yamada, K. D. (2019). MAFFT online service: multiple sequence alignment, interactive sequence choice and visualization. Brief Bioinform. 20, 1160–1166. doi: 10.1093/bib/bbx108
Kuenne, C., Voget, S., Pischimarov, J., Oehm, S., Goesmann, A., Daniel, R., et al. (2010). Comparative analysis of plasmids in the genus Listeria. PLoS One 5:e12511. doi: 10.1371/journal.pone.0012511
Lebrun, M., Audurier, A., and Cossart, P. (1994). Plasmid-borne cadmium resistance genes in Listeria monocytogenes are similar to cada and cadc of Staphylococcus aureus and are induced by cadmium. J. Bacteriol. 176, 3040–3048. doi: 10.1128/jb.176.10.3040-3048.1994
Li, H., and Durbin, R. (2010). Fast and accurate long-read alignment with Burrows-Wheeler transform. Bioinformatics 26, 589–595. doi: 10.1093/bioinformatics/btp698
Liu, Y., Orsi, R. H., Boor, K. J., Wiedmann, M., and Guariglia-Oropeza, V. (2017). Home alone: elimination of all but one alternative sigma factor in Listeria monocytogenes allows prediction of new roles for σB. Front. Microbiol. 8:1910. doi: 10.3389/fmicb.2017.01910
Liu, Y., Orsi, R. H., Gaballa, A., Wiedmann, M., Boor, K. J., and Guariglia-Oropeza, V. (2019). Systematic review of the Listeria monocytogenes sigma(B) regulon supports a role in stress response, virulence and metabolism. Future Microbiol. 14, 801–828. doi: 10.2217/fmb-2019-0072
Love, M. I., Huber, W., and Anders, S. (2014). Moderated estimation of fold change and dispersion for RNA-seq data with DESeq2. Genome Biol. 15:550.
Makariti, I. P., Printezi, A., Kapetanakou, A. E., Zeaki, N., and Skandamis, P. N. (2015). Investigating boundaries of survival, growth and expression of genes associated with stress and virulence of Listeria monocytogenes in response to acid and osmotic stress. Food Microbiol. 45, 231–244. doi: 10.1016/j.fm.2014.06.023
Marinho, C. M., Dos Santos, P. T., Kallipolitis, B. H., Johansson, J., Ignatov, D., Guerreiro, D. N., et al. (2019). The sigma(B)-dependent regulatory sRNA Rli47 represses isoleucine biosynthesis in Listeria monocytogenes through a direct interaction with the ilvA transcript. RNA Biol. 16, 1424–1437. doi: 10.1080/15476286.2019.1632776
Mittal, M., Picossi, S., and Sonenshein, A. L. (2009). CcpC-dependent regulation of citrate synthase gene expression in Listeria monocytogenes. J. Bacteriol. 191, 862–872. doi: 10.1128/JB.01384-08
Mraheil, M. A., Billion, A., Mohamed, W., Mukherjee, K., Kuenne, C., Pischimarov, J., et al. (2011). The intracellular sRNA transcriptome of Listeria monocytogenes during growth in macrophages. Nucleic Acids Res. 39, 4235–4248. doi: 10.1093/nar/gkr033
Mujahid, S., Bergholz, T. M., Oliver, H. F., Boor, K. J., and Wiedmann, M. (2012). Exploration of the role of the non-coding RNA SbrE in L. monocytogenes stress response. Int. J. Mol. Sci. 14, 378–393. doi: 10.3390/ijms14010378
Müller, A., Rychli, K., Muhterem-Uyar, M., Zaiser, A., Stessl, B., Guinane, C. M., et al. (2013). Tn6188 - a novel transposon in Listeria monocytogenes responsible for tolerance to benzalkonium chloride. PLoS One 8:e76835. doi: 10.1371/journal.pone.0076835
Müller, A., Rychli, K., Zaiser, A., Wieser, C., Wagner, M., and Schmitz-Esser, S. (2014). The Listeria monocytogenes transposon Tn6188 provides increased tolerance to various quaternary ammonium compounds and ethidium bromide. FEMS Microbiol. Lett. 361, 166–173. doi: 10.1111/1574-6968.12626
Naditz, A. L., Dzieciol, M., Wagner, M., and Schmitz-Esser, S. (2019). Plasmids contribute to food processing environment-associated stress survival in three Listeria monocytogenes ST121. ST8, and ST5 strains. Int. J. Food Microbiol. 299, 39–46. doi: 10.1016/j.ijfoodmicro.2019.03.016
Oliver, H. F., Orsi, R. H., Ponnala, L., Keich, U., Wang, W., Sun, Q., et al. (2009). Deep RNA sequencing of L. monocytogenes reveals overlapping and extensive stationary phase and sigma B-dependent transcriptomes, including multiple highly transcribed noncoding RNAs. BMC Genomics 10:641. doi: 10.1186/1471-2164-10-641
Oliver, H. F., Orsi, R. H., Wiedmann, M., and Boor, K. J. (2010). Listeria monocytogenes sigmaB has a small core regulon and a conserved role in virulence but makes differential contributions to stress tolerance across a diverse collection of strains. Appl. Environ. Microbiol. 76, 4216–4232. doi: 10.1128/aem.00031-10
Pandiripally, V. K., Westbrook, D. G., Sunki, G. R., and Bhunia, A. K. (1999). Surface protein p104 is involved in adhesion of Listeria monocytogenes to human intestinal cell line. Caco-2. J. Med. Microbiol. 48, 117–124. doi: 10.1099/00222615-48-2-117
Pleitner, A. M., Trinetta, V., Morgan, M. T., Linton, R. L., and Oliver, H. F. (2014). Transcriptional and phenotypic responses of Listeria monocytogenes to chlorine dioxide. Appl. Environ. Microbiol. 80, 2951–2963. doi: 10.1128/AEM.00004-14
Pöntinen, A., Aalto-Araneda, M., Lindstrom, M., and Korkeala, H. (2017). Heat resistance mediated by pLM58 plasmid-borne ClpL in Listeria monocytogenes. mSphere 2:e364-17.
R Development Core Team, (2018). R: A Language and Environment for Statistical Computing. Vienna: R Foundation for Stastical Computing.
Raengpradub, S., Wiedmann, M., and Boor, K. J. (2008). Comparative analysis of the sigma B-dependent stress responses in Listeria monocytogenes and Listeria innocua strains exposed to selected stress conditions. Appl. Environ. Microbiol. 74, 158–171. doi: 10.1128/aem.00951-07
Reglier-Poupet, H., Pellegrini, E., Charbit, A., and Berche, P. (2003). Identification of LpeA, a PsaA-like membrane protein that promotes cell entry by Listeria monocytogenes. Infect. Immun. 71, 474–482. doi: 10.1128/iai.71.1.474-482.2003
Reniere, M. L., Whiteley, A. T., and Portnoy, D. A. (2016). An in vivo selection identifies Listeria monocytogenes genes required to sense the intracellular environment and activate virulence factor expression. PLoS Pathog. 12:e1005741. doi: 10.1371/journal.ppat.1005741
Rossmanith, P., Krassnig, M., Wagner, M., and Hein, I. (2006). Detection of Listeria monocytogenes in food using a combined enrichment/real-time PCR method targeting the prfA gene. Res. Microbiol. 157, 763–771. doi: 10.1016/j.resmic.2006.03.003
Ryan, S., Begley, M., Gahan, C. G., and Hill, C. (2009). Molecular characterization of the arginine deiminase system in Listeria monocytogenes: regulation and role in acid tolerance. Environ. Microbiol. 11, 432–445. doi: 10.1111/j.1462-2920.2008.01782.x
Ryan, S., Begley, M., Hill, C., and Gahan, C. G. M. (2010). A five-gene stress survival islet (SSI-1) that contributes to the growth of Listeria monocytogenes in suboptimal conditions. J. Appl. Microbiol. 109, 984–995. doi: 10.1111/j.1365-2672.2010.04726.x
Rychli, K., Grunert, T., Ciolacu, L., Zaiser, A., Razzazi-Fazeli, E., Schmitz-Esser, S., et al. (2016). Exoproteome analysis reveals higher abundance of proteins linked to alkaline stress in persistent Listeria monocytogenes strains. Int. J. Food Microbiol. 218, 17–26. doi: 10.1016/j.ijfoodmicro.2015.11.002
Saier, M. H. Jr., Reddy, V. S., Tsu, B. V., Ahmed, M. S., Li, C., and Moreno-Hagelsieb, G. (2016). The transporter classification database (TCDB): recent advances. Nucleic Acids Res. 44, D372–D379. doi: 10.1093/nar/gkv1103
Sato, T., and Kobayashi, Y. (1998). The ars operon in the skin element of Bacillus subtilis confers resistance to arsenate and arsenite. J. Bacteriol. 180, 1655–1661.
Scallan, E., Griffin, P. M., Angulo, F. J., Tauxe, R. V., and Hoekstra, R. M. (2011). Foodborne illness acquired in the united states-unspecified agents. Emerg. Infect. Dis. 17, 16–22. doi: 10.3201/eid1701.091101p2
Schmitz-Esser, S., Gram, L., and Wagner, M. (2015a). Complete genome sequence of the persistent Listeria monocytogenes strain R479a. Genome Announc. 3, e150–e115. doi: 10.1128/genomeA.00150-15
Schmitz-Esser, S., Muller, A., Stessl, B., and Wagner, M. (2015b). Genomes of sequence type 121 Listeria monocytogenes strains harbor highly conserved plasmids and prophages. Front. Microbiol. 6:380. doi: 10.3389/fmicb.2015.00380
Schultze, T., Hilker, R., Mannala, G. K., Gentil, K., Weigel, M., Farmani, N., et al. (2015). A detailed view of the intracellular transcriptome of Listeria monocytogenes in murine macrophages using RNA-seq. Front. Microbiol. 6:1199. doi: 10.3389/fmicb.2015.01199
Sitthisak, S., Howieson, K., Amezola, C., and Jayaswal, R. K. (2005). Characterization of a multicopper oxidase gene from Staphylococcus aureus. Appl. Environ. Microbiol. 71, 5650–5653. doi: 10.1128/aem.71.9.5650-5653.2005
Sleator, R. D., Wemekamp-Kamphuis, H. H., Gahan, C. G., Abee, T., and Hill, C. (2005). A PrfA-regulated bile exclusion system (BilE) is a novel virulence factor in Listeria monocytogenes. Mol. Microbiol. 55, 1183–1195. doi: 10.1111/j.1365-2958.2004.04454.x
Soni, K. A., Nannapaneni, R., and Tasara, T. (2011). The contribution of transcriptomic and proteomic analysis in elucidating stress adaptation responses of Listeria monocytogenes. Foodborne Pathog. Dis. 8, 843–852. doi: 10.1089/fpd.2010.0746
Stasiewicz, M. J., Wiedmann, M., and Bergholz, T. M. (2011). The transcriptional response of Listeria monocytogenes during adaptation to growth on lactate and diacetate includes synergistic changes that increase fermentative acetoin production. Appl. Environ. Microbiol. 77, 5294–5306. doi: 10.1128/AEM.02976-10
Tang, S., Orsi, R. H., Den Bakker, H. C., Wiedmann, M., Boor, K. J., and Bergholz, T. M. (2015). transcriptomic analysis of the adaptation of Listeria monocytogenes to growth on vacuum-packed cold smoked salmon. Appl. Environ. Microbiol. 81, 6812–6824. doi: 10.1128/AEM.01752-15
Tessema, G. T., Moretro, T., Snipen, L., Heir, E., Holck, A., Naterstad, K., et al. (2012). Microarray-based transcriptome of Listeria monocytogenes adapted to sublethal concentrations of acetic acid, lactic acid, and hydrochloric acid. Can. J. Microbiol. 58, 1112–1123. doi: 10.1139/w2012-091
Thomas, M. K., Vriezen, R., Farber, J. M., Currie, A., Schlech, W., and Fazil, A. (2015). Economic cost of a Listeria monocytogenes outbreak in Canada, 2008. Foodborne Pathog. Dis. 12, 966–971. doi: 10.1089/fpd.2015.1965
Toledo-Arana, A., Dussurget, O., Nikitas, G., Sesto, N., Guet-Revillet, H., Balestrino, D., et al. (2009). The Listeria transcriptional landscape from saprophytism to virulence. Nature 459, 950–956. doi: 10.1038/nature08080
Tran, T. D., Kwon, H. Y., Kim, E. H., Kim, K. W., Briles, D. E., Pyo, S., et al. (2011). Decrease in penicillin susceptibility due to heat shock protein ClpL in Streptococcus pneumoniae. Antimicrob. Agents Chemother. 55, 2714–2728. doi: 10.1128/AAC.01383-10
Utratna, M., Shaw, I., Starr, E., and O’byrne, C. P. (2011). Rapid, transient, and proportional activation of sigma(B) in response to osmotic stress in Listeria monocytogenes. Appl. Environ. Microbiol. 77, 7841–7845. doi: 10.1128/AEM.05732-11
van der Veen, S., Hain, T., Wouters, J. A., Hossain, H., De Vos, W. M., Abee, T., et al. (2007). The heat-shock response of Listeria monocytogenes comprises genes involved in heat shock, cell division, cell wall synthesis, and the SOS response. Microbiology 153, 3593–3607. doi: 10.1099/mic.0.2007/006361-0
Verghese, B., Lok, M., Wen, J., Alessandria, V., Chen, Y., Kathariou, S., et al. (2011). comK prophage junction fragments as markers for Listeria monocytogenes genotypes unique to individual meat and poultry processing plants and a model for rapid niche-specific adaptation, biofilm formation, and persistence. Appl. Environ. Microbiol. 77, 3279–3292. doi: 10.1128/AEM.00546-11
Volker, U., Engelmann, S., Maul, B., Riethdorf, S., Volker, A., Schmid, R., et al. (1994). Analysis of the induction of general stress proteins of Bacillus subtilis. Microbiology 140(Pt 4), 741–752. doi: 10.1099/00221287-140-4-741
Wall, T., Bath, K., Britton, R. A., Jonsson, H., Versalovic, J., and Roos, S. (2007). The early response to acid shock in Lactobacillus reuteri involves the ClpL chaperone and a putative cell wall-altering esterase. Appl. Environ. Microbiol. 73, 3924–3935. doi: 10.1128/aem.01502-06
Wang, X., Kim, Y., Ma, Q., Hong, S. H., Pokusaeva, K., Sturino, J. M., et al. (2010). Cryptic prophages help bacteria cope with adverse environments. Nat. Commun. 1:147. doi: 10.1038/ncomms1146
Keywords: Listeria monocytogenes, oxidative stress, lactic acid stress, plasmid, transcriptome, non-coding RNA, Rli47
Citation: Cortes BW, Naditz AL, Anast JM and Schmitz-Esser S (2020) Transcriptome Sequencing of Listeria monocytogenes Reveals Major Gene Expression Changes in Response to Lactic Acid Stress Exposure but a Less Pronounced Response to Oxidative Stress. Front. Microbiol. 10:3110. doi: 10.3389/fmicb.2019.03110
Received: 09 October 2019; Accepted: 23 December 2019;
Published: 21 January 2020.
Edited by:
Si Hong Park, Oregon State University, United StatesReviewed by:
Teresa M. Bergholz, North Dakota State University, United StatesVeronica Guariglia-Oropeza, Cornell University, United States
Copyright © 2020 Cortes, Naditz, Anast and Schmitz-Esser. This is an open-access article distributed under the terms of the Creative Commons Attribution License (CC BY). The use, distribution or reproduction in other forums is permitted, provided the original author(s) and the copyright owner(s) are credited and that the original publication in this journal is cited, in accordance with accepted academic practice. No use, distribution or reproduction is permitted which does not comply with these terms.
*Correspondence: Stephan Schmitz-Esser, sse@iastate.edu
†These authors have contributed equally to this work