- 1Novo Nordisk Foundation Center for Biosustainability, Technical University of Denmark, Lyngby, Denmark
- 2Genomic and Applied Microbiology & Göttingen Genomics Laboratory, Georg-August University, Göttingen, Germany
- 3Institut für Mikrobiologie und Biotechnologie, Universität Ulm, Ulm, Germany
- 4Leibniz-Institut DSMZ-Deutsche Sammlung von Mikroorganismen und Zellkulturen GmbH, Brunswick, Germany
Fermentation of gases provides a promising opportunity for the production of biochemicals from renewable resources, which has resulted in a growing interest in acetogenic bacteria. Thermophilic organisms provide potential advantages for the fermentation of, e.g., syngas into for example volatile compounds, and the thermophiles Moorella thermoacetica and Moorella thermoautotrophica have become model organisms of acetogenic metabolism. The justification for the recognition of the closely related species M. thermoautotrophica has, however, recently been disputed. In order to expand knowledge on the genus, we have here genome sequenced a total of 12 different M. thermoacetica and M. thermoautotrophica strains. From the sequencing results, it became clear that M. thermoautotrophica DSM 1974T consists of at least two different strains. Two different strains were isolated in Lyngby and Ulm from a DSM 1974T culture obtained from the DSMZ (Leibniz-Institut DSMZ-Deutsche Sammlung von Mikroorganismen und Zellkulturen GmbH, Brunswick, Germany). Phylogenetic analysis revealed a close relationship between all the sequenced genomes, suggesting that the two strains detected in the type strain of the species M. thermoautotrophica could not be distinguished at the species level from M. thermoacetica. Despite genetic similarities, differences in genomic features were observed between the strains. Differences in compounds that can serve as carbon and energy sources for selected strains were also identified. On the contrary, strain DSM 21394, currently still named M. thermoacetica, obviously represents a new Moorella species. In addition, based on genome analysis and comparison M. glycerini NMP, M. stamsii DSM 26217T, and M. perchloratireducens An10 cannot be distinguished at the species level. Thus, this comprehensive analysis provides a significantly increased knowledge of the genetic diversity of Moorella strains.
Introduction
Interest from the research community and industry in acetogenic bacteria has grown within recent years due to their potential to produce valuable compounds from syngas (Latif et al., 2014). Thermophilic acetogens are of significance, since their use would reduce gas cooling requirements, allow for cost-efficient recovery of products with relatively low boiling point (Henstra et al., 2007; Redl et al., 2017), and decrease the risk of contamination.
A well-studied syngas-fermenting thermophile is Moorella thermoacetica. The species was isolated from horse feces in 1942 and named Clostridium thermoaceticum (Fontaine et al., 1942). The taxonomy of the genus Clostridium was restructured in 1994 and C. thermoaceticum was transferred to a new genus Moorella as M. thermoacetica (Collins et al., 1994). Several strains originating from the cultures isolated by Fontaine et al. (1942) are deposited in strain collections. The type strain DSM 521T and the strain ATCC 39073 have primarily served to elucidate the primary metabolism of M. thermoacetica (synonym C. thermoaceticum): they were used in experiments to study carbohydrate utilization (Andreesen et al., 1973), the acetate kinase (Schaupp and Ljungdahl, 1974), cytochromes and menaquinones (Gottwald et al., 1975), the formate dehydrogenase (Ljungdahl and Andreesen, 1977), and the utilization of CO (Diekert and Thauer, 1978). The genome of the non-type strain ATCC 39073 was sequenced in 2008 (Pierce et al., 2008) and the genome sequence of the type strain DSM 521T followed in 2015 (Poehlein et al., 2015). A spore sample of the original M. thermoacetica strain isolated in 1942 was deposited by Kerby and Zeikus (1983) as a second representative of the type strain (DSM 2955T) in the DSMZ (Leibniz-Institut DSMZ-Deutsche Sammlung von Mikroorganismen und Zellkulturen GmbH, Brunswick, Germany). It was shown to utilize H2/CO2 as substrate and was also adapted to growth on CO (Kerby and Zeikus, 1983). The ability to utilize gaseous substrates was not shown for ATCC 39073 and DSM 521T until 1990 (Daniel et al., 1990). Another M. thermoacetica strain (Y72) with higher transformation efficiency than ATCC 39073 was described and its draft genome published in 2014 (Tsukahara et al., 2014).
Wiegel et al. (1981) described the isolation of strains closely related to the already known C. thermoaceticum (M. thermoacetica) strains. The novel strains were shown to grow chemolithotrophically on H2/CO2 and chemoheterotrophically on several carbon sources. At that time, the aforementioned strains of C. thermoaceticum (M. thermoacetica) were not known to utilize H2/CO2 and CO. Furthermore, Wiegel et al. (1981) described differences in the cell shape in comparison to M. thermoacetica. In addition to C. aceticum and Acetobacterium woodii, this new strain was the third species known to grow autotrophically using H2 and CO2 while producing acetate. Therefore, a new species was proposed and a strain isolated from a Yellowstone hot spring (strain JW 701/3) was deposited as Clostridium thermoautotrophicum DSM 1974T (Wiegel et al., 1981). C. thermoautotrophicum was later re-classified as Moorella thermoautotrophica in the extensive study of Collins et al. (1994). In addition to M. thermoautotrophica DSM 1974T, which is the designated type strain, a second M. thermoautotrophica strain, DSM 7417, is available. This strain (DSM 7417) was first described in Rijssel et al. (1992) when it appeared as a contamination in a continuous culture. The authors based their decision to place the newly described strain in the species of M. thermoautotrophica instead of M. thermoacetica mainly on observations regarding the cell shape (Rijssel et al., 1992). Recently, Kimura et al. (2016) requested an opinion regarding the taxonomic status of M. thermoautotrophica. Based on DNA–DNA hybridization experiments and 16S rRNA gene sequence analysis, Kimura et al. (2016) concluded that the species M. thermoautotrophica should be reclassified as M. thermoacetica. Over time, phenotypic differences between M. thermoacetica and M. thermoautotrophica were described, but often with partly conflicting results (Cato et al., 1986; Das et al., 1989; Yamamoto et al., 1998; Carlier and Bedora-Faure, 2006).
Here, we report that M. thermoautotrophica DSM 1974T is a mixed culture of at least two strains, which we isolated. We sequenced the genome of those two strains as well as the genome of DSM 7417 and nine other M. thermoacetica strains, thereby considerably adding to the genomic information of this group of bacteria. We compared the genomes of the strains with the genome of the M. thermoacetica strain ATCC 39073 (Pierce et al., 2008) and the type strains DSM 2955T (Bengelsdorf et al., 2015) and DSM 521T (Poehlein et al., 2015). In addition, we performed genome comparison with all other genomes of the genus Moorella. Furthermore, differences in carbon utilization of the aforementioned strains were characterized. Based on this study, we conclude that the classification of the two strains isolated from DSM 1974T as a separate species, M. thermoautotrophica, is not justified and that based on the data collected both strains should be reclassified as strains of the species M. thermoacetica. However, a problem arises due to the fact that the designated type strain deposited in the DSMZ, as DSM 1974T, appears to be a mixture of two strains. The implications of these findings within the context of the rules of the International Code of Nomenclature (Parker et al., 2019) together with the content of the recent Request for an Opinion of Kimura et al. (2016) are discussed.
Materials and Methods
Strains
The strains DSM 521T, DSM 2955T, DSM 7417, DSM 21394, DSM 11768, DSM 12797, DSM 12993, DSM 6867, and DSM 11254T were purchased from DSMZ (Leibniz-Institut DSMZ-Deutsche Sammlung von Mikroorganismen und Zellkulturen GmbH, Brunswick, Germany). The strains isolated from the culture of DSM 1974T obtained from the DSMZ were deposited at the DSMZ with the numbers DSM 103284 (DSM 1974-Ulm) and DSM 103132 (DSM 1974-HH). Strain ATCC 39073 was purchased from the ATCC (Manassas, VA, United States) and was maintained by a series of transfers (here labeled as ATCC 39073-HH). Prior to extracting DNA for genome sequencing, a single colony was isolated on solid medium.
Cultivation
Strains were cultivated in 50-ml serum bottles (50% filled) closed with butyl rubber stoppers (bottles and stoppers: Ochs, Germany) containing a magnetic stirring bar and medium with the following composition (in g/l) [13]: KH2PO4 (0.5); NH4Cl (0.4); NaCl (0.4); NaHCO3 (3.5); yeast extract (0.5); 1% trace element solution was added to the medium. The trace element solution was prepared with 2 g/l nitrilotriacetic acid; the pH adjusted to 6.0 with KOH, and the following compounds added (in mg/l): MnSO4⋅H2O (1000); Fe(SO4)2(NH4)2⋅6 H2O (800); CoCl2⋅6 H2O (200); ZnSO4⋅7 H2O (200); CuCl2⋅2 H2O (20); NiCl2⋅6 H2O (20); Na2MoO4⋅2 H2O (20); Na2SeO4 (20); Na2WO4 (20) mg. The pH of the culture medium was adjusted to 6.5, flushed with N2:CO2 (80:20) and autoclaved at 140°C for 40 min. Solid medium contained 1% GelzanTM and the medium was sterilized at 120°C for 20 min. The following sterile stock solutions were added after autoclaving: CaCl2 (50 mg/l final), MgCl2 (330 mg/l final), vitamin solution (1%), cysteine-HCl (1 mM final). The vitamin solution contained (mg/l): biotin (2); folic acid (2); pyridoxine-HCl (10); thiamine HCl (5); riboflavin (5); nicotinic acid (5); calcium D-(+)-pantothenate (5); vitamin B12 (0.5); p-aminobenzoic acid (5); thioctic acid (5). The medium was pre-warmed before inoculation. The strains were cultivated at 60°C with stirring at 350 rpm. Fructose as carbon and energy source was added at a final concentration of 60 mM to the medium. The headspace was pressurized with N2:CO2 (80:20) to 3 bar. When gases served as carbon and energy sources, the headspace was flushed for several minutes before inoculation with the gas mixture, and the headspace pressurized to 3 bar after inoculation. H2:CO2 (80:20) served as gaseous substrates. Strain DSM 103132 was isolated from DSM 1974T using the medium described above solidified with 1% GelzanTM and using 60 mM fructose as the substrate. Strain DSM 103284 was isolated from DSM 1974T using the DSMZ medium 135, the solid medium contained 1.5% agar. In both cases, single colonies were picked and used for further cultivation.
Extraction of Genomic DNA
Cultures in mid-exponential phase were sampled, the cells were spun down, and DNA was extracted using the Wizard® Genomic DNA Purification Kit (Promega, Madison, WI, United States) and the MasterPureTM Gram Positive DNA Purification Kit (Epicentre, Madison, WI, United States) according to the manufacturer’s protocol. DNA was quantified using the Qubit dsDNA HS Assay Kit with the Qubit 2.0 fluorometer (Thermo Fisher Scientific, Waltham, MA, United States).
Genome Sequencing
ATCC 39073-HH and DSM 103132 were sequenced using a PacBio RSII instrument (Pacific Biosciences, Menlo Park, CA, United States). SMRTbellsTM libraries were constructed and sequenced following the recommended Pacific Biosciences template preparation protocol. Following SMRTbellTM construction, v2 primers and P4 polymerase were annealed and enzyme bound complexes attached to magnetic beads for loading. Each SMRTbellTM library was loaded onto a SMRT cell and sequenced on the PacBio RSII. The average reference coverage was above 500 for both strains, resulting from 129,760 and 134,994 reads of ATCC 39073-HH and DSM 103132, respectively, with an average read length of approximately 12,000 bp. Isolated DNA from all remaining strains was used to generate Illumina shotgun sequencing libraries. Sequencing was performed by employing a MiSeq system using MiSeq Reagent Kit v3 (600 cycles), as recommended by the manufacturer (Illumina, San Diego, CA, United States), resulting in 2 × 300 bp paired end reads. Strain DSM 103284 was sequenced with the Genome Analyzer IIx (Illumina, San Diego, CA, United States) resulting in 2 × 112 bp paired end reads. Quality filtering of the raw reads was done using Trimmomatic version 0.32 (Bolger et al., 2014). The de novo assembly was performed with the SPAdes genome assembler software (Bankevich et al., 2012). The assembly was validated and the read coverage determined with QualiMap (García-Alcalde et al., 2012). For scaffolding the contigs of strain DSM 103284, we used the Move Contigs tool of the Mauve Genome Alignment Software (Darling et al., 2010). Additionally, contigs that could not be ordered with Mauve were examined via Gene Ortholog Neighborhoods based on bidirectional best hits implemented at the IMG-ER (Integrated Microbial Genomes-Expert Review) system (Markowitz et al., 2013). For contig ordering, the genomes of M. thermoacetica DSM 521T (CP012369) and DSM 2955T (CP012370) were used as references. Sequence gaps were closed by PCR-based techniques and primer walking with conventional Sanger sequencing, using BigDye 3.0 chemistry on an ABI3730XL capillary sequencer (Applied Biosystems, Life Technologies GmbH, Darmstadt, Germany), and employing the Gap4 (v.4.11) software of the Staden Package (Staden et al., 1999). M. glycerini DSM 11254T has been sequenced using a combined approach with Illumina short read and Oxford Nanopore long read technology. Therefore, high molecular weight DNA (HWD) was isolated with the MasterPure Complete DNA & RNA Purification Kit (Biozym, Hessisch Oldendorf, Germany) as recommended by the manufacturer. Quality of isolated DNA was initially checked by agarose gel electrophoresis and validated on an Agilent Bioanalyzer 2100 using an Agilent DNA 12000 Kit as recommended by the manufacturer (Agilent Technologies, Waldbronn, Germany). Concentration and purity of the isolated DNA was first checked with a Nanodrop ND-1000 (PeqLab Erlangen, Germany), and exact concentration was determined using the Qubit® dsDNA HS Assay Kit as recommended by the manufacturer (Life Technologies GmbH, Darmstadt, Germany). Illumina shotgun libraries were prepared using the Nextera XT DNA Sample Preparation Kit and subsequently sequenced on a MiSeq system with the reagent kit v3 with 600 cycles (Illumina, San Diego, CA, United States) as recommended by the manufacturer resulting in 1,694,377 paired end reads. For Nanopore sequencing, 1.5 μg HWD was used for library preparation using the Ligation Sequencing Kit 1D (SQK-LSK109) and the Native Barcode Expansion Kit (EXP-NBD104) as recommended by the manufacturer. Sequencing was performed on a MinION device Mk1B using a SpotON Flow Cell R9.4.1 as recommended by the manufacturer for 72 h. This resulted in 162,721 reads with a mean read length of 4,155 bp. Unicycler v0.4.8 (Wick et al., 2017) was used with default settings to perform a hybrid assembly.
Genome Annotation
The genomes were annotated using the Prokka automatic annotation software (Seemann, 2014). Protein coding, rRNA, and tRNA sequences were annotated using Prodigal (Hyatt et al., 2010), RNAmmer (Lagesen et al., 2007), and Aragorn (Laslett and Canback, 2004) against databases using BLAST (Camacho et al., 2009) and HMMER (Finn et al., 2011). Prediction of non-coding RNAs and CRISPR repeats were done by infernal (Nawrocki and Eddy, 2013) and MinCED1 based on CRISPR Recognition Tool (Bland et al., 2007). Signal peptides were searched using SignalP (Petersen et al., 2011). Protein coding genes were analyzed for COG (Tatusov et al., 1997; Galperin et al., 2014) functional annotation using Batch CD-search tool (Marchler-Bauer and Bryant, 2004). No secondary metabolite clusters were predicted in an analysis using antiSMASH 2.0 (Medema et al., 2011). The genome sequences and annotation data of all M. thermoacetica strains have been deposited in DDBJ/ENA/GenBank, for detailed information see Table 1.
Genome Analysis
For MLSA and gene content analysis, total protein sequences from the 24 genomes were extracted from the corresponding GenBank files using cds_extractor.pl v0.62 and used for downstream analysis with an in-house pipeline at the Göttingen Genomics Laboratory. In detail, proteinortho version 4.25 (default specification: blast = blastp v2.2.24, E-value = 1e-10, alg.-conn. = 0.1, coverage = 0.5, percent_identity = 50, adaptive_similarity = 0.95, inc_pairs = 1, inc_singles = 1, selfblast = 1, unambiguous = 0) (Lechner et al., 2011) was used to generate clusters of orthologs groups, inparalogs were removed. MUSCLE (Edgar, 2004) was employed to align the remaining sequences and poorly aligned positions were automatically filtered from the alignments using Gblocks (Castresana, 2000). A maximum likelihood tree from 1,177 orthologs groups was inferred with 500 bootstraps with RAxML (Stamatakis, 2014). The script PO_2_MLSA.py is available at github3. Visualization of the tree was performed using Dendroscop (Huson and Scornavacca, 2012).
Average Nucleotide Identity (ANIm) analyses were performed using pyani.py4. Briefly, nucleotide sequences were extracted from the corresponding GenBank files using seq_format-converter.pl v0.25 and subsequently used to run pyani in ANIm mode (uses MUMmer/NUCmer) to align input sequences. PHASTER (PHAge Search Tool Enhanced Release, Arndt et al., 2016) has been used for the detection of prophage regions. The analysis of genomic islands was performed using IslandViewer 4 (Bertelli et al., 2017).
Results
Strain DSM 1974T was purchased from DSMZ by our labs (University of Ulm and Technical University of Denmark) separately in 2015. Genome sequencing of the strain in the Göttingen Genomics Laboratory and at the Technical University of Denmark suggested that DSM 1974T is a mixed culture. After suspecting cross-contamination in our labs, new DSM 1974T cultures were ordered from DSMZ, however, with the same result. We independently isolated single clones after cultivation of DSM 1974T on solid medium: DSM 103284 (DSM 1974-Ulm) at the University of Ulm and DSM 103132 (DSM 1974-HH) at the Technical University of Denmark as described in Section “Materials and Methods.” We sequenced the genome of both strains which were derived from the DSM 1974T culture, as well as the genome of DSM 7417 and the genome of another ATCC 39073 strain, here designated ATCC 39073-HH. In order to determine whether DSM 1947T is a mixed culture we ordered ATCC 33924T (that is derived from DSM 1974T) from the ATCC and sequenced the DNA directly isolated from the freeze-dried culture (data not shown) and from a single colony isolated with the same procedure as for strain DSM 103132. Sequencing results confirmed that ATCC 33924T = DSM 1974T deposited at the ATCC is also a mixed culture and the strain isolated from that culture is identical to DSM 103132. The differences between DSM 103132 (isolated in Denmark) and DSM 103284 (isolated in Germany) suggest that slightly different cultivation conditions may favor the selection of different strains from the original mixed culture of DSM 1974T. In addition, the genomes of 10 different M. thermoacetica strains, M. thermoautotrophica DSM 7417, and M. glycerini DSM 11254T were sequenced.
Genome Features
Table 1 shows an overview of the de novo sequenced genomes of the DSM 1974T-derived strains (DSM 103284 and DSM 103132) and all other strain sequences in this study compared to the published genomes of type strains DSM 521T, DSM 2955T, as well as ATCC 39073, M. thermoacetica Y72, M. glycerini DSM 11254T, M. glycerini NMP, M. humiferrea DSM 23268T, M. mulderi DSM 14980T, M. perchloratireducens An10, and M. stamsii DSM 26217T. In order to investigate the phylogeny of the strains, we first compared the 16S rRNA gene sequences of the type strains, ATCC 39073-HH, DSM 103132, and DSM 103284. The sequence similarity between the strains in the 16S rRNA gene region is at least 99.74%, as no more than 3 nucleotide mismatches could be found. In strains DSM 103284 and ATCC 39073-HH, the gene regions are identical. According to Stackebrandt and Goebel (1994), bacteria showing less than 97% similarity in their 16S rRNA gene sequences belong to different species, while additional methods must be taken into consideration when the 16S rRNA similarity values are above 97%. All strains were analyzed with respect to prophages and interestingly none of the strains harbors a complete prophage. In all strains, a different number of incomplete phages (between 1 and 5; for details see Supplementary Table S2) were detected. Six strains, M. thermoacetica DSM 103132 and DSM 103284, M. glycerini DSM 11254T and NMP, M. perchloratireducens An10, and M. stamsii DSM 26217T contain putative phages, marked as “questionable.” These DNA regions show similarity to different Bacillus phages or to a Stx2-converting phage (Supplementary Table S2). We also checked some completely sequenced strains for the presence of genomic islands and found 9 such regions in strains DSM 103284 and DSM 2955T as well as 10 genomic islands in strain DSM 521T and ATCC 39073. Strain DSM 103132 harbors 36 genomic islands in total and one of these regions has a size of 166 kbp (Supplementary Table S3). All genomic islands contain mainly hypothetical proteins, transposases, or transcriptional regulators and only a few genes coding for enzymes (for details see Supplementary Table S3). With respect to plasmids, a 50-kbp plasmid was found in M. glycerini DSM 11254T. None of the M. thermoacetica or M. thermoautotrophica strains was found to carry a plasmid. All other Moorella species could not be analyzed in detail, as they are draft genomes and there is no evidence for plasmid replication genes in these genomes.
Phylogenetic Analysis
We used MLSA based on the detected core genome (1,177 OGs excluding paralogs) to perform phylogenetic analysis of our strains (Figure 1) and an average nucleotide identity analysis (ANIm) (Figure 2). The phylogenetic tree yielded two main clades, one consisting of all M. thermoacetica and M. thermoautotrophica strains and the second of M. glycerini DSM 11254 T, M. glycerini NMP, M. humiferrea DSM 23268T, M. mulderi DSM 14980T, M. perchloratireducens An10, and M. stamsii DSM 26217T. The first main clade shows three distinct subclades, one consisting of the different versions of M. thermoacetica ATCC 39079, DSM 521T, DSM 2955T, ATCC 49707, ATCC 31490, ATCC 35608T, DSM 12993 and DSM 6867. It should be noted that DSM 521T, DSM 2955T, ATCC 35608T, and ATCC 49707 are all derived from the same original strain. The second subclade consists of strains DSM 12797, DSM 11786, Y72, and DSM 7417. Strains ATCC 33924 and DSM 103132 form the third subclade. Strain DSM 103284, isolated from the mixed culture of DSM 1974T and strain DSM 21394 cluster outside of the three subclades. Interestingly, DSM 21394 is the strain with the third highest number of singletons (300 OGs). Whilst MLSA can provide insight into the phylogenetic relationship of organisms, for taxonomic studies there is a requirement for other methods, such as ANI analysis (Richter and Rosselló-Móra, 2009), which is a suitable in silico alternative for DNA–DNA hybridization (Goris et al., 2007). We performed an ANI analysis based on MUMmer alignment (ANIm) of the 24 genomes to define species and their complexes (Figure 2). We identified a large cluster comprising all M. thermoacetica and M. thermoautotrophica strains including DSM 103132 and DSM 103284, which have been both re-isolated from DSM 1974T as well as DSM 7417. The latter two strains are currently considered to be M. thermoautotrophica strains. However, our analysis clearly shows that these strains would be more appropriately classified as M. thermoacetica isolates, since we identified ANIm values between 98 and 99% compared to M. thermoacetica DSM 512T and DSM 2955T, the two independent deposits of the type strain of this species in the DSMZ (Supplementary Table S1). Richter and Rosselló-Móra (2009) proposed a threshold for the species boundary of 95% ANI, making reference to both ANIb and ANIm values. However, careful examination of their original data suggests that ANIb and ANIm do not give the same values and the species boundary for the two may be different. ANIm values of 98–99% are clearly above this threshold, but values of 95–96% need to be taken with caution. Our analysis also revealed that strain DSM 21394 has an ANIm value of 94% (Supplementary Table S1) compared to the other M. thermoacetica strains, which is below the threshold for the species boundary and further studies would be needed to determine whether this strain should also be re-classified. This is also depicted in Figure 2, where all strains belonging to one species are marked in red tones. Interestingly, M. stamsii DSM 26217T, M. glycerini NMP, and M. perchloratireducens An10 showed an ANIm value of 100% and they should therefore belong to the same species. However, the name M. perchloratireducens has not been validly published and M. glycerini NMP is not the nomenclatural type of the species so no formal nomenclatural action is required under the International Code of Nomenclature of Prokaryotes (Parker et al., 2019), since the names M. glycerini and M. perchloratireducens can only be formally considered to be heterotypic synonyms if both are validly published and are the corresponding nomenclatural types. The ANIm value of the type strain of M. stamsii to the other two strains is also 100%, indicating that all three should be placed in the same species, i.e., M. stamsii, which has been validly published. These results are in contrast to the published viewpoint that M. stamsii and M. perchloratireducens represent distinct species. It is common practice to determine the 16S rRNA gene sequence of a novel isolate and initially investigate the similarity value to the 16S rRNA gene sequences of other type strains before deciding how to further characterize a strain. In the case of 16S rRNA gene sequence similarity values of 97% and greater it is common practice to determine DNA–DNA hybridization values (which is now being replaced by ANI or digital DNA–DNA hybridization studies) to evaluate whether one is dealing with a new species. Where the 16S rRNA gene sequence similarity values are less than 97%, it is generally assumed that one has a novel species. Key discrepancies in the study of M. glycerini, M. stamsii, and M. perchloratireducens are the 16S rRNA gene sequences and the genomic similarity. In the case of M. glycerini, the 16S rRNA gene sequence determined in the original study (Slobodkin et al., 1997), U82327, showed a pairwise similarity of 99.3% to the 16S rRNA sequence determined in the genome (CP046244). The 16S rRNA gene sequence determined in the original study of M. stamsii (Alves et al., 2013), HF563589, showed a pairwise similarity of 99.3% to the 16S rRNA sequence determined in the genome contig PVXL01000051. When U82327 and HF563589 were compared by Alves et al. (2013), the similarity values were 97%, but comparison of the 16S rRNA gene sequences obtained from the genomes (CP046244 and PVXL01000051) now gives 99.2% similarity and 100% similarity to CELZ01000013. In the case of DNA–DNA hybridization between these two strains the value was 51.1–53.3% (duplicated measurements). The 16S rRNA gene sequence from the genome of M. glycerini DSM 11254T contains a large deletion that does not occur in U82327 or any of the other PCR-amplified 16S rRNA gene sequences or those determined via genome sequencing of the same strain (Supplementary Figure S1). The PCR-amplified 16S rRNA gene from M. stamsii (HF563589) also appears to contain numerous additional bases.
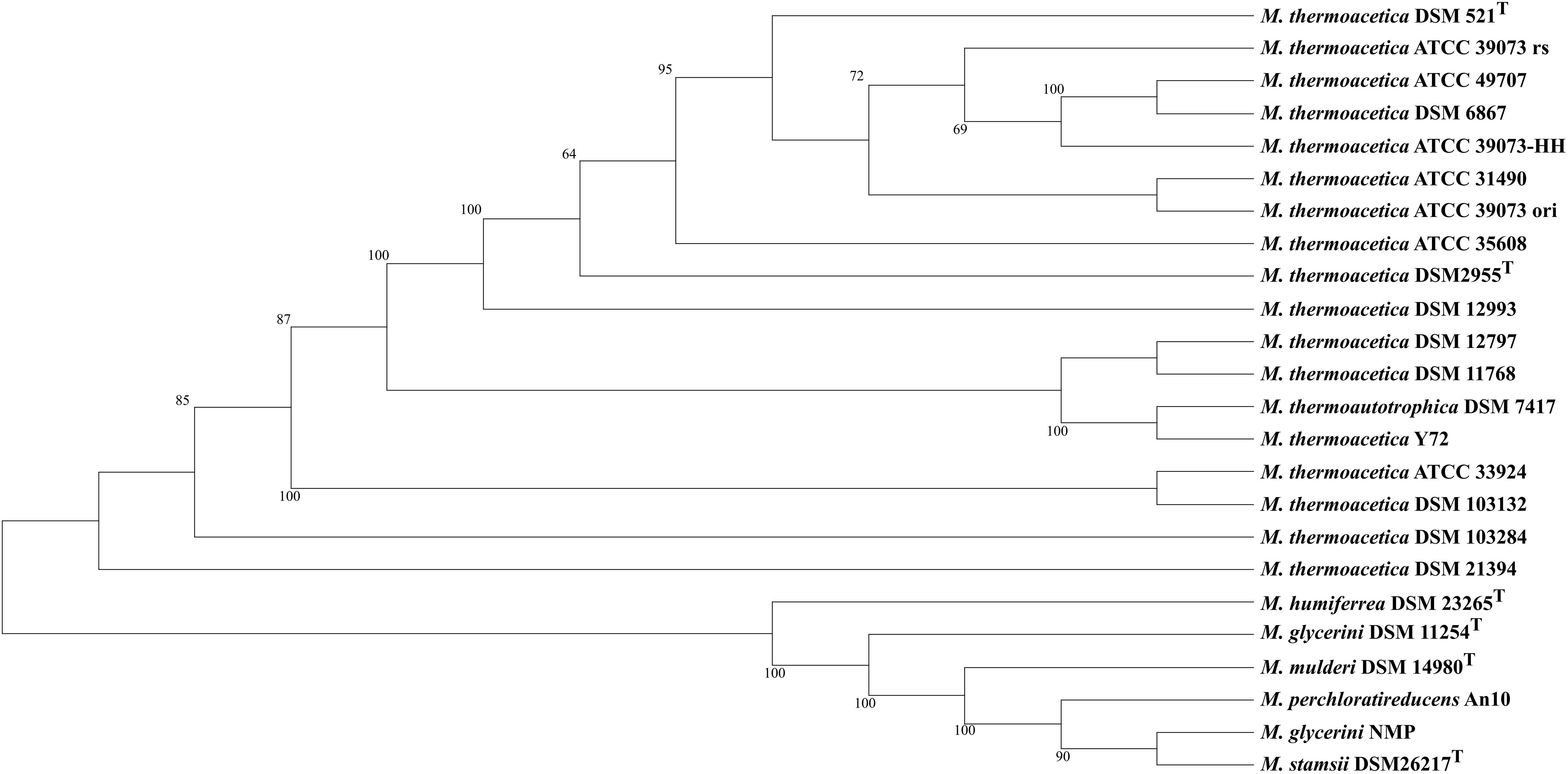
Figure 1. MLSA tree of 24 sequenced Moorella strains: maximum likelihood trees of 24 Moorella genome sequences were inferred with 500 repetitions with RAxML (Stamatakis, 2014) and visualized with Dendroscope (Huson and Scornavacca, 2012). M. thermoactica marked with ATCC 39073 ori is the original sequence of this strain, ATCC 39073 rs is a sequenced version of the genome performed by the JGI and ATCC 39073-HH is a sequenced version of the genome performed by Technical University of Denmark.
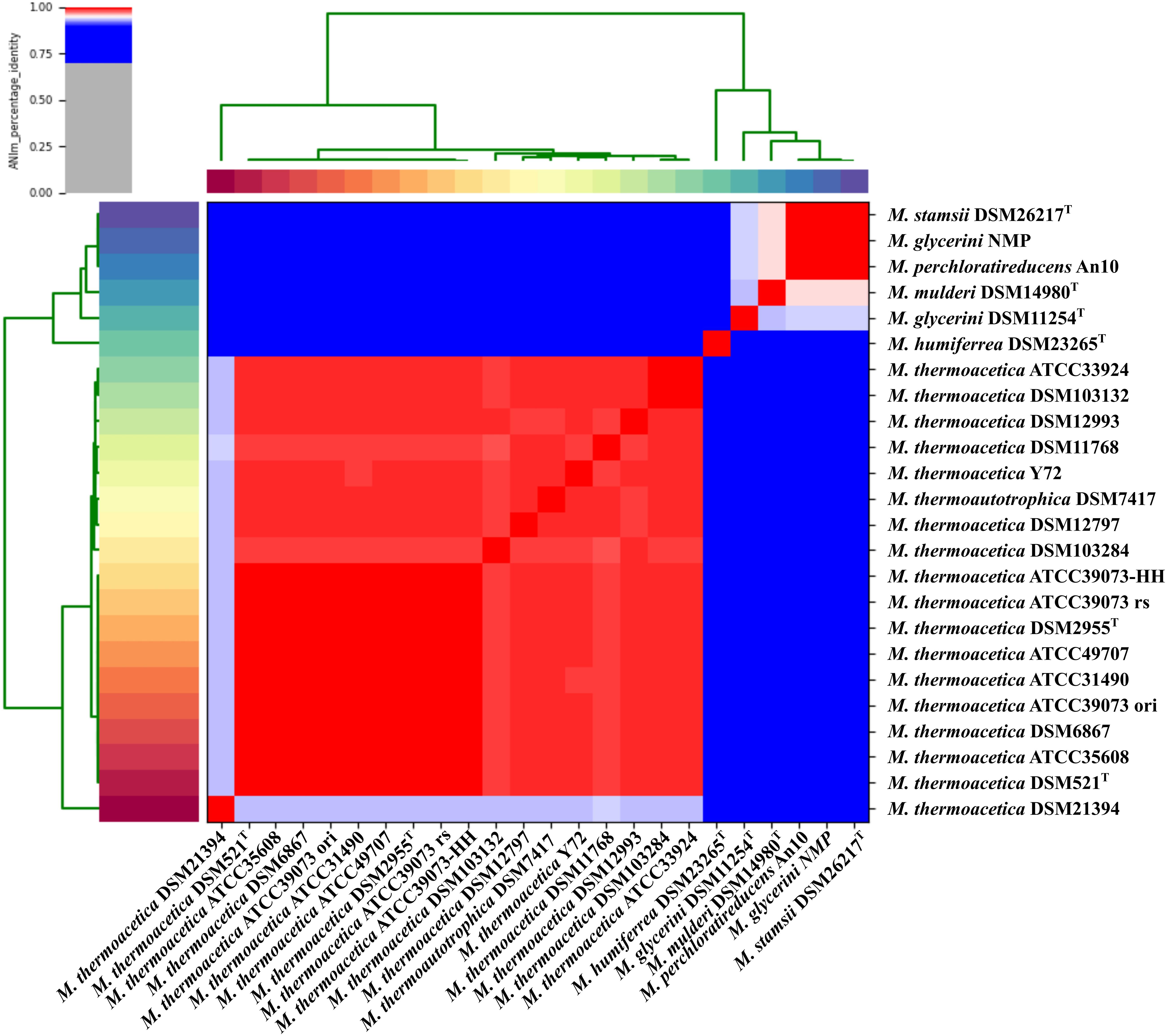
Figure 2. Average nucleotide identity analysis of the 24 sequenced strains: ANIm analysis based on MUMmer alignment (Delcher et al., 2002) of the genome sequences was performed and visualized using PYANI (https://github.com/widdowquinn/pyani). M. thermoactica marked with ATCC 39073 ori is the original sequence of this strain, ATCC 39073 rs is a sequenced version of the genome performed by the JGI and ATCC 39073-HH is a sequenced version of the genome performed by Technical University of Denmark.
In the case of M. perchloratireducens, comparison of the 16S rRNA gene sequence determined in the original study, EF060194 (Balk et al., 2008), with that extracted from the genome (Gp0011525) showed 95.1% similarity. While EF060194 showed 97% sequence similarity with the 16S rRNA gene sequence from the genome of M. thermoacetica ATCC 39073 (CP00232), comparisons with the 16S rRNA gene sequence from Gp0011525 indicated that the genome-derived sequences showed 95% sequence similarity. In contrast, comparisons between EF060194 (M. perchloratireducens) and U82327 (M. glycerini)/HF563589 (M. stamsii) gave sequence similarity values of 93.9 and 93.1%, respectively. However, comparisons based on the 16S rRNA gene sequences extracted from the genomes Gp0011525 (M. perchloratireducens), CP046244 (M. glycerini), and PVXL01000051 (M. stamsii) gave pairwise similarities of 99.2–100%. No DNA–DNA hybridization studies were carried out by Balk et al. (2008), because they used a 16S rRNA gene sequence “threshold” of 98% 16S rRNA similarity. These results suggest significant discrepancies between the 16S rRNA gene sequence EF060194 obtained by primer amplified sequencing and that determined by genome sequencing that are evident in the alignments (Supplementary Figure S1) and are difficult to attribute to experimental error without further confirmatory work. It is interesting to note that of the two deposits of M. perchloratireducens An10, ATCC BAA-1531 and JCM 14829 only ATCC BAA-1531 is currently available and is the source strain for the genome Gp0011525. In the case of M. mulderi DSM 14980, the genome-derived 16S rRNA gene sequence (LTBC01000042.1) contains a large insert not present in sequence of the original PCR-amplified gene deposited as AF487538.1 (Supplementary Figure S1).
The ANIm values between M. glycerini (strain NMP), M. stamsii, and M. perchloratireducens indicate that they belong to the same species. Although the 16S rRNA gene sequence of the type strains of M. glycerini and M. stamsii are 99.3%, the AMIm value of 94% indicates that they are different species. In the case of M. mulderi DSM 14980 the genome-based 16S rRNA gene sequence similarity to M. glycerini DSM 11254 is 98.8% and the AMIm value 93%, indicating that they are different species. When compared to the genome-based 16S rRNA gene sequences of M. glycerini (strain NMP), M. stamsii, and M. perchloratireducens the value is 99.3% and the ANIm value 96%; this would appear to indicate that M. mulderi DSM 14980 is a member of the same species as M. stamsii DSM 26217. However, the original work of Richter and Rosselló-Móra (2009) indicate that an ANI cut-off of 95% ANIb is equivalent to an ANIm value of 96.5%, indicating that M. mulderi DSM 14980 and M. stamsii DSM 26217 are not members of the same species. This work also indicates the importance of examining the data beyond simple similarity values, where examination of the individual 16S rRNA gene sequence alignments, the differences in gene content and genome size provide extra valuable detail.
Genome Comparison
Until recently, only the sequence of the non-type strains M. thermoacetica ATCC 39079 and M. thermoacetica Y72 were publicly available, but many other strains, including the two independently deposited type strains of the species (DSM 521T and DSM 2955T), and several other strains are available at the German Collection of Microorganisms and Cell Cultures (DSMZ Brunswick), including strain DSM 1974T. We sequenced the genomes of all these strains and performed whole genome comparison of all M. thermoacetica strains, and comparison with the genomes of five other species, namely M. stamsii DSM 26217T, M. humiferrea DSM 23265T, M. glycerini DSM 11254T, M. glycerini NMP, M. perchloratireducens An10, and M. mulderi DSM 14980T (Figure 3). All M. thermoacetica strains have a comparable genome size of 2.52–2.64 Mb, except the two closely clustering strains DSM 103132 and ATCC 33924, which have larger genomes (2.98 and 2.91 Mb). M. glycerini NMP has the largest genome size in our comparison with 3.58 Mb, followed by M. glycerini DSM 11254T with 3.56 Mb. A whole genome comparison based on protein encoding genes revealed a core genome shared by all 24 strains of 1,297 OGs including paralogs and a pan genome of 8,042 OGs (Figure 4). The pan genome includes the core and the flexible genome, OGs shared by at least two genomes, but not by all genomes in the comparison. The size of the core genomes is half the size of the complete genome of the M. thermoacetica strains, due to the high proportion of M. thermoacetica strains in our comparison. We found a broad range of singletons, meaning genome-specific genes, varying between 15 and 275 OGs in the M. thermoacetica group. The highest number of singletons (674 OGs) was found in the genome of M. glycerini DSM 11254T. The flexible genome harbors for example a complete gene cluster encoding a pyruvate:ferredoxin oxidoreductase, which is only present in DSM 103284, DSM 11768, DSM 512T, DSM 2955T, DSM 12797, and all ATCC 39073 genomes. A cluster encoding an anaerobic dimethylsulfoxide reductase (DSMO reductase) is present in all genomes compared here, except of M. mulderi DSM 14980 and M. thermoacetica DSM 103132, which has been re-isolated from the mixed culture DSM 1974. We also identified OGs that are specific for the above-mentioned phylogenetic clades. We identified, for example, a gene cluster coding for a carbohydrate-specific ABC transport system, which is exclusively present in the first main clade comprising all M. thermoacetica strains, but which is absent in the second main clade consisting of M. stamsii DSM 26217T, M. humiferrea DSM 23265T, M. glycerini DSM 11254T, M. perchloratireducens An10, and M. mulderi DSM 14980T. We also identified gene clusters specific for the first main clade, for example a cluster encoding, amongst other genes, a ribose permease, L-rhamnose mutarotase, and a L-fucose isomerase probably involved in rhamnose and fucose metabolism. There are also genome-specific genes. M. thermoacetica DSM 103284 for example harbors a hydrogenase gene cluster that could not be identified in any other genome analyzed in this study.
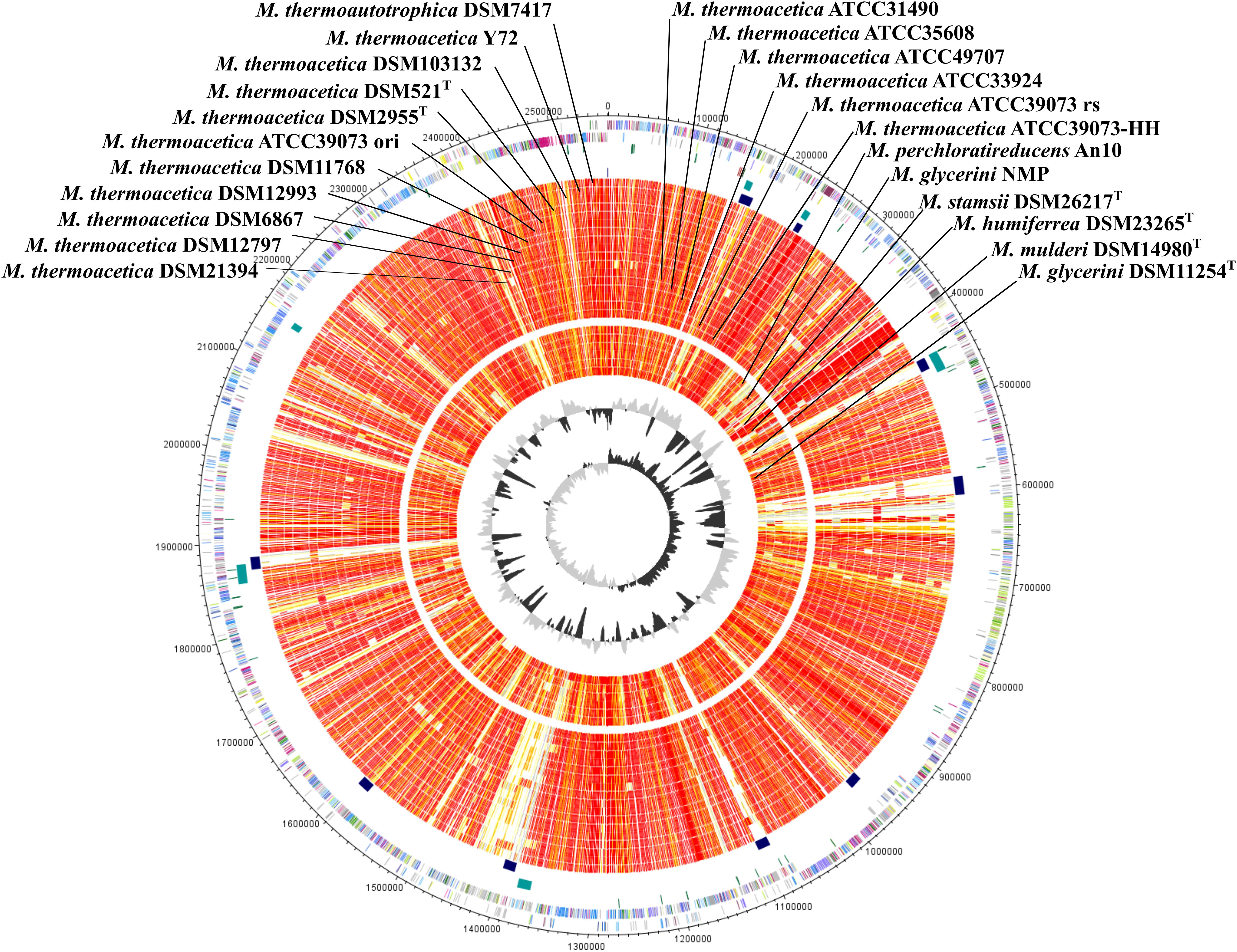
Figure 3. Circular representation of the genome comparison of M. thermoacetica DSM 103284 with other Moorella strains. The genes encoded by the leading and the lagging strand (outer circles 1 and 2) of M. thermoacetica DSM 103284 are marked in COG colors in the artificial chromosome map. tRNA (green) and rRNA (pink) genes were plotted on circle 3. Detected prophage regions (petrol) and genomic islands (dark blue) are shown on circles 4 and 5, respectively. The presence of orthologous genes (red, high similarity; orange, medium similarity; yellow, low similarity (see color code below) is indicated for the genomes in comparison to M. thermoacetica DSM 103284. The two innermost plots represent the GC content and the GC skew (circles 29 and 30). Visualization was done using Proteinortho (Lechner et al., 2011) results and DNAPlotter (Carver et al., 2009). COG categories of the genes were extracted from IMG database (Galperin et al., 2014) entries of M. thermoacetica DSM 103284. Color code according to E-values of the blastp analysis performed using Proteinortho4.26. Gray, 1e–20 to 1; light yellow, 1e–21 to 1e–50; gold, 1e–51 to 1e–90; light orange, 1e–91 to 1e-100; orange, 1e–101 to 1e–120; red, > 1e–120 M. thermoactica marked with ATCC 39073 ori is the original sequence of this strain, ATCC 39073 rs is a sequenced version of the genome performed by the JGI and ATCC 39073-HH is a sequenced version of the genome performed by Technical University of Denmark.
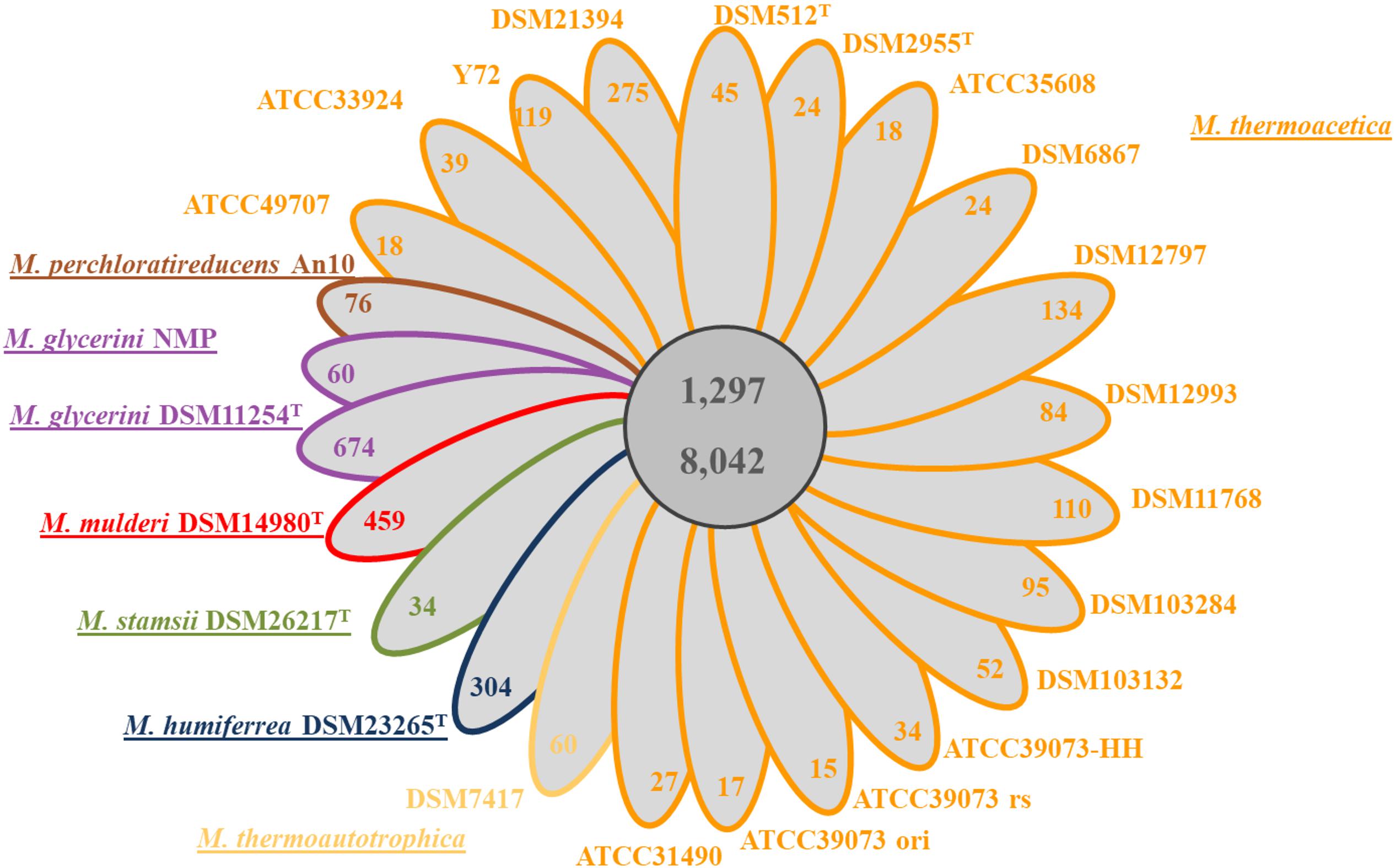
Figure 4. Core/Pan genome analysis of 24 Moorella genomes: a simplified Venn diagram showing the core and the pan genome of all 24 Moorella strains. The number of genome-specific OGs is depicted in the respective ellipse. Ortholog detection was done with blastp and the Proteinortho software (Lechner et al., 2011) with a similarity cut off of 50% and an E-value of 1e–10. M. thermoacetica marked with ATCC 39073 ori is the original sequence of this strain, ATCC 39073 rs is a sequenced version of the genome performed by the JGI and ATCC 39073-HH is a sequenced version of the genome performed by the Technical University of Denmark.
Phenotypical and Physiological Differences Between M. thermoacetica and M. thermoautotrophica Strains
Several phenotypical and physiological differences between M. thermoacetica and M. thermoautotrophica strains regarding compounds that can serve as carbon and energy source have been described in the literature. Those results are sometimes contradictory to each other. We therefore tested whether there are differences between the strains regarding carbon source utilization and whether the results can give a hint toward the identity of strain DSM 1974T. Our results largely agree with the results reported in the literature. Within the tested strains, only DSM 103132 can utilize arabinose (Table 2). As already published, M. thermoacetica (DSM 521T or ATCC 39073) (Fontaine et al., 1942; Andreesen et al., 1973; Cato et al., 1986) and DSM 1974T (Wiegel et al., 1981; Cato et al., 1986) are not able to utilize arabinose. DSM 103132 was also found to be the only strain that could utilize formate, but only reaching low optical densities. DSM 1974T has been reported to utilize formate (Wiegel et al., 1981; Fröstl et al., 1996), like ATCC 39073 (Fröstl et al., 1996). All tested strains were able to grow on fructose and glucose, and these substrates led to the highest cell density, which is in agreement with literature on M. thermoacetica (DSM 521T or ATCC 39073) (Fontaine et al., 1942; Andreesen et al., 1973). DSM 521T was the only strain that did not utilize H2 + CO2 as carbon and energy source in our experiments. ATCC 39073 (Daniel et al., 1990; Fröstl et al., 1996) and DSM 1974T (Wiegel et al., 1981; Savage and Drake, 1986; Fröstl et al., 1996) have been reported to utilize methanol. In agreement with our results, DSM 521T has been reported not to grow on methanol (Cato et al., 1986). All strains tested in this study grew with pyruvate as energy and carbon source. Interestingly, Cato et al. (1986) indicated that pyruvate does not serve as a growth-supportive substrate for DSM 1974T (Wiegel et al., 1981). None of the tested strains, except for DSM 103284, could utilize rhamnose, which is in line with DSM 1974T being the only M. thermoacetica/thermoautotrophica strain previously reported to utilize rhamnose (Cato et al., 1986). According to the literature, M. thermoacetica (DSM 521T or ATCC 39073) (Fontaine et al., 1942; Andreesen et al., 1973; Cato et al., 1986) and DSM 1974T (Wiegel et al., 1981; Cato et al., 1986) are not capable of utilizing sucrose, however, we observed growth for ATCC 39073-HH and DSM 103132 on that substrate. All tested strains, except DSM 103132, utilized xylose. In the case of DSM 1974T, contradictory results have been reported: according to Cato et al. (1986), in 61–89% of the tests, cultures were able to utilize xylose. Some of the differences in substrate utilization can be explained by comparison of the genomes. For example, the arabinose operon in the genome of DSM 103132 is not present in the genome of ATCC 39073-HH. The pathway for xylose utilization is encoded in the ATCC 39073-HH genome, but not in the DSM 103132 genome. Other differences in carbon source utilization between the various studies may be due to the fact that strains might have adapted to different substrates or that the substrate utilization depends on the growth stage of the inoculum (Wiegel et al., 1981), which may be caused by differences in transcriptional regulators between the strains (Marcellin et al., 2016). Our results do not allow an unambiguous conclusion to be drawn whether one of the strains (DSM 103132 and DSM 103284) corresponds to the strain originally studied by Wiegel et al. (1981) and deposited as DSM 1974T in the DSMZ. In addition to carbon source utilization, other phenotypical and physiological differences between the M. thermoacetica/thermoautotrophica strains have been described, such as differences in motility [DSM 1974T is motile (Cato et al., 1986), DSM 521T is not (Carlier and Bedora-Faure, 2006)] and growth temperature [DSM 1974T can grow at 70°C (Cato et al., 1986), while DSM 521T cannot (Carlier and Bedora-Faure, 2006)].
Discussion
Strains of M. thermoacetica and M. thermoautotrophica have become model organisms of the acetogenic metabolism. Due to the observation of conflicting phenotypic traits that have been connected with the two different species, the scientific community has already questioned the taxonomic status of the two species M. thermoautotrophica and M. thermoacetica (Carlier and Bedora-Faure, 2006; Kimura et al., 2016). In addition to the high similarity of the genomes’ 16S rRNA gene sequence, there are further similarities described for M. thermoacetica/thermoautotrophica strains such as a similar fatty acid and peptidoglycan profile (Yamamoto et al., 1998) and presence of the same menaquinone (Das et al., 1989). However, these features are generally conserved in “closely related” taxa and one would not expect significant differences between strains showing such a high degree of genetic similarity (Tindall, unpublished). Until a few years ago, only the sequence of the non-type strains M. thermoacetica ATCC 39079 and M. thermoacetica Y72 were publicly available, but many other strains, including the two type strains of the species (DSM 521T and DSM 2955T), and several other strains are available at the German Collection of Microorganisms and Cell Cultures (DSMZ Brunswick), including strain DSM 1974T. We wished to broaden knowledge of the genetic diversity of this group of organisms and therefore sequenced the genome of both strains which were derived from the DSM 1974T culture (DSM 103132 and DSM 103284), as well as the genome of DSM 7417 and the genome of another sub-culture of ATCC 39073 (ATCC 39073-HH). In addition, the genomes of eight different M. thermoacetica strains were sequenced. Comparison of the 16S rRNA gene sequences of the strains, ATCC 39073(-HH), DSM 103132, and DSM 103284, showed a sequence similarity between the strains higher than 99.74%. We used MLSA, gene content analysis, and ANI analysis to get insights into the phylogeny of the genus Moorella. With ANIm values between 98 and 99% compared to the other M. thermoacetica strains DSM 512T and DSM 2955T, the strains derived from DSM 1974T (DSM 103132 and DSM 103284) are clearly M. thermoacetica isolates. Through genome sequencing of different M. thermoacetica and M. thermoautotrophica strains, it was evident that M. thermoautotrophica DSM 1974T consists of at least two different strains, which are both very closely related to each other and to M. thermoacetica. Since phylogenetic analysis showed that all M. thermoacetica/thermoautotrophica strains described to date belong to the same species, there would appear to be no justification based on the currently available data for considering M. thermoautotrophica to be a separate species. Consequently, the strains DSM 103132 and DSM 103284 (both derived from DSM 1974T, the designated type strain of M. thermoautotrophica) must be designated as M. thermoacetica. Based on the current study, the observed phenotypic differences are likely to be due to strain variations within one species, as already indicated by Wiegel et al. (1981) and Cato et al. (1986). Furthermore, observed differences in carbon source utilization cannot serve as a suitable measure to distinguish species, since the substrate acceptance may be dependent on cultivation conditions. However, the picture is complicated by the fact that DSM 1974T, the strain which led to the proposal of the new species C. thermoautotrophicum (Wiegel et al., 1981) and was later transferred to the genus Moorella as M. thermoautotrophica (Collins et al., 1994) was consistently shown by genome sequencing to consist of two different strains. The isolation of two different strains that have subsequently been deposited as DSM 103132 and DSM 103284 confirms these observations. However, taking the original data of Wiegel et al. (1981) and comparing them with the data collected in this study for DSM 103132 and DSM 103284 does not show a large number of significant differences in the physiology of the strains. Based on the current data and taking into consideration the methods originally used by Wiegel et al. (1981) it is not possible to determine whether the original strain of Wiegel, JW 701/3, was a mixture of two different strains of the same species, whether the original strain was a pure culture, but a mixed culture was submitted for deposit (that methods used at the time would not have detected), or whether a second strain was introduced into the culture subsequent to accession to the DSMZ. Cross-contamination of strains is one possible explanation: the spores of Moorella species are highly heat-resistant and are not sufficiently inactivated by a standard autoclaving at 121°C (Fontaine et al., 1942). Byrer et al. (2000) for example described the strains JW/DB-2 and JW/DB-4 (ATCC number BAA-48) that show unusually heat-resistant spores. However, given the resolution of methods used at the time, one also cannot exclude with certainty that the original culture did not consist of more than one strain. One interesting aspect is that Wiegel et al. (1981) report that DNA–DNA hybridization supported the recognition of strains JW 701/3 and strain KIVU as members of the same species, but distinct from C. thermoaceticum (M. thermoacetica). Kimura et al. (2016) have previously reported a similar problem with the designated type strain of M. thermoautotrophica. Formulated as a Request for an Opinion, this limits any action that can be taken to a formal ruling by that body. However, their work concentrates largely on the interpretation of 16S rRNA gene sequences that appear to have been obtained by both cloning and the isolation of strains from the culture supplied. Representative partial sequences of the 16S rRNA genes of the seven groups obtained by cloning and sequencing of the isolates have been deposited as LC133084–LC133087 and designated in the publication as representing OUT-1 to OUT-4 in that order, respectively. Kimura et al. (2016) concentrate on a single 16S rRNA gene sequence deposited as L09168 (from DSM 1974) and do not mention that additional sequences are available, X58353 and X77849. X58353 (strain JW 701/3; 1155 bases, but with numerous Ns) was deposited in 1990 from the University of Kiel and will not be considered further. X77849 was deposited in 1994 from the University of Reading in co-operation with Dr. Hippe (DSMZ curator of the strain at the time) and is derived from DSM 1974 and presumably directly from stocks held in the DSMZ. L09168 was deposited in 1993 from The University of Queensland. A direct alignment of the two sequences L09168 and X77849 indicates that, ignoring a small number of Ns in X77849, the two are not identical making it difficult to conclude whether either of the two can be considered to be a 100% accurate reflection of the original gene sequences from the same strain. Similarly, a comparison with the 16S rRNA sequences from Kimura et al. (2016) also indicate that neither of the two sequences (X77849 and L09168) (Supplementary Figure S2) show 100% similarity with those obtained by Kimura et al. (2016). It should also be remembered that the sequences X77849 and L09168 are only one part of the evidence that were not obtained directly when the type strain was originally described and “verification” of X77849 vs. L09168 does not allow one to conclude that one sequence is “correct” and the other in error. If one were to extend the reasoning of Kimura et al. (2016) to other similar cases one would conclude that given the differences between the 16S rRNA gene sequence obtained by direct amplification and that extracted from the genome of M. stamsii that the type strain does not exist. An even more dramatic example is the case of Alterococcus agarolyticus (Shieh and Jean, 1998) that started its taxonomic career as an atypical member of the Enterobacteriaceae (Shieh and Jean, 1998) under the 16S rRNA gene sequence AF075271.1 (deposited 19th June 1998) that was substituted for by AF075271.2 (deposited 21st August 2002) and is widely accepted as a member of the Verrucomicrobia. Under these circumstances, the nomenclatural type currently available certainly does not correspond to the 16S rRNA gene sequence originally deposited as AF075271.1 and one would have to conclude that the type strain no longer exists. However, put in context other data in the original publication clearly indicates that Alterococcus agarolyticus was an atypical member of the Enterobacteriaceae and that the original 16S rRNA gene sequence AF075271.1 is in error and should have been verified.
In the case of M. thermoautotrophica, comparison with the 16S rRNA gene sequence deposited as X77849 and L09168 also needs to be treated with caution if the original source culture (DSM 1974T) was not a pure culture or where the quality/accuracy of gene sequencing technologies may have changed over the decades. No attempt was made to compare the physiological/biochemical properties of the strains studied by Kimura et al. (2016) with the original work of Wiegel et al. (1981) and relies solely on one older gene sequence (L09168) that is not corroborated by another sequence (X77849) obtained at about the same time from the same source culture, DSM 1974T. Examining the 16S rRNA sequences deposited by Kimura et al. (2016) (LC133084-LC133087) against L09168, X77849 and those extracted from the genomes derived from subcultures of DSM 1974 and ATCC 33924 (including re-deposits as DSM 103132 and DSM 103284), i.e., CP017019.1 (positions 154745–156300 and 147549–149104), CP017237.1 (positions 144877–146432), and VCDX01000030.1 (positions 1667–112) indicates that toward the end of the single primer amplified partial sequences LC133085 and LC133086 gaps are present that are not otherwise present in any of the other sequences in a region that could be considered to be conserved (Supplementary Figure S2). These gaps have, therefore, not been taken into consideration in the analysis here. Kimura et al. (2016) do not provide alignments of sequences in support of their work and make it impossible to determine why they consider “none of the sequences were similar to M. thermoautotrophica DSM 1974T (L09168),” when in fact they show only minimal differences in the alignments presented here. Although alignments are critical steps in the evaluation of sequence-based data (both nucleotide and amino acid based) they are rarely given, contrary to recommendations (Tindall et al., 2010), making the direct verification of the resulting interpretation via this critical step impossible and are therefore included in Supplementary Figures S1, S2. The sequence LC133087 appears to belong to a strain having the most similar 16S rRNA sequence to M. humiferrea strain 64_FGQT (GQ872425) and will not be considered further. In the alignment shown, CP017019.1 (positions 154745–156300), CP017019.1 (positions 147549–149104), VCDX01000030.1 (positions 1667–112), and LC133086.1 have a “T” at position 280 (alignment numbering, Supplementary Figure S2) while CP017237.1 (positions 144877–146432), LC133084.1, and LC133085.1 have a “C” at the same position. LC133086.1 differs from CP017019.1 (positions 154745–156300), CP017019.1 (positions 147549–149104), and VCDX01000030.1 (positions 1667–112) in having a “T” position 435 rather than a “C” that is present in all other sequences (Supplementary Figure S2). LC133084.1 appears to be identical in the aligned bases to CP017237.1 (positions 144877–146432), but LC133085.1 has an “A” at position 745 rather than a “G” that is present in all other sequences (Supplementary Figure S2). Based on these observations, the only organism recovered in this study and that of Kimura et al. (2016) is that represented by LC133084.1 and CP017237.1 (DSM 103284). While this demonstrates the care that has to be taken in evaluating the interpretation of the data used by Kimura et al. (2016), the major problem that arises centers on the fact that the strains isolated by Kimura et al. (2016) have not been deposited in a culture collection and comparison with the original physiological and biochemical data published by Wiegel et al. (1981) cannot be made. Based on an evaluation of the 16S rRNA sequences determined previously and those determined here it is not possible to conclude that the type strain no longer exists, since it was deposited as DSM 1974 and ATCC 33924 and the 16S rRNA sequences deposited as X77849 and L09168 do not appear to be fully accurate.
The Request for an Opinion of Kimura et al. (2016) also misinterprets the wording of Rule 18c and draws incorrect conclusions. Tindall (2016) provided a detailed discussion of the incorrect interpretation of Rule 18c that was also applied by Kimura et al. (2016). Based on the evidence presented by Kimura et al. (2016) and that obtained in this work one cannot conclude that the nomenclatural type no longer exists, but rather there may be an issue with the purity of the culture deposited/currently available. The current study covers the physiological/biochemical properties of strains isolated from DSM 1974T and expands on the genomic characterization of the strains studied. While it is clear that DSM 103132 and DSM 103284 (both derived from DSM 1974T, the designated type strain of M. thermoautotrophica) are more appropriately considered to be members of the species M. thermoacetica, there is a formal nomenclatural issue that also needs to be addressed that requires reference to be made to the International Code of Nomenclature of Prokaryotes (Parker et al., 2019). Typically, the nomenclatural type of a species as defined in Rule 18a is an axenic culture, but there are instances where one component part of a syntrophic co-culture has been named and the co-culture accepted as the nomenclatural type (type strain). However, when mixed cultures or consortia are considered (see Rule 31a and 31b) and these are treated as a “single” biological entity, the names associated with them are not validly published and could be applied to M. thermoautotrophica. In the case of DSM 1974T and ATCC 33924T, although the strains currently in circulation appear to be a mixed culture, there is no unambiguous evidence that the parent culture, strain JW 701/3, was also a mixed culture. In contrast to the study of Kimura et al. (2016), it has been possible to study in greater detail pure cultures of strains isolated from DSM 1974T (that is the parent deposit for all other culture collection strains) and subsequently deposited as DSM 103132 and DSM 103284. In both cases, the strains appear to be members of the species M. thermoacetica. One possible solution would be to designate one of them as a neotype, although based on the physiological and biochemical data presented here neither of the two strains (DSM 103132 or DSM 103284) can unambiguously be shown to be more similar in its properties than the other to the data originally published by Wiegel et al. (1981). Irrespective of which course of action is taken, it is clear that the culture of DSM 1974T made available to the current authors contains strains that should be classified in the species M. thermoacetica leading to the logical conclusion that DSM 103132 and DSM 103284 should be assigned to that species. This nomenclatural conclusion is inescapable, irrespective of whether one follows the arguments of Kimura et al. (2016), where the name M. thermoautotrophica would eventually be rejected, declared to not have been validly published, or whether one considers the names M. thermoacetica (Fontaine et al., 1942; Collins et al., 1994) and M. thermoautotrophica (Wiegel et al., 1981; Collins et al., 1994) to be heterotypic synonyms. In the latter case, priority is governed by Rule 23a, 38 and 42 where the dates of valid publication of the epithets are taken into consideration, i.e., thermoacetica Fontaine et al. (1942) has priority over thermoautotrophica Wiegel et al. (1981). This also leads to the use of the name M. thermoacetica (Fontaine et al., 1942; Collins et al., 1994) and recognition of M. thermoautotrophica (Wiegel et al., 1981; Collins et al., 1994) as the later heterotypic synonym when their respective nomenclatural types are considered to members of the same taxon. The current authors favor the latter course of action, but the Judicial Commission may also decide otherwise. Also, M. thermoautotrophica DSM 7417 should be reclassified as M. thermoacetica as well.
In addition to resolving the M. thermoacetica/thermoautotrophica problem, this comprehensive analysis of the genus Moorella by the study of a significant number of novel genome sequences and knowledge of phenotypic differences led to two other important conclusions. First, strain DSM 21394, currently still named M. thermoacetica, clearly does not belong to this species. Reclassification and renaming as a new species are required. Secondly, M. glycerini NMP, M. stamsii DSM 26217T, and M. perchloratireducens cannot be distinguished at species level. Furthermore, M. glycerini NMP has been wrongly assigned as M. glycerini as this strain shows an ANIm value of 94% similarity compared to the type strain DSM 11254T and is clearly a different species despite the high 16S rRNA gene sequence pairwise similarity of 99.7%. Based on the data presented here, M. glycerini NMP, M. stamsii DSM 26217T, and M. perchloratireducens are all members of the same species. Although reclassification of these three strains may be required, caution needs to be exercised when one considers differences between the data reported here and that previously reported in the literature (Slobodkin et al., 1997; Balk et al., 2008; Alves et al., 2013), especially with regards to the 16S rRNA gene sequences and the genomic similarity inferred from DNA–DNA hybridization experiments vs. in silico comparisons.
Data Availability Statement
The datasets generated for this study can be found in the IMG, GenBank, NCBI.
Author Contributions
SR, AP, FB, TJ, CJ, PD, and AN conceived and designed the experiments. SR, AP, CE, FB, and TJ performed the experiments. SR, AP, CE, FB, TJ, CJ, PD, and AN analyzed the data. SR, AP, FB, TJ, CJ, BT, RD, PD, and AN wrote the manuscript.
Funding
This work was supported by The Novo Nordisk Foundation to the Technical University of Denmark (grant number NNF10CC1016517) and a Ph.D. grant to SR from the People Programme (Marie Curie Actions) of the European Union Seventh Framework Programme FP7-People-2012-ITN, under grant agreement number 317058, “BACTORY.” TJ was supported by EU H2020’s Research and Innovation Programme under grant agreement number 731263 “AMBITION.” CJ was supported by a grant from the Novo Nordisk Foundation (grant number NNF15OC0015246). Support was also provided by the ERA-IB 7 Project “OBAC” (FKZ 031B0274) to the groups of RD and PD. Independent Research Fund Denmark under grant number 7026-00025B.
Conflict of Interest
BT was employed by company Leibniz-Institut DSMZ-Deutsche Sammlung von Mikroorganismen und Zellkulturen GmbH, Brunswick, Germany.
The remaining authors declare that the research was conducted in the absence of any commercial or financial relationships that could be construed as a potential conflict of interest.
Acknowledgments
We acknowledge the support for the PacBio sequencing given by Björn Hallström, Science for Life Laboratory (KTH) and conducted by Christian Tellgren-Roth (UGC, NGI). We thank Melanie Heinemann and Sarah Teresa Schüßler for technical support and Richard Egelkamp for help with graphic tools.
Supplementary Material
The Supplementary Material for this article can be found online at: https://www.frontiersin.org/articles/10.3389/fmicb.2019.03070/full#supplementary-material
Abbreviations
ANI, average nucleotide identity; ATCC, American Type Culture Collection; CRISPR, clustered regularly interspaced short palindromic repeats; DSM(Z), Deutsche Sammlung von Mikroorganismen (und Zellkulturen); MLSA, multi locus sequence analysis; OG, orthologous groups.
Footnotes
- ^ https://github.com/ctSkennerton/minced
- ^ https://github.com/aleimba/bac-genomics-scripts
- ^ https://github.com/jvollme
- ^ https://github.com/widdowquinn/pyani
- ^ https://github.com/aleimba/bac-genomics-scripts
References
Alves, J. I., van Gelder, A. H., Alves, M. M., Sousa, D. Z., and Plugge, C. M. (2013). Moorella stamsii sp. nov., a new anaerobic thermophilic hydrogenogenic carboxydotroph isolated from digester sludge. Int. J. Syst. Evol. Microbiol. 63, 4072–4076. doi: 10.1099/ijs.0.050369-0
Andreesen, J. R., Schaupp, A., Neurauter, C., Brown, A., and Ljungdahl, L. G. (1973). Fermentation of glucose, fructose, and xylose by Clostridium thermoaceticum: effect of metals on growth yield, enzymes, and the synthesis of acetate from CO2. J. Bacteriol. 114, 743–751.
Arndt, D., Grant, J. R., Marcu, A., Sajed, T., Pon, A., Liang, Y., et al. (2016). PHASTER: a better, faster version of the PHAST phage search tool. Nucleic Acids Res. 44, W16–W21. doi: 10.1093/nar/gkw387
Balk, M., van Gelder, T., Weelink, S. A., and Stams, A. J. M. (2008). (Per)chlorate reduction by the thermophilic bacterium Moorella perchloratireducens sp. nov., isolated from underground gas storage. Appl. Environ. Microbiol. 74, 403–409. doi: 10.1128/AEM.01743-07
Bankevich, A., Nurk, S., Antipov, D., Gurevich, A. A., Dvorkin, M., Kulikov, A. S., et al. (2012). SPAdes: a new genome assembly algorithm and its applications to single-cell sequencing. J. Comp. Biol. 19, 455–477. doi: 10.1089/cmb.2012.0021
Bengelsdorf, F. R., Poehlein, A., Esser, C., Schiel-Bengelsdorf, B., Daniel, R., and Dürre, P. (2015). Complete genome sequence of the acetogenic bacterium Moorella thermoacetica DSM 2955T. Genome Announc. 3:e1157-15. doi: 10.1128/genomeA.01157-15
Bertelli, C., Laird, M. R., Williams, K. P., Simon Fraser University Research Computing Group, Lau, B. Y., Hoad, G., et al. (2017). IslandViewer 4: expanded prediction of genomic islands for larger-scale datasets. Nucleic Acids Res. 45, W30–W35. doi: 10.1093/nar/gkx343
Bland, C., Ramsey, T. L., Sabree, F., Lowe, M., Brown, K., Kyrpides, N. C., et al. (2007). CRISPR recognition tool (CRT): a tool for automatic detection of clustered regularly interspaced palindromic repeats. BMC Bioinform. 8:209. doi: 10.1186/1471-2105-8-209
Bolger, A. M., Lohse, M., and Usadel, B. (2014). Trimmomatic: a flexible trimmer for Illumina sequence data. Bioinformatics 30, 2114–2120. doi: 10.1093/bioinformatics/btu170
Byrer, D. E., Rainey, F. A., and Wiegel, J. (2000). Novel strains of Moorella thermoacetica form unusually heat-resistant spores. Arch. Microbiol. 174, 334–339. doi: 10.1007/s002030000211
Camacho, C., Coulouris, G., Avagyan, V., Ma, N., Papadopoulos, J., Bealer, K., et al. (2009). BLAST+: architecture and applications. BMC Bioinform. 10:1. doi: 10.1186/1471-2105-10-421
Carlier, J.-P., and Bedora-Faure, M. (2006). Phenotypic and genotypic characterization of some Moorella sp. strains isolated from canned foods. Syst. Appl. Microbiol. 29, 581–588. doi: 10.1016/j.syapm.2006.01.002
Carver, T., Thomson, N., Bleasby, A., Berriman, M., and Parkhill, J. (2009). DNAPlotter: circular and linear interactive genome visualization. Bioinformatics 25, 119–120. doi: 10.1093/bioinformatics/btn578
Castillo Villamizar, G. A., and Poehlein, A. (2016). Genome sequence of the acetogenic bacterium Moorella mulderi DSM 14980T. Genome Announc. 4:e444-16. doi: 10.1128/genomeA.00444-16
Castresana, J. (2000). Selection of conserved blocks from multiple alignments for their use in phylogenetic analysis. Mol. Biol. Evol. 17, 540–552. doi: 10.1093/oxfordjournals.molbev.a026334
Cato, E. P., George, W. L., and Finegold, S. M. (1986). Genus Clostridium. Bergeys Manual of Syst. Bacteriol. 2, 1141–1200.
Collins, M. D., Lawson, P. A., Willems, A., Cordoba, J. J., Fernandez-Garayzabal, J., Garcia, P., et al. (1994). The phylogeny of the genus Clostridium: proposal of five new genera and eleven new species combinations. Int. J. Syst. Bacteriol. 44, 812–826. doi: 10.1099/00207713-44-4-812
Daniel, S. L., Hsu, T., Dean, S. I., and Drake, H. L. (1990). Characterization of the H2-and CO-dependent chemolithotrophic potentials of the acetogens Clostridium thermoaceticum and Acetogenium kivui. J. Bacteriol. 172, 4464–4471. doi: 10.1128/jb.172.8.4464-4471.1990
Darling, A. E., Mau, B., and Perna, N. T. (2010). progressiveMauve: multiple genome alignment with gene gain, loss and rearrangement. PLoS One 5:e11147. doi: 10.1371/journal.pone.0011147
Das, A., Hugenholtz, J., van Halbeek, H., and Ljungdahl, L. G. (1989). Structure and function of a menaquinone involved in electron transport in membranes of Clostridium thermoautotrophicum and Clostridium thermoaceticum. J. Bacteriol. 171, 5823–5829. doi: 10.1128/jb.171.11.5823-5829.1989
Delcher, A. L., Phillippy, A., Carlton, J., and Salzberg, S. L. (2002). Fast algorithms for large-scale genome alignment and comparison. Nucleic Acids Res. 30, 2478–2483. doi: 10.1093/nar/30.11.2478
Diekert, G. B., and Thauer, R. K. (1978). Carbon monoxide oxidation by Clostridium thermoaceticum and Clostridium formicoaceticum. J. Bacteriol. 136, 597–606.
Edgar, R. C. (2004). MUSCLE: multiple sequence alignment with high accuracy and high throughput. Nucleic Acids Res. 32, 1792–1797. doi: 10.1093/nar/gkh340
Finn, R. D., Clements, J., and Eddy, S. R. (2011). HMMER web server: interactive sequence similarity searching. Nucleic Acids Res. 39, W29–W37. doi: 10.1093/nar/gkr367
Fontaine, F. E., Peterson, W. H., McCoy, E., Johnson, M. J., and Ritter, G. J. (1942). A new type of glucose fermentation by Clostridium thermoaceticum. J. Bacteriol. 43, 701–715.
Fröstl, J. M., Seifritz, C., and Drake, H. L. (1996). Effect of nitrate on the autotrophic metabolism of the acetogens Clostridium thermoautotrophicum and Clostridium thermoaceticum. J. Bacteriol. 178, 4597–4603. doi: 10.1128/jb.178.15.4597-4603.1996
Galperin, M. Y., Makarova, K. S., Wolf, Y. I., and Koonin, E. V. (2014). Expanded microbial genome coverage and improved protein family annotation in the COG database. Nucleic Acids Res. 43, D261–D269. doi: 10.1093/nar/gku1223
García-Alcalde, F., Okonechnikov, K., Carbonell, J., Cruz, L. M., Götz, S., Tarazona, S., et al. (2012). Qualimap: evaluating next-generation sequencing alignment data. Bioinformatics 28, 2678–2679. doi: 10.1093/bioinformatics/bts503
Goris, J., Konstantinidis, K. T., Klappenbach, J. A., Coenye, T., Vandamme, P., and Tiedje, J. M. (2007). DNA–DNA hybridization values and their relationship to whole-genome sequence similarities. Int. J. Syst. Evol. Microbiol. 57, 81–91. doi: 10.1099/ijs.0.64483-0
Gottwald, M., Andreesen, J. R., LeGall, J., and Ljungdahl, L. G. (1975). Presence of cytochrome and menaquinone in Clostridium formicoaceticum and Clostridium thermoaceticum. J. Bacteriol. 122, 325–328.
Henstra, A. M., Sipma, J., Rinzema, A., and Stams, A. J. M. (2007). Microbiology of synthesis gas fermentation for biofuel production. Curr. Opin. Biotechnol. 18, 200–206. doi: 10.1016/j.copbio.2007.03.008
Huson, D. H., and Scornavacca, C. (2012). Dendroscope 3: an interactive tool for rooted phylogenetic trees and networks. Syst. Biol. 61, 1061–1067. doi: 10.1093/sysbio/sys062
Hyatt, D., Chen, G.-L., LoCascio, P. F., Land, M. L., Larimer, F. W., and Hauser, L. J. (2010). Prodigal: prokaryotic gene recognition and translation initiation site identification. BMC Bioinform. 11:1. doi: 10.1186/1471-2105-11-119
Kerby, R., and Zeikus, J. G. (1983). Growth of Clostridium thermoaceticum on H2/CO2 or CO as energy source. Curr. Microbiol. 8, 27–30. doi: 10.1007/bf01567310
Kimura, Z.-I., Hoshino, T., and Murakami, K. (2016). The status of the species Moorella thermoautotrophica Wiegel et al., 1981. Request for an Opinion. Int. J. Syst. Evol. Microbiol. 66, 3249–3251. doi: 10.1099/ijsem.0.001163
Lagesen, K., Hallin, P., Rødland, E. A., Stærfeldt, H.-H., Rognes, T., and Ussery, D. W. (2007). RNAmmer: consistent and rapid annotation of ribosomal RNA genes. Nucleic Acids Res. 35, 3100–3108. doi: 10.1093/nar/gkm160
Laslett, D., and Canback, B. (2004). ARAGORN, a program to detect tRNA genes and tmRNA genes in nucleotide sequences. Nucleic Acids Res. 32, 11–16. doi: 10.1093/nar/gkh152
Latif, H., Zeidan, A. A., Nielsen, A. T., and Zengler, K. (2014). Trash to treasure: production of biofuels and commodity chemicals via syngas fermenting microorganisms. Curr. Opin. Biotechnol. 27, 79–87. doi: 10.1016/j.copbio.2013.12.001
Lechner, M., Findeiß, S., Steiner, L., Marz, M., Stadler, P. F., and Prohaska, S. J. (2011). Proteinortho: detection of (co-) orthologs in large-scale analysis. BMC Bioinform. 12:1. doi: 10.1186/1471-2105-12-124
Liebensteiner, M. G., Pinkse, M. W., Nijsse, B., Verhaert, P. D., Tsesmetzis, N., Stams, A. J., et al. (2015). Perchlorate and chlorate reduction by the Crenarchaeon Aeropyrum pernix and two thermophilic Firmicutes. Environ. Microbiol. Rep. 7, 936–945. doi: 10.1111/1758-2229.12335
Ljungdahl, L. G., and Andreesen, J. R. (1977). Formate dehydrogenase, a selenium-tungsten enzyme from Clostridium thermoaceticum. Methods Enzymol. 53, 360–372. doi: 10.1016/s0076-6879(78)53042-5
Marcellin, E., Behrendorff, J. B., Nagaraju, S., DeTissera, S., Segovia, S., Palfreyman, R. W., et al. (2016). Low carbon fuels and commodity chemicals from waste gases–systematic approach to understand energy metabolism in a model acetogen. Green Chem. 18, 3020–3028. doi: 10.1039/c5gc02708j
Marchler-Bauer, A., and Bryant, S. H. (2004). CD-Search: protein domain annotations on the fly. Nucleic Acids Res. 32, W327–W331.
Markowitz, V. M., Chen, I.-M. A., Palaniappan, K., Chu, K., Szeto, E., Pillay, M., et al. (2013). IMG 4 version of the integrated microbial genomes comparative analysis system. Nucleic Acids Res. 42, D560–D567. doi: 10.1093/nar/gkt963
Matteuzzi, D., Hollaus, F., and Biavati, B. (1978). Proposal of neotype for Clostridium thermohydrosulfuricum and the merging of Clostridium tartarivorum with Clostridium thermosaccharolyticum. Int. J. Syst. Evol. Microbiol. 28, 528–531. doi: 10.1099/00207713-28-4-528
Medema, M. H., Blin, K., Cimermancic, P., Jager, V., de, Zakrzewski, P., et al. (2011). antiSMASH: rapid identification, annotation and analysis of secondary metabolite biosynthesis gene clusters in bacterial and fungal genome sequences. Nucleic Acids Res. 39, W339–W346. doi: 10.1093/nar/gkr466
Nawrocki, E. P., and Eddy, S. R. (2013). Infernal 1.1: 100-fold faster RNA homology searches. Bioinformatics 29, 2933–2935. doi: 10.1093/bioinformatics/btt509
Parker, C. T., Tindall, B. J., and Garrity, G. M. (2019). International Code of Nomenclature of Prokaryotes (2008 revision). Int. J. Syst. Evol. Microbiol. 69, S1–S111.
Petersen, T. N., Brunak, S., von Heijne, G., and Nielsen, H. (2011). SignalP 4.0: discriminating signal peptides from transmembrane regions. Nat. Methods 8, 785–786. doi: 10.1038/nmeth.1701
Pierce, E., Xie, G., Barabote, R. D., Saunders, E., Han, C. S., Detter, J. C., et al. (2008). The complete genome sequence of Moorella thermoacetica (f. Clostridium thermoaceticum). Environ. Microbiol. 10, 2550–2573. doi: 10.1111/j.1462-2920.2008.01679.x
Poehlein, A., Bengelsdorf, F. R., Esser, C., Schiel-Bengelsdorf, B., Daniel, R., and Dürre, P. (2015). Complete genome sequence of the type strain of the acetogenic bacterium Moorella thermoacetica DSM 521T. Genome Announc. 3:e1159-15. doi: 10.1128/genomeA.01159-15
Poehlein, A., Böer, T., Steensen, K., and Daniel, R. (2018a). Draft genome sequence of the hydrogenogenic carboxydotroph Moorella stamsii DSM 26271. Genome Announc. 6:e345-18. doi: 10.1128/genomeA.00345-18
Poehlein, A., Keyl, A., Milsch, J. C., and Daniel, R. (2018b). Draft genome sequence of the thermophilic acetogen Moorella humiferrea DSM 23265. Genome Announc. 6:e357-18. doi: 10.1128/genomeA.00357-18
Redl, S., Sukumara, S., Ploeger, T., Wu, L., Jensen, T. O., Nielsen, A. T., et al. (2017). Thermodynamics and economic feasibility of acetone production from syngas using the thermophilic production host Moorella thermoacetica. Biotechnol. Biofuels 10:150. doi: 10.1186/s13068-017-0827-8
Richter, M., and Rosselló-Móra, R. (2009). Shifting the genomic gold standard for the prokaryotic species definition. Proc. Natl. Acad. Sci. U.S.A. 106, 19126–19131. doi: 10.1073/pnas.0906412106
Rijssel, M., Veen, I., and Hansen, T. A. (1992). A lithotrophic Clostridium strain with extremely thermoresistant spores isolated from a pectin-limited continuous culture of Clostridium thermosaccharolyticum strain Haren. FEMS Microbiol. Lett. 91, 171–175. doi: 10.1016/0378-1097(92)90679-i
Savage, M. D., and Drake, H. L. (1986). Adaptation of the acetogen Clostridium thermoautotrophicum to minimal medium. J. Bacteriol. 165, 315–318. doi: 10.1128/jb.165.1.315-318.1986
Schaupp, A., and Ljungdahl, L. G. (1974). Purification and properties of acetate kinase from Clostridium thermoaceticum. Arch. Microbiol. 100, 121–129. doi: 10.1007/bf00446312
Seemann, T. (2014). Prokka: rapid prokaryotic genome annotation. Bioinformatics 30, 2068–2069. doi: 10.1093/bioinformatics/btu153
Shieh, W. Y., and Jean, W. D. (1998). Alterococcus agarolyticus, gen.nov., sp.nov., a halophilic thermophilic bacterium capable of agar degradation. Can. J. Microbiol. 44, 637–645. doi: 10.1139/w98-051
Slobodkin, A., Reysenbach, A. L., Mayer, F., and Wiegel, J. (1997). Isolation and characterization of the homoacetogenic thermophilic bacterium Moorella glycerini sp. nov. Int. J. Syst. Bacteriol. 47, 969–974. doi: 10.1099/00207713-47-4-969
Stackebrandt, E., and Goebel, B. M. (1994). Taxonomic note: a place for DNA-DNA reassociation and 16S rRNA sequence analysis in the present species definition in bacteriology. Int. J. Syst. Evol. Microbiol. 44, 846–849. doi: 10.1099/00207713-44-4-846
Staden, R., Beal, K. F., and Bonfield, J. K. (1999). The Staden package, 1998. Methods Mol. Biol. 132, 115–130. doi: 10.1385/1-59259-192-2:115
Stamatakis, A. (2014). RAxML version 8: a tool for phylogenetic analysis and post-analysis of large phylogenies. Bioinformatics 30, 1312–1313. doi: 10.1093/bioinformatics/btu033
Tatusov, R. L., Koonin, E. V., and Lipman, D. J. (1997). A genomic perspective on protein families. Science 278, 631–637. doi: 10.1126/science.278.5338.631
Tindall, B. J. (2016). What does rule 18c of the international code of nomenclature of bacteria really say? Int. J. Syst. Evol. Microbiol. 66, 3622–3624. doi: 10.1099/ijsem.0.001245
Tindall, B. J., Rosselló-Móra, R., Busse, H. J., Ludwig, W., and Kämpfer, P. (2010). Notes on the characterization of prokaryote strains for taxonomic purposes. Int. J. Syst. Evol. Microbiol. 60, 249–266. doi: 10.1099/ijs.0.016949-0
Tsukahara, K., Kita, A., Nakashimada, Y., Hoshino, T., and Murakami, K. (2014). Genomeguided analysis of transformation efficiency and carbon dioxide assimilation by Moorella thermoacetica Y72. Gene 535, 150–155. doi: 10.1016/j.gene.2013.11.045
Wick, R. R., Judd, L. M., Gorrie, C. L., and Holt, K. E. (2017). Unicycler: resolving bacterial genome assemblies from short and long sequencing reads. PLoS Comput. Biol. 13:e1005595. doi: 10.1371/journal.pcbi.1005595
Wiegel, J., Braun, M., and Gottschalk, G. (1981). Clostridium thermoautotrophicum species novum, a thermophile producing acetate from molecular hydrogen and carbon dioxide. Curr. Microbiol. 5, 255–260. doi: 10.1007/bf01571158
Keywords: anaerobic, thermophile, acetogen, gas fermentation, syngas fermentation, phylogenetic analysis, Moorella, Moorella thermoacetica
Citation: Redl S, Poehlein A, Esser C, Bengelsdorf FR, Jensen TØ, Jendresen CB, Tindall BJ, Daniel R, Dürre P and Nielsen AT (2020) Genome-Based Comparison of All Species of the Genus Moorella, and Status of the Species Moorella thermoacetica and Moorella thermoautotrophica. Front. Microbiol. 10:3070. doi: 10.3389/fmicb.2019.03070
Received: 22 August 2019; Accepted: 19 December 2019;
Published: 17 January 2020.
Edited by:
Martin G. Klotz, Washington State University, United StatesReviewed by:
Charles Lovell, University of South Carolina, United StatesAlexander V. Lebedinsky, Winogradsky Institute of Microbiology (RAS), Russia
Aharon Oren, Hebrew University of Jerusalem, Israel
Copyright © 2020 Redl, Poehlein, Esser, Bengelsdorf, Jensen, Jendresen, Tindall, Daniel, Dürre and Nielsen. This is an open-access article distributed under the terms of the Creative Commons Attribution License (CC BY). The use, distribution or reproduction in other forums is permitted, provided the original author(s) and the copyright owner(s) are credited and that the original publication in this journal is cited, in accordance with accepted academic practice. No use, distribution or reproduction is permitted which does not comply with these terms.
*Correspondence: Peter Dürre, cGV0ZXIuZHVlcnJlQHVuaS11bG0uZGU=
†These authors have contributed equally to this work