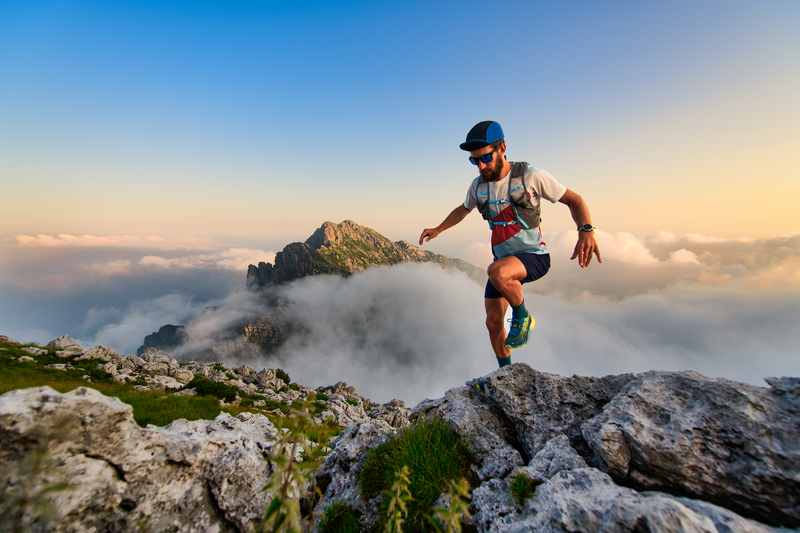
94% of researchers rate our articles as excellent or good
Learn more about the work of our research integrity team to safeguard the quality of each article we publish.
Find out more
MINI REVIEW article
Front. Microbiol. , 11 December 2019
Sec. Virology
Volume 10 - 2019 | https://doi.org/10.3389/fmicb.2019.02885
This article is part of the Research Topic Host And Pathogen Mechanisms Underpinning Viral Ecology And Emerging Infections View all 34 articles
A variety of pathogens take advantage of cellular heat shock proteins (HSPs) to complete their life cycle and exert pathogenic effects. MRJ (DNAJB6), a member of the heat shock protein 40 family, acts as a molecular chaperone for a wide range of cellular processes. MRJ mutations are linked to human diseases, such as muscular dystrophy and neurodegenerative diseases. There are two MRJ isoforms generated by alternative use of terminal exons, which differ in their C-terminus. This mini-review summarizes how these two MRJ isoforms participate differentially in viral production and virulence, and the possibility for MRJ as a therapeutic target.
Heat shock proteins (HSPs) function as molecular chaperones, thereby assisting protein folding, and non-covalent assembly or disassembly of macromolecules (Whitley et al., 1999). HSPs are structurally related proteins and classified based on their molecular weights, ranging from 10 to >100 kDa (Jee, 2016). HSP subfamily members exert similar functions across species. For example, small HSPs (HspB1 to HspB10) prevent the aggregation of misfolded proteins in an ATP-independent manner (Bakthisaran et al., 2015). ATP-dependent chaperones include Hsp60, Hsp70, and Hsp90. Hsp60 assists protein refolding throughout transport from the cytoplasm to the mitochondrial matrix (Cheng et al., 1989), while Hsp70 exerts the anti-aggregation activity with co-chaperones Hsp40 or Hsp110 (Kampinga and Craig, 2010). Hsp40 presents unfolded proteins to Hsp70 and stimulates its ATP hydrolysis (Bascos et al., 2017). Hsp90 regulates assembling, refolding and stabilizing of substrate proteins (Wandinger et al., 2008). HSPs function in a wide range of cellular processes to maintain protein homeostasis under physiological conditions and in response to environmental stresses (Hipp et al., 2019).
Invasion of pathogens, such as bacteria or viruses, may trigger cell stress responses and hence induces the production of cellular HSPs. Various viruses take advantage of cellular HSPs to overcome host environmental challenges and complete their infectious cycles (Neckers and Tatu, 2008). HSPs may participate in distinct steps during infection processes, such as viral entry, replication, and viral particle assembly and movement (Table 1). It is noteworthy that dengue virus particularly utilizes a set of Hsp70 family members for its entry, RNA replication and virion production (Taguwa et al., 2015). Moreover, some of the HSPs, particularly Hsp70, even become an integral component of virions (Santoro et al., 2009). All these findings emphasize the importance of HSPs in viral infection.
Heat shock proteins may also negatively impact viral infection. For example, two Hsp40 members inhibit the replication of human hepatitis B virus (HBV) (Sohn et al., 2006). Hsp70 interferes with nuclear import of the human immunodeficiency viruses (HIV) preinitiation complex, and viral gene expression and replication (Kumar et al., 2011). In addition, HSPs have immunomodulatory roles, although opposing. HSPs may act as a pro-inflammatory molecule by facilitating pathogenic antigen presentation on the antigen-presenting cells (Binder, 2014). On the other hand, HSPs may prevent immune activation by reducing inflammatory damages and promoting anti-inflammatory cytokines production (Hauet-Broere et al., 2006; Broere et al., 2011). Together, HSPs are engaged in both host immune response and viral pathogenesis during infection.
Mammalian relative of DnaJ (MRJ/DNAJB6) is an Hsp40 family member. The Hsp40 family can be categorized into three major types (I, II, and III), all of which share the ∼70-amino acid J-domain (Li et al., 2009; Figure 1A). In type-I Hsp40 proteins, the J-domain is at the N-terminus, followed by the glycine/phenylalanine (G/F)-rich region, the zinc finger domain, and the peptide-binding domain in the C-terminus. Type-II is similar to type-I but lacks a zinc finger domain. Type-III members contain only the J-domain, whose location differs between members (Qiu et al., 2006). Many, but not all, Hsp40 members act as cochaperones of Hsp70 by forming a heterodimer through the J-domain (Langer et al., 1992; Meacham et al., 1999; Lee et al., 2002). The J-domain of Hsp40 containing the invariant histidine-proline-aspartic acid (HPD) tripeptide stimulates the ATPase activity of Hsp70 and increases the affinity of Hsp70 for the polypeptide substrate released from Hsp40 (Summers et al., 2009). The G/F-rich region of MRJ contains several hydrophobic residues responsible for substrate recognition; phenylalanine mutations disrupt its anti-aggregation activity (Sarparanta et al., 2012; Palmio et al., 2015). The C-terminal part of MRJ contains a serine/threonine (S/T)-rich region, which is important for substrate binding (Kakkar et al., 2016). Nevertheless, Hsp40s bind and transfer non-native polypeptides to Hsp70 through distinct mechanisms, which are subject to further processing (Summers et al., 2009). In addition, the C-terminal region of MRJ is also involved in polydisperse oligomeric complexes and oligomerization (Hageman et al., 2010; Figure 1B, protein). All these functional domains are present in both splice isoforms of MRJ (see below).
Figure 1. Human MRJ gene and functions in the viral life cycle. (A) Schematic diagram of three major types of Hsp40. All contain the J-domain. The J-domain is located at the N-terminus of type I and II Hsp40 but is found at various locations within the type III members. Additional domains are as depicted. (B) Schematic diagram (upper) shows genomic organization of the human MRJ gene and its transcript isoforms that are generated by alternative splicing and polyadenylation. A reduction in the CstF64 level in macrophages favors MRJ-L production. A morpholino oligonucleotide targeting the 5’ splice site of intron 8 suppresses (MRJ-L expression. The bottom schematic diagram shows three major domains of the MRJ protein, including the J-domain followed by the G/F-rich domain in the N-terminal part, and the C-terminal peptide-binding domain, which interacts with denatured polypeptides and also directs the function of Hsp70. The conserved HPD motif of the J-domain, the SSF and SST motifs (namely the S/T-rich region) in the C-terminal peptide-binding domain and LGMD-associated mutations in the G/F domain are indicated. MRJ-S has a truncated C-terminal domain that lacks the NLS. (C) The two MRJ isoforms participate in the infection of the following viruses. HIV, MRJ-L facilitates nuclear import of the PIC via its interaction with Vpr (HIV-1) or Vpx (HIV-2), and hence facilitates the integration of the HIV genome into host chromosomes. HCMV, MRJ-L interacts with the DNA polymerase UL70 of HCMV and facilitates its nuclear import so that MRJ-L enhances viral genome synthesis. RSV, MRJ-L is essential for the expression of viral mRNAs and proteins, and viral production, but how it functions remains yet unclear. DENV: DENV replicates its genomes released from the pore of DENV-induced vesicle-like structures. The newly synthesized genome is packaged in the nucleocapsid with the capsid protein, which subsequently buds form proximal ER membranes. MRJ-S is colocalized with the viral capsid protein on the LD surface and facilitates viral assembly. Assembled viruses are transported to the Golgi apparatus for the maturation processes.)
MRJ is ubiquitously expressed in human tissues, with a higher level in the brain (Chuang et al., 2002). MRJ is upregulated during mitosis in HeLa cells perhaps to support mitotic activities (Dey et al., 2009). Human MRJ has two splice isoforms, MRJ-L and MRJ-S, generated through alternative splicing (Hanai and Mashima, 2003). The MRJ gene has ten exons. The first eight exons are included in both isoforms, while the last two exons are missing in MRJ-S (Ko et al., 2018; Figure 1B, mRNA). MRJ-L and MRJ-S proteins are comprised of 326 and 241 amino acid residues, respectively; both possess the aforementioned three functional domains. MRJ-L has a nuclear localization signal (NLS) in its very C-terminal region. Suppression of splicing in conjunction with activation of aberrant polyadenylation signals in intron 8 leads to MRJ-S expression. MRJ has been identified as a potential target transcript of cleavage stimulation factor subunit 2 (CstF64/CSTF2), a cleavage stimulation factor for mRNA 3’-end processing (Yao et al., 2012). Knockdown or overexpression of CstF64, respectively, increases and decreases the L/S isoform ratio (Ko et al., 2018). A decline in the CstF64 level during macrophage differentiation favors MRJ-L expression (Figure 1B, mRNA). In addition, serine/arginine-rich splicing factor 3 (SRSF3) may promote MRJ-S expression (Ko et al., 2018). Cyclin-dependent kinase 12 (CDK12) amplification in breast cancer results in downregulation of MRJ-L via modulating its terminal exon selection (Tien et al., 2017). Thus, the MRJ isoform ratio may be modulated in response to different cellular signals. Moreover, MRJ-L expression can be enhanced by increasing the strength of the 5’ splice site of intron 8. Single nucleotide variations in the proximal polyadenylation signal and the polypyrimidine tract of intron 8 also affect MRJ isoform ratios. Thus, both alternative splicing and alternative polyadenylation activities determine MRJ isoform expression (Ko et al., 2018).
MRJ knockout mice show embryonic lethality due in part to placental abnormalities and neural tube defects (Hunter et al., 1999; Watson et al., 2009). MRJ is involved in a variety of physiological processes, from transcription, cellular signaling to cell adhesion. MRJ suppresses the transcriptional activity of nuclear factors of activated T-cells (NFAT) by recruiting class II histone deacetylases, and hence, reduces calcineurin-induced cardiac myocyte growth. This observation suggests a role of MRJ in preventing cardiac hypertrophy (Dai et al., 2005). More notably, MRJ suppresses Wnt/β-catenin signaling through multiple pathways. Essentially, MRJ upregulates the secretary glycoprotein and Wnt inhibitor dickkopf 1 (DKK1) and maintains the dephosphorylation status of glycogen synthase kinase 3β (GSK3β) through the protein phosphatase PP2A and hence promotes degradation of β-catenin (Meng et al., 2016). This suppressive effect of MRJ on Wnt-β-catenin signaling negatively regulates tumor growth and metastases. Accordingly, a reduction of the MRJ level is present in various invasive and metastatic cancers as earlier mentioned. On the other hand, MRJ influences cytoskeletal organization, which is responsible for cell growth, division, and migration. For example, MRJ modulates intermediate filament organization via its direct interaction with keratins (Izawa et al., 2000). MRJ knockout causes actin cytoskeletal collapse in chorionic trophoblast cells (Watson et al., 2011). MRJ also contributes to cell adhesion and migration via its interaction with urokinase-type plasminogen activator receptor (uPAR) (De Bock et al., 2010; Lin et al., 2014). A recent report reveals that MRJ promotes spindle pole focusing via its interaction with dynactin, which is essential for chromosome segregation during cell division (Rosas-Salvans et al., 2019).
Genetic mutations or dysfunction of MRJ have been observed in human diseases such as limb-girdle muscular dystrophy (LGMD), myopathy and neurodegenerative diseases. Phenylalanine mutations in the (G/F)-rich region of MRJ are found in LGMD and distal myopathy, indicating that the chaperone activity of MRJ is critical for preventing proteinopathy (Harms et al., 2012; Sarparanta et al., 2012; Li et al., 2016; Jonson et al., 2018). MRJ mutations result in myofibrillar aggregates containing ubiquitin, ubiquitin-binding protein p62 and TAR DNA-binding protein 43 (TDP-43) (Sato et al., 2013; Sandell et al., 2016). Notably, TDP-43 aggregation is a characteristics of amyotrophic lateral sclerosis (Tamaki et al., 2018), emphasizing the pathological effect of defective MRJ in neurodegenerative disorders. The C-terminal S/T-rich region in MRJ exhibits the suppressive effect on the formation of different aggregation-prone peptides such as amyloid-β and polyglutamine peptides that are involved in the pathogenesis of Alzheimer’s disease and Huntington’s disease, respectively (Kakkar et al., 2016; Mansson et al., 2018; Bason et al., 2019). MRJ has also been implicated in Parkinson’s disease. Upregulation of MRJ in parkinsonian astrocytes prevents the neuronal release of α-synuclein/SNCA, which has the potential to form toxic aggregates, suggesting a protective role of MRJ (Durrenberger et al., 2009; Aprile et al., 2017). A more recent study indicates that the chaperone activity of MRJ also suppresses mutant parkin aggregation (Kakkar et al., 2016). Thus, it is likely that the chaperone function of MRJ contributes to preventing protein misfolding in neurodegenerative diseases.
In addition to the cellular functions above mentioned, both MRJ isoforms have been implicated in infection and pathogenesis of multiple human viruses. A recent report unveils the involvement of a translocon complex factor, Sec61, in the biogenesis of several different viral proteins, suggesting that targeting Sec61 can provide an antiviral strategy against multiple viruses (Heaton et al., 2016). In light of this finding, we review the roles of MRJ in infection and propagation of several viruses and discuss the potential of targeting MRJ as an antiviral strategy.
Human immunodeficiency viruses is a retrovirus that causes acquired immunodeficiency syndrome (AIDS), which destroys the immune system of infections (Sharp and Hahn, 2011). HIV infects macrophages and CD4+ T helper cells through the CD4 receptor and its coreceptor, i.e., chemokine receptor CCR5 or CXCR4 (Maartens et al., 2014). After infection, HIV is integrated into the human genome, which is essential for the viral life cycle (Moir et al., 2011). For integration, HIV establishes the pre-integration complex (PIC), consisting of the cDNA that is converted from its genomic RNA and several cellular and viral proteins including the viral protein R (Vpr). Vpr participates in proviral integration into the host genome (Chiang et al., 2014; Pirrone et al., 2014). MRJ-L facilitates nuclear localization of the HIV-1 pre-integration complex via its direct interaction with Vpr (Chiang et al., 2014). As compared to MRJ-L, MRJ-S displays a relatively weak activity in nuclear localization of Vpr/Vpx likely due to its lack of the C-terminal NLS of MRJ-L (Cheng et al., 2008; Chiang et al., 2014). Notably, mutations in the HPD motif of MRJ-L disrupt the activity of MRJ-L in facilitating Vpx (or HIV) nuclear import, indicating the involvement of Hsp70 (Cheng et al., 2008). Analogously, MRJ-L assists nuclear import of the HIV-2 viral protein X (Vpx), the paralog of HIV-1 Vpr. Depletion of MRJ-L restricts HIV-2 replication due to reduced nuclear import of the PIC (Cheng et al., 2008). On the other hand, overexpression of MRJ-S suppresses HIV proviral transcription and hence compromises HIV-1 production (Urano et al., 2013). These observations together suggest that a higher L/S ratio of MRJ may promote HIV infection (Figure 1C, HIV). A cohort study reveals that HIV-infected individuals indeed exhibit a slightly higher level of MRJ-L in macrophages than healthy subjects (Chiang et al., 2014), supporting the positive role of MRJ-L in HIV-1 infection. It is speculated that cis-element polymorphisms of MRJ that favor L isoform expression may increase the probability of HIV infection (Ko et al., 2018). Therefore, the MRJ-L level difference between individuals may predict HIV susceptibility. On the other hand, the negative regulatory factor (Nef) of HIV facilitates nuclear translocation of Hsp40, which subsequently facilitates viral gene expression. Nevertheless, Hsp70 can counteract the nuclear import of Vpr-mediated PIC complex and hence inhibits viral replication (Iordanskiy et al., 2004).
Human cytomegalovirus is a common opportunistic pathogen that may establish long-life latency without any symptoms in healthy individuals but may threaten immunocompromised individuals and neonates (Kenneson and Cannon, 2007). HCMV has the largest genome among the human herpesviruses and replicates in the nucleus of cells. The HCMV DNA-dependent RNA polymerase, i.e., the primase UL70, forms the helicase-primase complex with UL102/105 to synthesize short RNA primers for viral DNA replication (McMahon and Anders, 2002). MRJ-L interacts with UL70 through a fragment containing the G/F-rich region and facilitates nuclear entry of UL70, thereby promoting viral DNA synthesis (Figure 1C, HCMV). On the other hand, MRJ-S is co-localized with the primase in the cytoplasm that reduces viral genome expression and synthesis (Pei et al., 2012). Thus, MRJ isoforms differentially modulate HCMV replication. Reduction of the MRJ-L expression level conceivably inhibits viral lytic infection and can be used as an anti-HCMV strategy (Biron, 2006).
Human RSV causes lower respiratory tract infection in infants and children worldwide. RSV infection shows a higher risk of mortality compared to seasonal influenza infection in elder individuals (Kwon et al., 2017). RSV belongs to the Paramyxoviridae family, consisting of a negative-sense single-stranded RNA genome that replicates in the host cytoplasm. The viral RNA-dependent RNA polymerase is responsible for both viral transcription and replication (Noton et al., 2019). Intriguingly, knockdown of MRJ-L reduces viral mRNA and protein expression and virion production, while depletion of MRJ-S has no such effects (Ko et al., 2018), indicating the critical role of MRJ-L in RSV propagation. Nevertheless, whether MRJ-L interferes with the RNA polymerase activity of RSV remains to be determined. Additionally, whether the nuclear localization property of MRJ-L is required for RSV viral production also remains puzzling (Figure 1C, RSV). If this were the case, it would be interesting to elucidate why an RNA virus, which completes its life cycle in the cytoplasm, requires the nuclear function(s) of MRJ-L for propagation.
Dengue virus is a mosquito-transmitted pathogen and its infection may cause haemorrhagic fever (Brady et al., 2012; Bhatt et al., 2013). DENV is a member of the Flaviviridae family with a positive-sense single-stranded RNA genome. Viral genome replication and package solely occur in the host cytoplasm. DENV infection induces autophagy that targets cellular lipid droplets (LDs), which are endoplasmic reticulum-derived storage organelles of neutral lipids, and hence stimulates lipid metabolism (Randall, 2018). On the other hand, LDs are essential for DENV production. During virion assembly, the DENV capsid protein binds to an LD surface protein and forms the nucleocapsids with viral genomes in the endoplasmic reticulum (Samsa et al., 2009; Heaton and Randall, 2010). HSPs participate in multiple steps in the DENV life cycle (Taguwa et al., 2015). Among them, MRJ-S is colocalized with the capsid protein on LDs and aids viral particle assembly (Taguwa et al., 2015; Figure 1C, DENV). Depletion of MRJ-S impairs viral RNA replication and virion production (Taguwa et al., 2015). MRJ-S with mutations in the HPD motif fails to rescue viral production in MRJ-S-depleted cells, indicating the cooperative role of MRJ-S and Hsp70 in viral particle biogenesis. Nevertheless, MRJ-L is not engaged in the process of DENV propagation (Taguwa et al., 2015).
Antisense morpholino oligonucleotides targeting viral RNAs or host mRNAs that encode proteins essential for viral propagation have been designed for treatment of viral infection (Warren et al., 2012). For example, a splice switching morpholino oligonucleotide can restrict influenza viral replication by suppressing exon inclusion of the host transmembrane serine protease 2 (TMPRSS2) (Bottcher-Friebertshauser et al., 2011). In light of the findings that MRJ is involved in viral propagation, it is possible to interfere with viral infection by targeting MRJ or modulating its splice isoform expression. Depletion of MRJ-L by siRNAs inhibits viral life cycles of HCMV and HIV (Cheng et al., 2008; Pei et al., 2012). Our recent report shows that a morpholino oligonucleotide complementary to the 5’ splice site of MRJ intron 8 efficiently inhibits MRJ-L expression in vitro (Ko et al., 2018). This morpholino disrupts the propagation of both pesudotyped and native HIV-1 in macrophage-like cells, and also effectively restricts subgenomic synthesis of RSV (Ko et al., 2018). It is likewise possible that masking the polyadenylation signal in intron 8 can suppress MRJ-S production. Since flaviviruses share a similar viral processing mechanism in LDs, it would be interesting to know whether MRJ-S-targeting agents may have a broad-spectrum antiviral effect. Since small molecule splicing modulators developed recently demonstrate their therapeutic potentials (Bates et al., 2017), it is worthy to evaluate whether any of them could influence MRJ isoform ratios, and hence impact viral infection. As described above, MRJ acts as an efficient suppressor of polyglutamine aggregation (Chuang et al., 2002). Therefore, harnessing the expression or chaperone activity of MRJ would be used for treatment of neurodegenerative disorders. Together, MRJ holds a great potential as drug targets.
It has been demonstrated that single nucleotide polymorphisms near splice sites or polyadenylation sites of MRJ affect its isoform expression ratio (Ko et al., 2018). Thus, individuals may vary their susceptibility to viral infection, cancer or other disorders. Although the chaperone activity of MRJ is likely important for protein proteostasis, particularly disease-causing proteins, it is yet unclear whether this activity is critical for its various functions in viral infection. Nevertheless, the importance of the MRJ HPD motif in supporting HIV and dengue viral production suggests that the ATPase activity of Hsp70 contributes to viral propagation. Identification of small molecule compounds that selectively target HSPs has the values in prevention and treatment of viral infection. It has been demonstrated that Hsp70 inhibitors exert substantial antiviral activities against DENV as well as other flaviviruses (Taguwa et al., 2015, 2019). Small molecules targeting different domains of Hsp90 or interfering with its cochaperone or substrate protein binding have also shown the potential in therapeutic treatment of cancer or neurodegenerative disorders (Shrestha et al., 2016). However, pharmacologically manipulating the activity of Hsp40 is not yet available. Therefore, to have a better understanding of the isoform expression and domain structure-function relations of MRJ would be important for drug design toward viral infection.
S-HK and W-YT designed the figures and wrote the manuscript. L-MH and W-YT edited the manuscript.
The authors acknowledge the funding supported by an intramural grant from the National Taiwan University to L-MH and M-RC, an NTU-Academia Sinica grant (NTU-AS-106R104514 to L-MH and W-YT), and the Ministry of Science and Technology, Taiwan (Grant 103-2314-B-002-056- MY3 to L-MH).
The authors declare that the research was conducted in the absence of any commercial or financial relationships that could be construed as a potential conflict of interest.
Aprile, F. A., Kallstig, E., Limorenko, G., Vendruscolo, M., Ron, D., and Hansen, C. (2017). The molecular chaperones DNAJB6 and Hsp70 cooperate to suppress alpha-synuclein aggregation. Sci. Rep. 7:9039. doi: 10.1038/s41598-017-08324-z
Bakthisaran, R., Tangirala, R., and Rao, Ch, M. (2015). Small heat shock proteins: role in cellular functions and pathology. Biochim. Biophys. Acta 1854, 291–319. doi: 10.1016/j.bbapap.2014.12.019
Baquero-Perez, B., and Whitehouse, A. (2015). Hsp70 isoforms are essential for the formation of Kaposi’s Sarcoma-associated herpesvirus replication and transcription compartments. PLoS Pathog. 11:e1005274. doi: 10.1371/journal.ppat.1005274
Bascos, N. A. D., Mayer, M. P., Bukau, B., and Landry, S. J. (2017). The Hsp40 J-domain modulates Hsp70 conformation and ATPase activity with a semi-elliptical spring. Protein Sci. 26, 1838–1851. doi: 10.1002/pro.3223
Bason, M., Meister-Broekema, M., Alberts, N., Dijkers, P., Bergink, S., Sibon, O. C. M., et al. (2019). Astrocytic expression of the chaperone DNAJB6 results in non-cell autonomous protection in Huntington’s disease. Neurobiol. Dis. 124, 108–117. doi: 10.1016/j.nbd.2018.10.017
Bates, D. O., Morris, J. C., Oltean, S., and Donaldson, L. F. (2017). Pharmacology of modulators of alternative splicing. Pharmacol. Rev. 69, 63–79. doi: 10.1124/pr.115.011239
Batra, J., Tripathi, S., Kumar, A., Katz, J. M., Cox, N. J., Lal, R. B., et al. (2016). Human Heat shock protein 40 (Hsp40/DnaJB1) promotes influenza A virus replication by assisting nuclear import of viral ribonucleoproteins. Sci. Rep. 6:19063. doi: 10.1038/srep19063
Bhatt, S., Gething, P. W., Brady, O. J., Messina, J. P., Farlow, A. W., Moyes, C. L., et al. (2013). The global distribution and burden of dengue. Nature 496, 504–507. doi: 10.1038/nature12060
Binder, R. J. (2014). Functions of heat shock proteins in pathways of the innate and adaptive immune system. J. Immunol. 193, 5765–5771. doi: 10.4049/jimmunol.1401417
Biron, K. K. (2006). Antiviral drugs for cytomegalovirus diseases. Antiviral Res. 71, 154–163. doi: 10.1016/j.antiviral.2006.05.002
Bottcher-Friebertshauser, E., Stein, D. A., Klenk, H. D., and Garten, W. (2011). Inhibition of influenza virus infection in human airway cell cultures by an antisense peptide-conjugated morpholino oligomer targeting the hemagglutinin-activating protease TMPRSS2. J. Virol. 85, 1554–1562. doi: 10.1128/JVI.01294-10
Brady, O. J., Gething, P. W., Bhatt, S., Messina, J. P., Brownstein, J. S., Hoen, A. G., et al. (2012). Refining the global spatial limits of dengue virus transmission by evidence-based consensus. PLoS Negl. Trop. Dis. 6:e1760. doi: 10.1371/journal.pntd.0001760
Broere, F., Van Der Zee, R., and Van Eden, W. (2011). Heat shock proteins are no DAMPs, rather ‘DAMPERs’. Nat. Rev. Immunol. 11:565. doi: 10.1038/nri2873-c1
Brown, G., Rixon, H. W., Steel, J., Mcdonald, T. P., Pitt, A. R., Graham, S., et al. (2005). Evidence for an association between heat shock protein 70 and the respiratory syncytial virus polymerase complex within lipid-raft membranes during virus infection. Virology 338, 69–80. doi: 10.1016/j.virol.2005.05.004
Cao, M., Wei, C., Zhao, L., Wang, J., Jia, Q., Wang, X., et al. (2014). DnaJA1/Hsp40 is co-opted by influenza A virus to enhance its viral RNA polymerase activity. J. Virol. 88, 14078–14089. doi: 10.1128/JVI.02475-14
Cheng, M. Y., Hartl, F. U., Martin, J., Pollock, R. A., Kalousek, F., Neupert, W., et al. (1989). Mitochondrial heat-shock protein hsp60 is essential for assembly of proteins imported into yeast mitochondria. Nature 337, 620–625. doi: 10.1038/337620a0
Cheng, X., Belshan, M., and Ratner, L. (2008). Hsp40 facilitates nuclear import of the human immunodeficiency virus type 2 Vpx-mediated preintegration complex. J. Virol. 82, 1229–1237. doi: 10.1128/jvi.00540-07
Chiang, Y. P., Sheng, W. H., Shao, P. L., Chi, Y. H., Chen, Y. M., Huang, S. W., et al. (2014). Large isoform of mammalian relative of DnaJ is a major determinant of human susceptibility to HIV-1 infection. EBioMedicine 1, 126–132. doi: 10.1016/j.ebiom.2014.10.002
Chuang, J. Z., Zhou, H., Zhu, M., Li, S. H., Li, X. J., and Sung, C. H. (2002). Characterization of a brain-enriched chaperone, MRJ, that inhibits Huntingtin aggregation and toxicity independently. J. Biol. Chem. 277, 19831–19838. doi: 10.1074/jbc.m109613200
Dai, Y. S., Xu, J., and Molkentin, J. D. (2005). The DnaJ-related factor Mrj interacts with nuclear factor of activated T cells c3 and mediates transcriptional repression through class II histone deacetylase recruitment. Mol. Cell Biol. 25, 9936–9948. doi: 10.1128/mcb.25.22.9936-9948.2005
De Bock, C. E., Lin, Z., Mekkawy, A. H., Byrne, J. A., and Wang, Y. (2010). Interaction between urokinase receptor and heat shock protein MRJ enhances cell adhesion. Int. J. Oncol. 36, 1155–1163.
Dey, S., Banerjee, P., and Saha, P. (2009). Cell cycle specific expression and nucleolar localization of human J-domain containing co-chaperone Mrj. Mol. Cell Biochem. 322, 137–142. doi: 10.1007/s11010-008-9950-y
Durrenberger, P. F., Filiou, M. D., Moran, L. B., Michael, G. J., Novoselov, S., Cheetham, M. E., et al. (2009). DnaJB6 is present in the core of Lewy bodies and is highly up-regulated in parkinsonian astrocytes. J. Neurosci. Res. 87, 238–245. doi: 10.1002/jnr.21819
Dutta, D., Chattopadhyay, S., Bagchi, P., Halder, U. C., Nandi, S., Mukherjee, A., et al. (2011). Active participation of cellular chaperone Hsp90 in regulating the function of rotavirus nonstructural protein 3 (NSP3). J. Biol. Chem. 286, 20065–20077. doi: 10.1074/jbc.m111.231878
Gonzalez, O., Fontanes, V., Raychaudhuri, S., Loo, R., Loo, J., Arumugaswami, V., et al. (2009). The heat shock protein inhibitor Quercetin attenuates hepatitis C virus production. Hepatology 50, 1756–1764. doi: 10.1002/hep.23232
Gurer, C., Hoglund, A., Hoglund, S., and Luban, J. (2005). ATPgammaS disrupts human immunodeficiency virus type 1 virion core integrity. J. Virol. 79, 5557–5567. doi: 10.1128/jvi.79.9.5557-5567.2005
Hageman, J., Rujano, M. A., Van Waarde, M. A., Kakkar, V., Dirks, R. P., Govorukhina, N., et al. (2010). A DNAJB chaperone subfamily with HDAC-dependent activities suppresses toxic protein aggregation. Mol. Cell 37, 355–369. doi: 10.1016/j.molcel.2010.01.001
Hanai, R., and Mashima, K. (2003). Characterization of two isoforms of a human DnaJ homologue. HSJ2. Mol. Biol. Rep. 30, 149–153.
Harms, M. B., Sommerville, R. B., Allred, P., Bell, S., Ma, D., Cooper, P., et al. (2012). Exome sequencing reveals DNAJB6 mutations in dominantly-inherited myopathy. Ann. Neurol. 71, 407–416. doi: 10.1002/ana.22683
Hauet-Broere, F., Wieten, L., Guichelaar, T., Berlo, S., Van Der Zee, R., and Van Eden, W. (2006). Heat shock proteins induce T cell regulation of chronic inflammation. Ann. Rheum. Dis. 65(Suppl. 3), iii65–iii68. doi: 10.1136/ard.2006.058495
Heaton, N. S., Moshkina, N., Fenouil, R., Gardner, T. J., Aguirre, S., Shah, P. S., et al. (2016). Targeting viral proteostasis limits influenza virus, hiv, and dengue virus infection. Immunity 44, 46–58. doi: 10.1016/j.immuni.2015.12.017
Heaton, N. S., and Randall, G. (2010). Dengue virus-induced autophagy regulates lipid metabolism. Cell Host Microbe 8, 422–432. doi: 10.1016/j.chom.2010.10.006
Hipp, M. S., Kasturi, P., and Hartl, F. U. (2019). The proteostasis network and its decline in ageing. Nat. Rev. Mol. Cell Biol. 20, 421–435. doi: 10.1038/s41580-019-0101-y
Hunter, P. J., Swanson, B. J., Haendel, M. A., Lyons, G. E., and Cross, J. C. (1999). Mrj encodes a DnaJ-related co-chaperone that is essential for murine placental development. Development 126, 1247–1258.
Iordanskiy, S., Zhao, Y., Dimarzio, P., Agostini, I., Dubrovsky, L., and Bukrinsky, M. (2004). Heat-shock protein 70 exerts opposing effects on Vpr-dependent and Vpr-independent HIV-1 replication in macrophages. Blood 104, 1867–1872. doi: 10.1182/blood-2004-01-0081
Izawa, I., Nishizawa, M., Ohtakara, K., Ohtsuka, K., Inada, H., and Inagaki, M. (2000). Identification of Mrj, a DnaJ/Hsp40 family protein, as a keratin 8/18 filament regulatory protein. J. Biol. Chem. 275, 34521–34527. doi: 10.1074/jbc.m003492200
Jee, H. (2016). Size dependent classification of heat shock proteins: a mini-review. J. Exerc. Rehabil. 12, 255–259. doi: 10.12965/jer.1632642.321
Jonson, P. H., Palmio, J., Johari, M., Penttila, S., Evila, A., Nelson, I., et al. (2018). Novel mutations in DNAJB6 cause LGMD1D and distal myopathy in French families. Eur. J. Neurol. 25, 790–794. doi: 10.1111/ene.13598
Kakkar, V., Mansson, C., De Mattos, E. P., Bergink, S., Van Der Zwaag, M., Van Waarde, M., et al. (2016). The S/T-Rich motif in the DNAJB6 chaperone delays polyglutamine aggregation and the onset of disease in a mouse model. Mol. Cell 62, 272–283. doi: 10.1016/j.molcel.2016.03.017
Kampinga, H. H., and Craig, E. A. (2010). The HSP70 chaperone machinery: J proteins as drivers of functional specificity. Nat. Rev. Mol. Cell Biol. 11, 579–592. doi: 10.1038/nrm2941
Katoh, H., Kubota, T., Kita, S., Nakatsu, Y., Aoki, N., Mori, Y., et al. (2015). Heat shock protein 70 regulates degradation of the mumps virus phosphoprotein via the ubiquitin-proteasome pathway. J. Virol. 89, 3188–3199. doi: 10.1128/JVI.03343-14
Katoh, H., Kubota, T., Nakatsu, Y., Tahara, M., Kidokoro, M., and Takeda, M. (2017). Heat shock protein 90 ensures efficient mumps virus replication by assisting with viral polymerase complex formation. J. Virol. 91:e02220. doi: 10.1128/JVI.02220-16
Kenneson, A., and Cannon, M. J. (2007). Review and meta-analysis of the epidemiology of congenital cytomegalovirus (CMV) infection. Rev. Med. Virol. 17, 253–276. doi: 10.1002/rmv.535
Ko, S. H., Liau, Y. J., Chi, Y. H., Lai, M. J., Chiang, Y. P., Lu, C. Y., et al. (2018). Interference of DNAJB6/MRJ isoform switch by morpholino inhibits replication of HIV-1 and RSV. Mol. Ther. Nucleic Acids 14, 251–261. doi: 10.1016/j.omtn.2018.12.001
Kumar, M., Rawat, P., Khan, S. Z., Dhamija, N., Chaudhary, P., Ravi, D. S., et al. (2011). Reciprocal regulation of human immunodeficiency virus-1 gene expression and replication by heat shock proteins 40 and 70. J. Mol. Biol. 410, 944–958. doi: 10.1016/j.jmb.2011.04.005
Kwon, Y. S., Park, S. H., Kim, M. A., Kim, H. J., Park, J. S., Lee, M. Y., et al. (2017). Risk of mortality associated with respiratory syncytial virus and influenza infection in adults. BMC Infect. Dis. 17:785. doi: 10.1186/s12879-017-2897-4
Langer, T., Lu, C., Echols, H., Flanagan, J., Hayer, M. K., and Hartl, F. U. (1992). Successive action of DnaK, DnaJ and GroEL along the pathway of chaperone-mediated protein folding. Nature 356, 683–689. doi: 10.1038/356683a0
Lee, S., Fan, C. Y., Younger, J. M., Ren, H., and Cyr, D. M. (2002). Identification of essential residues in the type II Hsp40 Sis1 that function in polypeptide binding. J. Biol. Chem. 277, 21675–21682. doi: 10.1074/jbc.m111075200
Li, J., Qian, X., and Sha, B. (2009). Heat shock protein 40: structural studies and their functional implications. Protein Pept. Lett. 16, 606–612. doi: 10.2174/092986609788490159
Li, S., Zhang, P., Freibaum, B. D., Kim, N. C., Kolaitis, R. M., Molliex, A., et al. (2016). Genetic interaction of hnRNPA2B1 and DNAJB6 in a Drosophila model of multisystem proteinopathy. Hum. Mol. Genet. 25, 936–950. doi: 10.1093/hmg/ddv627
Lin, Y., Peng, N., Zhuang, H., Zhang, D., Wang, Y., and Hua, Z. C. (2014). Heat shock proteins HSP70 and MRJ cooperatively regulate cell adhesion and migration through urokinase receptor. BMC Cancer 14:639. doi: 10.1186/1471-2407-14-639
Maartens, G., Celum, C., and Lewin, S. R. (2014). HIV infection: epidemiology, pathogenesis, treatment, and prevention. Lancet 384, 258–271. doi: 10.1016/s0140-6736(14)60164-1
Mansson, C., Van Cruchten, R. T. P., Weininger, U., Yang, X., Cukalevski, R., Arosio, P., et al. (2018). Conserved S/T residues of the human chaperone DNAJB6 are required for effective inhibition of abeta42 amyloid fibril formation. Biochemistry 57, 4891–4902. doi: 10.1021/acs.biochem.8b00353
McMahon, T. P., and Anders, D. G. (2002). Interactions between human cytomegalovirus helicase-primase proteins. Virus Res. 86, 39–52. doi: 10.1016/s0168-1702(02)00054-0
Meacham, G. C., Lu, Z., King, S., Sorscher, E., Tousson, A., and Cyr, D. M. (1999). The Hdj-2/Hsc70 chaperone pair facilitates early steps in CFTR biogenesis. EMBO J. 18, 1492–1505. doi: 10.1093/emboj/18.6.1492
Meng, E., Shevde, L. A., and Samant, R. S. (2016). Emerging roles and underlying molecular mechanisms of DNAJB6 in cancer. Oncotarget 7, 53984–53996. doi: 10.18632/oncotarget.9803
Moir, S., Chun, T. W., and Fauci, A. S. (2011). Pathogenic mechanisms of HIV disease. Annu. Rev. Pathol. 6, 223–248. doi: 10.1146/annurev-pathol-011110-130254
Momose, F., Naito, T., Yano, K., Sugimoto, S., Morikawa, Y., and Nagata, K. (2002). Identification of Hsp90 as a stimulatory host factor involved in influenza virus RNA synthesis. J. Biol. Chem. 277, 45306–45314. doi: 10.1074/jbc.m206822200
Naito, T., Momose, F., Kawaguchi, A., and Nagata, K. (2007). Involvement of Hsp90 in assembly and nuclear import of influenza virus RNA polymerase subunits. J. Virol. 81, 1339–1349. doi: 10.1128/jvi.01917-06
Neckers, L., and Tatu, U. (2008). Molecular chaperones in pathogen virulence: emerging new targets for therapy. Cell Host Microbe 4, 519–527. doi: 10.1016/j.chom.2008.10.011
Noton, S. L., Tremaglio, C. Z., and Fearns, R. (2019). Killing two birds with one stone: how the respiratory syncytial virus polymerase initiates transcription and replication. PLoS Pathog. 15:e1007548. doi: 10.1371/journal.ppat.1007548
Palmio, J., Jonson, P. H., Evila, A., Auranen, M., Straub, V., Bushby, K., et al. (2015). Novel mutations in DNAJB6 gene cause a very severe early-onset limb-girdle muscular dystrophy 1D disease. Neuromuscul. Disord. 25, 835–842. doi: 10.1016/j.nmd.2015.07.014
Park, S. G., Lim, S. O., and Jung, G. (2002). Binding site analysis of human HBV pol for molecular chaperonin, hsp60. Virology 298, 116–123. doi: 10.1006/viro.2002.1496
Pei, Y., Fu, W., Yang, E., Shen, A., Chen, Y. C., Gong, H., et al. (2012). A Hsp40 chaperone protein interacts with and modulates the cellular distribution of the primase protein of human cytomegalovirus. PLoS Pathog. 8:e1002968. doi: 10.1371/journal.ppat.1002968
Pirrone, V., Mell, J., Janto, B., and Wigdahl, B. (2014). Biomarkers of HIV susceptibility and disease progression. EBioMedicine 1, 99–100. doi: 10.1016/j.ebiom.2014.11.003
Qiu, X. B., Shao, Y. M., Miao, S., and Wang, L. (2006). The diversity of the DnaJ/Hsp40 family, the crucial partners for Hsp70 chaperones. Cell Mol. Life Sci. 63, 2560–2570. doi: 10.1007/s00018-006-6192-6
Radhakrishnan, A., Yeo, D., Brown, G., Myaing, M. Z., Iyer, L. R., Fleck, R., et al. (2010). Protein analysis of purified respiratory syncytial virus particles reveals an important role for heat shock protein 90 in virus particle assembly. Mol. Cell Proteomics 9, 1829–1848. doi: 10.1074/mcp.M110.001651
Randall, G. (2018). Lipid droplet metabolism during dengue virus infection. Trends Microbiol. 26, 640–642. doi: 10.1016/j.tim.2018.05.010
Rosas-Salvans, M., Scrofani, J., Modol, A., and Vernos, I. (2019). DnaJB6 is a RanGTP-regulated protein required for microtubule organization during mitosis. J. Cell Sci. 132:jcs227033. doi: 10.1242/jcs.227033
Samsa, M. M., Mondotte, J. A., Iglesias, N. G., Assuncao-Miranda, I., Barbosa-Lima, G., Da Poian, A. T., et al. (2009). Dengue virus capsid protein usurps lipid droplets for viral particle formation. PLoS Pathog. 5:e1000632. doi: 10.1371/journal.ppat.1000632
Sandell, S., Huovinen, S., Palmio, J., Raheem, O., Lindfors, M., Zhao, F., et al. (2016). Diagnostically important muscle pathology in DNAJB6 mutated LGMD1D. Acta Neuropathol. Commun. 4:9. doi: 10.1186/s40478-016-0276-9
Santoro, M. G., Amici, C., and Rossi, A. (2009). “Role of hear shock proteins in viral infection,” in Prokaryotic and Eukaryotic Heat Shock Proteins in Infectious Disease Heat Shock Proteins, Vol. 4, eds A. G. Pockley, S. K. Calderwood, and M. G. Santoro (Springer), 51–84. doi: 10.1007/978-90-481-2976-8_3
Sarparanta, J., Jonson, P. H., Golzio, C., Sandell, S., Luque, H., Screen, M., et al. (2012). Mutations affecting the cytoplasmic functions of the co-chaperone DNAJB6 cause limb-girdle muscular dystrophy. Nat. Genet. 44, S451–S452. doi: 10.1038/ng.1103
Sato, T., Hayashi, Y. K., Oya, Y., Kondo, T., Sugie, K., Kaneda, D., et al. (2013). DNAJB6 myopathy in an Asian cohort and cytoplasmic/nuclear inclusions. Neuromuscul. Disord. 23, 269–276. doi: 10.1016/j.nmd.2012.12.010
Sharp, P. M., and Hahn, B. H. (2011). Origins of HIV and the AIDS pandemic. Cold Spring Harb. Perspect. Med. 1:a006841. doi: 10.1101/cshperspect.a006841
Shrestha, L., Bolaender, A., Patel, H. J., and Taldone, T. (2016). Heat shock protein (HSP) drug discovery and development: targeting heat shock proteins in disease. Curr. Top. Med. Chem. 16, 2753–2764. doi: 10.2174/1568026616666160413141911
Sohn, S. Y., Kim, S. B., Kim, J., and Ahn, B. Y. (2006). Negative regulation of hepatitis B virus replication by cellular Hsp40/DnaJ proteins through destabilization of viral core and X proteins. J. Gen. Virol. 87, 1883–1891. doi: 10.1099/vir.0.81684-0
Summers, D. W., Douglas, P. M., Ramos, C. H., and Cyr, D. M. (2009). Polypeptide transfer from Hsp40 to Hsp70 molecular chaperones. Trends Biochem Sci 34, 230–233. doi: 10.1016/j.tibs.2008.12.009
Taguwa, S., Maringer, K., Li, X., Bernal-Rubio, D., Rauch, J. N., Gestwicki, J. E., et al. (2015). Defining Hsp70 subnetworks in dengue virus replication reveals key vulnerability in flavivirus infection. Cell 163, 1108–1123. doi: 10.1016/j.cell.2015.10.046
Taguwa, S., Yeh, M. T., Rainbolt, T. K., Nayak, A., Shao, H., Gestwicki, J. E., et al. (2019). Zika virus dependence on host Hsp70 provides a protective strategy against infection and disease. Cell Rep. 26:e903. doi: 10.1016/j.celrep.2018.12.095
Tamaki, Y., Shodai, A., Morimura, T., Hikiami, R., Minamiyama, S., Ayaki, T., et al. (2018). Elimination of TDP-43 inclusions linked to amyotrophic lateral sclerosis by a misfolding-specific intrabody with dual proteolytic signals. Sci. Rep. 8:6030. doi: 10.1038/s41598-018-24463-3
Tanaka, Y., Kanai, F., Kawakami, T., Tateishi, K., Ijichi, H., Kawabe, T., et al. (2004). Interaction of the hepatitis B virus X protein (HBx) with heat shock protein 60 enhances HBx-mediated apoptosis. Biochem. Biophys. Res. Commun. 318, 461–469. doi: 10.1016/j.bbrc.2004.04.046
Tien, J. F., Mazloomian, A., Cheng, S. G., Hughes, C. S., Chow, C. C. T., Canapi, L. T., et al. (2017). CDK12 regulates alternative last exon mRNA splicing and promotes breast cancer cell invasion. Nucleic Acids Res. 45, 6698–6716. doi: 10.1093/nar/gkx187
Ujino, S., Yamaguchi, S., Shimotohno, K., and Takaku, H. (2009). Heat-shock protein 90 is essential for stabilization of the hepatitis C virus nonstructural protein NS3. J. Biol. Chem. 284, 6841–6846. doi: 10.1074/jbc.M806452200
Urano, E., Morikawa, Y., and Komano, J. (2013). Novel role of HSP40/DNAJ in the regulation of HIV-1 replication. J. Acquir. Immune Defic. Syndr. 64, 154–162. doi: 10.1097/QAI.0b013e31829a2ef8
Wandinger, S. K., Richter, K., and Buchner, J. (2008). The Hsp90 chaperone machinery. J. Biol. Chem. 283, 18473–18477. doi: 10.1074/jbc.r800007200
Warren, T. K., Shurtleff, A. C., and Bavari, S. (2012). Advanced morpholino oligomers: a novel approach to antiviral therapy. Antiviral Res. 94, 80–88. doi: 10.1016/j.antiviral.2012.02.004
Watson, E. D., Hughes, M., Simmons, D. G., Natale, D. R., Sutherland, A. E., and Cross, J. C. (2011). Cell-cell adhesion defects in Mrj mutant trophoblast cells are associated with failure to pattern the chorion during early placental development. Dev. Dyn. 240, 2505–2519. doi: 10.1002/dvdy.22755
Watson, E. D., Mattar, P., Schuurmans, C., and Cross, J. C. (2009). Neural stem cell self-renewal requires the Mrj co-chaperone. Dev. Dyn. 238, 2564–2574. doi: 10.1002/dvdy.22088
Whitley, D., Goldberg, S. P., and Jordan, W. D. (1999). Heat shock proteins: a review of the molecular chaperones. J. Vasc. Surg. 29, 748–751.
Yao, C., Biesinger, J., Wan, J., Weng, L., Xing, Y., Xie, X., et al. (2012). Transcriptome-wide analyses of CstF64-RNA interactions in global regulation of mRNA alternative polyadenylation. Proc. Natl. Acad. Sci. U.S.A. 109, 18773–18778. doi: 10.1073/pnas.1211101109
Keywords: heat shock protein, Hsp40, MRJ, virus, morpholino oligonucleotide
Citation: Ko S-H, Huang L-M and Tarn W-Y (2019) The Host Heat Shock Protein MRJ/DNAJB6 Modulates Virus Infection. Front. Microbiol. 10:2885. doi: 10.3389/fmicb.2019.02885
Received: 08 July 2019; Accepted: 29 November 2019;
Published: 11 December 2019.
Edited by:
Ian M. Jones, University of Reading, United KingdomReviewed by:
Arun Upadhyay, Northwestern University, United StatesCopyright © 2019 Ko, Huang and Tarn. This is an open-access article distributed under the terms of the Creative Commons Attribution License (CC BY). The use, distribution or reproduction in other forums is permitted, provided the original author(s) and the copyright owner(s) are credited and that the original publication in this journal is cited, in accordance with accepted academic practice. No use, distribution or reproduction is permitted which does not comply with these terms.
*Correspondence: Li-Min Huang, bG1odWFuZ0BudHUuZWR1LnR3; Woan-Yuh Tarn, d3Rhcm5AaWJtcy5zaW5pY2EuZWR1LnR3
Disclaimer: All claims expressed in this article are solely those of the authors and do not necessarily represent those of their affiliated organizations, or those of the publisher, the editors and the reviewers. Any product that may be evaluated in this article or claim that may be made by its manufacturer is not guaranteed or endorsed by the publisher.
Research integrity at Frontiers
Learn more about the work of our research integrity team to safeguard the quality of each article we publish.