- 1Département de Biologie, Centre SÈVE, Université de Sherbrooke, Sherbrooke, QC, Canada
- 2Department of Microbiology, Faculty of Science, Ain Shams University, Cairo, Egypt
The outer potato periderm layer consists of dead suberized cells. Suberin, a protective biopolymer, is made of a polyaliphatic portion covalently linked to polyaromatic moieties. Evidence accumulates that Streptomyces scabies, the main causal agent of potato common scab, can degrade the suberin aliphatic part but its ability to degrade the aromatic portion has not been documented. This polyaromatic portion is mainly composed of cinnamic acids. In this study, two cinnamates (trans-ferulic or p-coumaric acids) were added to the culture medium of S. scabies strains EF-35 and 87.22. HPLC quantification revealed that both strains efficiently utilized these compounds. A proteomic study coupled with gene expression analysis led to the identification of putative catabolic pathways for cinnamates. Catabolism of both compounds appeared to occur via the β-ketoadipate pathway. Gene SCAB_15301, encoding for a putative vanillate monooxygenase, was partly deleted from S. scabies strain 87.22 genome. The mutant retained its ability to catabolize trans-ferulic acid into vanillate but lost its ability to further degrade the latter compound. When the wild-type mutant and complemented strains were grown in the presence of suberin-enriched potato periderm, accumulation of vanillic acid was observed only in the mutant culture medium. This work presents evidence that S. scabies can degrade not only the aliphatic part of suberin but also the constituents of suberin aromatic portion. This may provide ecological and pathological advantages to S. scabies as a saprophyte and pathogen.
Introduction
Streptomyces scabies is the main causal agent of potato common scab, a disease which reduces both crop value and tuber marketability. This disease is characterized by dark colored lesions covering the tubers surface. The pathogenicity of S. scabies is mainly caused by the production of toxins known as thaxtomins, which induce cell death in plant cells and tissues (Goyer et al., 1998; Healy et al., 2000; Duval et al., 2005).
The potato skin contains high levels of suberin, a biopolymer playing a protective role against pathogen entry in potato tuber tissues. Suberin is a complex polymer consisting of two covalently linked portions (see model proposed by Bernards, 2002). The polyaliphatic portion located between the plasma membrane and the primary cell wall consists of esterified long-chain fatty acids. Evidence accumulates that S. scabies produces esterases degrading this aliphatic part (Beaulieu et al., 2016). A secretome analysis of S. scabies cultures grown in the presence of suberin was performed to identify the enzymes potentially involved in the degradation of suberin (Komeil et al., 2014). Glycosyl hydrolases were the most abundant proteins in the supernatant of suberin-containing medium, in addition to several enzymes involved in lipid metabolism (Komeil et al., 2014).
The polyaromatic portion of suberin is a lignin-like structure embedded in the primary cell wall. It is mainly composed of polyhydroxycinnamates (Bernards et al., 1995; Bernards and Lewis, 1998), where trans-ferulic and p-coumaric acids figure among the main hydroxycinnamates forming the aromatic moiety of suberin (Riley and Kolattukudy, 1975). Previous work has shown the importance of trans-ferulic acid in keeping the integrity of both suberin and periderm wax (Serra et al., 2010), therefore preventing the pathogen entry.
Suberin degradation, especially of the aromatic part, has been mostly investigated in fungi. As an example, Aspergillus nidulans was reported to degrade the aromatic part of suberin by monitoring the degradation products when A. nidulans was grown on suberized cell walls (Martins et al., 2014). The microbial degradation of suberin is a process that has been poorly characterized. However, there are several Streptomyces species that have been reported to degrade aromatic compounds. Streptomyces sp. strain ERI-CPDA-1, isolated from oil contaminated soil, was able to degrade petroleum and polycyclic aromatic hydrocarbons (PAHs). The degradation products detected were benzaldehyde, catechol, phenylacetic acid and protocatechuic acid (Balachandran et al., 2012). Streptomyces setonii strain ATCC 39116, a thermophilic soil actinomycete, was also shown to be able to degrade single aromatic compounds, including phenol and benzoate, through the ortho-cleavage pathway (Park and Kim, 2003).
A previous study showed that in the presence of suberin, S. scabies secreted the extracellular protein C9Z2P6, a putative 3-oxo-5,6-dehydrosuberyl-CoA semialdehyde dehydrogenase, which might be involved in the degradation of aromatic compounds. Moreover, the gene encoding this protein was overexpressed in presence of suberin (Komeil et al., 2014), suggesting that S. scabies has the ability to degrade the aromatic part of suberin.
In the present study, the ability of S. scabies to degrade the main aromatic constituents of the potato suberin, trans-ferulic acid and p-coumaric acid, is investigated. A hypothetical degradation pathway of trans-ferulic and p-coumaric acids in S. scabies 87.22 is proposed.
Materials and Methods
Culture Conditions
Strains and plasmids used in this study are listed in Table 1. Inocula of S. scabies strains were prepared by inoculating approximately 108 spores in 50 mL of yeast malt extract (4 g L–1 glucose, 4 g L–1 yeast extract, and 10 g L–1 malt extract). The bacteria were then incubated at 30°C with shaking (250 rpm) for 48 h followed by bacterial cells recovery using centrifugation (3,500 × g) for 10 min. The collected pellets were resuspended in 5 volumes of saline solution (NaCl 0.85%) and the resulting bacterial suspensions were used as inocula in further experiments. These bacterial inocula (100 μL) were added to 50 mL of the control medium (CM) composed of 0.5 g L–1 l-asparagine, 0.5 g L–1 K2HPO4, 0.2 g L–1 MgSO4⋅7H2O, 10 mg L–1 FeSO4⋅7H2O, and 0.05% (w/v) casein hydrolysate. This medium could be supplemented with trans-ferulic acid (60 μM), p-coumaric acid (50 μM), vanillic acid (60 μM), protocatechuate (60 μM), suberin 0.1% (w/v) or a combination of both ferulic and vanillic acids. Suberin was purified from potato tubers according to Kolattukudy and Agrawal (1974) with modifications proposed by Komeil et al. (2013). Culture media were adjusted to pH 7.0 and incubated with shaking (250 rpm) at 30°C. Escherichia coli strains were grown on LB agar plates at 37°C. When necessary, spectinomycin (100 μg mL–1 final concentration) was added to the culture medium.
Quantification of Cinnamic Acids in Streptomyces scabies Culture Broths
Amounts of trans-ferulic, p-coumaric and vanillic acids in culture broths were quantified by high performance liquid chromatography (HPLC). These cinnamic acids were detected using an Agilent 1260 Infinity high-performance liquid chromatograph equipped with a reverse-phase C18 column with fully porous particles of 3.5 μm size (Zorbax SB-C18, 4.6 × 150 mm, Agilent). Cinnamic acids were eluted using a 25 to 75% acetonitrile linear gradient for 10 min at a flow rate of 1 mL min–1 and monitored at 310 nm (trans-ferulic and p-coumaric acids) or 254 nm (vanillic acid). Standard curves were established for each compound analyzed using dilutions of known quantity of the compounds.
Proteomics Analysis
Streptomyces scabies strain 87.22 was grown in CM for 4 days. Six and 12 h later, trans-ferulic acid (60 μM) was then added or not to the culture medium and the culture was incubated for an additional 6 h. Bacterial cells were then collected by centrifugation at 3,500 × g for 10 min and were washed with phosphate buffered saline (PBS). PBS was removed by centrifugation and the intracellular proteins of collected cells were extracted by adding PBS and freezing the cells in ethanol kept at –80°C. The cells were thawed for 10 min at room temperature. This freeze-thaw lysis was repeated twice, and the suspension was sonicated for 10 s. The suspension was centrifuged at 4°C for 10 min (3,500 × g) and supernatant containing the proteins was kept at 4°C. Intracellular proteins were subjected to sodium dodecyl sulfate-polyacrylamide gel electrophoresis [10% (w/v) SDS-PAGE] and in-gel protein digestion were carried out according to Komeil et al. (2013). Mass spectrometry was conducted at the Proteomics Platform of the Quebec Genomics Center (Quebec City, Canada) using a hybrid quadrupole time-of-flight (QqTOF) (TripleTOF 5600 plus, SCIEX) coupled to a capillary HPLC for peptide separation via a nanospray ionization source. All MS/MS spectra were then interpreted using Mascot (Matrix Science, London, United Kingdom) to search S. scabies strain 87.22 Uniref100 database to provide statistically validated matches between observed spectra and identified peptides and list of proteins. The results were then uploaded to the scaffold software program (version Scaffold 4.8.8, Proteome Software, Portland, OR, United States) and a filter was set with a 95% minimum protein ID probability with a minimum number of two unique peptides, in which the cut-offs for peptide thresholds were set to 1.0% false discovery rate (FDR). Protein function was predicted using the UniProt, NCBI, KEGG, and COG databases. The normalized spectral count (NSpC) of proteins was obtained by dividing the number of spectra (SpC) for a protein by the molecular weight (MW) of the corresponding protein (Neilson et al., 2011). The experiment was done in duplicate.
qRT-PCR
The expression of the potential cinnamic acid catabolic genes was determined in the presence of ferulate or coumarate as follows. S. scabies strain 87.22 was grown in CM for 4 days and then trans-ferulic or p-coumaric acid was added or not to the culture medium. Three hours later, 10 mL of each culture medium was mixed with 2 mL of stop solution (ethanol/acidic phenol, 95:5, [v/v]) to prevent RNA degradation (Joshi et al., 2007). Bacterial cell pellets were recovered by centrifugation at 4°C for 10 min at 3,500 × g and stored at –80°C until further use. RNA was extracted from cells using the RNeasy Mini kit (Qiagen) (Lerat et al., 2010). Two μg of total isolated RNA were then reverse transcribed to cDNA using the Maxima First strand cDNA synthesis kit (ThermoFisher Scientific) according to the manufacturer’s instructions. Diluted cDNA (10×) was used to perform quantitative real-time reverse-transcription polymerase chain reaction (qRT-PCR) using Mx3000P qPCR system (Agilent Technologies) with the BrightGreen 2x qPCR MasterMix-Low ROX. The q-RT PCR conditions were 95°C for 10 min followed by 35 cycles at 95°C for 15 s and 60°C for 30 s. Relative expression levels were determined by using the comparative CT values according to Pfaffl (2001) with gyrA gene as a reference gene. Primers used in this assay are listed in Table 2.
Deletion of the Putative Vanillate Monooxygenase Gene SCAB_15301 From Streptomyces scabies 87.22 Genome
Streptomyces scabies strain 87.22 carrying a non-functional SCAB_15301 gene was constructed using the CRISPR-Cas9 system. The procedure of Wang et al. (2016), specifically developed for Streptomyces species, was followed with plasmid pCRISPomyces-2 (Addgene) to carry the construction.
Briefly, a short DNA sequence, unique to SCAB_15301 in the S. scabies genome (protospacer: CCGGTCGACCTCGCGTAGAA), was introduced into pCRISPomyces-2, generating plasmid pCRISPo-proto15301. A partially deleted version of gene SCAB_15301 (generating a stop codon) was then created, using the Gibson Assembly Master Mix (New England Biolabs). Pairs of primers used to create the homology arms required for the Gibson assembly were 5′-GTTGCCGCCGGGCGTTTTTTATCCTTCCTGACCTGCGAT AAC-3′ + 5′-GATCCGCCCCTCGATGGCGGGCACTCCGCGT CGTCCATGT-3′ and 5′- ACGCGGAGTGCCCGCCATCGAGG GGCGGATCACCCGCTG-3′ + 5′- GCCTTTTTACGGTTCCT GGCCTGTTCCAGGGCGAAGTTGTT -3′-). The resulting DNA construct was inserted in pCRISPo-proto15301 and the obtained plasmid was named pCRISPo-15301-full. This region serves as repair template to the recombination process that follows the disruption of target DNA by the CRISPR-Cas9 system.
pCRISPo-15301-full was introduced in the E. coli conjugation strain ET12567-pUZ8002. Conjugation between S. scabies 87.22 and E. coli ET12567- pUZ8002 carrying plasmid pCRISPo-15301-full was performed as described in Kieser et al. (2000). The insertion of non-functional SCAB_15301 gene in the genome of exconjugants was confirmed by DNA sequencing using primers annealing slightly upstream and downstream of the editing template sequence (5′-CTTCTGCGAGGAAACCAACA-3′ and 5′-CAGCATCGGCAGGATGG-3′). Finally, the plasmid carrying the pCRISPomyces system was cleared from the S. scabies recombinant strain after three serial cultures in ISP-2 medium (Wang et al., 2016).
Complementation of ΔSCAB_15301 Deletion Mutant
Genomic DNA was obtained from S. scabies strain 87.22 using the salting-out procedure (Kieser et al., 2000). SCAB_15301 along with the upstream region (1799 bp) were amplified from the wild type strain using two primers to which the restriction sequences of the digestion enzymes were added (5′-CGGAATTCCGTCTCCTCCTCGGTCCTCACCC-3′ and 5′-GCTCTAGAGCTCACAGGACCTTCTCCACCGG-3′). PCR reactions were carried out in a final volume of 50 μL containing 1 μL (233 ng μL–1) of template DNA, 2.5 μL (10 μM) of each primer, 10 μL of 10 × buffer, 1 μL of 10 mM dNTPs, 0.5 μL of Q5® high-fidelity DNA polymerase, 10 μL of Q5 high GC enhancer. The PCR cycling conditions were as follows: an initial pre-denaturing step at 98°C for 30 s, 35 cycles at 98°C for 10 s, 72°C for 1 min and a final extension step at 72°C for 2 min using Bio-Rad T100 thermal Cycler.
Amplicons of the putative vanillate monooxygenase gene SCAB_15301 and integrative vector pSET152m (Lu et al., 2007) were both digested with EcoRI and XbaI at 37°C for 2 h. SCAB_15301 gene was ligated into the digested plasmid using DNA ligase at 16°C for 2 h. The resulting plasmid pSET15301 was transformed to E. coli ET12567-pUZ8002. The transformation was done as described by Kieser et al. (2000). The conjugation between E. coli ET12567-pUZ8002-pSET15301 and S. scabies ΔSCAB_15301 was carried out as proposed by Kieser et al. (2000). The complementation was confirmed by sequencing the inserted gene SCAB_15301 using the same primers used for SCAB_15301 amplification. Sequencing was carried out at Genome Sequencing and Genotyping Platform (Quebec City, Canada) using Sanger technique.
Results
Utilization of Cinnamic Acids by Streptomyces scabies
A time course study of both trans-ferulic and p-coumaric acids depletion was carried out by sampling the S. scabies culture at 3-h intervals for 15 h. Both S. scabies strains EF-35 and 87.22 gradually utilized these compounds. After 15 h of incubation, most of trans-ferulic and p-coumaric acids were consumed (Figures 1A,B). During the first hours of incubation, both tested strains showed higher degradation affinity toward trans-ferulic acid when compared to p-coumaric acid, and especially S. scabies 87.22 which consumed about 84% of the added trans-ferulic acid after 6 h of inoculation (Figure 1B).
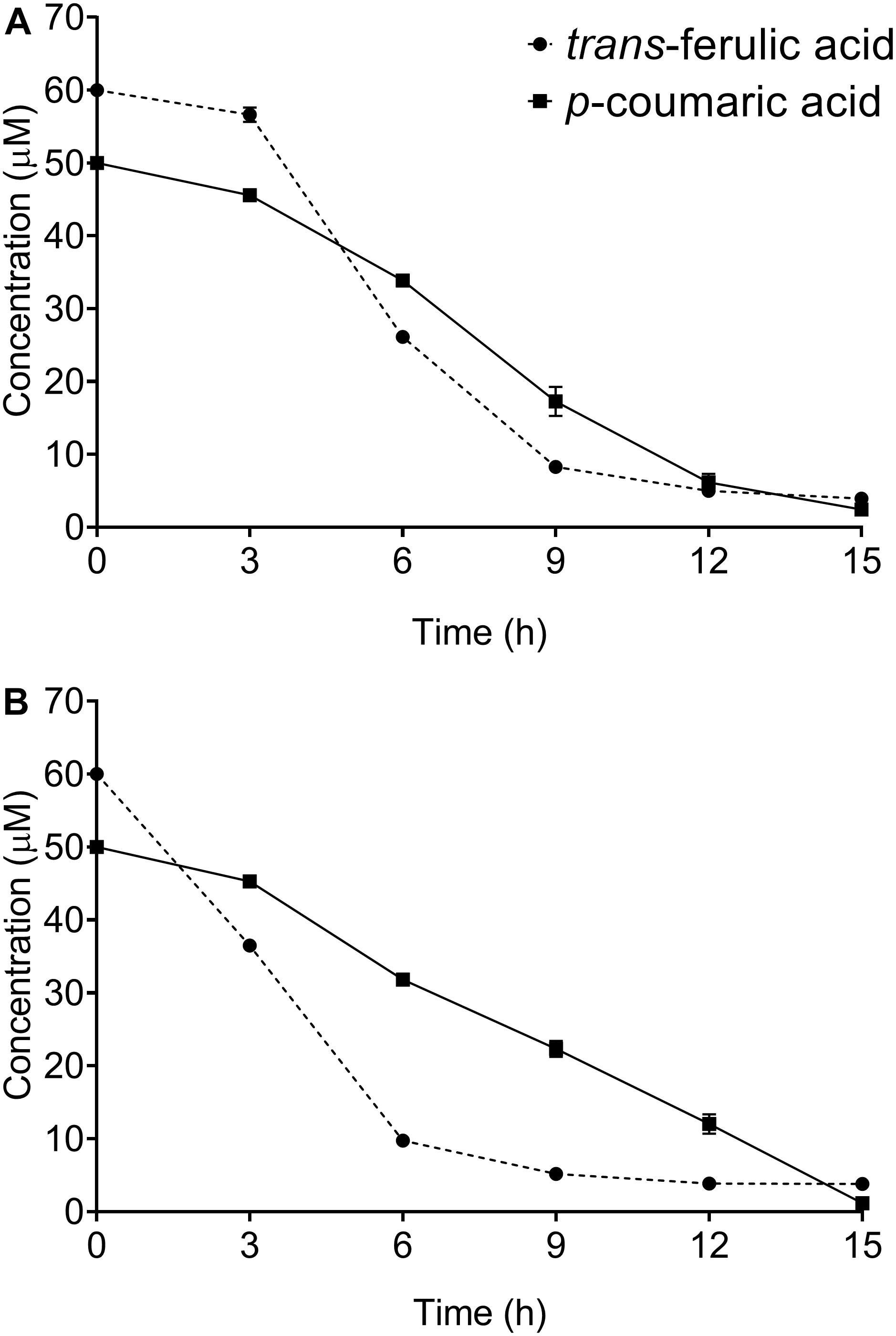
Figure 1. Kinetics of trans-ferulic and p-coumaric acids utilization in Streptomyces scabies strains EF-35 (A) and 87.22 (B). Data shown are the mean of three replicates (±SD).
SCAB_15301 has been identified in the S. scabies 87.22 genome [using basic local alignment search tool (BLAST)] as a gene encoding a vanillate monooxygenase (Supplementary Figure S1), which is responsible for bioconversion of trans-ferulic acid into protocatechuate. To confirm the predicted function of this gene, SCAB_15301 was deleted from S. scabies 87.22 genome using CRISPR/Cas9-based method. The CRISPR/Cas9-based method has been shown to be effective in Streptomyces genome editing (Wang et al., 2016). However, the success rate in S. scabies using the CRISPR/Cas9-based system was very low as only one exconjugant was obtained and all efforts to obtain other mutants failed. Nonetheless, depletion of ferulic acid by the deletion mutant S. scabies ΔSCAB_15301 was observed. This mutant was able to convert over 80% of the initial amount of ferulic acid into vanillate within 9 h (Figure 2C). This contrasted with both the wild and complemented strains, where vanillate did not accumulate into the culture medium (Figures 2A,B). Moreover, when vanillic acid was added to the culture medium of strains 87.22, ΔSCAB_15301 and S. scabies com15301, catabolism of vanillate was observed in strain 87.22 and the complemented strain but not in ΔSCAB_15301 (Figure 3), indicating that SCAB_15301 effectively codes for a putative vanillate monooxygenase.
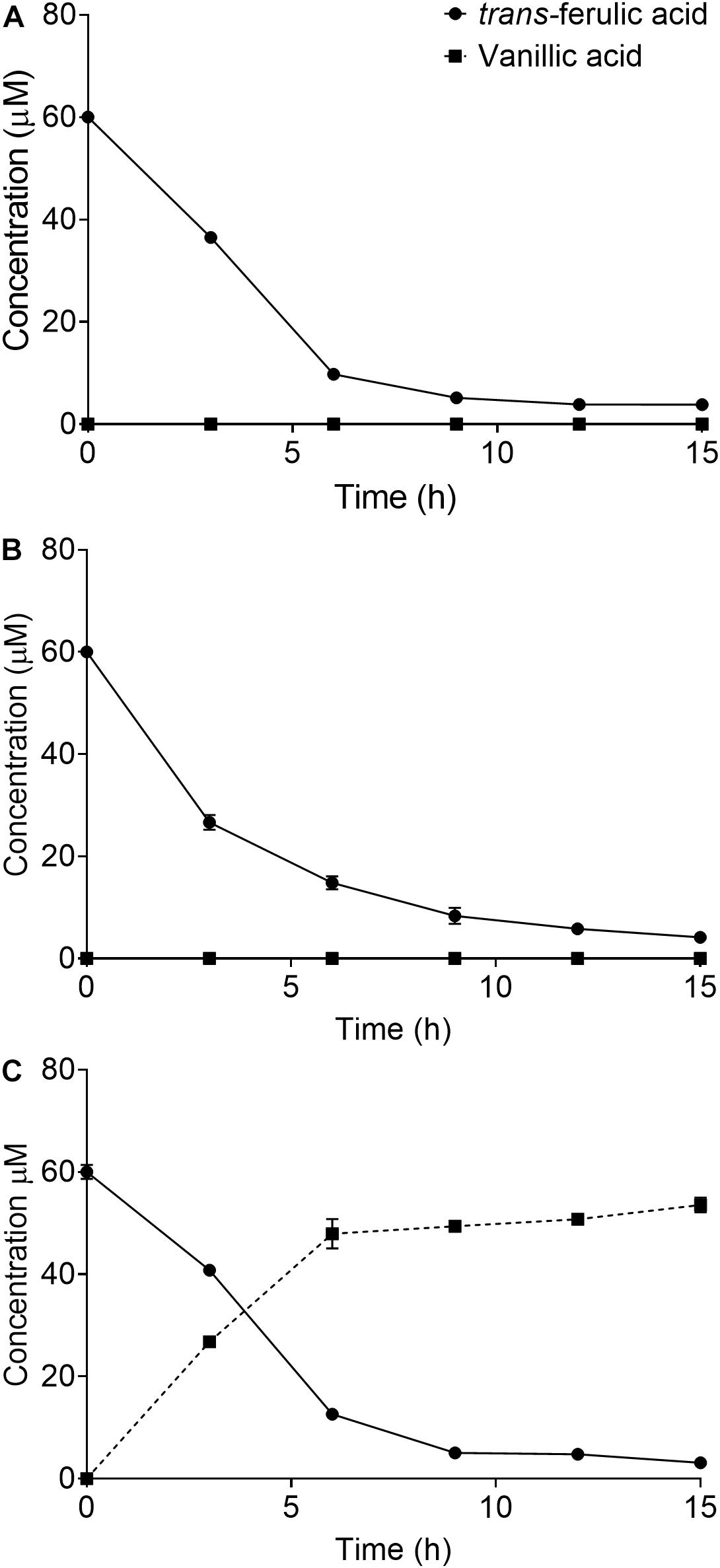
Figure 2. Utilization of ferulic acid by S. scabies: (A) S. scabies 87.22 utilized trans-ferulic acid but did not accumulate vanillate. (B) S. scabies com15301 utilized trans-ferulic acid without vanillate accumulation. (C) ΔSCAB_15301 converted over 80% of the initial amount of trans-ferulic acid into vanillate within 9 h. Data shown are the mean of three replicates (±SD).
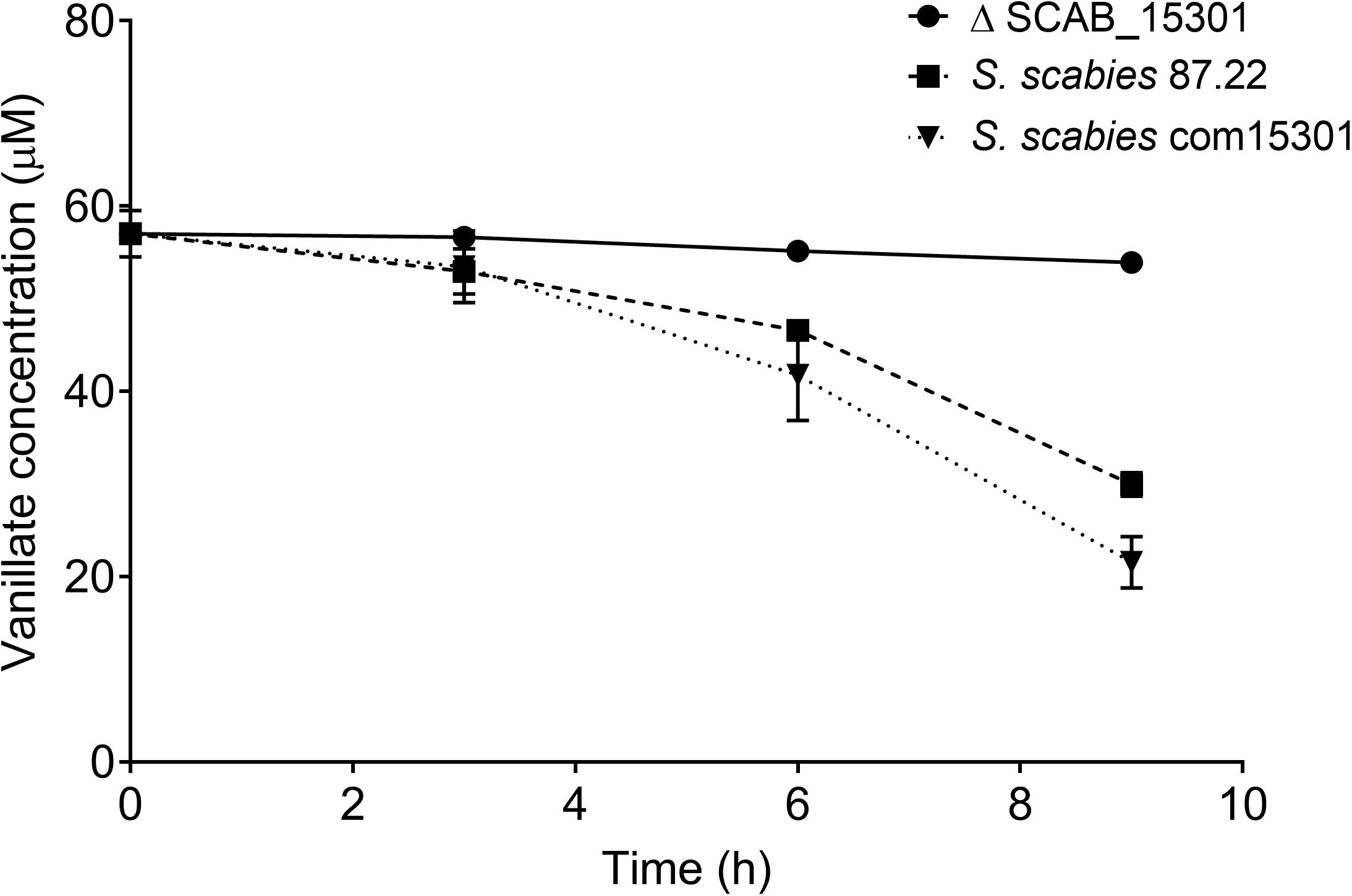
Figure 3. Catabolism of vanillate in S. scabies strains 87.22, ΔSCAB_15301 and com15301. Data shown are the mean of three replicates (±SD).
Effect of Trans-Ferulic Acid on Streptomyces scabies 87.22 Proteome
Intracellular proteins produced by S. scabies 87.22 during growth in CM supplemented or not with trans-ferulic acid are listed in Supplementary Table S1. Sixty-nine proteins were found exclusively in the presence of trans-ferulic acid. These proteins were categorized according to their functional groups (Table 3). Among them, seven proteins were identified to be potentially involved in the degradation of aromatic compounds (Table 3) and five were included in the stress mechanism functional group. Only two proteins were detected exclusively in CM without trans-ferulic acid; a transmembrane efflux protein (C9Z0L4) with NSpC of 0.03 and a membrane protein with unknown function with NSpC of 0.04.
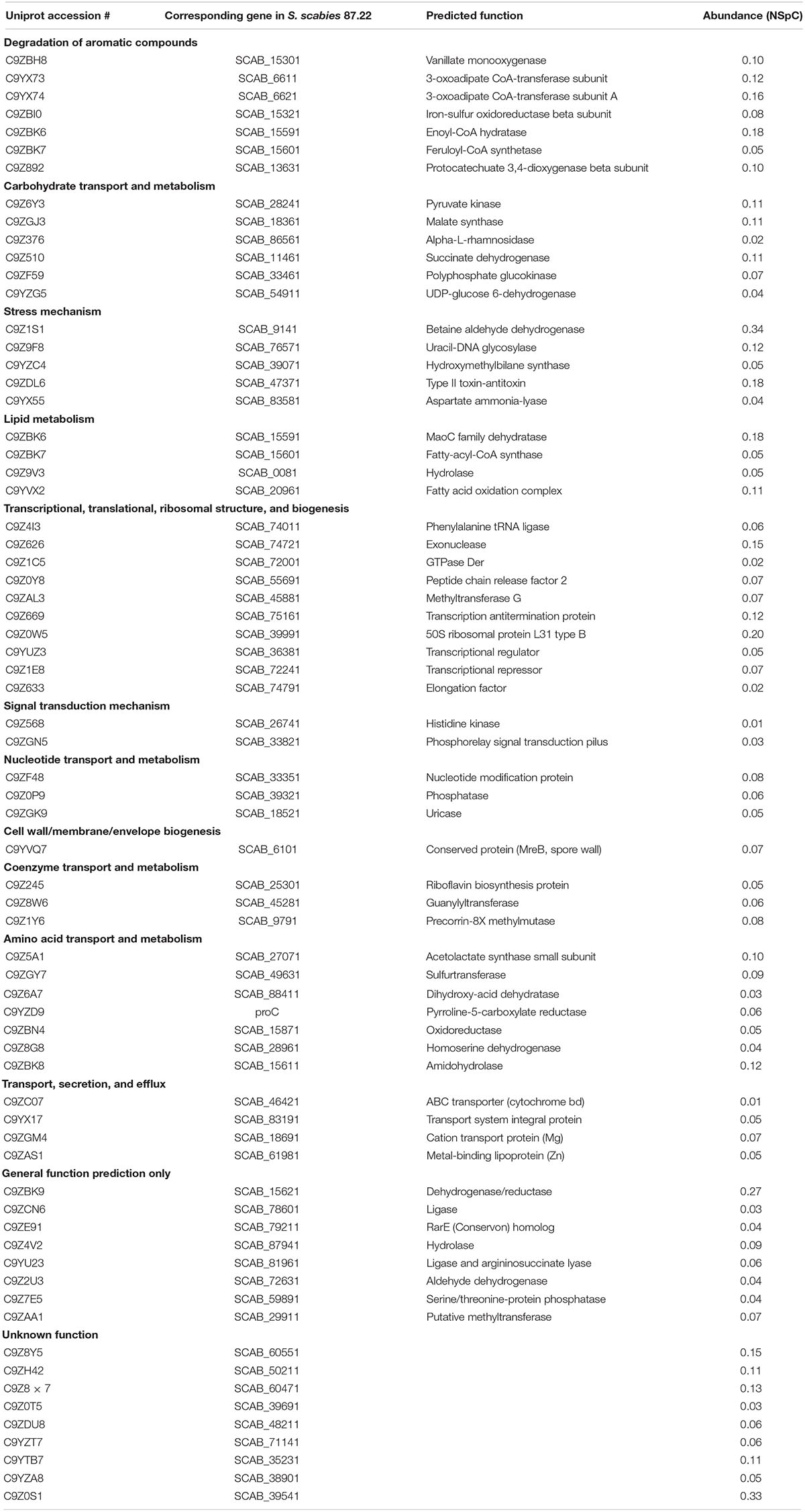
Table 3. Proteins detected only in Streptomyces scabies 87.22 proteome in control medium supplemented with ferulic acid.
Effects of Hydroxycinnamates on the Expression of the Putative Aromatic Compounds Degradation Genes
The expression of seven genes associated with the degradation of aromatic compounds functional group (Table 3) and of three additional genes that were identified using basic local alignment search tool (BLAST) from S. scabies 87.22 genome was investigated. The three additional genes were SCAB_15331 (a putative IclR-family transcriptional regulator gene located in the vicinity of SCAB_15301 and SCAB_15321), SCAB_2141 (2,3-dihydroxy-2,3-dihydro-phenylpropionate dehydrogenase encoding gene) and SCAB_13661 (3-oxoadipate enol-lactone hydrolase-encoding gene). All genes tested were overexpressed in the presence of at least one of the two cinnamic acids (trans-ferulic acid or p-coumaric acid) (Figure 4).
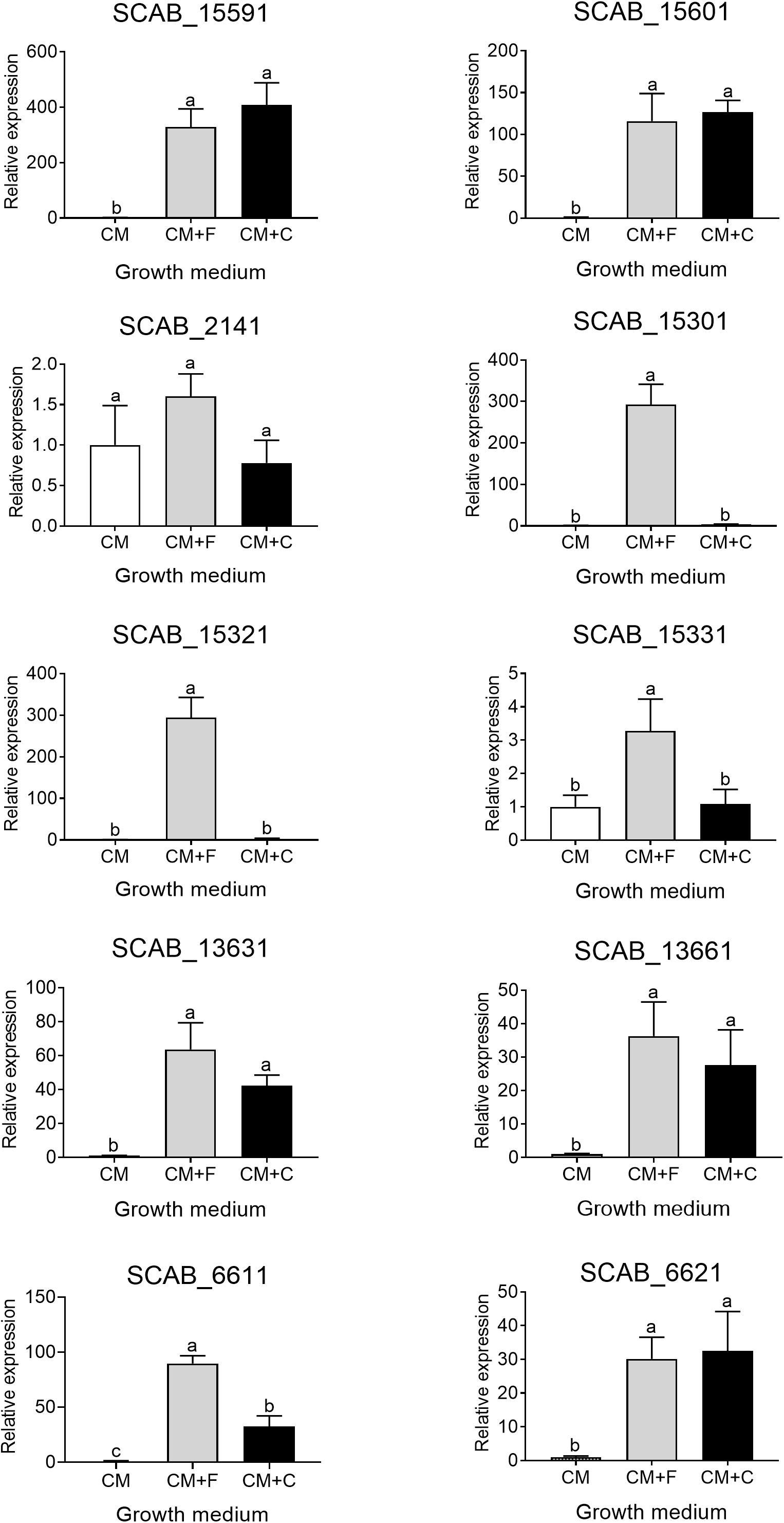
Figure 4. Relative expression levels (+SD) of targeted genes involved in trans-ferulic and p-coumaric acids degradation from S. scabies 87.22 grown in control medium (CM) alone, CM supplemented with trans-ferulic acid (CM+F, gray bars) and CM supplemented with p-coumaric acid (CM+C, black bars). Data were normalized with the gyrA gene which was used as an internal control. Data shown are the mean of three replicates. Data with the same letter are not significantly different (P < 0.05, LSD test).
SCAB_15601 and SCAB_15591 code for a putative feruloyl-CoA synthetase and a putative enoyl-CoA hydratase, respectively. They are potentially responsible for the transformation of ferulic acid into vanillic acid. Their gene expression was increased over 100- and 300-fold in the presence of ferulic and coumaric acids, respectively (Figure 4). The expression of the three genes identified as potential candidates for the conversion of vanillate into protocatechuate showed between 15-fold (SCAB_15331) and 300-fold overexpression (SCAB_15301 and SCAB_15321) in the presence of trans-ferulic acid (Figure 4). In contrast, the relative expression of these three genes was not statistically different when the bacteria were grown in the control medium supplemented or not with p-coumaric acid.
SCAB_2141 expression was found to be induced by p-coumaric acid but not by trans-ferulic acid. SCAB_2141 showed high expression in the presence of p-coumarate (ca. 20-fold upregulated). Whereas, no difference was recorded when trans-ferulic acid was added to the control medium (Figure 4).
The four genes involved in the main β-ketoadipate pathway that were tested showed very similar expression patterns toward both substrates. SCAB_13631 showed a relative expression increasing from 40- to 60-fold in the presence of p-coumaric and trans-ferulic acid, respectively. SCAB_13661, a gene predicted to be responsible for conversion of γ-carboxymuconolactone into β-ketoadipate enol-lactone, showed a 36-fold overexpression when the control medium was supplemented with trans-ferulic acid and a 27-fold overexpression with p-coumarate. SCAB_6611 showed higher expression in the presence of trans-ferulic acid when compared to the presence of p-coumaric acid. SCAB_6621, which is predicted to further metabolize β-ketoadipate into β-ketoadipyl-CoA, showed around 30-fold overexpression with both substrates when compared to the control medium. A hypothetical degradation pathway of trans-ferulic and p-coumaric acids is proposed (Figure 5).
To determine if trans-ferulic or p-coumaric acid or one of their degradation products were the inducers of the β-ketoadipate pathway, the expression of SCAB_13631 (the first gene in the proposed β-ketoadipate pathway) was tested in presence of trans-ferulic, p-coumaric or protocatechuic acids. The gene SCAB_13631 showed around 60-fold overexpression in the presence of trans-ferulic acid in the wild strain. Addition of trans-ferulic acid in the culture medium of the deletion mutant strain ΔSCAB_15301 did not significantly induce transcription of gene SCAB_13631 (relative expression of 1.1 ± 0.31). SCAB_13631 showed similar expression rate in the presence of coumarate (42.36 ± 4.18 and 44.3 ± 3.9, respectively) or protocatechuate (4.05 ± 0.38 and 4.23 ± 0.97, respectively) in the wild strain and the deletion mutant.
Effects of Suberin on the Expression of the Putative Aromatic Compounds Degradation Genes
The expression of the genes predicted to be involved in the degradation of aromatic compounds (see above) was tested in the presence of suberin-enriched potato periderm. All tested genes, except gene SCAB_6621, showed a higher level of expression when S. scabies 87.22 was grown in the presence of suberin (between 2- and 30-fold overexpression) (Figure 6).
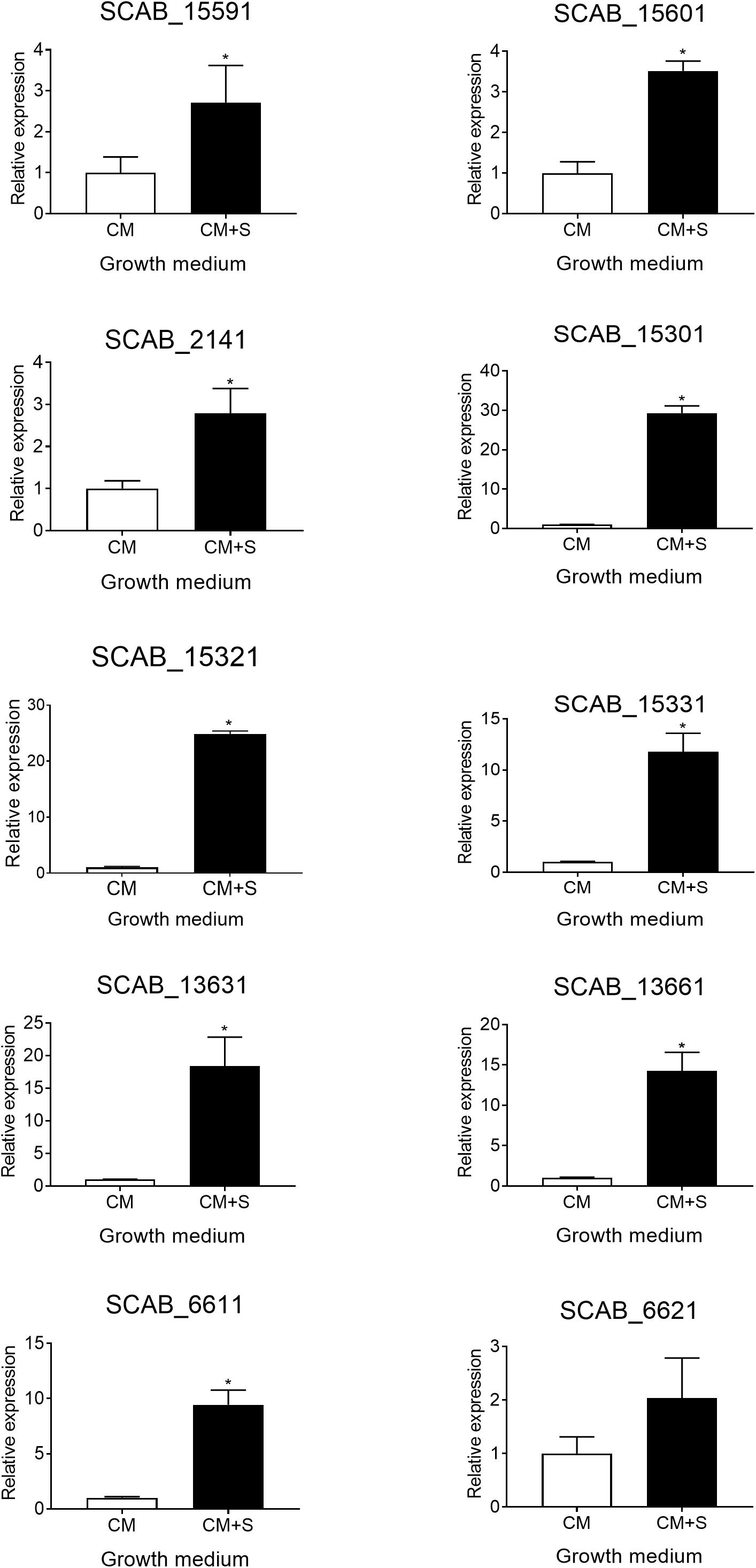
Figure 6. Relative expression levels (+SD) of targeted genes involved in trans-ferulic and p-coumaric acids degradation from S. scabies 87.22 grown in control medium (CM) alone and control medium (CM) supplemented with suberin (CM+S, black bars). Data were normalized with the gyrA gene which was used as an internal control. Data shown are the mean of three replicates. Data with ∗ are significantly different from the control (P < 0.05, t-test).
Vanillate Accumulation in the Mutant Culture Media
When the wild strain S. scabies 87.22, deletion mutant ΔSCAB_15301 and complemented strain S. scabies com15301 were grown in the presence of suberin-enriched potato periderm, production of vanillic acid was observed only in the mutant culture medium (Figure 7A). The accumulation of vanillic acid from the suberin-enriched potato periderm rapidly reached a plateau (Figure 7A). Accumulation of vanillate may interfere with ferulic acid degradation as suggested by the fact that strain ΔSCAB_15301 was less efficiently catabolizing ferulic acid when grown in the presence of both trans-ferulic and vanillic acids (Figure 7C) than with ferulic acid alone (Figure 7B).
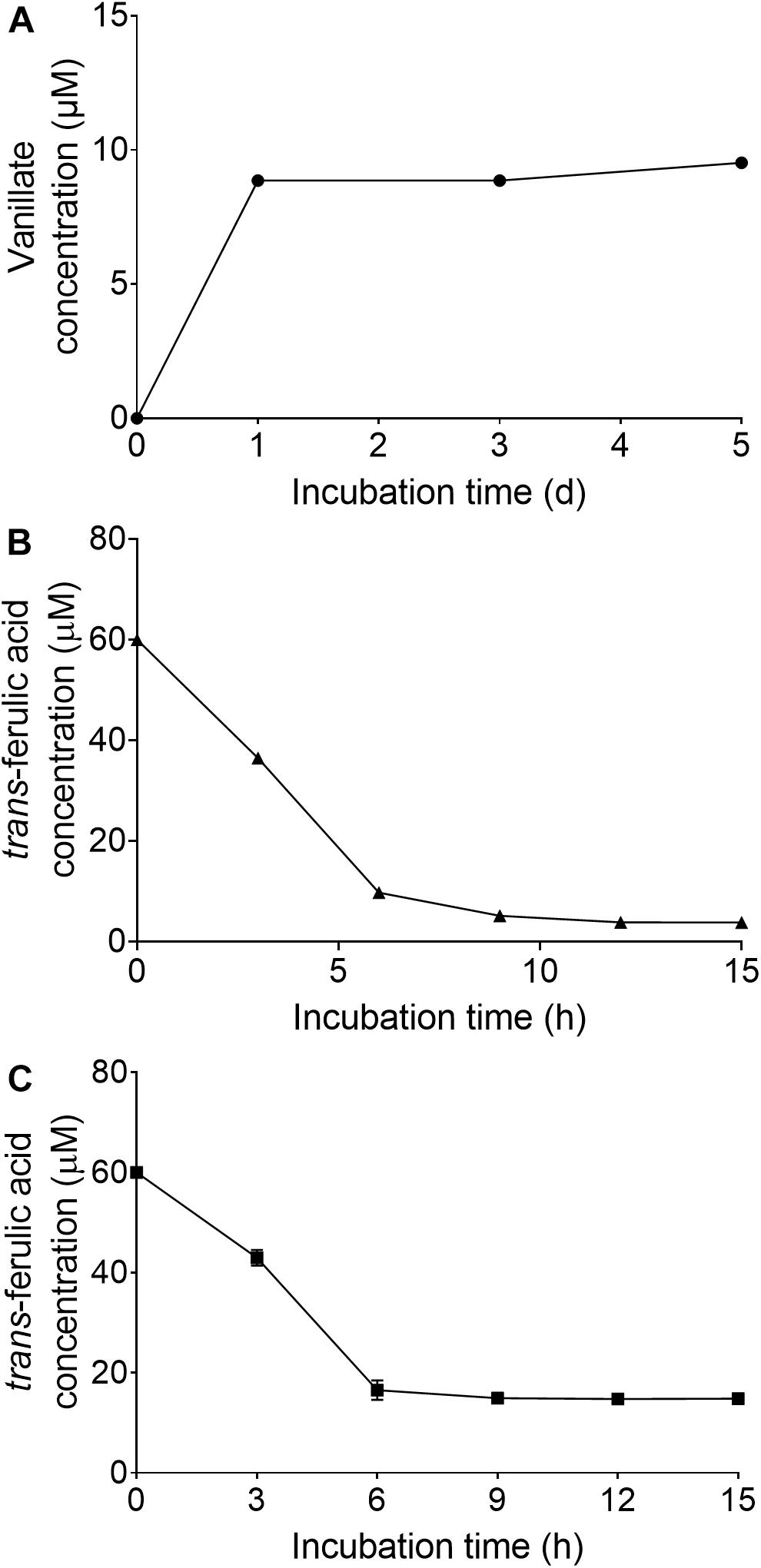
Figure 7. (A) Vanillate accumulation from suberin-enriched potato periderm in ΔSCAB_15301 (no accumulation was detected with the wild-type or the complemented strain). (B) Utilization of trans-ferulic acid in the ΔSCAB_15301 when grown in the presence of trans-ferulic acid. (C) Utilization of trans-ferulic acid in the ΔSCAB_15301 when grown in the presence of both trans-ferulic acid and vanillate. Data shown are the mean of three replicates (±SD).
Discussion
Beaulieu et al. (2016) previously demonstrated the ability of S. scabies to degrade the aliphatic moiety of suberin, but there has been no report about the degradation of the aromatic part of suberin by this pathogen. Nevertheless, a previous proteomic analysis revealed the presence of proteins predicted to play a role in the degradation of aromatic compounds when S. scabies was grown in the presence of suberin (Komeil et al., 2014). This study aimed to demonstrate the ability of S. scabies strains to utilize trans-ferulic and p-coumaric acids which are the main constituents of the suberin aromatic moiety.
In this study, S. scabies strains EF-35 and 87.22 were grown in a control medium supplemented with trans-ferulic and p-coumaric acids. Results revealed the ability of the two strains to efficiently degrade both substrates. The ability to degrade these cinnamic acids is shared by other streptomycetes such as S. setonii (Sutherland et al., 1983), Streptomyces sp. strain V-1 (Yang et al., 2013), Streptomyces sannanensis (Ghosh et al., 2007), Streptomyces canus GLY-P2 (Wu et al., 2019), Streptomyces coelicolor and Streptomyces viridosporus (Davis and Sello, 2010).
Sutherland et al. (1983) reported the ability of S. setonii to catabolize ferulic acid into vanillin, vanillic acid, and protocatechuic acid. The proteomic study of S. scabies 87.22 in the presence of ferulic acid allowed the identification of proteins that could also be involved in the conversion of ferulic acid into vanillate (a putative feruloyl-CoA hydratase and feruloyl-CoA synthetase) and further degradation of vanillate into protocatechuate (a putative vanillate monooxygenase, iron-sulfur oxidoreductase beta subunit and IclR-family transcriptional regulator). In contrast, S. sannanensis was shown to bioconvert ferulic acid into vanillate without further degradation into protocatechuic acid, possibly because of the absence of vanillic acid demethylase and vanillic acid decarboxylase activities in this bacterium (Ghosh et al., 2007).
SCAB_15591 and SCAB_15601 genes, encoding putative feruloyl-CoA hydratase and feruloyl-CoA synthetase, respectively, which convert trans-ferulic acid into vanillate, were highly expressed in the presence of both ferulic and coumaric acids. This could be attributed to their broad substrate specificity. Masai et al. (2002) identified those two genes in Sphingomonas paucimobilis SYK-6 and showed that these enzymes exhibited broad substrate specificity as they were able to degrade p-coumaric acid, caffeic acid, and sinapinic acid. On the other hand, three genes were identified in S. scabies to be potentially involved in the catabolism of vanillate (SCAB_15301, SCAB_15321, and SCAB_15331). The fact that those three genes were induced by ferulate suggests that they are involved in the conversion of vanillate into protocatechuic acid. These genes were found to be induced in the presence of ferulic acid but not coumaric acid, suggesting their substrate specificity.
The predicted function of SCAB_15301 (vanillate monooxygenase) was confirmed by the fact that the mutant degraded trans-ferulic and accumulated vanillate as a degradation product. By contrast, in the wild-type strain, no accumulation of vanillic acid was detected when grown in the presence of trans-ferulic acid. Absence of ferulic acid degradation product suggests further metabolism of ferulate in S. scabies.
Previous work showed that when p-coumaric acid was used as an initial growth substrate for S. setonii, it was catabolized into p-hydroxybenzaldehyde, p-hydroxybenzoic acid and protocatechuic acid (Sutherland et al., 1983). SCAB_2141 gene showed sequence homology with 2,3-dihydroxy-2,3-dihydro-phenylpropionate dehydrogenase, suggesting that this gene could be responsible for the conversion of p-coumaric acid into p-hydroxybenzoic acid. The fact that this gene was induced by coumaric acid but not by ferulic acid also supports this metabolic function.
Some proteins found in the proteome of S. scabies 87.22 cultured in the presence of ferulic acid were predicted to be involved in the catabolism of protocatechuate through the β-ketoadipate pathway (protocatechuate 3,4-dioxygenase beta subunit, 3-oxoadipate CoA-transferase subunit and 3-oxoadipate CoA-transferase subunit A). The β-ketoadipate pathway is a chromosomally encoded pathway that is widely distributed in soil bacteria and fungi (Harwood and Parales, 1996). This highly conserved pathway has two branches; one of them converts protocatechuate, derived from phenolic compounds such as ferulic and coumaric acids, to β- ketoadipate. The gene cluster for protocatechuic acid catabolism was characterized in Streptomyces sp. strain 2065 (Iwagami et al., 2000). In this strain, a protocatechuate 3,4-dioxygenase was purified and seven genes were identified to be involved in the degradation of protocatechuate via the β-ketoadipate pathway (Iwagami et al., 2000).
In several studies, it was reported that the expression of β-ketoadipate pathway genes was induced by aromatic compounds or by β-ketoadipate (Harwood and Parales, 1996). The high expression of genes involved in the β-ketoadipate pathway in the presence of both trans-ferulic and p-coumaric acids in S. scabies supports the hypothesis that these two phenolic compounds share a common degradation pathway (β-ketoadipate pathway). In ΔSCAB_15301, the gene SCAB_13631, which encodes the first key enzyme in the β-ketoadipate pathway, was not induced in the presence of ferulic acid, suggesting that neither ferulic nor vanillic acid was the real inducer of the β-ketoadipate pathway. However, SCAB_13631 was induced in the presence of protocatechuate in both the mutant and the wild-type strains, suggesting that protocatechuate or its degradation products are the real inducers of the pathway. These results are in accordance with Davis and Sello (2010) who showed that both S. coelicolor and S. viridosporus contained the pca structural genes. These pca genes encode the enzymes of the protocatechuate branch of the β-ketoadipate pathway and were shown to be induced by protocatechuate and by p-hydroxybenzoate.
Most of the genes identified as being involved in degradation of aromatic compounds showed high expression in the presence of potato suberin, which could be attributed to the hydroxycinnamates content of suberin (Bernards et al., 1995; Bernards and Lewis, 1998). However, their expression in the presence of suberin was much lower than in the presence of ferulic and coumaric acids. This could be due to the fact that suberin degradation is a slow process (Hamer et al., 2012; Beaulieu et al., 2016). Vanillic acid accumulated when ΔSCAB_15301 was grown in the presence of suberin, suggesting that S. scabies 87.22 can retrieve ferulic acid from potato periderm. Similarly, previous work revealed the ability of another actinobacterium, S. cinnamoneus, to release ferulic acid from biomass (Uraji et al., 2018).
Phenolic compounds were shown to induce stress in bacteria. p-coumaric acid can disturb protein structure, affect the properties of the cell membrane and interfere with DNA replication of Lactobacillus casei BL23 (Rivas-Sendra et al., 2011). The proteome analysis of S. scabies 87.22 in the presence of ferulic acid revealed the production of stress proteins, especially protein involved in acid stress tolerance. Among these proteins is a uracil-DNA glycosylase, which has been reported to be involved in DNA repair, mutation prevention and tolerance to acidified nitrite in G+C-rich bacteria (Venkatesh et al., 2003). An aspartate ammonia-lyase (AspA) was also detected. This protein was shown to increase the acid survival in Yersinia pseudotuberculosis by producing ammonia (Hu et al., 2010). The α-acetolactate synthase (ALS) may contribute to S. scabies pH homeostasis following the addition of ferulic acid as an α-acetolactate synthase played this function in Lactococcus lactis under acid stress conditions (Zuljan et al., 2014). The most abundant protein found in the presence of ferulic acid only is a putative betaine aldehyde dehydrogenase. Since betaine is known to be an osmolyte produced in response to stress, especially to osmotic stress (Park et al., 1995), the induction of betaine aldehyde dehydrogenase in the presence of ferulic acid may represent a response to general stress. However, streptomycetes have been shown to produce several NADPH/NADH-producing enzymes in presence of recalcitrant compounds that could help to offset the low level of energy obtained from their catabolism (Sineli et al., 2018). These findings reveal that S. scabies has undergone various adaptive physiological mechanisms in response to ferulic acid.
Streptomyces scabies also produced proteins involved in lipid metabolism after the addition of ferulic acid to its growth medium. In potato periderm, ferulate esters are important structural elements but represent only a small fraction of suberin constituents which is mainly composed of fatty acids (Beaulieu et al., 2016). Therefore, ferulic acid might act as a signal to produce enzymes such as those of the fatty acids oxidation complex. This protein complex which breaks down CoA activated derivatives of fatty acids into acetyl-CoA by the β-oxidation pathway (Menendez-Bravo et al., 2017) might be important for the degradation of the aliphatic constituents of suberin. Interestingly, the second most abundant protein induced by the addition of ferulic acid, SCAB_3954, is encoded by a gene of unknown function that is located in a gene cluster dedicated to Coenzyme A biosynthesis. Such relationship between fatty acid metabolism and catabolism of aromatic compounds has also been suggested in another actinobacterium, Rhodococcus sp. strain BUBNP1. A transcriptomics study revealed that strain BUBNP1 degrades concurrently 4-nitrophenol and fatty acids (Sengupta et al., 2019).
Our study indicates that S. scabies can utilize hydroxycinnamates, contained in suberin, as carbon and energy sources via the β-ketoadipate pathway. This may be advantageous in environments where carbon sources are limited. In the last step of the β-ketoadipate pathway, once β-ketoadipyl-CoA is formed, it is converted to succinyl-CoA and acetyl-CoA. The latter two compounds are further metabolized via Krebs cycle, yielding energy. This could offer not only an ecological advantage to S. scabies as a saprophyte but also as a pathogen. Further research is required to elucidate the importance of degradation of aromatic compounds via β-ketoadipate in S. scabies environment, as it was reported before that the catabolism of aromatic compounds through β-ketoadipate pathway is necessary for the pathogenicity of other plant pathogens such as Fusarium oxysporum (Michielse et al., 2012).
Data Availability Statement
All datasets generated for this study are included in the article/Supplementary Material.
Author Contributions
CB and NB conceived and designed the experiments. MK and SL performed the lab work and analyzed the data. MK and CB wrote the manuscript with the critical review of NB and SL. CB supervised the project. All authors approved the final version of the manuscript.
Funding
This study was funded by the Natural Sciences and Engineering Research Council of Canada to CB (grant number 018602). MK was supported by a Ph.D. scholarship from the Ministry of Higher Education, Egypt and Centre SÈVE, QC, Canada.
Conflict of Interest
The authors declare that the research was conducted in the absence of any commercial or financial relationships that could be construed as a potential conflict of interest.
Acknowledgments
The authors thank Chantal Binda for critical review of the manuscript.
Supplementary Material
The Supplementary Material for this article can be found online at: https://www.frontiersin.org/articles/10.3389/fmicb.2019.02795/full#supplementary-material
References
Balachandran, C., Duraipandiyan, V., Balakrishna, K., and Ignacimuthu, S. (2012). Petroleum and polycyclic aromatic hydrocarbons (PAHs) degradation and naphthalene metabolism in Streptomyces sp. (ERI-CPDA-1) isolated from oil contaminated soil. Bioresour. Technol. 112, 83–90. doi: 10.1016/j.biortech.2012.02.059
Beaulieu, C., Sidibé, A., Jabloune, R., Simao-Beaunoir, A.-M., Lerat, S., Monga, E., et al. (2016). Physical, chemical and proteomic evidence of potato suberin degradation by the plant pathogenic bacterium Streptomyces scabiei. Microbes Environ. 31, 427–434. doi: 10.1264/jsme2.ME16110
Bernards, M. A., and Lewis, N. G. (1998). The macromolecular aromatic domain in suberized tissue: a changing paradigm. Phytochemistry 47, 915–933. doi: 10.1016/s0031-9422(98)80052-6
Bernards, M. A., Lopez, M. L., Zajicek, J., and Lewis, N. G. (1995). Hydroxycinnamic acid-derived polymers constitute the polyaromatic domain of suberin. J. Biol. Chem. 270, 7382–7386. doi: 10.1074/jbc.270.13.7382
Cobb, R. E., Wang, Y., and Zhao, H. (2015). High-efficiency multiplex genome editing of Streptomyces species using an engineered CRISPR/Cas System. ACS Synth. Biol. 4, 723–728. doi: 10.1021/sb500351f
Davis, J., and Sello, J. (2010). Regulation of genes in Streptomyces bacteria required for catabolism of lignin-derived aromatic compounds. Appl. Microb. Biotechnol. 86, 921–929. doi: 10.1007/s00253-009-2358-0
Duval, I., Brochu, V., Simard, M., Beaulieu, C., and Beaudoin, N. (2005). Thaxtomin A induces programmed cell death in Arabidopsis thaliana suspension-cultured cells. Planta 222, 820–831. doi: 10.1007/s00425-005-0016-z
Faucher, E., Savard, T., and Beaulieu, C. (1992). Characterization of actinomycetes isolated from common scab lesions on potato tubers. Can. J. Plant Pathol. 14, 197–202. doi: 10.1080/14786419.2018.1511554
Ghosh, S., Sachan, A., Sen, S. K., and Mitra, A. (2007). Microbial transformation of ferulic acid to vanillic acid by Streptomyces sannanensis MTCC 6637. J. Ind. Microbiol. Biotechnol. 34, 131–138. doi: 10.1007/s10295-006-0177-1
Goyer, C., Vachon, J., and Beaulieu, C. (1998). Pathogenicity of Streptomyces scabies mutants altered in thaxtomin A production. Phytopathology 88, 442–445. doi: 10.1094/PHYTO.1998.88.5.442
Hamer, U., Rumpel, C., and Dignac, M.-F. (2012). Cutin and suberin biomarkers as tracers for the turnover of shoot and root derived organic matter along a chronosequence of Ecuadorian pasture soils. Eur. J. Soil Sci. 63, 808–819. doi: 10.1111/j.1365-2389.2012.01476.x
Harwood, C. S., and Parales, R. E. (1996). The beta-ketoadipate pathway and the biology of self-identity. Annu. Rev. Microbiol. 50, 553–590. doi: 10.1146/annurev.micro.50.1.553
Healy, F. G., Wach, M., Krasnoff, S. B., Gibson, D. M., and Loria, R. (2000). The txtAB genes of the plant pathogen Streptomyces acidiscabies encode a peptide synthetase required for phytotoxin thaxtomin A production and pathogenicity. Mol. Microbiol. 38, 794–804. doi: 10.1046/j.1365-2958.2000.02170.x
Hu, Y., Lu, P., Zhang, Y., Li, L., and Chen, S. (2010). Characterization of an aspartate-dependent acid survival system in Yersinia pseudotuberculosis. FEBS Lett. 584, 2311–2314. doi: 10.1016/j.febslet.2010.03.045
Iwagami, S. G., Yang, K., and Davies, J. (2000). Characterization of the protocatechuic acid catabolic gene cluster from Streptomyces sp. strain 2065. Appl. Environ. Microbiol. 66, 1499–1508. doi: 10.1128/aem.66.4.1499-1508.2000
Joshi, M. V., Bignell, D. R. D., Johnson, E. G., Sparks, J. P., Gibson, D. M., and Loria, R. (2007). The AraC/XylS regulator TxtR modulates thaxtomin biosynthesis and virulence in Streptomyces scabies. Mol. Microbiol. 66, 633–642. doi: 10.1111/j.1365-2958.2007.05942.x
Kieser, T., Bibb, M. J., Buttner, M. J., Chater, K. F., and Hopwood, D. A. (2000). Practical Streptomyces Genetics. Norwich: The John Innes Foundation.
Kolattukudy, P. E., and Agrawal, V. P. (1974). Structure and composition of aliphatic constituents of potato tuber skin (suberin). Lipids 9, 682–691. doi: 10.1007/bf02532176
Komeil, D., Padilla-Reynaud, R., Lerat, S., Simao-Beaunoir, A.-M., and Beaulieu, C. (2014). Comparative secretome analysis of Streptomyces scabiei during growth in the presence or absence of potato suberin. Proteome Sci. 12, 35. doi: 10.1186/1477-5956-12-35
Komeil, D., Simao-Beaunoir, A.-M., and Beaulieu, C. (2013). Detection of potential suberinase-encoding genes in Streptomyces scabiei strains and other actinobacteria. Can. J. Microbiol. 59, 294–303. doi: 10.1139/cjm-2012-0741
Lerat, S., Simao-Beaunoir, A.-M., Wu, R., Beaudoin, N., and Beaulieu, C. (2010). Involvement of the plant polymer suberin and the disaccharide cellobiose in triggering thaxtomin A biosynthesis, a phytotoxin produced by the pathogenic agent Streptomyces scabies. Phytopathology 100, 91–96. doi: 10.1094/PHYTO-100-1-0091
Loria, R., Bukhalid, R. A., Creath, R. A., Leiner, R. H., Olivier, M., and Steffens, J. C. (1995). Differential production of thaxtomins by pathogenic Streptomyces species in vitro. Phytopathology 85, 537–541.
Lu, Y., Wang, W., Shu, D., Zhang, W., Chen, L., Qin, Z., et al. (2007). Characterization of a novel two-component regulatory system involved in the regulation of both actinorhodin and a type I polyketide in Streptomyces coelicolor. Appl. Microbiol. Biotechnol. 77, 625–635. doi: 10.1007/s00253-007-1184-5
MacNeil, D. J., Gewain, K. M., Ruby, C. L., Dezeny, G., Gibbons, P. H., and MacNeil, T. (1992). Analysis of Streptomyces avermitilis genes required for avermectin biosynthesis utilizing a novel integration vector. Gene 111, 61–68. doi: 10.1016/0378-1119(92)90603-m
Martins, I., Garcia, H., Varela, A., Núñez, O., Planchon, S., Galceran, M. T., et al. (2014). Investigating Aspergillus nidulans secretome during colonisation of cork cell walls. J. Proteomics 98, 175–188. doi: 10.1016/j.jprot.2013.11.023
Masai, E., Harada, K., Peng, X., Kitayama, H., Katayama, Y., and Fukuda, M. (2002). Cloning and characterization of the ferulic acid catabolic genes of Sphingomonas paucimobilis SYK-6. Appl. Environ. Microbiol. 68, 4416–4424. doi: 10.1128/aem.68.9.4416-4424.2002
Menendez-Bravo, S., Paganini, J., Avignone-Rossa, C., Gramajo, H., and Arabolaza, A. (2017). Identification of FadAB complexes involved in fatty acid β-oxidation in Streptomyces coelicolor and construction of a triacylglycerol overproducing strain. Front. Microbiol. 8:1428. doi: 10.3389/fmicb.2017.01428
Michielse, C. B., Reijnen, L., Olivain, C., Alabouvette, C., and Rep, M. (2012). Degradation of aromatic compounds through the β-ketoadipate pathway is required for pathogenicity of the tomato wilt pathogen Fusarium oxysporum f. sp. lycopersici. Mol. Plant Pathol. 13, 1089–1100. doi: 10.1111/j.1364-3703.2012.00818.x
Neilson, K. A., Ali, N. A., Muralidharan, S., Mirzaei, M., Mariani, M., Assadourian, G., et al. (2011). Less label, more free: approaches in label-free quantitative mass spectrometry. Proteomics 11, 535–553. doi: 10.1002/pmic.201000553
Park, H.-J., and Kim, E.-S. (2003). An inducible Streptomyces gene cluster involved in aromatic compound metabolism. FEMS Microbiol. Lett. 226, 151–157. doi: 10.1016/s0378-1097(03)00585-8
Park, S., Smith, L. T., and Smith, G. M. (1995). Role of glycine betaine and related osmolytes in osmotic stress adaptation in Yersinia enterocolitica ATCC 9610. Appl. Environ. Microbiol. 61, 4378–4381.
Pfaffl, M. W. (2001). A new mathematical model for relative quantification in real-time RT-PCR. Nucleic Acids Res. 29:e45.
Riley, R. G., and Kolattukudy, P. E. (1975). Evidence for covalently attached p-coumaric acid and ferulic acid in cutins and suberins. Plant Physiol. 56, 650–654. doi: 10.1104/pp.56.5.650
Rivas-Sendra, A., Landete, J. M., Alcántara, C., and Zúñiga, M. (2011). Response of Lactobacillus casei BL23 to phenolic compounds. J. Appl. Microbiol. 111, 1473–1481. doi: 10.1111/j.1365-2672.2011.05160.x
Sengupta, K., Swain, M. T., Livingstone, P. G., Whitworth, D. E., and Saha, P. (2019). Genome sequencing and comparative transcriptomics provide a holistic view of 4-nitrophenol degradation and concurrent fatty acid catabolism by Rhodococcus sp. strain BUPNP1. Front. Microbiol. 9:3209. doi: 10.3389/fmicb.2018.03209
Serra, O., Hohn, C., Franke, R., Prat, S., Molinas, M., and Figueras, M. (2010). A feruloyl transferase involved in the biosynthesis of suberin and suberin-associated wax is required for maturation and sealing properties of potato periderm. Plant J. 62, 277–290. doi: 10.1111/j.1365-313X.2010.04144.x
Sineli, P. E., Herrera, H. M., Cuozzo, S. A., and Dávila Costa, J. S. (2018). Quantitative proteomic and transcriptional analyses reveal degradation pathway of γ-hexachlorocyclohexane and the metabolic context in the actinobacterium Streptomyces sp. M7. Chemosphere 211, 1025–1034. doi: 10.1016/j.chemosphere.2018.08.035
Sutherland, J. B., Crawford, D. L., and Pometto, A. L. (1983). Metabolism of cinnamic, p-coumaric, and ferulic acids by Streptomyces setonii. Can. J. Microbiol. 29, 1253–1257. doi: 10.1139/m83-195
Uraji, M., Tamura, H., Mizohata, E., Arima, J., Wan, K., Ogawa, K., et al. (2018). Loop of Streptomyces feruloyl esterase plays an important role in the enzyme’s catalyzing the release of ferulic acid from biomass. Appl. Environ. Microbiol. 84, e2300–e2317.
Venkatesh, J., Kumar, P., Krishna, P. S. M., Manjunath, R., and Varshney, U. (2003). Importance of uracil DNA glycosylase in Pseudomonas aeruginosa and Mycobacterium smegmatis, G+C-rich bacteria, in mutation prevention, tolerance to acidified nitrite, and endurance in mouse macrophages. J. Biol. Chem. 278, 24350–24358. doi: 10.1074/jbc.m302121200
Wang, Y., Cobb, R. E., and Zhao, H. (2016). High-efficiency genome editing of Streptomyces species by an engineered CRISPR/Cas system. Methods Enzymol. 2016, 271–284. doi: 10.1016/bs.mie.2016.03.014
Wu, F., Shi, Q., Wang, X.-J., Sun, Z.-T., Wang, W., Li, X., et al. (2019). Streptomyces canus GLY-P2 degrades ferulic and p-hydroxybenzoic acids in soil and affects cucumber antioxidant enzyme activity and rhizosphere bacterial community. Plant Soil 436, 71–89. doi: 10.1007/s11104-018-03911-z
Yang, W., Tang, H., Ni, J., Wu, Q., Hua, D., Tao, F., et al. (2013). Characterization of two Streptomyces enzymes that convert ferulic acid to vanillin. PLoS One 8:e67339. doi: 10.1371/journal.pone.0067339
Keywords: common scab, hydroxycinnamates, trans-ferulic acid, Streptomyces scabiei, suberin
Citation: Khalil M, Lerat S, Beaudoin N and Beaulieu C (2019) The Plant Pathogenic Bacterium Streptomyces scabies Degrades the Aromatic Components of Potato Periderm via the β-Ketoadipate Pathway. Front. Microbiol. 10:2795. doi: 10.3389/fmicb.2019.02795
Received: 02 July 2019; Accepted: 18 November 2019;
Published: 04 December 2019.
Edited by:
Davide Zannoni, University of Bologna, ItalyReviewed by:
Kapil Tahlan, Memorial University of Newfoundland, CanadaDawn Bignell, Memorial University of Newfoundland, Canada
Copyright © 2019 Khalil, Lerat, Beaudoin and Beaulieu. This is an open-access article distributed under the terms of the Creative Commons Attribution License (CC BY). The use, distribution or reproduction in other forums is permitted, provided the original author(s) and the copyright owner(s) are credited and that the original publication in this journal is cited, in accordance with accepted academic practice. No use, distribution or reproduction is permitted which does not comply with these terms.
*Correspondence: Carole Beaulieu, Y2Fyb2xlLmJlYXVsaWV1QHVzaGVyYnJvb2tlLmNh