- 1CAS Key Laboratory of Biogeography and Bioresource in Arid Land, Xinjiang Institute of Ecology and Geography, Ürümqi, China
- 2Leibniz Centre for Agricultural Landscape Research (ZALF), Müncheberg, Germany
- 3Faculty of Biology, National University of Uzbekistan, Tashkent, Uzbekistan
- 4DST-CPR, Babasaheb Bhimrao Ambedkar University, Lucknow, India
- 5Department of Environmental Science, Babasaheb Bhimrao Ambedkar University, Lucknow, India
Soil salinity has emerged as a serious issue for global food security. It is estimated that currently about 62 million hectares or 20 percent of the world’s irrigated land is affected by salinity. The deposition of an excess amount of soluble salt in cultivable land directly affects crop yields. The uptake of high amount of salt inhibits diverse physiological and metabolic processes of plants even impacting their survival. The conventional methods of reclamation of saline soil which involve scraping, flushing, leaching or adding an amendment (e.g., gypsum, CaCl2, etc.) are of limited success and also adversely affect the agro-ecosystems. In this context, developing sustainable methods which increase the productivity of saline soil without harming the environment are necessary. Since long, breeding of salt-tolerant plants and development of salt-resistant crop varieties have also been tried, but these and aforesaid conventional approaches are not able to solve the problem. Salt tolerance and dependence are the characteristics of some microbes. Salt-tolerant microbes can survive in osmotic and ionic stress. Various genera of salt-tolerant plant growth promoting rhizobacteria (ST-PGPR) have been isolated from extreme alkaline, saline, and sodic soils. Many of them are also known to mitigate various biotic and abiotic stresses in plants. In the last few years, potential PGPR enhancing the productivity of plants facing salt-stress have been researched upon suggesting that ST-PGPR can be exploited for the reclamation of saline agro-ecosystems. In this review, ST-PGPR and their potential in enhancing the productivity of saline agro-ecosystems will be discussed. Apart from this, PGPR mediated mechanisms of salt tolerance in different crop plants and future research trends of using ST-PGPR for reclamation of saline soils will also be highlighted.
Introduction
Since the inception of agricultural practices, the question that how to enhance crop productivity to feed the growing population has been challenging. As highlighted in 2018 Global Agricultural Productivity (GAP) Index the current growth rate of agricultural production is not enough to meet the projected food demand of 10 billion people in 2050 (GAP Report, 2018). The report also stated that under such circumstances GAP must be increased by 1.75% annually. Enhancing crop productivity in agro-ecosystems is intricate and greatly influenced by pedo-climatic conditions, farming systems and management techniques (Nemecek and Gaillard, 2010). A number of abiotic factors including temperature, salinity, drought, pesticides and fertilizer application, soil pH, and heavy metal contamination also hamper crop productivity (Ahmad, 2014). Amongst all these, salinization of arable land is being considered as a real menace for agricultural production. Recently, the United Nations’ Food and Agriculture Organization (FAO and ITPS, 2015) report “The status of the world’s soil resources” has identified nine major threats to soil functions and soil salinization is one of them. Soil salinization is globally expanding and in the past few years, the accelerated rate of salinization has also created food insecurity in several countries. The delta regions of India, Myanmar and Bangladesh which majorly contribute in world rice production are facing serious threats to food security due to salinization of coastal soil (Abedin et al., 2014; Szabo et al., 2016). According to Ghassemi et al. (1995) salt-affected, irrigated areas caused annual income losses in terms of $12 billion globally. In the United States, the central California region is losing approximately $3.7 billion in agricultural crop yield every year due to salinity (Dove, 2017). In Alberta, western province of Canada, on an average, 25 percent of crop yields are reduced annually due to salinity. Sindh region of Pakistan is suffering 31 percent crop loss annually due to waterlogging and salinity (Ilyas, 2017). These are just a few examples of this global menace.
In excess, any form of salt is deleterious for plant health. Soils with high salinity levels interfere with plants’ physiological process. The high salt concentration adversely affects important soil processes such as respiration, residue decomposition, nitrification, denitrification, soil biodiversity and microbial activity (Schirawski and Perlin, 2018). The loss of crop productivity and high salinity is also noticed where fertilizer input is too high in soil (Rütting et al., 2018). Application of high salt index fertilizers impose an osmotic effect which causes difficulty in extraction of water required for plant growth (Herger et al., 2015). It has also been observed that farming practices also affect crop production in saline soils. In such cases plowing down or tilling deeper increases the evaporation of water from the soil surface and deposit more salts. Apart from this, salts present in irrigation water may also increase soil salinity that results in productivity loss (Rengasamy, 2010; Arora et al., 2018). Earlier it was estimated that around 20 to 50 percent of irrigated lands worldwide were salt-affected (Szabolcs, 1992). It has also been projected that a huge proportion of the globe i.e., 24% or 60 million ha is being affected due to inappropriate irrigation practices (WWAP, 2012). Removal of salt from saline soil is an intensive process and requires too much time and money (Qadir et al., 2014). However, since long, reclamation of saline soils is mainly performed by physical and chemical processes. In a physical process, soluble salts in the root zone are removed by scraping, flushing and leaching methods (Ayyam et al., 2019). However, in chemical methods use of gypsum and lime as neutralizing agents is commonly done (Keren, 2005). But these methods are not sustainable and also considered inefficient when the salt concentration is too high.
Planting salt-tolerant crop varieties such as barley and canola on saline soils is common practice (Fita et al., 2015). However, due to normal salt tolerance profile, these crops have limited global reach and can’t be used in the soil with moderate or high electrical conductivity (EC) levels. Morton et al. (2019) also highlighted that despite vigorous efforts from the research community, only few salt tolerance genes have been identified having real applications in improving productivity of saline soils.
Hence, securing attainable crop yield in saline soils is the need of the hour and aside from using salt-tolerant varieties or amelioration methods involving chemical neutralizers, sustainable approaches should also be employed. In the last few years, research showed that the use of salt-tolerant plant growth promoting rhizobacteria (ST-PGPR) in saline agriculture can be harnessed for enhancing productivity and improving soil fertility as well (Grover et al., 2011). Their adaptive responses toward salt stress are related to the ability to produce osmoprotectants, compatible solutes, and specialized transporters. ST-PGPR are now being used as bioinoculants for enhancing crop yields, protection from phytopathogens and improving soil health. The present review targets the use of ST-PGPR for improving the productivity of crops grown in salt-affected soil. Their application in the form of bioinoculants for enhancing crop yield is also targeted. Apart from this, ST-PGPR mediated mechanisms, including the new insights and perspectives on productivity enhancement of crops facing the salinity stress are also discussed.
Saline Soils: Global Distribution
Although, it has been realized that all continents on the globe are facing the problem of soil salinity (Figure 1) yet accurate estimation of the locations and distribution of saline soils is missing. The Food and Agriculture Organization (FAO), United Nations Educational, Scientific and Cultural Organization –United Nations Environment Programme (UNESCO-UNEP), and International Society of Soil Science (ISSS) are the leading world agencies that paid attention in gathering data on quality of soil across the globe. The Soil Map of the World (FAO, 1971–1981) documented that a total area of 953 Million hectares (Mha) is salt-affected. According to FAO report on “Status of the World’s Soil Resources” soil of more than 100 countries with an estimated area of approximately one billion hectares is afflicted with the problem of salinity (FAO and ITPS, 2015). Due to very high amount of soluble salts (NaCl and Na2SO4) the EC of these soils exceeds 4 dSm–1. Currently, the soil classification system is governed by the World Reference Base for Soil Resources (WRB) which is endorsed by the International Union of Soil Sciences (IUSS) and it replaced the FAO/UNESCO Legend for the Soil Map of the World. The legend of the soil map described salt-affected soil as solonchak and solonetz. Solonchaks are characterized by the accumulation of high soluble salts. The salic horizon starts within ≤50 cm from the soil surface and are largely distributed to the arid and semi-arid coastal regions in all climatic zones in the world. The estimated global area of solonchaks is around 260 million hectares (IUSS Working Group WRB, 2015). According to national soil classification systems, solonchaks belong to Halomorphic Soils (Russia), Halosols (China) and Salids (United States of America) and EC may range from 8 to 15 dSm–1. On the basis of salt precipitation, there may be external solonchaks (deposition at the surface) or internal solonchaks (deposition at depth). Whereas solonetz which are commonly known as alkaline soils and sodic soils contain a large proportion of adsorbed Na and in some cases Mg ions also. However, solonetz with free Na2O3 are considered highly alkaline and their pH is recorded to be higher than 8.5. Globally, they are distributed in the semi-arid temperate continental climate. Area-wide, a total of 135 Mha solonetz are found in Ukraine, the Russian Federation, Kazakhstan, Hungary, Bulgaria, Romania, China, the United States of America, Canada, South Africa, Argentina, and Australia (IUSS Working Group WRB, 2015).
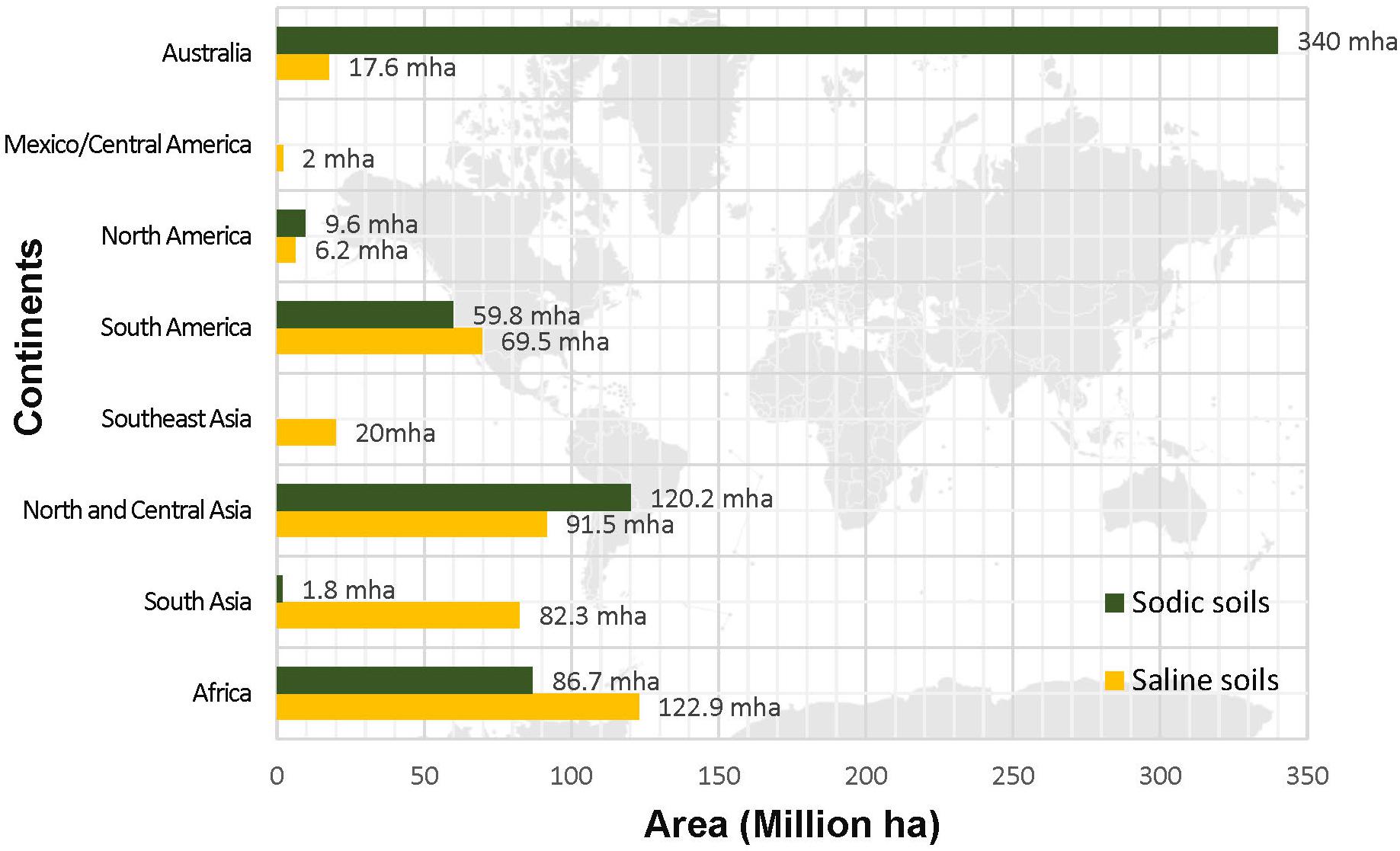
Figure 1. Global distribution of saline and sodic soil (Source: FAO and ITPS, 2015).
The Diversity of Salt-Tolerant Plant Growth Promoting Rhizobacteria (St-Pgpr)
Soil has a huge versatility of microorganisms, belonging to different groups of bacteria, fungi, and archaea. Amongst microbes, some are now well known for their inherent capability to tolerate different concentrations of salt and to promote plant growth as well. These salt-tolerant plant beneficial microbes have great importance in agriculture. They have shown their potential in improving crop productivity in arid and semiarid regions (Niu et al., 2018). The genera Pseudomonas, Bacillus, Enterobacter, Agrobacterium, Streptomyces, Klebsiella, and Ochromobacter are best reported for improving the productivity of diverse crops under saline conditions (Sharma et al., 2016; Singh and Jha, 2016; Sarkar et al., 2018). The diazotrophic salt-tolerant bacterial strains of Klebsiella, Agrobacterium, Pseudomonas, and Ochrobactrum isolated from the roots of a halophytic plant, Arthrocnemum indicum showed salinity tolerance ranging from 4 to 8% NaCl, and improved the productivity of peanut in saline as well as in control conditions (Sharma et al., 2016). Planococcus rifietoensis, an alkaliphilic bacterium is reported to enhance growth and yield of wheat crop under salinity stress (Rajput et al., 2013). Upadhyay et al. (2009) explored the genetic diversity of ST-PGPR isolated from the wheat rhizosphere. They found that most of the isolates were able to tolerate up to 8% NaCl and belong to the genus Bacillus. The diversity of salt-tolerant bacteria isolated from paddy rhizosphere in Taoyuan, China was reported by Zhang et al. (2018). They isolated 305 bacterial strains, and amongst them, 162 were tested for salt tolerance up to 150 g/l NaCl concentration. Phylogenic analysis of 74 of these salt-tolerant strains showed that they belong to orders Bacillales (72%), Actinomycetales (22%), Rhizobiales (1%), and Oceanospirillales (4%). Most of the isolates also showed their potential in improving salt tolerance, growth, and yield of rice under salt-stress conditions. ST-PGPR strain Bacillus licheniformis SA03 isolated from Chrysanthemum plants grown in saline-alkaline soil of China conferred increased salt tolerance in Chrysanthemum (Zhou et al., 2017).
The diversity of salt-tolerant bacilli was also deciphered in the soil of eastern Indo Gangetic plains of India by Sharma et al. (2015). They isolated 95 bacterial strains and amongst them, 55 showed plant growth promoting characteristics and salt-tolerance to more than 4% NaCl. Several researchers also report the diversity of ST-PGPR in the coastal areas. For example, in Tsunami affected regions in Andaman and Nicobar Islands of India, 121 bacterial strains were isolated, and amongst them 23 showed salt tolerance up to 10% NaCl with PGP characteristics including production of indole acetic acid (IAA), siderophore, extracellular enzymes and phosphate solubilization (Amaresan et al., 2016). The study revealed that the majority of isolates were Bacillus spp. and rest were Alcaligenes faecalis, Microbacterium resistance, Enterobacter sp., Lysinibacillus sp. A recent study claimed the presence of a novel salt-tolerant bacterial strain Pseudomonas sp. M30-35 from the rhizosphere of Haloxylon ammodendron, a C4 perennial succulent xerohalophyte with outstanding drought and salt tolerance capabilities. Pseudomonas sp. M30-35 was found to contain 34 genes possessing homology with certain genes associated with PGP traits and abiotic stress tolerance (He et al., 2018). Recently, the genome of many ST-PGPR have also been sequenced which envisaged information about their salt tolerance and plant growth promoting attributes. Kothari et al. (2013) reported that Bacillus safensis VK from the desert of Gujrat, India, showed salt tolerance up to 14% NaCl and pH ranging from 4 to 8. Further, study of this B. safensis strain deciphered that its genome harbors several genes associated with PGP traits functioning in conditions of high salt concentrations, drought, heavy metals, and polyaromatic hydrocarbons (PAHs) contamination. ST-PGPR Klebsiella sp. IG 3 isolated from the rhizosphere of wheat showed salt tolerance up to 20%. This strain positively modulated the expression profile of rbcL (codes for the ribulose-1,5-bisphosphate carboxylase/oxygenase RuBisCo) and WRKY1 (transcription factor dealing with plants reaction to biotic stress) genes under salt-stress conditions (Sapre et al., 2018). In another study, whole genome analysis of a halotolerant PGPR Klebsiella sp. D5A revealed the presence of salt tolerance genes with a wide range of pH adaptability and PGP traits including phosphate solubilization, IAA biosynthesis, acetoin, and 2,3-butanediol synthesis, siderophore production, and N2 fixation (Liu et al., 2016). Pseudomonas putida and Novosphingobium sp. are reported to reduce salt-stress induced damage in citrus plants by lowering the level of abscisic acid (ABA) and salicylic acid (SA), reducing the efficiency of photosystem II (Fv/Fm), increasing accumulation of IAA in the leaf and inhibiting accumulation of root chloride and proline during salt stress (Vives-Peris et al., 2018). A Pseudomonas strain isolated from halophilic grass Distichlis spicata also improved the growth of different crops under salt stress (Palacio-Rodríguez et al., 2017). A ST-PGPR strain Enterobacter sp. UPMR18 with ability to produce 1-aminocyclopropane-1-carboxylic acid (ACC) deaminase showed improvement in crop productivity through induction of reactive oxygen species (ROS) scavenging enzymes including superoxide dismutase (SOD), ascorbate peroxidase (APX) and catalase (CAT) and upregulating to ROS pathway genes (Habib et al., 2016a). Recently, functional metagenomics provided a magnificent way of identification of various genes responsible for salt resistance in microorganisms.
Effect of Soil Salinization on Crops
Soil salinity has an overall detrimental effect on plants’ health (Figure 2). Salinity affects flowering and fruiting pattern, aberration in reproductive physiology, which ultimately influences crop yields and biomass. Salinity may cause up to 50% reduction in flowering of pigeon pea (Cajanus cajan L. Mill) (Promila and Kumar, 1982). In tomato, high salt stress (150 mm NaCl) is reported to affect flowering transition time and causes delay in the first inflorescence along with reduction in the growth of shoot and root (Ghanem et al., 2009). In chickpea (Cicer arietinum L.), delayed flowering was directly linked with the higher concentrations of Na+ in the laminae of completely expanded leaves (Pushpavalli et al., 2016). Salt Overly Sensitive (SOS) pathway is a major defense pathway involved in Na+ extrusion and maintaining ion homeostasis at the cellular level (Zhu et al., 1998; Zhu, 2003; Ji et al., 2013). There are several reports where both SOS and photoperiodical and circadian clock switch proteins related with flowering are deactivated by salt stress (Kim et al., 2013; Park et al., 2013, 2016; Ryu et al., 2014).
Salt stress remarkably affects plant reproductive physiology. Ghanem et al. (2009) reported that in tomato the exposure of salinity stress results in Na+ accumulation in style, ovaries, and anther intermediate layers which caused an increase in the rate of flower abortion, reduction of pollen number and viability of the plant. Läuchli and Grattan (2007) reported that salt stress decelerates reproductive growth of wheat by inhibiting spike development and decreasing the yield potential, whereas in salt-sensitive rice, lowering of yield by the reduction in tillers, and formation of sterile spikelet is also experienced. The effect of salinity on Arabidopsis was explored in hydroponic solution which induced many symptoms including reduced fertility, decline in fruit length, transient wilting and fruits with predominantly aborted ovules and embryos which were narrower and reduced in size (Sun et al., 2004). Similarly, the effect of salt on early flowering and male gametophyte of canola (Brassica napus) plant showed a reduction in pollen grain numbers and abnormal growth of anthers. All these symptoms indirectly lead to reduced crop yield (Mahmoodzadeh and Bemani, 2008).
The drastic effect of salt stress can be seen in terms of yield loss. The primary effects related to crop yield can be in terms of germination which either decreases or sometimes ceases under extreme saline conditions. A study by Ali Khan et al. (2012) showed that under saline conditions growth, yield, and biomass of pearl millet is adversely affected in terms of germination percentage, plant height, leaf area, total biomass and grain yield plant–1. Impact of salinity on pea was also found to adversely affect growth, yield and biomass (Wolde and Adamu, 2018). Farooq et al. (2017) also reviewed the effects of salt stress on grain legumes, and they described that in different legumes salinity may reduce crop yield by 12–100%. Salt tolerance of black cumin (Nigella sativa L.) and its effect on seed emergence and germination, and yield were studied by Faravani et al. (2013). They showed that an increase in salinity level from 0.3 to 9 dS m–1 reduced the average seed and biological yield. Similarly, the effect of different levels of salinity on a weed plant Portulaca oleracea L. which is of nutritional importance and being utilized in same ways as spinach and lettuce in many countries, showed a reduction in biomass and yield, changes in physiological attributes, and alteration in stem and root structure (Amirul Alam et al., 2015). Salinity has thus a wide level of impacts on different crops, including even complete loss of yields. This also has impact on soil quality, greenhouse gas (GHG) emissions and food security.
Mechanisms of Pgpr Mediated Salt Stress Tolerance
The ST-PGPR utilize an array of mechanisms (Figure 3) which directly or indirectly take part in amelioration of salt stress in crop plants (Egamberdieva et al., 2016; Hashem et al., 2016). Studies confirmed that ST-PGPR produce various types of phytohormones, such as auxins, gibberellins, cytokinins (Dodd et al., 2010), synthesize ACC deaminase (Glick et al., 2007), produce secondary compounds such as exopolysaccharides (Upadhyay et al., 2012; Timmusk et al., 2014) and osmolytes (proline, trehalose, and glycine betaines) (Bano and Fatima, 2009; Upadhyay and Singh, 2015), regulate plant defense systems and activate plant’s antioxidative enzymes under salt stress (Hashem et al., 2016).
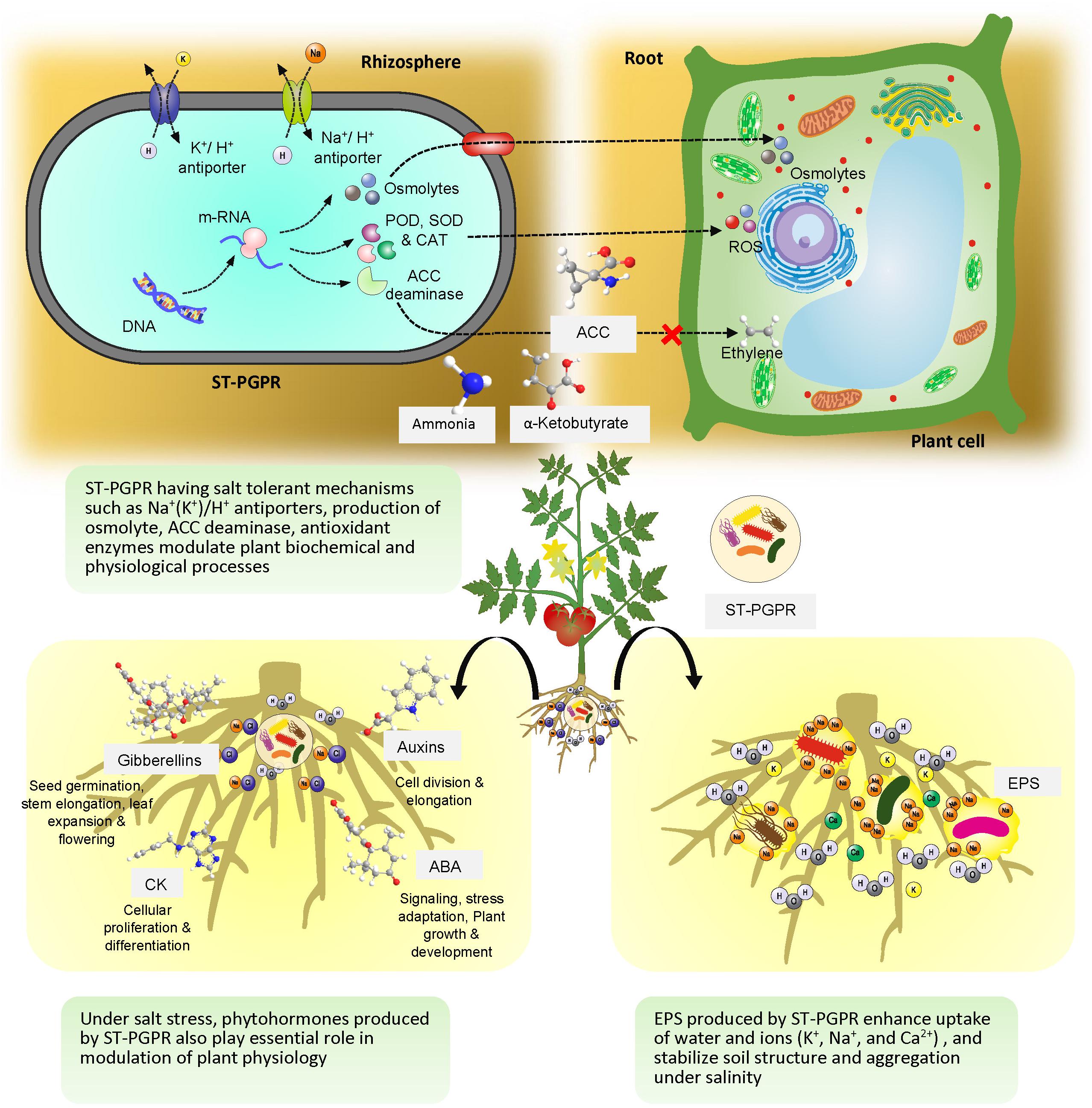
Figure 3. Salt-tolerant plant growth promoting rhizobacteria mediated mitigation of salt stress in plants.
Plant Growth Regulators
Phytohormones produced by ST-PGPR play an essential role in modulation of plant physiology under salt-stress (Egamberdieva and Kucharova, 2009). The ST-PGPR produce IAA which is required for cell division and elongation in plants coping with salt stress. Some of the best-known ST-PGPR producing IAA under salt stress are Azotobacter, Arthrobacter, Azospirillum, Pseudomonas, Stenotrophomonas, and Rahnella (Egamberdieva et al., 2008, 2018; Piccoli et al., 2011; Abd_Allah et al., 2017). Studies have confirmed that under salt stress yield loss in crops can be minimized by the application of phytohormone producing ST-PGPR. Under saline conditions, P. putida modulated IAA synthesis in plant tissue and increased the growth parameters of cotton (Yao et al., 2010). It has also been observed that inoculation of ST-PGPR increased the uptake of minerals, protected plants from ion toxicity and enhanced root and shoot growth under saline conditions (Egamberdieva et al., 2017a). Sadeghi et al. (2012) reported that salt-tolerant Streptomyces isolates with IAA producing ability improved the root system of wheat under salt stress. Recently, Kang et al. (2019) reported that IAA produced by a ST-PGPR Leclercia adecarboxylata MO1 has linkages with sugar synthesis, organic acid production and chlorophyll fluorescence improvement (Fv/Fm) in tomato. Apart from auxins other phytohormones are also reported to alleviate the effect of salt stress in plants. For example, production of cytokinins (CK), which are important for cellular proliferation and differentiation have been reported in salt-tolerant Arthrobacter, Bacillus, Halomonas, Azospirillum, and Pseudomonas species (García de Salamone et al., 2001; Karadeniz et al., 2006; Naz et al., 2009; TrParray et al., 2016). ABA is also synthesized by strains of ST-PGPR including Proteus mirabilis, Bacillus megaterium, B. licheniformis, Pseudomonas fluorescens, and Achromobacter xylosoxidans (Karadeniz et al., 2006; Forchetti et al., 2007; Salomon et al., 2014). Gibberellin producing bacterial strains such as Bacillus pumilus, B. licheniformis, Azospirillium sp. were also reported by Bottini et al. (2004). There are reports where ST-PGPR are known to produce more than one type of phytohormones. Patel and Saraf (2017) reported that bacterial strains of Pseuodomonas stutzeri, Stenotrophomonas maltophilia, and P. putida isolated from the Coleus rhizosphere produced IAA, gibberellic acid, and CK under saline conditions. Recently, Tewari and Arora (2018) reported the role of SA in ameliorating salinity stress and enhancing the growth of sunflower in saline soils.
ACC Deaminase Activity
The presence of ACC deaminase activity in ST-PGPR is a common phenomenon especially when exposed to high salt stress. The enzyme ACC deaminase cleaves ACC into ammonia and α-ketobutyrate which are consumed by the bacteria as nitrogen (N) and carbon sources (Glick, 2014). The presence of ST-PGPR dramatically lowers the level of stress ethylene and prevents inhibition of down-regulated genes involved in ethylene-induced plant stress and up-regulates genes involved in plant growth (Glick et al., 2007). There are many examples where ACC deaminase activity by ST-PGPR not only improved plant survival in saline soils but also enhanced productivity. Stenotrophomonas rhizophila synthesized ACC deaminase and stimulated growth of cucumber in saline soil (Egamberdieva et al., 2011). Ali et al. (2014) reported improvement in physiological properties of plants under salt stress by ACC deaminase producing P. fluorescens and Pseudomonas migulae strains. In another study, halotolerant bacterial strains, Brachybacterium saurashtrense (JG-06), Brevibacterium casei (JG-08), and Haererohalobacter (JG-11), producing ACC deaminase, improved salt tolerance in peanut grown in saline soils (Shukla et al., 2012). It has been found that ACC deaminase producing ST-PGPR have effects on other biochemical properties of plant cell, including membrane stability, biocompatible solutes formation and photosynthetic pigment production under drought and salt stress (Tiwari et al., 2018). Aslam and Ali (2018) also reported that ACC-deaminase activity in halolerant bacterial genera of Arthrobacter, Bacillus, Brevibacterium, Gracilibacillus, Virgibacillus, Salinicoccus, and Pseudomonas, Exiguobacterium isolated from the rhizosphere and phytoplane of Suaeda fruticosa (L.) Forssk stimulated growth of maize under saline conditions. The effect of ACC deaminase by ST-PGPR on nodule formation in legume crops is also well documented (Ahmad et al., 2011; Barnawal et al., 2014). In nodulation process, ACC deaminase is found to play an essential role to enhance persistence of infection threads which is negatively affected by ethylene level and thus help in nodule formation under saline conditions (Nascimento et al., 2016).
Osmoprotectants
Plants accumulate organic osmolytes such as proline, glycine, betaine, polyamines, quaternary ammonium compounds, and other amino acids in response to various abiotic stresses (Sandhya et al., 2010). ST-PGPR also employ this mechanism for protection against osmotic stress which is more common in saline soils (Mishra et al., 2018). While exposed to salt stress, salt-tolerant bacteria may temporarily increase their cytoplasmic content of K+, but the accumulation of osmolytes is a more sustained stress response to prevent water loss (Bremer and Kramer, 2019). During salt stress, internal concentration of organic osmolytes may reach up to 1 M in certain halophilic bacteria and has a major role in destabilization of the double helix and lower the Tm of DNA (Rajendrakumar et al., 1997). In moderately halophilic bacteria salinity induced expression of proline biosynthesis genes proH, proJ, and proA was reported at 2.5 M NaCl which lead to the highest accumulation of proline (Saum and Müller, 2007). Recently, Kushwaha et al. (2019) explored osmoprotection in Halomonas sp. SBS 10 and found that at low NaCl, betaine accumulation suppresses the de novo synthesis of ectoine whereas at a high NaCl concentration, the ectoine concentration increases abruptly as compared to the betaine. Further, they concluded that ectoine accumulation is transcriptionally up-regulated by the salinity stress. In another study it was observed that Azospirillum spp. accumulate proline, glycine betaine, and trehalose, supporting the plant to withstand osmotic stress (Rodríguez-Salazar et al., 2009). A ST-PGPR strain of Bacillus sp. increased growth and development of maize under drought and salinity through accumulation of proline and soluble sugars (Vardharajula et al., 2011). Role of the trehalose as an osmoprotectant under salt-stress is also well documented and a large number of ST-PGPR have been discovered having genes for trehalose biosynthetic pathways (Qin et al., 2018; Orozco-Mosqueda et al., 2019; Shim et al., 2019).
Exopolysaccharides (EPS)
Production of EPS or surface polysaccharides is a general characteristic of some rhizosphere bacteria. Although, the composition and amount of EPS may vary in different ST-PGPR strains, copious amount of EPS is formed in adverse conditions (Bomfeti et al., 2011; Tewari and Arora, 2014a; Khan and Bano, 2019). EPS work as physical barrier around roots and support plant growth in high salinity stress (Vaishnav et al., 2016). Inoculation of EPS producing ST-PGPR also showed ameliorative effects on the uptake of K+, Na+, and Ca2+ in plants (Ashraf et al., 2004; Kohler et al., 2006). Qurashi and Sabri (2012a) showed that EPS producing ST-PGPR Halomonas variabilis (HT1) and P. rifietoensis (RT4) improved chickpea growth and stabilization of soil structure and aggregation under salinity. The presence of EPS in the biofilm also has positive effects on root colonization by ST-PGPR (Qurashi and Sabri, 2012b). In the context of yield improvement the role of EPS producing ST-PGPR is very significant as they are being used as priming agents of seeds and help in enhancing germination (Tewari and Arora, 2014a). Atouei et al. (2019) found that salt-tolerant EPS producing B. subtilis subsp. inaquosorum and Marinobacter lipolyticus SM19 reduced adverse effects of salinity and drought stresses in wheat. Recently, Chu et al. (2019) showed possible role of EPS producing salt-tolerant Pseudomonas PS01 strain in regulation of genes related to stress tolerance in Arabidopsis thaliana. They found that a LOX2 gene which encodes a lipoxygenase that constitutes an essential component of the jasmonic acid (JA) synthesis pathway was up-regulated. As JA itself is a positive regulator and accumulates rapidly in plants (under salt stress), bacterial EPS provide additional benefits to survive under salt stress.
Antioxidant Enzymes
Inoculation of plants with ST-PGPR decreases the negative effects of oxidative stress by producing antioxidative enzymes (Manaf and Zayed, 2015; Islam et al., 2016). There are evidences where ST-PGPR produced high concentration of antioxidant enzymes including POD, SOD, CAT, and nitrate reductase (NR), glutathione reductase (GR) under salinity stress (Jha and Subramanian, 2013; Sen and Chandrasekhar, 2015; Ansari et al., 2019). El-Esawi et al. (2019) observed that Azospirillum lipoferum FK1 inoculated plants demonstrated a higher expression level of the antioxidant genes and thus improved antioxidant enzymes and non-enzymatic metabolites, nutrient uptake, phenols and flavonoids content, growth, and development of chickpea. Similarly, Kohler et al. (2009) observed an increase in CAT activity in tissue of lettuce, reducing oxidative damage under saline conditions. Some ST-PGPR such as Enterobacter clocae, Pseudomonas pseudoalcaligenes and Bacillus sp., increased levels of APX and CAT in Jatropha leaves in response to salt stress and also stimulated the roots, increased biomass, N, phosphorus (P), potassium (K) uptake and chlorophyll content in the vegetative parts of the plant (Patel and Saraf, 2013). The salt-tolerant plant Sulla carnosa showed better growth and stress tolerance after inoculation with strains of Pseudomonas sp. and Bacillus sp. in saline Tunisian soils. The bacterial inoculants increased root and shoot biomass, and nutrient acquisition under salt stress by reducing stomatal conductance, and modulated antioxidant activities involved in plant stress responses (Hidri et al., 2016).
Taken together, these findings clearly suggest the important role of ST-PGPR in plant stress tolerance by modulating plant physiological and biochemical processes such as stress-related genes, osmolytes, and enzymatic and non-enzymatic antioxidants.
St-Pgpr for Improving Crop Productivity
It is now widely accepted that ST-PGPR are endowed with the inherent capability to cope with high concentration of salts in the soil. Their presence in the soil provides a direct benefit to plants. Their application in the form of bioinoculants/bioformulations not only improves crop productivity but also makes the survival of plants easier under extreme saline conditions (Table 1). In this section, the role of ST-PGPR in enhancing productivity of various crops is discussed.
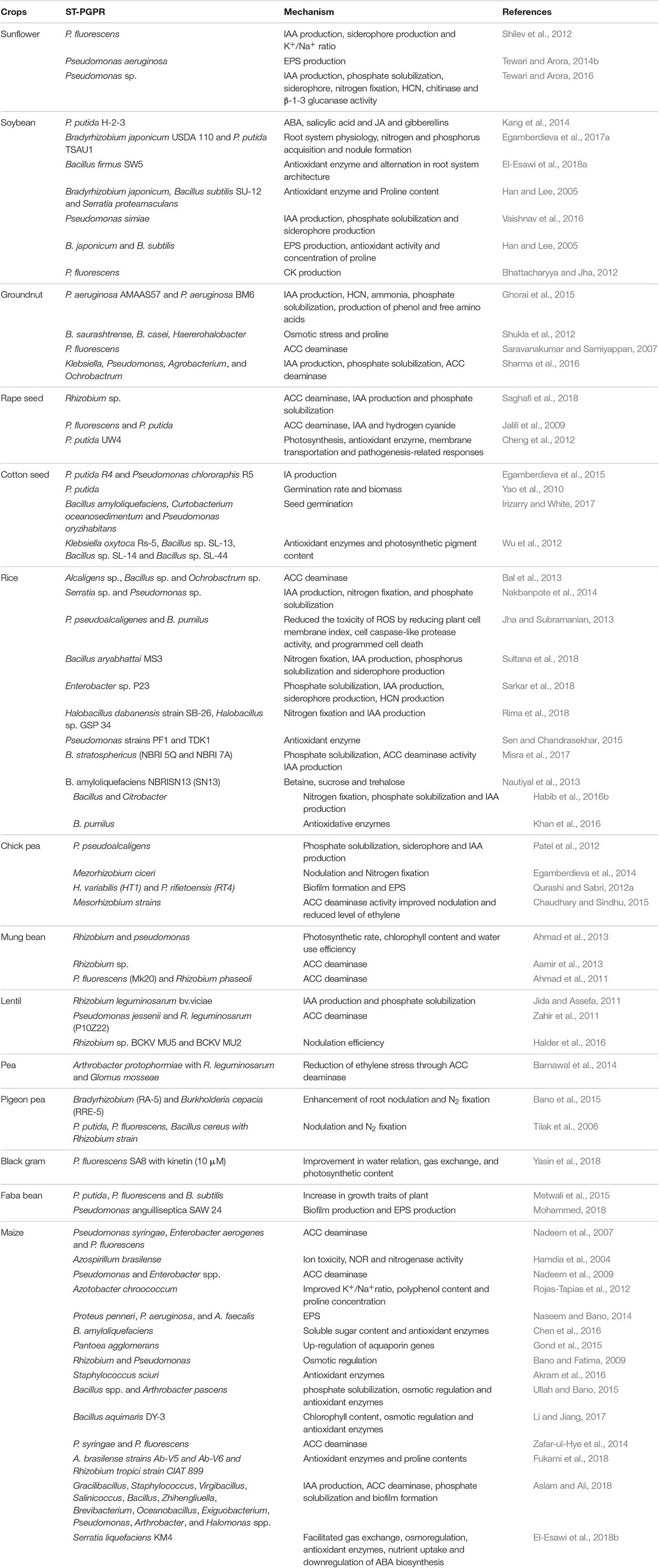
Table 1. Salt-tolerant plant growth promoting rhizobacteria and their possible roles in enhancing yield and growth of diverse plants/crops.
Cereal Crops
Cereal crops are the main source of energy and protein in the human diet. Worldwide, in comparison to other crops, cereal crops are cultivated in much higher quantity. Wheat, maize, rice, barley, oats, sorghum, and millet are known as major cereal crops. However, very few of them are reported as salt-tolerant. Since decades, most common approaches of enhancing yield of saline soils, which include conventional breeding, marker-assisted selection, and genetic engineering have also proven to be successful, but only for wheat and rice (Shahbaz and Ashraf, 2013; Roy et al., 2014). It has been realized that application of ST-PGPR in salt-affected soil not only assisted in the survival of crop but also improved yield in a wide range of cereal crops (Singh and Jha, 2016). In a study, ST-PGPR B. subtilis enhanced wheat yield by around 18% in salt-affected soil (EC 5.2 dSm–1) (Upadhyay and Singh, 2015). Research shows that application of ST-PGPR in rice may increase germination, promote seedling growth, induce antioxidative enzymes against ROS, favor osmolyte accumulation and modulate expression of genes related to salt stress (Nautiyal et al., 2013; Paul and Lade, 2014; Rima et al., 2018; Sarkar et al., 2018). The effect of various ST-PGPR in enhancing the productivity of salt-tolerant rice and wheat cultivated on sodic soils was explored by Damodaran et al. (2019) and they found that Lysinibacillus sp. was most effective in ameliorating the adverse effect of salinity. Similarly, a comprehensive research on the diversity of ST-PGPR in different agro-climatic zones was conducted by Misra et al. (2017) and they revealed that amongst all, Bacillus sp. with ACC deaminase activity were most dominant in amelioration of salt stress and enhancing biomass of rice. A ST-PGPR, Pseudomonas strain 002 was found to improve root formation in maize under salinity (150 mM NaCl) stress (Zerrouk et al., 2016). The effect of ST-PGPR S. sciuri SAT-17 strain on anti-oxidative defense mechanisms and modulation of maize growth under salt stress was studied by Akram et al. (2016). They reported that inoculation of maize with SAT-17 improved plant growth and decreased the ROS levels by increasing the cellular antioxidant enzyme activities (CAT, POD, and proline) under salinity treatments (75 and 150 mM NaCl).
Legumes and Oil Yielding Crops
Along with the cereals, legumes reserve their position as important source of protein in the human diet. Salinity hampers production of grain and food legumes in many regions around the globe (Manchanda and Garg, 2008). In legumes, salt-stress adversely effects root-nodule formation, symbiotic relation and finally the nitrogen fixation capacity (Manchanda and Garg, 2008). Symbiotic association of rhizobia with legumes under salinity stress is still a broad area of research (Zahran, 1991, 1999; Graham, 1992). Most of the findings reveal that application of salt-tolerant rhizobia is a sustainable solution for enhancing the productivity of legume crops grown under salinity stress (Abiala et al., 2018). Some workers have demonstrated that negative impacts of salinity on legumes including soybean, pigeon pea, common bean, mung bean, groundnut and even tree legumes can be minimized by the application of salt-tolerant rhizobial strains (Bashan and Holguin, 1997; Kumar et al., 1999; Dobbelaere et al., 2001; Bashan et al., 2004; Dardanelli et al., 2008; Meena et al., 2017; Yasin et al., 2018). Salt-tolerant species of Pseudomonas are reported to improve the health of groundnut (Saravanakumar and Samiyappan, 2007), common beans (Egamberdieva, 2011), faba beans (Metwali et al., 2015), and chickpea (Jatan et al., 2019). In a study, application of ST-PGPR B. firmus SW5 on soybean grown under salt stress showed its beneficial effect on nutrient uptake, chlorophyll synthesis, osmolyte levels, gas exchange parameters, total phenolics, flavonoid contents, and antioxidant enzyme activities, in comparison to control (El-Esawi et al., 2018a). Wang et al. (2016) found that ACC-deaminase containing rhizobacterium Variovorax paradoxus 5C-2 increased total biomass of pea by 25 and 54% under salinity levels of 70 and 130mM NaCl, respectively. In a study, Yanni et al. (2016) used four strains of Rhizobium as bioinoculants for common bean in saline and drought stressed fields and found that inoculation increased seed yield considerably. A combined effect of R. phaseoli with L-tryptophan on the mung bean grown under salinity stress was evaluated by Zahir et al. (2010) and it was found that the application induced more pronounced effects and increased the plant height, number of nodules per plant, plant biomass, grain yield, and grain N concentration as compared with the untreated control. In another study, Ahmad et al. (2011) evaluated the combined application of salt-tolerant Rhizobium and Pseudomonas under salt-stressed conditions for improving the productivity of mung bean. They found that co-inoculation increased all the growth parameters including seed yield by 150% as compared with un-inoculated control. B. japonicum USDA 110 and P. putida TSAU1 were reported with salt tolerance activity and abilities to work synergistically with each other to enhance growth and productivity of soybean under high salinity (Egamberdieva et al., 2017b).
Salinity halts the growth and affects the oil yield in several legumes. Field application of ST- PGPR in oil-yielding crops has proven beneficial in remediation of salt stress (Tewari and Arora, 2014a,b). In other studies fluorescent Pseudomonas improved root and shoot length of sunflower under saline conditions (Tewari and Arora, 2016) and ST-PGPR Klebsiella, Agrobacterium, Pseudomonas, and Ochrobactrum enhanced salt-tolerance in groundnut crop (Sharma et al., 2016). Similarly, salt-tolerant P. fluorescens strain TDK1 with ACC deaminase activity also enhanced the salt resistance in groundnut plants, which in turn enhanced yields (Saravanakumar and Samiyappan, 2007). Furthermore, co-inoculation of B. japonicum USDA 110 and salt-tolerant P. putida TSAU1 were found to improve growth parameters, protein content, N, and P uptake and root system architecture of soybean under salt stress (Egamberdieva et al., 2017a).
Vegetable Crops
Globally, about 1.1 percent of the total agricultural area is covered with vegetables1 and China and India are the leading countries in this regard. The threshold of salinity for most vegetable crops is ≤2.5 dS m–1 hence compared with others, vegetable crops are more prone to damage caused due to salinity stress (Shannon and Grieve, 1998). Unfortunately, research on the role of ST-PGPR in alleviating salt stress of vegetable crops is very scarce. However, there are some reports about the role of ST-PGPR in enhancing the performance of vegetable crops under salinity stress. Mayak et al. (2004) isolated an Achromobacter piechaudii bacterium from Arava region of southern Israel which significantly increased the fresh and dry weights of tomato seedlings at high salt concentration. Similarly, Tank and Saraf (2010) also found that the ST-PGPR P. stutzeri improved salt tolerance in tomato plants. Recently, Van Oosten et al. (2018) reported that inoculation of tomato plants with A. chroococcum 76A under both moderate (50 mM NaCl) and severe (100 mM NaCl) salt stresses increased growth parameters i.e., shoot dry weight, fresh fruit weight and fruit number per plant as compared to the control. Abd El-Azeem et al. (2012) reported that ST-PGPR strains of Xanthobacter autotrophicus BM13, E. aerogenes BM10, and Bacillus brevis FK2 alleviated negative effects of salt and improved growth and yield in egg plant. Furthermore, Ge and Zhang (2019) showed that under salt stress (3% NaCl), Rhodopseudomonas palustris G5 increased shoot height, root length, fresh weight, dry weight, total chlorophyll content and soluble sugar content of cucumber seedlings. Recently, Tahir et al. (2019) conferred that consortium of salt-tolerant Bacillus strains enhanced potato tuber yield by the production of auxin, antioxidant enzymes and regulating uptake of Na+, K+, and Ca+2 in normal and salt affected soils. ST-PGPR have proven to be beneficial for cultivation of vegetables in saline soils, however, this needs to be explored and utilized further.
Future Prospects
Although much progress has been made in understanding the effect of salinity on plants, little success is achieved in the sustainable management of productivity losses. The potential of ST-PGPR can be harnessed for the improvement of crop yield of saline soils. According to Pan et al. (2019) physiological roles played by ST-PGPR could improve plant performance under saline conditions. It has been realized that elucidation of mechanisms of osmo-adaptation in ST-PGPR may contribute to the long-term goal of improvement of productivity in crops grown in saline agro-ecosystems (Paul, 2013). Knowledge of salt tolerance mechanisms in ST-PGPR is still not up to mark, particularly about the involvement of bacterial genes in osmotic regulation and plant-microbe interactions (under saline conditions). Although, in the last few years, molecular mechanisms of salt tolerance have been studied in several halophilic bacteria. This revealed that amongst various mechanisms, bacterial salt-related antiporters such as Na+/H+ play specific roles in salinity tolerance in plants as well (Zhong et al., 2012). Ma et al. (2019) stated that the understanding of regulatory networks of ST-PGPR in inducing salt tolerance in plants, could serve as a promising measure to alleviate salt stress and improve global food production. However, a deeper investigation of microbial responses to soil salinity are required for their better utilization in the reclamation of saline soils. Kim et al. (2019) suggested that identification of the dominant indigenous microflora from the highly saline soil and their possible adaptation mechanisms may provide a better understanding for exploring ecological and evolutionary responses in ecosystems. The role of metagenomic and metabolomic approaches becomes very important in case of harnessing and identifying novel ST-PGPR, along with the key genes and metabolites involved in salt tolerance.
Crop specific field trials (Table 1) have already suggested strong ability of ST-PGPR in mitigation of salt stress (in diverse crops). However, replication of these research findings with same vigor in different geographical regions and on different plants has remained a challenge. This is why, in some of the instances, erratic results are obtained while using PGPR inoculants at field level (Souza et al., 2015; Ambrosini et al., 2016). Recently, Kumar et al. (2019) suggested that salinity related research on PGPR should follow certain protocols and screening is an important issue to maintain the quality and accuracy. They also addressed that use of indigenous ST-PGPR strains should be preferred in bioinoculant development as they can easily adapt to the local field conditions. Similarly, development of ST-PGPR based bioinoculant exclusively designed for saline soils could be more efficient approach in managing productivity loss in several crops (Mishra et al., 2018). Whereas prospects on future research of developing novel bioinoculants providing metabolites (osmolytes, biosurfactants, precursor of phytohormones and stress enzymes) along with efficient ST-PGPR strains (including consortia) should also be explored. Consortia based bioinoculants have recently gained importance because by involving diverse ST-PGPR not only salinity stress can be managed but phytopathogens can also be controlled and nutrients be assimilated simultaneously (Woo and Pepe, 2018). It has also been suggested that use of the diverse microbes such as PGPR, mycorrhiza and endosymbionts in consortial formulations can be a very promising strategy for combating stress in plants (Ilangumaran and Smith, 2017). At present there is lack of products of ST-PGPR for specific use in saline agro-ecosystems and available bioinoculants do not work in such conditions. This makes the end-user skeptical about the use of biological alternatives. The use of metabolites such as EPS has already been suggested and explored in some latest bio-products (Tewari and Arora, 2014b; Arora and Mishra, 2016). EPS can be cheaply produced at lab and industrial levels and can be added to bioinoculants prepared for saline soils. This metabolite can act as protectant for both the inoculated PGPR and the plant. Similarly other metabolites and carriers can also be explored for development of tailor-made bioformulations for saline soils.
Nowadays, several researchers are vigorously engaged to enhance the efficacy and applicability of ST-PGPR containing bioinoculants in field conditions (Ambrosini et al., 2016). Furthermore, a major part of research has to be devoted to improve the issues related with formulation development (Mishra et al., 2018). In recent past, development in the field of polymeric science devised amalgamated use of biopolymers and PGPR in agriculture (Raj et al., 2011; Sharif et al., 2018). For example, chitosan-immobilized aggregated Methylobacterium oryzae CBMB20 has been used as a bioinoculant to promote growth of tomato under salt stress conditions (Chanratana et al., 2019). Li et al. (2019) also showed synergistic use of a Super Absorbent Polymer (SAP) along with PGPR strains of Paenibacillus beijingensis BJ-18 and Bacillus sp. L-56 to overcome salinity stress in wheat and cucumber. Enhancing the productivity of saline soils will not only help in achieving food security but also result in enhanced content and quality of soil organic matter in these nutritionally poor agro-systems. This can help in reducing the carbon footprint and combating climate change as well (Arora, 2019; SDG, 2019).
Conclusion
Salt-tolerant plant growth promoting rhizobacteria have evolved several mechanisms to cope with salinity stress. They can easily withstand high salinity stress through various mechanisms such as efflux systems, formation and accumulation of compatible solutes for balancing external osmotic pressure, formation of ROS, secondary metabolites and other means. Several genes and metabolites are involved in maintaining the cell integrity and plant-microbe interactions under salinity stress. Still a lot is yet to be explored at molecular and biochemical level on how the ST-PGPR support themselves and their symbiotic partner under salinity stress which has multi-dimensional impacts on the cell (of both bacteria and plant). Research on ST-PGPR also indicates their vast potential in remediation and productivity enhancement of agro-ecosystems suffering from problems of salinity. However, in-depth studies targeting gene level expression and functional characteristics of ST-PGPR involved in plant growth promotion under salinity stress have to be conducted in the near future to design tailor-made bioformulations for saline soil systems, which are increasing worldwide, day by day. Utilization of this green biotechnology will have multi-faceted positive impacts on agro-ecosystems and rural environment.
Author Contributions
DE and NA conceptualized the idea. JM and NA prepared the illustrations. All authors equally contributed in writing of the manuscript.
Funding
This research was supported by the Chinese Academy of Sciences “President’s International Fellowship Initiative” (Grant No. 2018VBA002S) to DE.
Conflict of Interest
The authors declare that the research was conducted in the absence of any commercial or financial relationships that could be construed as a potential conflict of interest.
Acknowledgments
NA is thankful to Vice Chancellor, BBA University, Lucknow, Uttar Pradesh, India for support.
Footnotes
References
Aamir, M., Aslam, A., Khan, M. Y., and Usman, M. (2013). Co-inoculation with rhizobium and plant growth promoting rhizobacteria (PGPR) for inducing salinity tolerance in mung bean under field condition of semi-arid climate. Asian J. Agri. Biol. 1:7.
Abd El-Azeem, S. A. M., Elwan, M. W. M., Sung, J. K., and Ok, Y. S. (2012). Alleviation of salt stress in eggplant (Solanum melongena L.) by plant-growth-promoting rhizobacteria. Commun. Soil Sci. Plan. 43, 1303–1315. doi: 10.1080/00103624.2012.666305
Abd_Allah, E. F., Alqarawi, A. A., Hashem, A., Radhakrishnan, R., Al-Huqail, A. A., Al-Otibi, F. A., et al. (2017). Endophytic bacterium Bacillus subtilis (BERA 71) improves salt tolerance in chickpea plants by regulating the plant defense mechanisms. J. Plant Interact. 3, 37–44. doi: 10.1080/17429145.2017.1414321
Abedin, M. A., Habiba, U., and Shaw, R. (2014). Salinity Scenario in Mekong, Ganges, and Indus River Deltas Water Insecurity: A Social Dilemma. Bingley: Emerald Group Publishing Limited, 115–138. doi: 10.1108/S2040-726220130000013012
Abiala, M. A., Abdelrahman, M., Burritt, D. J., and Tran, L.-S. P. (2018). Salt stress tolerance mechanisms and potential applications of legumes for sustainable reclamation of salt-degraded soils. Land Degrad. Dev. 29, 3812–3822. doi: 10.1002/ldr.3095
Ahmad, M., Zahir, Z. A., Asghar, H. N., and Asghar, M. (2011). Inducing salt tolerance in mung bean through coinoculation with rhizobia and plant-growth-promoting rhizobacteria containing 1-aminocyclopropane-1-carboxylate deaminase. Can. J. Microbiol. 57, 578–589. doi: 10.1139/W11-044
Ahmad, M., Zahir, Z. A., Khalid, M., Nazli, F., and Arshad, M. (2013). Efficacy of Rhizobium and Pseudomonas strains to improve physiology, ionic balance and quality of mung bean under salt-affected conditions on farmer’s fields. Plant Physiol. Bioch. 63, 170–176. doi: 10.1016/j.plaphy.2012.11.024
Ahmad, P. (2014). Oxidative Damage to Plants: Antioxidant Networks and Signaling. Cambridge, MA: Academic Press.
Akram, M. S., Shahid, M., Tariq, M., Azeem, M., Javed, M. T., Saleem, S., et al. (2016). Deciphering Staphylococcus sciuri SAT-17 mediated anti-oxidative defense mechanisms and growth modulations in salt stressed maize (Zea mays L.). Front. Microbiol. 7:867. doi: 10.3389/fmicb.2016.00867
Ali, S., Charles, T. C., and Glick, B. R. (2014). Amelioration of high salinity stress damage by plant growth-promoting bacterial endophytes that contain ACC deaminase. Plant Physiol. Biochem. 80, 160–167. doi: 10.1016/j.plaphy.2014.04.003
Ali Khan, M., Shahid Shaukat, S., Shahzad, A., and Arif, H. (2012). Growth and yield responses of pearl millet (Pennisetum glaucum [L.] R.Br.) irrigated with treated effluent from waste stabilization ponds. Pak. J. Bot. 46, 1011–1018.
Amaresan, N., Kumar, K., Madhuri, K., and Usharani, G. K. (2016). Isolation and characterization of salt tolerant plant growth promoting rhizobacteria from plants grown in tsunami affected regions of Andaman and Nicobar Islands. Geomicrobiol. J. 33, 942–947. doi: 10.1080/01490451.2015.1128994
Ambrosini, A., de Souza, R., and Passaglia, L. M. P. (2016). Ecological role of bacterial inoculants and their potential impact on soil microbial diversity. Plant Soil. 400, 193–207. doi: 10.1007/s11104-015-2727-7
Amirul Alam, M., Juraimi, A. S., Rafii, M. Y., Hamid, A. A., Aslani, F., and Alam, M. Z. (2015). Effects of salinity and salinity-induced augmented bioactive compounds in purslane (Portulaca oleracea L.) for possible economical use. Food Chem. 169, 439–447. doi: 10.1016/j.foodchem.2014.08.019
Ansari, F. A., Ahmad, I., and Pichtel, J. (2019). Growth stimulation and alleviation of salinity stress to wheat by the biofilm forming Bacillus pumilus strain FAB10. Appl. Soil Ecol. 3, 45–54. doi: 10.1016/j.apsoil.2019.05.023
Arora, N. K. (2019). Impact of climate change on agriculture production and its sustainable solutions. Environmental Sust. 2, 95–96. doi: 10.1007/s42398-019-00078-w
Arora, N. K., Fatima, T., Mishra, I., Verma, M., Mishra, J., and Mishra, V. (2018). Environmental sustainability: challenges and viable solutions. Environ. Sust. 1, 309–340. doi: 10.1007/s42398-018-00038-w
Arora, N. K., and Mishra, J. (2016). Prospecting the roles of metabolites and additives in future bioformulations for sustainable agriculture. Appl. Soil Ecol. 107, 405–407. doi: 10.1016/j.apsoil.2016.05.020
Ashraf, M., Hasnain, S., Berge, O., and Mahmood, T. (2004). Inoculating wheat seedlings with exopolysaccharide-producing bacteria restricts sodium uptake and stimulates plant growth under salt stress. Biol. Fert. Soils 40, 157–162.
Aslam, F., and Ali, B. (2018). Halotolerant bacterial diversity associated with Suaeda fruticosa (L.) forssk. improved growth of maize under salinity stress. Agronomy 8:131. doi: 10.3390/agronomy8080131
Atouei, M. T., Pourbabaee, A. A., and Shorafa, M. (2019). Alleviation of salinity stress on some growth parameters of wheat by exopolysaccharide-producing bacteria. Ir. J. Sci. Technol. Trans. A 43, 2725–2733. doi: 10.1007/s40995-019-00753-x
Ayyam, V., Palanivel, S., and Chandrakasan, S. (2019). “Approaches in land degradation management for productivity enhancement,” in Coastal Ecosystems of the Tropics – Adaptive Management, eds V. Ayyam, S. Palanivel, and S. Chandrakasan (Singapore: Springer).
Bal, H. B., Nayak, L., Das, S., and Adhya, T. K. (2013). Isolation of ACC deaminase producing PGPR from rice rhizosphere and evaluating their plant growth promoting activity under salt stress. Plant Soil 366, 93–105. doi: 10.1007/s11104-012-1402-5
Bano, A., and Fatima, M. (2009). Salt tolerance in Zea mays (L). following inoculation with Rhizobium and Pseudomonas. Biol. Fertil. Soils 45, 405–413. doi: 10.1007/s00374-008-0344-9
Bano, D. A., Singh, R. K., Waza, S. A., and Singh, N. P. (2015). Effect of cowpea Bradyrhizobium (RA-5) and Burkholderia cepacia (RRE-5) on growth parameters of pigeonpea under salt stress conditions. J. Pure Appl. Microbio. 9, 2539–2546.
Barnawal, D., Bharti, N., Maji, D., Chanotiya, C. S., and Kalra, A. (2014). ACC deaminase-containing Arthrobacter protophormiae induces NaCl stress tolerance through reduced ACC oxidase activity and ethylene production resulting in improved nodulation and mycorrhization in Pisum sativum. J. Plant Physiol. 171, 884–894. doi: 10.1016/j.jplph.2014.03.007
Bashan, Y., and Holguin, G. (1997). Azospirillum – plant relationships: environmental and physiological advances (1990–1996). Can. J. Microbiol. 43, 103–121. doi: 10.1139/m97-015
Bashan, Y., Holguin, G., and de-Bashan, L. E. (2004). Azospirillum-plant relationships: physiological, molecular, agricultural, and environmental advances (1997-2003). Can. J. Microbiol. 50, 521–577. doi: 10.1139/w04-035
Bhattacharyya, P. N., and Jha, D. K. (2012). Plant growth-promoting rhizobacteria (PGPR): emergence in agriculture. World J. Microbiol. Biotechnol. 28, 1327–1350. doi: 10.1007/s11274-011-0979-9
Bomfeti, C. A., Florentino, A. L., Guimarães, A. P., and Cardoso, P. (2011). Exopolysaccharides produced by the symbiotic nitrogen-fixing bacteria of leguminosae. RevBras Ciênc Solo 35, 657–671. doi: 10.1590/s0100-06832011000300001
Bottini, R., Cassán, F., and Piccoli, P. (2004). Gibberellin production by bacteria and its involvement in plant growth promotion and yield increase. Appl. Microbiol. Biotechnol. 65, 497–503.
Bremer, E., and Kramer, R. (2019). Responses of microorganisms to osmotic stress. Annu. Rev. Microbiol. 73, 313–334. doi: 10.1146/annurev-micro-020518-115504
Chanratana, M., Joe, M. M., Choudhury, A. R., Anandham, R., Krishnamoorthy, R., Kim, K., et al. (2019). Physiological response of tomato plant to chitosan-immobilized aggregated Methylobacterium oryzae CBMB20 inoculation under salinity stress. Biotechnolgy 9:397. doi: 10.1007/s13205-019-1923-1
Chaudhary, D., and Sindhu, S. S. (2015). Inducing salinity tolerance in chickpea (Cicer arietinum L.) by inoculation of 1-aminocyclopropane-1-carboxylic acid deaminase-containing Mesorhizobium strains. Afr. J. Microbiol. Res. 9, 117–124. doi: 10.5897/ajmr2014.7087
Chen, L., Liu, Y., Wu, G., Veronican Njeri, K., Shen, Q., Zhang, N., et al. (2016). Induced maize salt tolerance by rhizosphere inoculation of Bacillus amyloliquefaciens SQR9. Physiol. Plant 158, 34–44. doi: 10.1111/ppl.12441
Cheng, Z., Woody, O. Z., Mcconkey, B. J., and Glick, B. R. (2012). Combined effects of the plant growth-promoting bacterium Pseudomonas putida UW4 and salinity stress on the Brassica napus proteome. Appl. Soil Ecol. 61, 255–263. doi: 10.1016/j.apsoil.2011.10.006
Chu, T. N., Tran, B. T. H., Van Bui, L., and Hoang, M. T. T. (2019). Plant growth-promoting rhizobacterium Pseudomonas PS01 induces salt tolerance in Arabidopsis thaliana. BMC Res. Notes 12:11. doi: 10.1186/s13104-019-4046-1
Damodaran, T., Mishra, V. K., Jha, S. K., Pankaj, U., Gupta, G., and Gopal, R. (2019). Identification of rhizosphere bacterial diversity with promising salt tolerance, PGP traits and their exploitation for seed germination enhancement in sodic soil. J. Agric. Res. 8, 36–43. doi: 10.1007/s40003-018-0343-5
Dardanelli, M. S., Fernández de Córdoba, F. J., Espuny, M. R., Rodríguez Carvajal, M. A., Soria Díaz, M. E., Gil Serrano, A. M., et al. (2008). Effect of Azospirillum brasilense coinoculated with Rhizobium on Phaseolus vulgaris flavonoids and Nod factor production under salt stress. Soil Biol. Biochem. 40, 2713–2721. doi: 10.1016/j.soilbio.2008.06.016
Dobbelaere, S., Croonenborghs, A., Thys, A., Ptacek, D., Vanderleyden, J., Dutto, P., et al. (2001). Responses of agronomically important crops to inoculation with emph type=”2Azospirillum</emph>. Funct. Plant Biol. 28, 871–879. doi: 10.1071/PP01074
Dodd, I. C., Zinovkina, N. Y., Safronova, V. I., and Belimov, A. A. (2010). Rhizobacterial mediation of plant hormone status. Ann Appl. Biol. 157, 361–379. doi: 10.3389/fpls.2019.01368
Dove, A. (2017). Central California is Losing $3.7 Billion in Crop Yield Every Year. Report from Department of Civil and Environmental Engineering. Pittsburgh, PA: Carnegie Mellon University.
Egamberdieva, D. (2011). Survival of Pseudomonas extremorientalis TSAU20 and P. chlororaphis TSAU13 in the rhizosphere of common bean (Phaseolus vulgaris) under saline conditions. Plant Soil Environ. 57, 122–127. doi: 10.17221/316/2010-pse
Egamberdieva, D., Jabborova, D., and Berg, G. (2016). Synergistic interactions between Bradyrhizobium japonicum and the endophyte Stenotrophomonas rhizophila and their effects on growth, and nodulation of soybean under salt stress. Plant Soil 405, 35–45. doi: 10.1007/s11104-015-2661-8
Egamberdieva, D., Jabborova, D., and Hashem, A. (2015). Pseudomonas induces salinity tolerance in cotton (Gossypium hirsutum) and resistance to Fusarium root rot through the modulation of indole-3-acetic acid. Saudi J. Biol. Sci. 22, 773–779. doi: 10.1016/j.sjbs.2015.04.019
Egamberdieva, D., Jabborova, D., Wirth, S., Alam, P., Alyemeni, M. N., and Ahmad, P. (2018). Interactive effects of nutrients and Bradyrhizobium japonicum on the growth and root architecture of soybean (Glycine max L.). Front. Microbiol. 9:1000. doi: 10.3389/fmicb.2018.01000
Egamberdieva, D., Kamilova, F., Validov, S., Gafurova, L., Kucharova, Z., and Lugtenberg, B. (2008). High incidence of plant growth-stimulating bacteria associated with the rhizosphere of wheat grown on salinated soil in Uzbekistan. Environ. Microbiol. 10, 1–9. doi: 10.1111/j.1462-2920.2007.01424.x
Egamberdieva, D., and Kucharova, Z. (2009). Selection for root colonising bacteria stimulating wheat growth in saline soils. Biol. Fertil. Soils 45, 563–571. doi: 10.1007/s00374-009-0366-y
Egamberdieva, D., Kucharova, Z., Davranov, K., Berg, G., Makarova, N., Azarova, T., et al. (2011). Bacteria able to control foot and root rot and to promote growth of cucumber in salinated soils. Biol. Fertil. Soils 47, 197–205. doi: 10.1007/s00374-010-0523-3
Egamberdieva, D., Shurigin, V., Gopalakrishnan, S., and Sharma, R. (2014). Growth and symbiotic performance of chickpea (Cicer arietinum) cultivars under saline soil conditions. J. Biol. Chem. Res. 56, 1–10.
Egamberdieva, D., Wirth, S., Jabborova, D., Räsänen, L. A., and Liao, H. (2017a). Coordination between Bradyrhizobium and Pseudomonas alleviates salt stress in soybean through altering root system architecture. J. Plant Interact. 12, 100–107. doi: 10.1080/17429145.2017.1294212
Egamberdieva, D., Wirth, S., Shurigin, V., Hashem, A., and AbdAllah, E. F. (2017b). Endophytic bacteria improve plant growth, symbiotic performance of chickpea (Cicer arietinum L.) and induce suppression of root rot caused by Fusarium solani under salt stress. Front. Microbiol. 28:1887. doi: 10.3389/fmicb.2017.01887
El-Esawi, M. A., Alaraidh, I. A., Alsahli, A. A., Alamri, S. A., Ali, H. M., and Alayafi, A. A. (2018a). Bacillus firmus (SW5) augments salt tolerance in soybean (Glycine max L.) by modulating root system architecture, antioxidant defense systems and stress-responsive genes expression. Plant Physiol. Biochem. 132, 375–384. doi: 10.1016/j.plaphy.2018.09.026
El-Esawi, M. A., Alaraidh, I. A., Alsahli, A. A., Alzahrani, S. A., Ali, H. M., Alayafi, A. A., et al. (2018b). Serratia liquefaciens KM4 improves salt stress tolerance in maize by regulating redox potential, ion homeostasis, leaf gas exchange and stress-related gene expression. Int. J. Mol. Sci. 19:3310. doi: 10.3390/ijms19113310
El-Esawi, M. A., Al-Ghamdi, A. A., Ali, H. M., and Alayafi, A. A. (2019). Azospirillum lipoferum FK1 confers improved salt tolerance in chickpea (Cicer arietinumL.) by modulating osmolytes, antioxidant machinery and stress-related genes expression. Environ. Exp. Bot. 159, 55–65. doi: 10.1016/j.envexpbot.2018.12.001
FAO and ITPS (2015). Status of the World’s Soil Resources (SWSR) – Main Report. Rome: Food and Agriculture Organization of the United Nations and Intergovernmental Technical Panel on Soils.
Faravani, M., Emami, S. D., Gholami, B. A., and Faravani, A. (2013). The effect of salinity on germination, emergence, seed yield and biomass of black cumin. J. Agric. Sci. 58, 41–49. doi: 10.2298/JAS1301041F
Farooq, M., Gogoi, N., Hussain, M., Barthakur, S., Paul, S., Bharadwaj, N., et al. (2017). Effects, tolerance mechanisms and management of salt stress in grain legumes. Plant Physiol. Biochem. 118, 199–217. doi: 10.1016/j.plaphy.2017.06.020
Fita, A., Rodriíguez-Burruezo, A., Boscaiu, M., Prohens, J., and Vicente, O. (2015). Breeding and domesticating crops adapted to drought and salinity: a new paradigm for increasing food production. Front. Plant Sci. 6:978. doi: 10.3389/fpls.2015
Forchetti, G., Masciarelli, O., Alemano, S., Alvarez, D., and Abdala, G. (2007). Endophytic bacteria in sunflower (Helianthus annuus L.): isolation, characterization, and production of jasmonates and abscisic acid in culture medium. Appl. Microbiol. Biotechnol. 76, 1145–1152. doi: 10.1007/s00253-007-1077-7
Fukami, J., de la Osa, C., Ollero, F. J., Megías, M., and Hungria, M. (2018). Co-inoculation of maize with Azospirillum brasilense and Rhizobium tropici as a strategy to mitigate salinity stress. Funct. Plant Biol. 45, 328–339.
GAP Report (2018). Global Agricultural Productivity Report§(GAP Report§) Global Harvest Initiative, Washington. Available at: https://globalagriculturalproductivity.org/wp-content/uploads/2019/01/GHI_2018-GAP-Report_FINAL-10.03.pdf (assessed April 15, 2019).
García de Salamone, I. E., Hynes, R. K., and Nelson, L. M. (2001). Cytokinin production by plant growth promoting rhizobacteria and selected mutants. Can. J. Microbiol. 47, 404–411. doi: 10.1139/w01-029
Ge, H., and Zhang, F. (2019). Growth-Promoting Ability of Rhodopseudomonas palustris G5 and its effect on induced resistance in cucumber against salt stress. J. Plant Growth Regul. 38, 180–188. doi: 10.1007/s00344-018-9825-8
Ghanem, M. E., van Elteren, J., Albacete, A., Quinet, M., Martínez-Andújar, C., Kinet, J. M., et al. (2009). Impact of salinity on early reproductive physiology of tomato (Solanum lycopersicum) in relation to a heterogeneous distribution of toxic ions in flower organs. Funct. Plant. Biol. 36, 125–136.
Ghassemi, F., Jakeman, A. J., and Nix, H. A. (1995). Salinisation of Land and Water Resources: Human Causes, Extent, Management and Case Studies. Wallingford: CAB international.
Ghorai, S., Pal, K. K., and Dey, R. (2015). Alleviation of salinity stress in groundnut by application of PGPR. Int. J. Res. Eng. Technol. 2, 742–750.
Glick, B. R. (2014). Bacteria with ACC deaminase can promote plant growth and help to feed the world. Microbiol. Res. 169, 30–39. doi: 10.1016/j.micres.2013.09.009
Glick, B. R., Todorovic, B., Czarny, J., Cheng, Z., Duan, J., and McConkey, B. (2007). Promotion of plant growth by bacterial ACC deaminase. Crit. Rev. Plant. Sci. 26, 227–242. doi: 10.1080/07352680701572966
Gond, S. K., Torres, M. S., Bergen, M. S., Helsel, Z., and White, J. F. Jr. (2015). Induction of salt tolerance and up-regulation of aquaporin genes in tropical corn by rhizobacterium Pantoea agglomerans. Lett. Appl. Microbiol. 60, 392–399. doi: 10.1111/lam.12385
Graham, P. H. (1992). Stress tolerance in Rhizobium and Bradyrhizobium, and nodulation under adverse soil conditions. Can. J. Microbiol. 38, 475–484. doi: 10.1139/m92-079
Grover, M., Ali, S. Z., Sandhya, V., Rasul, A., and Venkateswarlu, B. (2011). Role of microorganisms in adaptation of agriculture crops to abiotic stresses. World J. Microbiol. Biotechnol. 27, 1231–1240. doi: 10.1007/s00425-015-2435-9
Habib, S. H., Kausar, H., and Halimi, M. (2016a). Plant growth-promoting rhizobacteria enhance salinity stress tolerance in okra through ROS-scavenging enzymes. Biomed. Res. Int. 2016:6284547. doi: 10.1155/2016/6284547
Habib, S. H., Kausar, H., Saud, H. M., Ismail, M. R., and Othman, R. (2016b). Molecular characterization of stress tolerant plant growth promoting rhizobacteria (PGPR) for growth enhancement of rice. Int. J. Agric. Biol. 18, 184–191. doi: 10.17957/ijab/15.0094
Halder, A., Banerjee, J., Bhattacharyya, P., Pramanik, K., and Debnath, A. (2016). Isolation of lentil-specific salt tolerant nitrogen fixing bacteria from Murshidabad district of West Bengal. J. Crop Weed. 12, 14–19.
Hamdia, M. A. E. S., Shaddad, M. A. K., and Doaa, M. M. (2004). Mechanisms of salt tolerance and interactive effects of Azospirillum brasilense inoculation on maize cultivars grown under salt stress conditions. Plant Growth Regul. 44, 165–174. doi: 10.1023/b:grow.0000049414.03099.9b
Han, H. S., and Lee, K. D. (2005). Physiological responses of soybean-inoculation of Bradyrhizobium japonicum with PGPR in saline soil conditions. Res. J. Agric. Biol. Sci. 1, 216–221.
Hashem, A., Abd_Allah, E. F., Alqarawi, A. A., Al-Huqail, A. A., Wirth, S., and Egamberdieva, D. (2016). The interaction between arbuscular mycorrhizal fungi and endophytic bacteria enhances plant growth of Acacia gerrardii under salt stress. Front. Microbiol. 7:1089. doi: 10.3389/fmicb.2016.01089
He, A. L., Niu, S. Q., Zhao, Q., Li, Y. S., Gou, J. Y., Gao, H. J., et al. (2018). Induced salt tolerance of perennial ryegrass by a novel bacterium strain from the rhizosphere of a desert shrub Haloxylon ammodendron. Int. J. Mol. Sci. 19:469. doi: 10.3390/ijms19020469
Herger, G., Nielsen, R., and Margheim, J. (2015). Fertilizer History P3: in WWII Nitrogen Production Issues in Age of Modern Fertilizers. Available at: http://cropwatch.unl.edu/fertilizer-history-p3 (accessed April 10, 2015).
Hidri, I. R., Barea, J. M., Mahmoud, M. B., and Azcon, A. R. (2016). Impact of microbial inoculation on biomass accumulation by Sulla carnosa provenances, and in regulating nutrition, physiological and antioxidant activities of this species under non-saline and saline conditions. J. Plant Physiol. 201, 28–41. doi: 10.1016/j.jplph.2016.06.013
Ilangumaran, G., and Smith, D. L. (2017). Plant growth promoting rhizobacteria in amelioration of Salinity stress: a systems biology perspective. Front. Plant Sci. 8:1768. doi: 10.3389/fpls.2017.01768
Ilyas, F. (2017). Sindh Suffers 31pc Crop Loss Annually Due to Waterlogging, Salinity. Dawn. Available at: https://www.dawn.com/news/1357033 (assessed October 12, 2019).
Irizarry, I., and White, J. (2017). Application of bacteria from non-cultivated plants to promote growth, alter root architecture and alleviate salt stress of cotton. J. Appl. Microbiol. 122, 1110–1120. doi: 10.1111/jam.13414
Islam, F., Yasmeen, T., Arif, M. S., Ali, S., Ali, B., Hameed, S., et al. (2016). Plant growth promoting bacteria confer salt tolerance in Vigna radiata by up-regulating antioxidant defense and biological soil fertility. Plant Growth Regul. 80, 23–36. doi: 10.1007/s10725-015-0142-y
IUSS Working Group WRB (2015). World Reference Base for Soil Resources 2014, update 2015 International Soil Classification System for Naming Soils and Creating Legends for Soil Maps. World Soil Resources Reports No. 106, Rome: FAO.
Jalili, F., Khavazi, K., Pazira, E., Nejati, A., Rahmani, H. A., Sadaghiani, H. R., et al. (2009). Isolation and characterization of ACC deaminase-producing fluorescent Pseudomonads, to alleviate salinity stress on canola (Brassica napus L.) growth. J. Plant Physiol. 166, 667–674. doi: 10.1016/j.jplph.2008.08.004
Jatan, R., Chauhan, P. S., and Lata, C. (2019). Pseudomonas putida modulates the expression of miRNAs and their target genes in response to drought and salt stresses in chickpea (Cicer arietinum L.). Genomics 111, 509–519. doi: 10.1016/j.ygeno.2018.01.007
Jha, Y., and Subramanian, R. B. (2013). Paddy plants inoculated with PGPR show better growth physiology and nutrient content under saline condition. Chil. J. Agr. Res. 73, 213–219. doi: 10.4067/s0718-58392013000300002
Ji, H., Pardo, J. M., Batelli, G., Van Oosten, M. J., Bressan, R. A., and Li, X. (2013). The Salt Overly Sensitive (SOS) pathway: established and emerging roles. Mol. Plant 6, 275–286. doi: 10.1093/mp/sst017
Jida, M., and Assefa, F. (2011). Phenotypic and plant growth promoting characteristics of Rhizobium leguminosarum bv. viciae from lentil growing areas of Ethiopia. Afr. J. Microbiol. Res. 5, 4133–4142.
Kang, S. M., Radhakrishnan, R., Khan, A. L., Kim, M. J., Park, J. M., Kim, B. R., et al. (2014). Gibberellin secreting rhizobacterium, Pseudomonas putida H-2-3 modulates the hormonal and stress physiology of soybean to improve the plant growth under saline and drought conditions. Plant Physiol. Biochem. 84, 115–124. doi: 10.1016/j.plaphy.2014.09.001
Kang, S. M., Shahzad, R., Bilal, S., Khan, A. L., Park, Y. G., Lee, K. E., et al. (2019). Indole-3-acetic-acid and ACC deaminase producing Leclercia adecarboxylata MO1 improves Solanum lycopersicum L. growth and salinity stress tolerance by endogenous secondary metabolites regulation. BMC Microbiol. 19:80. doi: 10.1186/s12866-019-1450-6
Karadeniz, A., Topcuoğlu, Ş. F., and Inan, S. (2006). Auxin, gibberellin, cytokinin and abscisic acid production in some bacteria. World J. Microbiol. Biotechnol. 22, 1061–1064. doi: 10.1007/s11274-005-4561-1
Keren, R. (2005). “Salt-affected soils, reclamation,” in Encyclopedia of Soils in the Environment, ed. D. Hillel (Oxford: Elsevier), 454–461. doi: 10.1016/b0-12-348530-4/00503-8
Khan, A., Zhao, X. Q., Javed, M. T., Khan, K. S., Bano, A., Shen, R. F., et al. (2016). Bacillus pumilus enhances tolerance in rice (Oryza sativa L.) to combined stresses of NaCl and high boron due to limited uptake of Na+. Environ. Exp. Bot. 124, 120–129. doi: 10.1016/j.envexpbot.2015.12.011
Khan, N., and Bano, A. (2019). Exopolysaccharide producing rhizobacteria and their impact on growth and drought tolerance of wheat grown under rainfed conditions. PLoS One 14:e0222302. doi: 10.1371/journal.pone.0222302
Kim, J., Geng, R., Gallenstein, R. A., and Somers, D. E. (2013). The F-box protein ZEITLUPE controls stability and nucleocytoplasmic partitioning of GIGANTEA. Development 140, 4060–4069. doi: 10.1242/dev.096651
Kim, K., Samaddar, S., Chatterjee, P., Krishnamoorthy, R., Jeon, S., and Sa, T. (2019). Structural and functional responses of microbial community with respect to salinity levels in a coastal reclamation land. Appl. Soil Ecol. 137, 96–105. doi: 10.1016/j.apsoil.2019.02.011
Kohler, J., Caravaca, F., Carrasco, L., and Roldan, A. (2006). Contribution of Pseudomonas mendocina and Glomus intraradices to aggregate stabilization and promotion of biological fertility in rhizosphere soil of lettuce plants under field conditions. Soil Use Manag. 22, 298–304. doi: 10.1111/j.1475-2743.2006.00041.x
Kohler, J., Hernández, J. A., Caravaca, F., and Roldán, A. (2009). Induction of antioxidant enzymes is involved in the greater effectiveness of a PGPR versus AM fungi with respect to increasing the tolerance of lettuce to severe salt stress. Environ. Exp. Bot. 65, 245–252. doi: 10.1016/j.envexpbot.2008.09.008
Kothari, V. V., Kothari, R. K., Kothari, C. R., Bhatt, V. D., Nathani, N. M., Koringa, P. G., et al. (2013). Genome sequence of salt-tolerant Bacillus safensis strain VK, isolated from Saline Desert Area of Gujarat, India. Genome Announc. 1:e671-13. doi: 10.1128/genomeA.00671-13
Kumar, A., Patel, J. S., Meena, V. S., and Srivastava, R. (2019). Recent advances of PGPR based approaches for stress tolerance in plants for sustainable agriculture. Biocatal. Agric. Biotechnol. 20:101271. doi: 10.1016/j.bcab.2019.101271
Kumar, H., Arora, N. K., Kumar, V., and Maheshwari, D. K. (1999). Isolation, characterization and selection of salt-tolerant rhizobia nodulating Acacia catechu and Acacia nilotica. Symbiosis 26, 279–288.
Kushwaha, B., Jadhav, I., Verma, H. N., Geethadevi, A., Parashar, D., and Jadhav, K. (2019). Betaine accumulation suppresses the de-novo synthesis of ectoine at a low osmotic concentration in Halomonas sp. SBS 10, a bacterium with broad salinity tolerance. Mol. Biol. Rep. 46, 4779–4786. doi: 10.1007/s11033-019-04924-2
Läuchli, A., and Grattan, S. R. (2007). “Plant growth and development under salinity stress,” In Advances in Molecular Breeding Toward Drought and Salt Tolerant Crops, eds M. A. Jenks, P. M. Hasegawa, and S. M. Jain (Dordrecht: Springer), 1–32. doi: 10.1007/978-1-4020-5578-2_1
Li, H. Q., and Jiang, X. W. (2017). Inoculation with plant growth-promoting bacteria (PGPB) improves salt tolerance of maize seedling. Russ. J. Plant Physl. 64, 235–241. doi: 10.1134/s1021443717020078
Li, Y., Shi, H., Zhang, H., and Chen, S. (2019). Amelioration of drought effects in wheat and cucumber by the combined application of super absorbent polymer and potential biofertilizer. Peer J. 7, e6073. doi: 10.7717/peerj.6073
Liu, W., Wang, Q., Hou, J., Tu, C., Luo, Y., and Christie, P. (2016). Whole genome analysis of halotolerant and alkalotolerant plant growth-promoting rhizobacterium Klebsiella sp. D5A. Sci. Rep. 6:26710. doi: 10.1038/srep26710
Ma, Y., Vosátka, M., and Freitas, H. (2019). Editorial: beneficial microbes alleviate climatic stresses in plants. Front. Plant Sci. 10:595.
Mahmoodzadeh, H., and Bemani, M. (2008). Influence of salinity at early stage of flowering on the development of male gametophyte in Canola (Brassica napus L.) cv. Symbol. Res. J. Environ. Sci. 2, 415–423. doi: 10.3923/rjes.2008.415.423
Manaf, H. H., and Zayed, M. S. (2015). Productivity of cowpea as affected by salt stress in presence of endomycorrhizae and Pseudomonas fluorescens. Ann. Agri. Sci. 60, 219–226. doi: 10.1016/j.aoas.2015.10.013
Manchanda, G., and Garg, N. (2008). Salinity and its effects on the functional biology of legumes. Acta. Physiologiae Plantarum. 30, 595–618. doi: 10.1007/s11738-008-0173-3
Mayak, S., Tirosh, T., and Glick, B. R. (2004). Plant growth-promoting bacteria confer resistance in tomato plants to salt stress. Plant Physiol. Biochem. 42, 565–572. doi: 10.1016/j.plaphy.2004.05.009
Meena, K. K., Sorty, A. M., Bitla, U. M., Choudhary, K., Gupta, P., Pareek, A., et al. (2017). Abiotic stress responses and microbe-mediated mitigation in plants: the omics strategies. Front. Plant Sci. 8:172. doi: 10.3389/fpls.2017.00172
Metwali, E. M., Abdelmoneim, T. S., Bakheit, M. A., and Kadasa, N. (2015). Alleviation of salinity stress in faba bean (Vicia faba L.) plants by inoculation with plant growth promoting rhizobacteria (PGPR). Plant Omics 8:449.
Mishra, J., Fatima, T., and Arora, N. K. (2018). “Role of secondary metabolites from plant growth-promoting rhizobacteria in combating salinity stress,” in Plant Microbiome: Stress Response, eds P. Ahmad, and D. Egamberdieva (Singapore: Springer), 127–163. doi: 10.1007/978-981-10-5514-0_6
Misra, S., Dixit, V. K., Khan, M. H., Kumar Mishra, S., Dviwedi, G., Yadav, S., et al. (2017). Exploitation of agro-climatic environment for selection of 1-aminocyclopropane-1-carboxylic acid (ACC) deaminase producing salt tolerant indigenous plant growth promoting rhizobacteria. Microbiol. Res. 205, 25–34. doi: 10.1016/j.micres.2017.08.007
Mohammed, A. F. (2018). Effectiveness of exopolysaccharides and biofilm forming plant growth promoting rhizobacteria on salinity tolerance of faba bean (Vicia faba L.). Afri. J. Microbiol. Res. 12, 399–404. doi: 10.5897/ajmr2018.8822
Morton, M. J., Awlia, M., Al-Tamimi, N., Saade, S., Pailles, Y., Negrão, S., et al. (2019). Salt stress under the scalpel–dissecting the genetics of salt tolerance. Plant J. 97, 148–163. doi: 10.1111/tpj.14189
Nadeem, S. M., Zahir, Z. A., Naveed, M., and Arshad, M. (2007). Preliminary investigations on inducing salt tolerance in maize through inoculation with rhizobacteria containing ACC deaminase activity. Can. J. Microbiol. 53, 1141–1149. doi: 10.1139/w07-081
Nadeem, S. M., Zahir, Z. A., Naveed, M., and Arshad, M. (2009). Rhizobacteria containing ACC-deaminase confer salt tolerance in maize grown on salt-affected fields. Can. J. Microbiol. 55, 1302–1309. doi: 10.1139/w09-092
Nakbanpote, W., Panitlurtumpai, N., Sangdee, A., Sakulpone, N., Sirisom, P., and Pimthong, A. (2014). Salt-tolerant and plant growth-promoting bacteria isolated from Zn/Cd contaminated soil: identification and effect on rice under saline conditions. J. Plant Interact. 9, 379–387. doi: 10.1080/17429145.2013.842000
Nascimento, F. X., Brígido, C., Glick, B. R., and Rossi, M. J. (2016). The role of rhizobial ACC deaminase in the nodulation process of leguminous plants. Int. J. Agro. 2016:1369472.
Naseem, H., and Bano, A. (2014). Role of plant growth-promoting rhizobacteria and their exopolysaccharide in drought tolerance of maize. J. Plant Interact. 9, 689–701. doi: 10.1080/17429145.2014.902125
Nautiyal, C. S., Srivastava, S., Chauhan, P. S., Seem, K., Mishra, A., and Sopory, S. K. (2013). Plant growth-promoting bacteria Bacillus amyloliquefaciens NBRISN13 modulates gene expression profile of leaf and rhizosphere community in rice during salt stress. Plant Physiol. Biochem. 66, 1–9. doi: 10.1016/j.plaphy.2013.01.020
Naz, I., Bano, A., and Ul-Hassan, T. (2009). Isolation of phytohormones producing plant growth promoting rhizobacteria from weeds growing in Khewra salt range, Pakistan and their implication in providing salt tolerance to Glycine max L. Afr. J. Biotechnol. 8, 5762–5766.
Nemecek, T., and Gaillard, G. (2010). “Challenges in assessing the environmental impacts of crop production and horticulture,” in Environmental Assessment and Management in the Food Industry, eds U. Sonesson, J. Berlin, and F. Ziegler (Sawston: Woodhead Publishing), 98–116. doi: 10.1533/9780857090225.2.98
Niu, X., Song, L., Xiao, Y., and Ge, W. (2018). Drought-tolerant plant growth-promoting rhizobacteria associated with foxtail millet in a semi-arid agroecosystem and their potential in alleviating drought stress. Front. Microbiol. 8:2580. doi: 10.3389/fmicb.2017.02580
Orozco-Mosqueda, M. D. C., Duan, J., DiBernardo, M., Zetter, E., Campos-García, J., Glick, B. R., et al. (2019). The Production of ACC deaminase and trehalose by the plant growth promoting bacterium Pseudomonas sp. UW4 synergistically protect tomato plants against salt stress. Front. Microbiol. 10:1392. doi: 10.3389/fmicb.2019.01392
Palacio-Rodríguez, R., Coria-Arellano, J. L., López-Bucio, J., Sánchez-Salas, J., Muro-Pérez, G., Castañeda-Gaytán, G., et al. (2017). Halophilic rhizobacteria from Distichlis spicata promote growth and improve salt tolerance in heterologous plant hosts. Symbiosis 73, 179–189. doi: 10.1007/s13199-017-0481-8
Pan, J., Peng, F., Xue, X., You, Q., Zhang, W., Wang, T., et al. (2019). The growth promotion of two salt-tolerant plant groups with PGPR inoculation: a meta-analysis. Sustain 11:378. doi: 10.3390/su11020378
Park, H. J., Kim, W. Y., and Yun, D. J. (2013). A role for GIGANTEA: keeping the balance between flowering and salinity stress tolerance. Plant Signal. Behav. 8:e24820. doi: 10.4161/psb.24820
Park, H. J., Kim, W.-Y., and Yun, D.-J. (2016). A new insight of salt stress signaling in plant. Mol. Cells 39, 447–459. doi: 10.14348/molcells.2016.0083
Patel, D., Jha, C. K., Tank, N., and Saraf, M. (2012). Growth enhancement of chickpea in saline soils using plant growth-promoting rhizobacteria. J. Plant Growth Regul. 31, 53–62. doi: 10.1007/s00344-011-9219-7
Patel, D., and Saraf, M. (2013). Influence of soil ameliorants and microflora on induction of antioxidant enzymes and growth promotion of (Jatropha curcas L.) under saline condition. Eur. J. Soil Biol. 55, 47–54. doi: 10.1016/j.ejsobi.2012.12.004
Patel, T., and Saraf, M. (2017). Biosynthesis of phytohormones from novel rhizobacterial isolates and their in vitro plant growth-promoting efficacy. J. Plant Interact. 12, 480–487. doi: 10.1080/17429145.2017.1392625
Paul, D. (2013). Osmotic stress adaptations in rhizobacteria. J. Basic Microbiol. 53, 101–110. doi: 10.1002/jobm.201100288
Paul, D., and Lade, H. (2014). Plant-growth-promoting rhizobacteria to improve crop growth in saline soils: a review. Agron. Sustain. Dev. 34, 737–752. doi: 10.1007/s13593-014-0233-6
Piccoli, P., Travaglia, C., Cohen, A., Sosa, L., Cornejo, P., Masuelli, R., et al. (2011). An endophytic bacterium isolated from roots of the halophyte Prosopis strombulifera produces ABA, IAA, gibberellins A 1 and A 3 and jasmonic acid in chemically-defined culture medium. Plant Growth Regul. 64, 207–210. doi: 10.1007/s10725-010-9536-z
Promila, K., and Kumar, S. (1982). Effect of salinity on flowering and yield characters in pigeonpea. Ind. J. Plant Physiol. 25, 252–257.
Pushpavalli, R., Quealy, J., Colmer, T., Turner, N., Siddique, K., Rao, M., et al. (2016). Salt stress delayed flowering and reduced reproductive success of chickpea (Cicer arietinum L.), a response associated with Na+ accumulation in leaves. J. Agron. Crop Sci. 202, 125–138. doi: 10.1111/jac.12128
Qadir, M., Quillérou, E., Nangia, V., Murtaza, G., Singh, M., Thomas, R. J., et al. (2014). Economics of salt-induced land degradation and restoration. Nat. Resour. Forum 38, 282–295. doi: 10.1111/1477-8947.12054
Qin, S., Feng, W. W., Zhang, Y. J., Wang, T. T., Xiong, Y. W., and Xing, K. (2018). Diversity of bacterial microbiota of coastal halophyte Limonium sinense and amelioration of salinity stress damage by symbiotic plant growth-promoting actinobacterium Glutamicibacter halophytocola KLBMP 5180. Appl. Environ. Microbiol. 84:e1533-18. doi: 10.1128/AEM.01533-18
Qurashi, A. W., and Sabri, A. N. (2012a). Bacterial exopolysaccharide and biofilm formation stimulate chickpea growth and soil aggregation under salt stress. Braz. J. Microbiol. 43, 1183–1191. doi: 10.1590/s1517-83822012000300046
Qurashi, A. W., and Sabri, A. N. (2012b). Biofilm formation in moderately halophilic bacteria is influenced by varying salinity levels. J. Basic Microbiol. 52, 566–572. doi: 10.1002/jobm.201100253
Raj, S. N., Lavanya, S. N., Sudisha, J., and Shetty, H. S. (2011). “Applications of biopolymers in agriculture with special reference to role of plant derived biopolymers in crop protection,” in Biopolymers: Biomédical and Environmental Applications, eds S. Kalia, and L. Avérous (Hoboken, NJ: Wiley Publishing LLC), 461–481.
Rajendrakumar, C. S. V., Suryanarayana, T., and Reddy, A. R. (1997). DNA helix destabilization by proline and betaine: possible role in the salinity tolerance process. FEBS Lett. 410, 201–205. doi: 10.1016/s0014-5793(97)00588-7
Rajput, L. U. B. N. A., Imran, A., Mubeen, F., and Hafeez, F. Y. (2013). Salt-tolerant PGPR strain Planococcus rifietoensis promotes the growth and yield of wheat (Triticum aestivum L.) cultivated in saline soil. Pak. J. Bot. 45, 1955–1962.
Rengasamy, P. (2010). Soil processes affecting crop production in salt-affected soils. Funct. Plant Biol. 37, 613–620.
Rima, F. S., Biswas, S., Sarker, P. K., Islam, M. R., and Seraj, Z. I. (2018). Bacteria endemic to saline coastal belt and their ability to mitigate the effects of salt stress on rice growth and yields. Ann. Microbiol. 68, 525–535. doi: 10.1007/s13213-018-1358-7
Rodríguez-Salazar, J., Suárez, R., Caballero-Mellado, J., and Iturriaga, G. (2009). Trehalose accumulation in Azospirillum brasilense improves drought tolerance and biomass in maize plants. FEMS Microbiol. Lett. 296, 52–59. doi: 10.1111/j.1574-6968.2009.01614.x
Rojas-Tapias, D., Moreno-Galván, A., Pardo-Díaz, S., Obando, M., Rivera, D., and Bonilla, R. (2012). Effect of inoculation with plant growth-promoting bacteria (PGPB) on amelioration of saline stress in maize (Zea mays). Appl. Soil Ecol. 61, 264–272. doi: 10.1016/j.apsoil.2012.01.006
Roy, S. J., Negrão, S., and Tester, M. (2014). Salt resistant crop plants. Curr. Opin. Biotech. 26, 115–124. doi: 10.1016/j.copbio.2013.12.004
Rütting, T., Aronsson, H., and Delin, S. (2018). Efficient use of nitrogen in agriculture. Nutr. Cycl. Agroecosys. 110, 1–5. doi: 10.1007/s10705-017-9900-8
Ryu, J. Y., Lee, H.-J., Seo, P. J., Jung, J.-H., Ahn, J. H., and Park, C.-M. (2014). The Arabidopsis floral repressor BFT delays flowering by competing with FT for FD binding under high salinity. Mol. Plant 7, 377–387. doi: 10.1093/mp/sst114
Sadeghi, A., Karimi, E., Dahaji, P. A., Javid, M. G., Dalvand, Y., and Askari, H. (2012). Plant growth promoting activity of an auxin and siderophore producing isolate of Streptomyces under saline soil conditions. World J. Microbiol. Biotechnol. 28, 1503–1509. doi: 10.1007/s11274-011-0952-7
Saghafi, D., Ghorbanpour, M., and Lajayer, B. A. (2018). Efficiency of Rhizobium strains as plant growth promoting rhizobacteria on morpho-physiological properties of Brassica napus L. under salinity stress. J. Soil Sci. Plant Nutr. 18, 253–268.
Salomon, M. V., Bottini, R., de Souza Filho, G. A., Cohen, A. C., Moreno, D., Gil, M., et al. (2014). Bacteria isolated from roots and rhizosphere of Vitis vinifera retard water losses, induce abscisic acid accumulation and synthesis of defense-related terpenes in in vitro cultured grapevine. Physiol. Plant 151, 359–374. doi: 10.1111/ppl.12117
Sandhya, V. S. K. Z., Ali, S. Z., Grover, M., Reddy, G., and Venkateswarlu, B. (2010). Effect of plant growth promoting Pseudomonas spp. on compatible solutes, antioxidant status and plant growth of maize under drought stress. Plant Growth Regul. 62, 21–30. doi: 10.1007/s10725-010-9479-4
Sapre, S., Gontia-Mishra, I., and Tiwari, S. (2018). Klebsiella sp. confers enhanced tolerance to salinity and plant growth promotion in oat seedlings (Avena sativa). Microbiol. Res. 206, 25–32. doi: 10.1016/j.micres.2017.09.009
Saravanakumar, D., and Samiyappan, R. (2007). ACC deaminase from Pseudomonas fluorescens mediated saline resistance in groundnut (Arachis hypogea) plants. J Appl. Microbiol. 102, 1283–1292. doi: 10.1111/j.1365-2672.2006.03179.x
Sarkar, A., Ghosh, P. K., Pramanik, K., Mitra, S., Soren, T., Pandey, S., et al. (2018). A halotolerant Enterobacter sp. displaying ACC deaminase activity promotes rice seedling growth under salt stress. Microbiol. Res. 169, 20–32. doi: 10.1016/j.resmic.2017.08.005
Saum, S. H., and Müller, V. (2007). Salinity-dependent switching of osmolyte strategies in a moderately halophilic bacterium: glutamate induces proline biosynthesis in Halobacillus halophilus. J. Bacteriol. 189, 6968–6975. doi: 10.1128/jb.00775-07
Schirawski, J., and Perlin, M. H. (2018). Plant– microbe interaction 2017-the good, the bad and the diverse. Int. J. Mol. Sci. 19:1374. doi: 10.3390/ijms19051374
SDG (2019). The Sustainable Development Goals Report- United Nations, NewYork. Available at: https://unstats.un.org/sdgs/report/2019/The-Sustainable-Development-Goals-Report-2019.pdf (assessed October 12, 2019).
Sen, S., and Chandrasekhar, C. N. (2015). Effect of PGPR on enzymatic activities of rice (Oryza sativa L.) under salt stress. Asian J. Plant Sci. Res. 5, 44–48.
Shahbaz, M., and Ashraf, M. (2013). Improving salinity tolerance in cereals. Crit. Rev. Plant Sci. 32, 237–249. doi: 10.1080/07352689.2013.758544
Shannon, M. C., and Grieve, C. M. (1998). Tolerance of vegetable crops to salinity. Sci. Horticult. 78, 5–38. doi: 10.1016/S0304-4238(98)00189-7
Sharif, R., Mujtaba, M., Ur Rahman, M., Shalmani, A., Ahmad, H., Anwar, T., et al. (2018). The multifunctional role of chitosan in horticultural crops; a review. Molecules 23:872. doi: 10.3390/molecules23040872
Sharma, A., Singh, P., Kumar, S., Kashyap, P. L., Srivastava, A. K., Chakdar, H., et al. (2015). Deciphering diversity of salt-tolerant bacilli from saline soils of eastern indo-gangetic plains of India. Geomicrobiol. J. 32, 170–180. doi: 10.1080/01490451.2014.938205
Sharma, S., Kulkarni, J., and Jha, B. (2016). Halotolerant rhizobacteria promote growth and enhance salinity tolerance in peanut. Front. Microbiol. 7:1600. doi: 10.3389/fmicb.2016.01600
Shilev, S., Sancho, E. D., and Benlloch-González, M. (2012). Rhizospheric bacteria alleviate salt-produced stress in sunflower. J. Environ. Manage. 95, S37–S41. doi: 10.1016/j.jenvman.2010.07.019
Shim, J. S., Seo, J.-S., Seo, J. S., Kim, Y., Koo, Y., Do Choi, Y., et al. (2019). Heterologous expression of bacterial trehalose biosynthetic genes enhances trehalose accumulation in potato plants without adverse growth effects. Plant Biotechnol. Rep. 13, 409–418. doi: 10.1007/s11816-019-00554-z
Shukla, P. S., Agarwal, P. K., and Jha, B. (2012). Improved salinity tolerance of (Arachis hypogaea L.) by the interaction of halotolerant plant-growth-promoting rhizobacteria. J. Plant Growth Regul. 31, 195–206. doi: 10.1007/s00344-011-9231-y
Singh, R. P., and Jha, P. N. (2016). The multifarious PGPR Serratia marcescens CDP-13 augments induced systemic resistance and enhanced salinity tolerance of wheat (Triticum aestivum L.). PLoS One 11:e0155026. doi: 10.1371/journal.pone.0155026
Souza, R. D., Ambrosini, A., and Passaglia, L. M. (2015). Plant growth-promoting bacteria as inoculants in agricultural soils. Genet. Mol. Biol. 38, 401–409. doi: 10.1590/S1415-475738420150053
Sultana, S., Paul, S. C., and Karim, M. M. (2018). Salinity intrusion and coastal agriculture: adaptation strategies using salt-tolerant plant-growth promoting rhizobacteria for sustainable food security. Reg. Probl. 21, 58–61. doi: 10.31433/1605-220x-2018-21-3(1)-58-61
Sun, K., Hunt, K., and Hauser, B. A. (2004). Ovule abortion in arabidopsis triggered by stress. Plant Physiol. 135, 2358–2367. doi: 10.1104/pp.104.043091
Szabo, S., Hossain, M. S., Adger, W. N., Matthews, Z., Ahmed, S., Lázár, A. N., et al. (2016). Soil salinity, household wealth and food insecurity in tropical deltas: evidence from south-west coast of Bangladesh. Sustain. Sci. 11, 411–421. doi: 10.1007/s11625-015-0337-1
Szabolcs, I. (1992). Salinization of soil and water and its relation to desertification. Desertific. Control Bull. 21, 32–37.
Tahir, M., Ahmad, I., Shahid, M., Shah, G. M., Farooq, A. B. U., Akram, M., et al. (2019). Regulation of antioxidant production, ion uptake and productivity in potato (Solanum tuberosum L.) plant inoculated with growth promoting salt tolerant Bacillus strains. Ecotox. Environ. Safe 178, 33–42. doi: 10.1016/j.ecoenv.2019.04.027
Tank, N., and Saraf, M. (2010). Salinity-resistant plant growth promoting rhizobacteria ameliorates sodium chloride stress on tomato plants. J. Plant Interact. 5, 51–58. doi: 10.1080/17429140903125848
Tewari, S., and Arora, N. K. (2014a). Multifunctional exopolysaccharides from Pseudomonas aeruginosa PF23 involved in plant growth stimulation, biocontrol and stress amelioration in sunflower under saline conditions. Curr. Microbiol. 69, 484–494. doi: 10.1007/s00284-014-0612-x
Tewari, S., and Arora, N. K. (2014b). Talc based exopolysaccharides formulation enhancing growth and production of Hellianthus annuus under saline conditions. Cell. Mol. Biol. 60, 73–81.
Tewari, S., and Arora, N. K. (2016). Fluorescent Pseudomonas sp. PF17 as an efficient plant growth regulator and biocontrol agent for sunflower crop under saline conditions. Symbiosis 68, 99–108. doi: 10.1007/s13199-016-0389-8
Tewari, S., and Arora, N. K. (2018). Role of salicylic acid from Pseudomonas aeruginosa PF23EPS+ in growth promotion of sunflower in saline soils infested with phytopathogen Macrophomina phaseolina. Environ. Sustain. 1, 49–59. doi: 10.1007/s42398-018-0002-6
Tilak, K. V. B. R., Ranganayaki, N., and Manoharachari, C. (2006). Synergistic effects of plant-growth promoting rhizobacteria and Rhizobium on nodulation and nitrogen fixation by pigeonpea (Cajanus cajan). Eur. J. Soil Sci. 57, 67–71. doi: 10.1111/j.1365-2389.2006.00771.x
Timmusk, S., El-Daim, I. A. A., Copolovici, L., Tanilas, T., Kännaste, A., Behers, L., et al. (2014). Drought-tolerance of wheat improved by rhizosphere bacteria from harsh environments: enhanced biomass production and reduced emissions of stress volatiles. PLoS One 9:e96086. doi: 10.1371/journal.pone.0096086
Tiwari, G., Duraivadivel, P., Sharma, S., and Hariprasad, P. (2018). 1-Aminocyclopropane-1-carboxylic acid deaminase producing beneficial rhizobacteria ameliorate the biomass characters of Panicum maximum Jacq. by mitigating drought and salt stress. Sci. Rep. 8:17513. doi: 10.1038/s41598-018-35565-3
TrParray, A. P., Jan, S., Kamili, A. N., Qadri, R. A., Egamberdieva, D., and Ahmad, P. (2016). Current perspectives on plant growth promoting rhizobacteria. Plant Growth Regul. 35, 877–902.
Ullah, S., and Bano, A. (2015). Isolation of plant-growth-promoting rhizobacteria from rhizospheric soil of halophytes and their impact on maize (Zea mays L.) under induced soil salinity. Can. J. Microbiol. 61, 307–313. doi: 10.1139/cjm-2014-0668
Upadhyay, S. K., and Singh, D. P. (2015). Effect of salt-tolerant plant growth-promoting rhizobacteria on wheat plants and soil health in a saline environment. Plant Biol. 17, 288–293. doi: 10.1111/plb.12173
Upadhyay, S. K., Singh, D. P., and Saikia, R. (2009). Genetic diversity of plant growth promoting rhizobacteria isolated from rhizospheric soil of wheat under saline condition. Curr. Microbiol. 59, 489–496. doi: 10.1007/s00284-009-9464-1
Upadhyay, S. K., Singh, J. S., Saxena, A. K., and Singh, D. P. (2012). Impact of PGPR inoculation on growth and antioxidant status of wheat under saline conditions. Plant Biol. 14, 605–611. doi: 10.1111/j.1438-8677.2011.00533.x
Vaishnav, A., Kumari, S., Jain, S., Varma, A., Tuteja, N., and Choudhary, D. K. (2016). PGPR-mediated expression of salt tolerance gene in soybean through volatiles under sodium nitroprusside. J. Basic Microbiol. 56, 1274–1288. doi: 10.1002/jobm.201600188
Van Oosten, M. J., Di Stasio, E., Cirillo, V., Silletti, S., Ventorino, V., Pepe, O., et al. (2018). Root inoculation with Azotobacter chroococcum 76A enhances tomato plants adaptation to salt stress under low N conditions. BMC Plant Biol. 18:205. doi: 10.1186/s12870-018-1411-5
Vardharajula, S., Ali, S. Z., Grover, M., Reddy, G., and Bandi, V. (2011). Drought-tolerant plant growth promoting Bacillus spp.: effect on growth, osmolytes, and antioxidant status of maize under drought stress. J. Plant Interact. 6, 1–14. doi: 10.1080/17429145.2010.535178
Vives-Peris, V., Gomez-Cadenas, A., and Perez-Clemente, R. M. (2018). Salt stress alleviation in citrus plants by plant growth-promoting rhizobacteria Pseudomonas putida and Novosphingobium sp. Plant Cell Rep. 37, 1557–1569. doi: 10.1007/s00299-018-2328-z
Wang, Q., Dodd, I. C., Belimov, A. A., and Jiang, F. (2016). Rhizosphere bacteria containing 1-aminocyclopropane-1-carboxylate deaminase increase growth and photosynthesis of pea plants under salt stress by limiting Na+ accumulation. Funct. Plant Biol. 43, 161–172.
Wolde, G., and Adamu, C. (2018). Impact of salinity on seed germination and biomass yields of field pea (Pisum sativum L.). Asian J. Sci. Tech. 09, 7565–7569.
Woo, S. L., and Pepe, O. (2018). Microbial consortia: promising probiotics as plant biostimulants for sustainable agriculture. Front. Plant Sci. 9:1801. doi: 10.3389/fpls.2018.01801
Wu, Z., Yao, L., Kaleem, I., and Li, C. (2012). “Application efficacy of biological seed coating agent from combination of PGPR on cotton in the field,” in Information Technology and Agricultural Engineering. Advances in Intelligent and Soft Computing, Vol. 134, eds E. Zhu, and S. Sambath (Berlin: Springer).
WWAP (2012). World Water Assessment Programme. The United Natins World Water Development Report 4: Managing Water under Uncertainity and Risk. Paris: UNESCO.
Yanni, Y., Zidan, M., Dazzo, F., Rizk, R., Mehesen, A., Abdelfattah, F., et al. (2016). Enhanced symbiotic performance and productivity of drought stressed common bean after inoculation with tolerant native rhizobia in extensive fields. Agric. Ecosys. Environ. 232, 119–128. doi: 10.1016/j.agee.2016.07.006
Yao, L., Wu, Z., Zheng, Y., Kaleem, I., and Li, C. (2010). Growth promotion and protection against salt stress by Pseudomonas putida Rs-198 on cotton. Eur. J. Soil Biol. 46, 49–54. doi: 10.1016/j.ejsobi.2009.11.002
Yasin, N. A., Khan, W. U., Ahmad, S. R., Ali, A., Ahmad, A., and Akram, W. (2018). Imperative roles of halotolerant plant growth-promoting rhizobacteria and kinetin in improving salt tolerance and growth of black gram (Phaseolus mungo). Environ. Sci. Pollut. Res. 25, 4491–4505. doi: 10.1007/s11356-017-0761-0
Zafar-ul-Hye, M., Muhammad, H., Zahir, F., Ahmad, Z., Hussain, M., and Hussain, A. (2014). Application of ACC-deaminase containing rhizobacteria with fertilizer improves maize production under drought and salinity stress. Int. Int. J. Agric. Biol. 16, 591–596.
Zahir, Z. A., Shah, M. K., Naveed, M., and Akhter, M. J. (2010). Substrate-dependent auxin production by Rhizobium phaseoli improves the growth and yield of Vigna radiata L. under salt stress conditions. J. Microbiol. Biotechnol. 20, 1288–1294. doi: 10.4014/jmb.1002.02010
Zahir, Z. A., Zafar-ul-Hye, M., Sajjad, S., and Naveed, M. (2011). Comparative effectiveness of Pseudomonas and Serratia sp. containing ACC-deaminase for coinoculation with Rhizobium leguminosarum to improve growth, nodulation, and yield of lentil. Biol. Fert. Soils. 47, 457–465. doi: 10.1007/s00374-011-0551-7
Zahran, H. H. (1991). Conditions for successful Rhizobium-legume symbiosis in saline environments. Biol. Fert. Soils. 12, 73–80. doi: 10.1007/BF00369391
Zahran, H. H. (1999). Rhizobium-legume symbiosis and nitrogen fixation under severe conditions and in an arid climate. Microbiol. Mol. Biol. Rev. 63, 968–989.
Zerrouk, I. Z., Benchabane, M., Khelifi, L., Yokawa, K., Ludwig-Müller, J., and Baluska, F. (2016). A Pseudomonas strain isolated from date-palm rhizospheres improves root growth and promotes root formation in maize exposed to salt and aluminum stress. J. Plant Physiol. 191, 111–119. doi: 10.1016/j.jplph.2015.12.009
Zhang, S., Fan, C., Wang, Y., Xia, Y., Xiao, W., and Cui, X. (2018). Salt-tolerant and plant-growth-promoting bacteria isolated from high-yield paddy soil. Can. J. Microbiol. 64, 968–978. doi: 10.1139/cjm-2017-2571
Zhong, N., Han, L., Wu, X., Wang, L., Wang, F., Ma, Y., et al. (2012). Ectopic expression of a bacterium NhaD-type Na+/H+ antiporter leads to increased tolerance to combined salt/alkali stresses. J. Integr. Plant Biol. 54, 412–421. doi: 10.1111/j.1744-7909.2012.01129.x
Zhou, C., Zhu, L., Xie, Y., Li, F., Xiao, X., Ma, Z., et al. (2017). Bacillus licheniformis SA03 confers increased saline-alkaline tolerance in Chrysanthemum plants by induction of abscisic acid accumulation. Front. Plant Sci. 8:1143–1143. doi: 10.3389/fpls.2017.01143
Zhu, J. K. (2003). Regulation of ion homeostasis under salt stress. Curr. Opin. Plant Biol. 6, 441–445. doi: 10.1016/S1369-5266(03)00085-2
Keywords: salinity, climate change, PGPR, crop productivity, agro-ecosystem
Citation: Egamberdieva D, Wirth S, Bellingrath-Kimura SD, Mishra J and Arora NK (2019) Salt-Tolerant Plant Growth Promoting Rhizobacteria for Enhancing Crop Productivity of Saline Soils. Front. Microbiol. 10:2791. doi: 10.3389/fmicb.2019.02791
Received: 30 April 2019; Accepted: 18 November 2019;
Published: 18 December 2019.
Edited by:
Charlotte Grossiord, Swiss Federal Institute for Forest, Snow and Landscape Research (WSL), SwitzerlandReviewed by:
Vijay Singh Meena, ICAR-Vivekananda Parvatiya Krishi Anusandhan Sansthan, IndiaMuhammad Naveed, University of Agriculture Faisalabad, Pakistan
Copyright © 2019 Egamberdieva, Wirth, Bellingrath-Kimura, Mishra and Arora. This is an open-access article distributed under the terms of the Creative Commons Attribution License (CC BY). The use, distribution or reproduction in other forums is permitted, provided the original author(s) and the copyright owner(s) are credited and that the original publication in this journal is cited, in accordance with accepted academic practice. No use, distribution or reproduction is permitted which does not comply with these terms.
*Correspondence: Dilfuza Egamberdieva, ZGlsZnV6YS5lZ2FtYmVyZGlldmFAemFsZi5kZQ==; Naveen K. Arora, bmthcm9yYS5iYmF1QGdtYWlsLmNvbQ==