- 1Department of Oral Diagnosis, Piracicaba Dental School, UNICAMP, Piracicaba, Brazil
- 2Department of Immunology and Infectious Disease, The Forsyth Institute, Cambridge, MA, United States
S. mitis is an abundant member of the commensal microbiota of the oral cavity and pharynx, which has the potential to promote systemic infections. By analyzing a collection of S. mitis strains isolated from the oral cavity at commensal states or from systemic infections (blood strains), we established that S. mitis ubiquitously express the surface immunodominant protein, PcsB (also called GbpB), required for binding to sucrose-derived exopolysaccharides (EPS). Immuno dot blot assays with anti-PcsB antibodies and RT-qPCR transcription analyses revealed strain-specific profiles of PcsB production associated with diversity in pcsB transcriptional activities. Additionally, blood strains showed significantly higher levels of PcsB expression compared to commensal isolates. Because Streptococcus mutans co-colonizes S. mitis dental biofilms, and secretes glucosyltransferases (GtfB/C/D) for the synthesis of highly insoluble EPS from sucrose, profiles of S. mitis binding to EPS, biofilm formation and evasion of the complement system were assessed in sucrose-containing BHI medium supplemented or not with filter-sterilized S. mutans culture supernatants. These analyses showed significant S. mitis binding to EPS and biofilm formation in the presence of S. mutans supernatants supplemented with sucrose, compared to BHI or BHI-sucrose medium. In addition, these phenotypes were abolished if strains were grown in culture supernatants of a gtfBCD-defective S. mutans mutant. Importantly, GtfB/C/D-associated phenotypes were enhanced in high PcsB-expressing strains, compared to low PcsB producers. Increased PcsB expression was further correlated with increased resistance to deposition of C3b/iC3b of the complement system after exposure to human serum, when strains were previously grown in the presence of S. mutans supernatants. Finally, analyses of PcsB polymorphisms and bioinformatic prediction of epitopes with significant binding to MHC class II alleles revealed that blood isolates harbor PcsB polymorphisms in its functionally conserved CHAP-domain, suggesting antigenic variation. These findings reveal important roles of PcsB in S. mitis-host interactions under commensal and pathogenic states, highlighting the need for studies to elucidate mechanisms regulating PcsB expression in this species.
Introduction
Streptococcus mitis is abundant in multiple oropharyngeal sites, including mucosal and dental surfaces (Aas et al., 2005; Human Microbiome Project, 2012). Although classically recognized as a commensal organism, this species also emerges as an important opportunistic pathogen of systemic infections (Mitchell, 2011). The broad profile of S. mitis interactions with host sites compared to other streptococcal species might result from its high genetic and phenotypic diversity (Bek-Thomsen et al., 2008; Mitchell, 2011; Kilian et al., 2014). However, the molecular functions of S. mitis strains involved in host colonization as commensals and/or as opportunistic pathogens are poorly understood. Taxonomically grouped into the Mitis group along with Streptococcus oralis and Streptococcus pneumoniae (Facklam, 2002), S. mitis typically co-inhabits mucosal niches of streptococcal pathogens including Streptococcus pyogenes and S. pneumoniae, as well as other commensal streptococci, e.g., Streptococcus salivarius (Salivarius group) (Aas et al., 2005; Human Microbiome Project, 2012). S. mitis strains further participate in the colonization of dental surfaces (Diaz et al., 2006; Heller et al., 2016), interacting with commensal species of the Sanguinis group, and with species associated with caries pathogenesis (Streptococcus mutans).
Because the genomes of S. mitis strains harbor gene orthologs found in several streptococcal species co-habiting their major host niches (Johnston et al., 2010; Kilian et al., 2014; Lessa et al., 2018), it is likely that gene expression profiles of pioneer S. mitis strains influence immune responses to shared epitopes expressed by related species under commensal or pathogenic life-styles (Smith and Mattos-Graner, 2008; Nogueira et al., 2012; Lessa et al., 2018). One of these conserved antigens is PcsB (Protein required for cell wall separation of group B Streptococcus, PcsB), also known as GbpB (Glucan-binding protein B) in S. mutans (Mattos-Graner et al., 2001). In S. pneumoniae and S. mutans, PcsB/GbpB are secreted and surface-associated proteins involved in cell wall division (Ng et al., 2003; Duque et al., 2011; Sham et al., 2011) and/or surface interaction with exopolysaccharides (EPS) during biofilm formation (Mattos-Graner et al., 2001, 2006). Importantly, high titers of salivary IgA antibody to S. mutans GbpB or of serum IgG antibody to S. pneumoniae PcsB are naturally developed in young children and/or adults, who show limited carriage of these respective species, suggesting protective effects of anti-GbpB/PcsB antibodies (Nogueira et al., 2005; Giefing et al., 2008). The reasons for these individual robust immune responses to GbpB/PcsB remain to be elucidated. One hypothesis is that expression of GbpB/PcsB orthologs by pioneer strains of commensal streptococci, e.g., S. mitis, might prime immune responses to conserved epitopes capable of modulating persistence of more pathogenic lineages. However, there is no information about conservation of PcsB epitopes within S. mitis streptococci, nor about S. mitis profiles of PcsB expression associated with virulence and persistence traits. Therefore in this study, we assessed pcsB polymorphisms within conserved PcsB epitopes, and investigated whether diversity in PcsB expression within S. mitis strains isolated from different host sites could be associated with PcsB-mediated phenotypes.
Materials and Methods
Bacterial Strains, Plasmids, and Growth Conditions
A total of 20 S. mitis strains were included in this study (Table 1). Twelve of these strains were isolated from seven healthy infants who were 2–16 months of age, and who attended an oral health education and prevention program of the Centro de Pesquisas e Atendimento Odontológico para Pacientes Especiais (CEPAE) of the Piracicaba Dental School, State University of Campinas (UNICAMP), SP, Brazil. These isolates were obtained from the oral mucosal sites (gingival crevices, cheeks, palate and tongue dorsum) with sterile swabs, using a protocol previously approved by the Ethics Committee of FOP-UNICAMP (protocol 055/2010), as previously described (Palma et al., 2016). These isolates were identified as those of S. mitis species by using an identification system with specific primers (Garnier et al., 1997) and confirmed by sequencing of 16S rRNA genes. Eight S. mitis strains with available genomes at the GenBank1, which were isolated from the oral cavity/dental biofilms (n = 3) or from the bloodstream of patients with clinical symptoms of bacteremia or septicemia (n = 5) were also analyzed. These strains were provided by Dr. Mogens Killian (Aarhus University, Denmark) and were deposited at CCUG as indicated in Table 1. Clinical backgrounds of patients harboring the blood strains SK579, SK569, and SK575 were previously described (Bochud et al., 1994). There is no published information about clinical conditions of subjects harboring strains SK616 and SK1073. The streptococcal strains were cultured in BHI (37°C) under aerobic (10% CO2 in air or aerobic shaking) or anaerobic (80% N2, 10% CO2, 10% H2) atmospheres. Escherichia coli strains TOP10 and BL21 were aerobically grown in Luria-Bertani medium supplemented with ampicillin (100 mg/ml) when required.
Analyses of PcsB Polymorphisms and Conserved Epitopes
Complete sequences of pcsB were obtained from all the studied strains. Briefly, genomic DNAs were purified using Master Pure DNA purification kit (Epicenter Technologies, Madison, WI, USA), and applied for amplification of the chromosomal region located 84 bp upstream to 169 bp downstream of pcsB encoding region, using primer sets designed using the genome of strain NCTC12261 as reference (Table 2). Amplicons were sequenced using a 3,500 Genetic Analyzer 8 capillary sequencer (Applied Biosystems HITACHI) and sequences edited using BioEdit 7.2.52. Multiple sequence alignments were performed using ClustalW3 and/or BoxShade v 3.21 tools4. A similarity cladogram of S. mitis PcsB and orthologous proteins of oropharyngeal streptococci were obtained using the Phylogeny.fr platform (MABL; http://www.phylogeny.fr/) (Dereeper et al., 2008, 2010). Orthologous protein of Enterococcus faecium DO (secreted antigen A; GenBank accession number YP_006377165.1) was used as outgroup in the phylogenetic comparisons. Potential peptides with binding affinity to human alleles of Major Histocompatibility Complex (MHC) class II molecules within PcsB sequences were identified using Tepitope/Proped bioinformatics tool5 (Singh and Raghva, 2001).
Recombinant PcsB Protein and Monoclonal Antibodies
Recombinant His-tag S. mitis PcsB (rPcsB) was obtained by cloning the pcsB encoding region (amplified from strain NCTC12261 using specific primers; Table 2) into NcoI and XhoI cloning sites of plasmid pET22b + (Novagen) to yield PET-pcsB. PET-pcsB was transformed into E. coli BL21, and the recombinant protein isolated from 1 l cultures in LB medium supplemented with ampicillin (100 mg/ml) (A550nm 0.5) after induction of rPcsB expression during 3–4 h with 1 mM isopropyl-β-D-thiogalactopyranoside (IPTG). Afterward, rPcsB was purified by affinity chromatography using the Ni-NTA Purification System (Thermo Fisher Scientific, U.S.A), as described elsewhere (Camargo et al., 2018). Samples were dialyzed in phosphate buffered saline (PBS) at 4°C, and stored at −20°C until use. Protein extracts were monitored in 10% SDS-PAGE gels stained with Coomassie blue. Monoclonal antibodies (MAb) against rPcsB were produced in mice using standard protocols (Rhea Biotech, SP, Brasil). Specificity of anti-PcsB MAbs were analyzed in ELISA and western blot assays with rPcsB and with cell extracts of S. mitis NCTC12261.
Preparation of Protein Extracts of S. mitis Strains
Production of secreted and cell-associated PcsB was analyzed respectively in culture supernatants and whole cell extracts of cells grown in BHI under aerobic and anaerobic conditions until the mid-log phase of growth (A550nm 0.3). Briefly, adjusted numbers of cells from 18 h cultures in BHI were transferred to 25 ml of fresh BHI medium and incubated (37°C) under aerobiosis (shaking at 160 rpm) or anaerobiosis (10% CO2; 10% H2; 80% N2) until the A550nm 0.3. The culture supernatants of these cultures were collected by centrifugation (twice at 6,000 × g; 4°C; 4 min), neutralized by addition of 1 M NaOH and 10 μM of phenylmethylsulfonyl fluoride (PMSF), and stored at −70°C until use. For preparation of whole cell extracts, the cells harvested from volumes of 25 ml of the same cultures were washed twice with saline solution, suspended in 2 ml of MilliQ water, and mechanically disrupted in a Bead Beater (Biospec Products) with 0.16 g of 0.1-mm zirconia beads (2 cycles of 45 s with 1 min rest on ice). Extracts were then centrifuged (12,000 × g; 4°C; 1 min) and the supernatants stored at −70°C until use. Culture supernatants were dialyzed (overnight at 4°C) against cold phosphate buffer (PB; 0.2 M; pH 6.5), and then against cold Tris-HCl (0.125 M; pH 6.8; diluted 1:100). Samples were then 100-fold concentrated by freeze-drying. The protein concentrations of samples were determined using a Bradford assay (Sigma) according to the manufacturer’s protocol.
Imuno Dot Blot Assays
Amounts of PcsB in culture supernatants and cell extracts were quantified in immune dot blot assays, using MAbs anti-S. mitis rPcsB. Dot blotting of cell extracts or culture supernatants was performed as described (Mattos-Graner et al., 2001) with minor modifications. Briefly, nitrocellulose membranes (BioRad, CA, USA) were washed with phosphate buffer (PB) (0.2 M; pH 6.8) and applied to the dot blot apparatus (BioRad). Wells were then washed twice with 200 ul of PB, and 100 μl of culture supernatants or of protein extracts (equivalent to 10 μg of total protein) was applied per well. Samples of serially diluted rPcsB (1.95–250 ng) were also applied to each membrane for standard curves. After sample drainage, wells were washed twice with PB under vacuum, and the membranes removed and blocked with PBS supplemented with 5% skim milk (37°C under stirring, 60 min.). Afterward, the membranes were washed with PBS and incubated with anti-rPcsB MAbs (1:1,000) during 1.5 h at rt. After a new series of washes with PBS, the membranes were incubated with goat anti-mouse IgG antibody conjugated with horseradish peroxidase (1:10,000) (Thermo Scientific) (1.5 h at rt). As negative controls, membranes blotted with standard protein extracts obtained from a reference strain (NCTC12261) were probed only with the secondary antibody at the same conditions. PcsB probing was detected using the chemiluminescent SuperSignal West Dura system (Thermo Scientific, MA, USA), according to the manufacturer’s instructions, and converted by digital images using a GS-700 Imaging Densitometer. The intensities of PcsB signals for each well were then measured using the ImageJ 1.47 t software6 and converted to nanograms of PcsB based on the standard curves obtained in each blot. Relative levels of PcsB produced by each strain were obtained by summing of the respective measures of PcsB in culture supernatant and cell extract samples, which were expressed as arbitrary values. Three independent experiments were performed.
RNA Isolation and Reverse Transcription qPCR
Amounts of pcsB transcripts were determined in S. mitis at mid-log growth phase (A550nm 0.3), under aerobic or anaerobic conditions. Briefly, cells were harvested (6,000 × g; 4°C; 5 min.) from 25 ml of BHI cultures, resuspended in 1 ml of 0.9% saline, and frozen at −70°C. Afterward, these cells were mechanically disrupted in a Mini-BeadBeater (Biospec) with 0.16 g zirconia beads (0.1 mm diameter). RNA was isolated using a modified protocol from the RNeasy Mini kit (Qiagen) described elsewhere (Stipp et al., 2013). Briefly, disrupted cells were homogenized in RLT buffer (850 μl) and centrifuged (10,000 × g, 1 min, 4°C) for collection of the supernatants (700 μl), which were then mixed with ethanol (500 μl) and loaded onto columns. Further RNA purification steps were performed as recommended by the manufacturer. Afterward, samples were treated with 10 U Turbo DNase (Ambion) according to the manufacturer’s protocol, for removal of DNA. One microgram of RNA was then used for reverse transcription (RT) with random primers, using the SuperScript III system (Thermo Fisher Scientifc, USA), as described elsewhere (Stipp et al., 2008). Quantitative PCR was performed in a StepOne real-time PCR system (Thermo Fisher USA) in reaction samples (10 μl) containing 1 μl of cDNA samples, 1X Power SYBR green PCR master mix (Lifetech), and 10 μM of each primer for 16SrRNA or for pcsB (Table 2). The cycling conditions included incubation at 95°C (10 min), followed by 40 cycles of 95°C (15 s), 54°C (15 s), and 72°C (30 s). Assays were performed in duplicate with RNA samples obtained from three independent experiments.
Analysis of S. mitis Interactions With Sucrose-Derived Exopolysaccharides and Biofilm Formation
S. mitis interactions with sucrose-derived EPS were analyzed as previously described (Alves et al., 2016), in BHI with 1% sucrose supplemented or not with cell-free culture supernatants of S. mutans MT8148, as a source of glucosyltransferases (GtfB, GtfC, and GtfD). These three secreted enzymes are required for optimal synthesis of highly stable insoluble glucan EPS from sucrose by S. mutans (Ooshima et al., 2001; Bowen and Koo, 2011). As controls, culture supernatant of a triple ΔgtfBCD mutant obtained in MT8148 (designated BC7s) (Ooshima et al., 2001) was also used in these assays. Briefly, volumes of 5 ml of BHI or of filter-sterilized BHI culture supernatants of S. mutans strains (pH ≈ 6.6–6.8) were supplemented with 1% sucrose and inoculated with adjusted numbers of S. mitis tested strains. Samples were then incubated (37°C, 10% CO2 in air) for 18 h, and then the intensity of aggregation was scored visually from 0 to 3. To prepare the culture supernatants, the S. mutans strains (MT8148, BC7s, or UA159) were grown in 25 ml of BHI medium (37°C, 10% CO2 in air) until the mid-log phase of growth (A550nm 0.3, pH ≈ 6.6–6.8). Afterward, these cultures were centrifuged (6,000 × g; 4°C; 10 min), and the obtained supernatants sterilized by filtration through membranes with pores 0.22 μm in diameter (Corning®). As controls, S. mitis cultures were also mixed with S. mutans culture supernatants not supplemented with sucrose, or with fresh BHI with 1% sucrose.
Biofilm formation was assessed in microtiter plates as previously described (Mattos-Graner et al., 2001), with modifications. Briefly, BHI or filter-sterilized BHI culture supernatants of S. mutans (MT8148 or BC7s) supplemented with 1% sucrose were inoculated with adjusted numbers of S. mitis cells, and transferred in four replicates (200 μl per well) to polystyrene 96-well plates (flat-bottom; Cralplast). After incubation (37°C, 10% CO2 in air) for 18 h, plates were washed with distilled water to remove loosely attached cells, and the biofilms were stained with crystal violet. Stain was then eluted from biofilms in ethanol (30 min. at room temperature), and the absorbances of the eluates (A575nm) were expressed as indirect measures of biofilm biomass. The planktonic growth (A550nm) was assessed in the same cultures used in the biofilm assays to monitor bacterial growth.
Analysis of C3b Deposition on S. mitis Strains
Binding of C3b to S. mitis strains grown in BHI, BHI 1% sucrose, or in BHI S. mutans UA159 culture supernatant supplemented with 1% sucrose was assessed as previously described (Alves et al., 2016), with modifications. Serum samples applied in these assays were collected from one volunteer who showed standard serum levels of C3 and IgG immunoglobulins, and reference profiles of C3b-mediated opsonization (Alves et al., 2016, 2019), according to a standard protocol previously approved by the Ethical Committee of the Piracicaba Dental School, State University of Campinas, SP, Brazil (proc. n° 153/2014). Briefly, strains were grown until the A550nm 0.3, and approximately 107 CFU of cells were harvested by centrifugation (10,000 × g, 4°C), washed twice with PBS (pH 7.4), and incubated (37°C, 30 min) with 20% serum (in PBS). Cells were then washed twice with PBS-Tween 0.05% (PBST), and incubated (on ice, during 40 min.) with fluorescein isothiocyanate (FITC)-conjugated polyclonal goat anti-human C3 IgG antibody (ICN, CA, U.S.A) (1:300 in PBST). Afterward, cells were washed twice with PBST and fixed in 3% paraformaldehyde (in PBS) for analysis on a FACSCalibur flow cytometer (BD Biosciences). Levels of surface-bound C3b on C3b-positive cells were expressed as the geometric mean of fluorescence intensity (MFI), using forward and side scatter parameters to gate at least 25,000 bacteria. Control samples included bacteria treated only with PBS. Heat-inactivated sera (56°C for 20 min) were also used as negative controls in preliminary experiments.
Statistical Analysis
Phenotypic comparisons were performed using Kruskal-Wallis with post hoc Dunn’s multiple comparisons or Mann-Whitney tests. Spearman’s rank correlation was applied to analyze associations between relative amounts of PcsB produced and transcript levels of pcsB. Differences were considered significant when values of p ≤0.05 were obtained.
Results
Gene Structure and Conserved Immunogenic Epitopes of PcsB in S. mitis and Other Oropharyngeal Streptococci
BlastP analysis of S. mitis PcsB was initially used to investigate conservation of PcsB orthologs within streptococcal species of the oropharynx. The genomic structures of pcsB loci were then analyzed. PcsB orthologs identified in the analyzed genomes were annotated as SagA (secreted antigen), GSP-781 (general stress protein-781), CHAP-domain containing protein, or GbpB (Glucan-binding protein B). The degree of protein similarity and gene structure of pcsB loci were compatible with phylogenetic relationships of the species analyzed (Figure 1). Except for the S. oralis strain Uo5, gene structure or pcsB loci were highly conserved within the Mitis species (Figure 1A). In these species, genes located downstream to pcsB encode putative 30S ribosomal proteins (rpsB). Differently in S. oralis strain Uo5, the pcsB downstream genes included a gene encoding an additional “CHAP-domain containing protein,” which was spanned by genes encoding transposases. Upstream to pcsB genes, two genes encoding putative cell-shape determining proteins (mreC/D) were highly conserved in all streptococcal species analyzed, except for S. pyogenes. In Salivarius, Sanguinis, and Mutans group species, as well as in S. pyogenes strain MGAS8232, genes encoding ribose-phosphate diphosphokinase protein (prs) were located downstream to pcsB genes. We could not identify a PcsB ortholog in the S. pyogenes strain M1 GAS SF370. S. mitis PcsB shows high amino acid sequence similarity with most of the oral species (Figure 1B). The genomes of S. salivarius SK12 and S. pyogenes strain MGAS8232 include proteins with the lowest percentages of similarity with S. mitis PcsB (Figure 1B). Apart from differences in protein identity/similarity, all the PcsB orthologs found in the streptococcal species show a typical domain structure. These include an N-terminal signal peptide for protein secretion (amino acids 1–27 in S. mitis), by a leucine zipper domain (amino acids 65–93 in S. mitis), a variable alanine-rich linker region, and a C-terminal CHAP domain (Cys, His-dependent amidohydrolase/peptidase) with two conserved residues (Cys315 and His366) involved in peptidoglycan hydrolytic activity (Figure 2A). Because PcsBs were found to be immunodominant antigens of S. mutans and S. pneumoniae (Smith et al., 2003; Nogueira et al., 2005; Giefing-Kröll et al., 2011), we further investigated potential PcsB T epitopes with binding affinity to human MHC class II alleles (Tepitope/Proped tool). These bioinformatic analyses revealed five T epitopes localized in the most conserved part of PcsB proteins (Figures 2A, 3). Four of them were located in the N-terminal part of PcsB, and included sequences within or spanning the signal peptide and the leucine zipper domains (Figures 2A, 3). An additional C-terminal epitope was located in the CHAP domain. Therefore, PcsB T epitopes are located in functional regions, which are conserved within different streptococcal species of the oral cavity and pharynx.
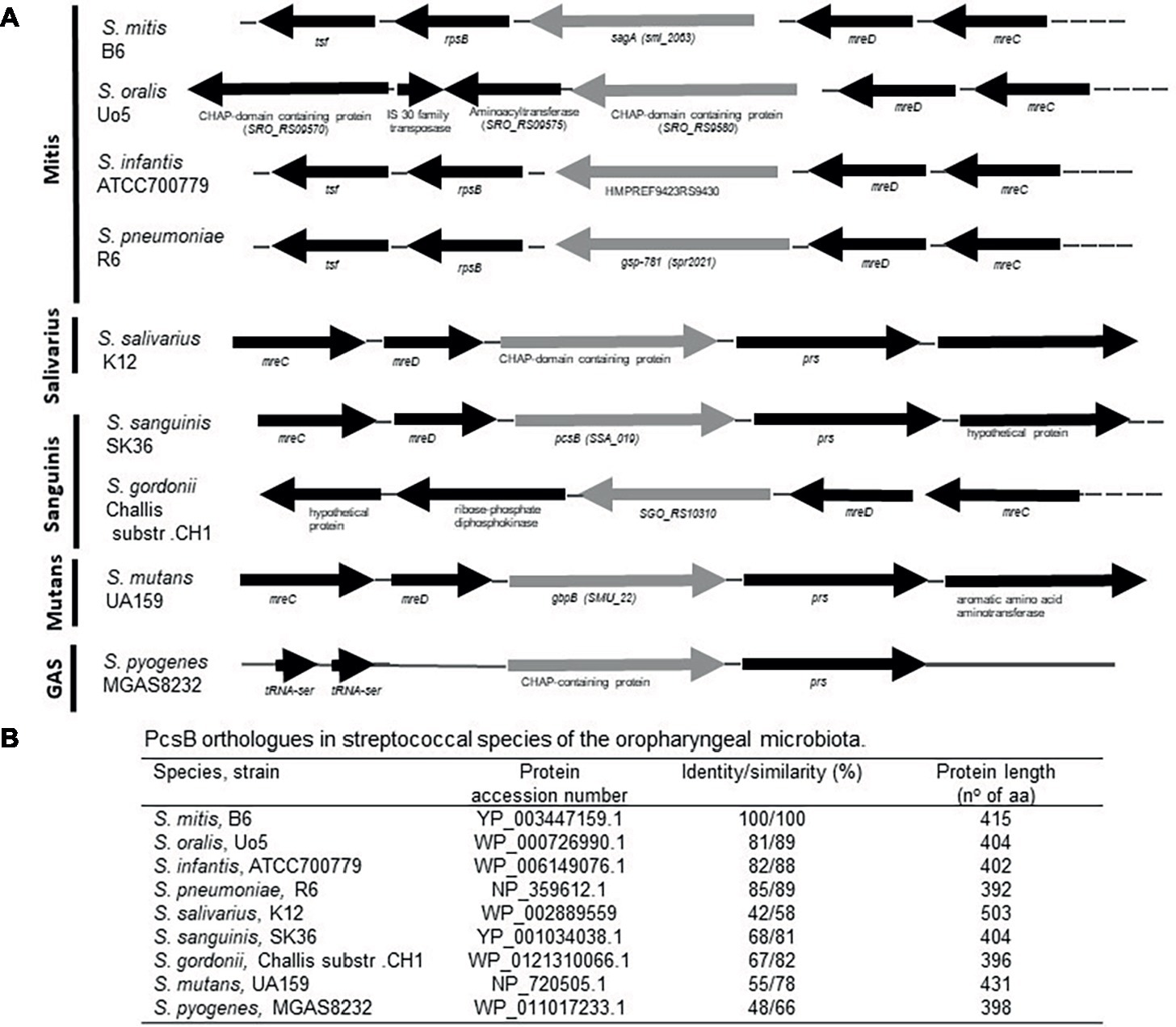
Figure 1. Comparative analyses of pcsB gene structure and homology in streptococcal species of the oral cavity and pharynx. (A) Schematic representation of the pcsB chromosomal loci in representative strains of eight species of the Mitis, Salivarius, Sanguinis, Mutans groups and of S. pyogenes (strain MGAS8232). Arrows represent direction of gene transcription; genes encoding the PcsB orthologs are represented in gray. Gene organization and designation were obtained from the GenBank database (http://www.ncbi.nlm.nih.gov/). (B) Results of BLASTp analyses using S. mitis PcsB sequence.
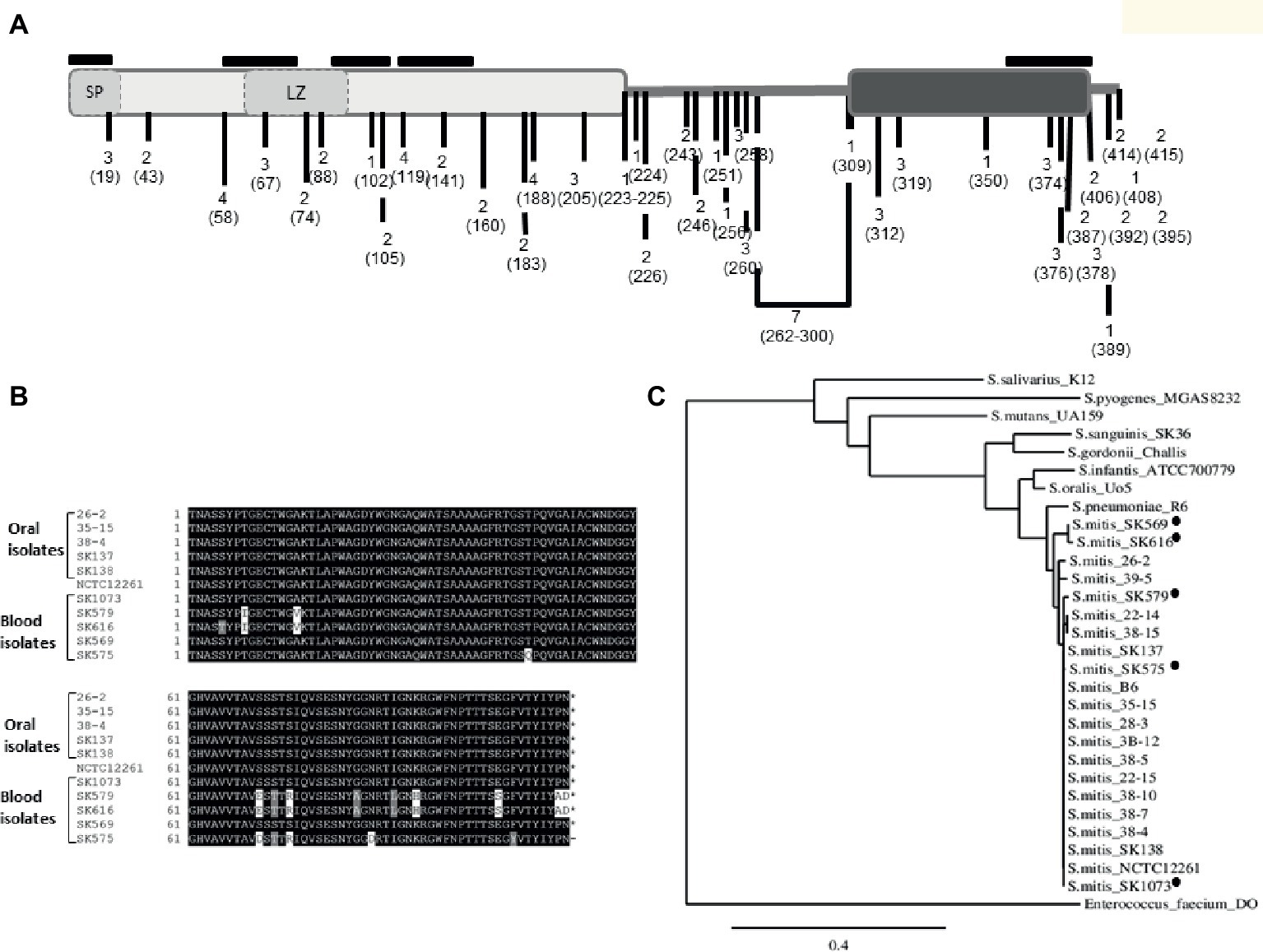
Figure 2. Polymorphisms of PcsB in S. mitis strains. (A) Schematic representation of S. mitis PcsB precursor protein with conserved functional domains. The PcsB N-terminal and C-terminal parts, respectively represented by light and dark gray rectangles, are linked by an alanine-rich variable region, which is represented by the bold line. In the N-terminal part, the signal peptide (SP) and the leucine zipper (LZ) motifs are represented by dashed rectangles. The frequencies of protein polymorphisms identified within 20 strains analyzed are indicated with numbers; the position of the amino acid changes is also indicated within parenthesis. The positions of five T cell epitope peptides are indicated with black lines, above the protein scheme. (B) BoxShade alignment of PcsB CHAP-domain sequences determined in five blood strains and in five representative oral strains. (C) Similarity cladogram of S. mitis PcsB and streptococcal orthologous proteins obtained using the Phylogeny.fr platform (http://www.phylogeny.fr/). S. mitis blood strains isolated from systemic infections are indicated with black circles. Orthologous protein of Enterococcus faecium DO (annotated as SagA) was used as outgroup.
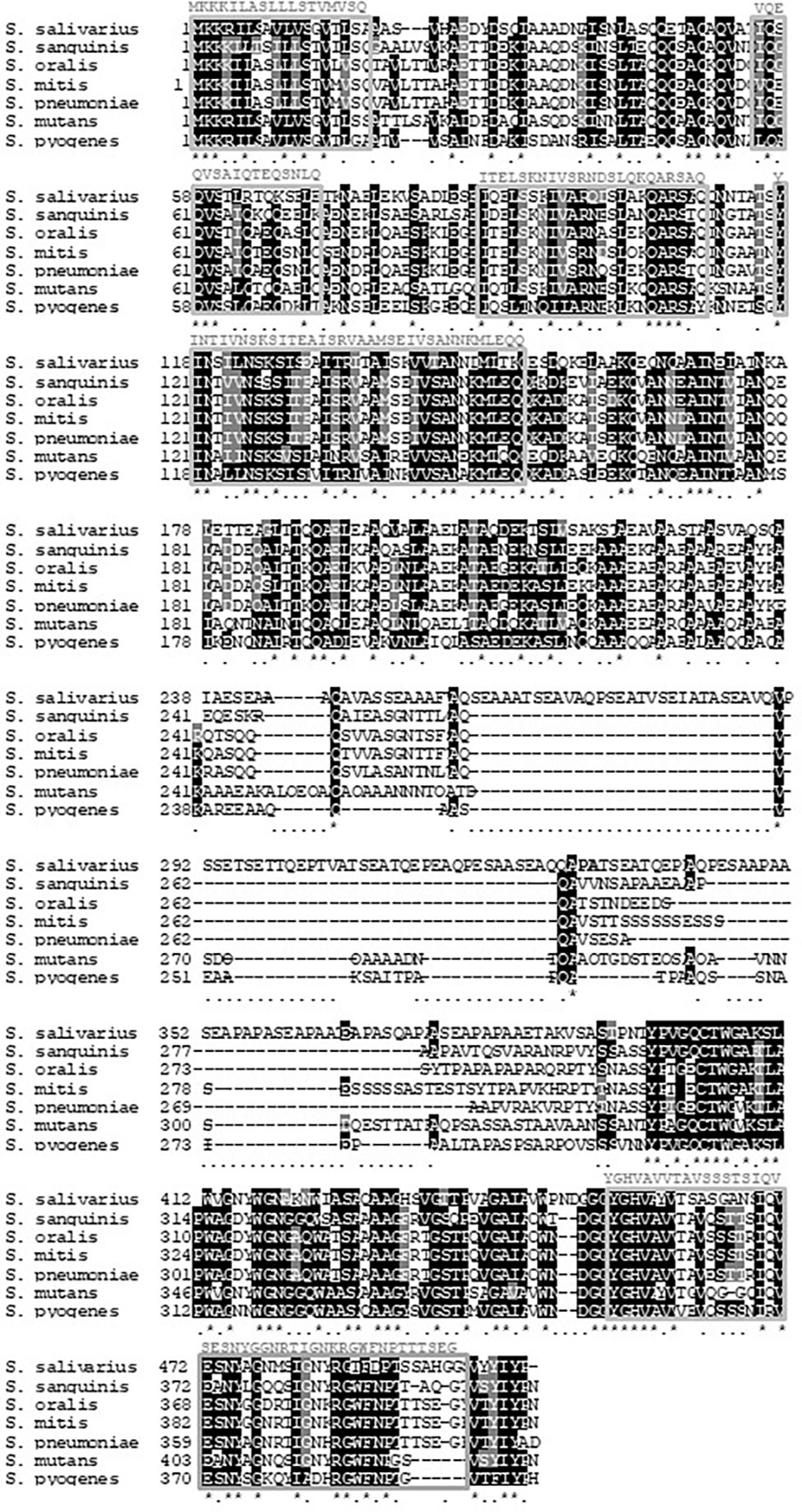
Figure 3. Localization of peptides with significant binding potential to MHC class II human alleles in PcsB orthologs. Sequences of PcsB orthologs identified in streptococcal species of the oral cavity and pharynx were aligned using BoxShade v 3.21 tools (https://embnet.vital-it.ch/software/BOX_form.html); asterisks indicate conserved residues; dots indicate non conserved residues. Five PcsB epitopes located at PcsB functional domains are indicated within boxes; predicted S. mitis PcsB epitope sequences are indicated above the respective boxes.
The Existence of Polymorphisms in S. mitis PcsB Proteins Associated With Functional Domains and Potential Immunogenic Epitopes
Comparisons of pcsB of the 20 studied strains (using strain NCTC12261 as reference) revealed several polymorphisms associated with amino acid changes within conserved functional domains, as well as insertion and deletions at the variable linker region. Figure 2A illustrates the frequency of amino acid changes within strains and the position of these protein polymorphisms, in relation to functional domains and to predicted T epitopes. A significant proportion of amino acid changes were within the five putative MHC class II peptides, and several of these mutations (n = 16) were within the C-terminal extremity of the CHAP-domain (position 305–385). Interestingly, most of the mutations on MHC class II peptides were present in three blood isolates (SK575, SK579, and SK616). The blood strain SK569 also accounted for a significant number of amino acid changes (n = 14), but most of them were located within the four N-terminal epitopes. The strains isolated from the oral cavity of infants showed a reduced number of polymorphisms associated with T epitopes (n = 2), and most of the polymorphisms found in these strains were not within functional domains. Figure 2B shows alignment of the N-terminal PcsB sequences containing the entire CHAP-domain (111 amino acids in length) of five blood strains as well as five representative oral strains. Phylogenetic analysis of PcsB sequences (Figure 2C) further shows that apart from polymorphisms identified within PcsB expressed by S. mitis strains, these proteins are more closely related compared to PcsB orthologs expressed by other species of oropharyngeal streptococci.
Diversity in Production of PcsB in S. mitis Strains Associated With Transcriptional Activities of pcsB
Profiles of PcsB production and protein localization were initially investigated in the studied S. mitis strains using protein cell extracts and samples of culture supernatants at mid-log phase of growth. Measures of secreted and cell-associated PcsB were assessed at different atmospheric conditions (aerobiosis and anaerobiosis), because S. mitis colonizes oral niches with varying oxygen tensions. Immuno dot blot analyses of secreted (culture supernatants) and cell extracts revealed significant diversity in total amounts of PcsB produced between strains. Diversity in total amounts of PcsB produced (secreted plus cell-associated PcsB) under aerobic conditions (Figure 4A) was significantly associated with diversity in PcsB production under anaerobiosis (Figure 4B) (Spearmans’s correlation analysis: r: 0.80; p < 0.05). In both atmospheric conditions, most of the PcsB was present in the culture supernatants (means: 84.57 ± 43.58 and 67.81 ± 40.32 in aerobiosis and anaerobiosis, respectively) compared to the cell fractions (means: 2.37 ± 1.95 and 0.68 ± 1.52 in aerobiosis and anaerobiosis, respectively). To further investigate mechanisms underlying diversity in PcsB production, we analyzed pcsB transcriptional activities by RT-qPCR, using RNA samples obtained from cells harvested from the same cultures assayed for protein production. As shown in Figures 4C,D, there was significant positive association between amounts of PcsB production and transcript levels of pcsB in both atmospheric conditions, implying that diversity in PcsB production between strains was promoted by differences in transcriptional activities of the respective pcsB genes.
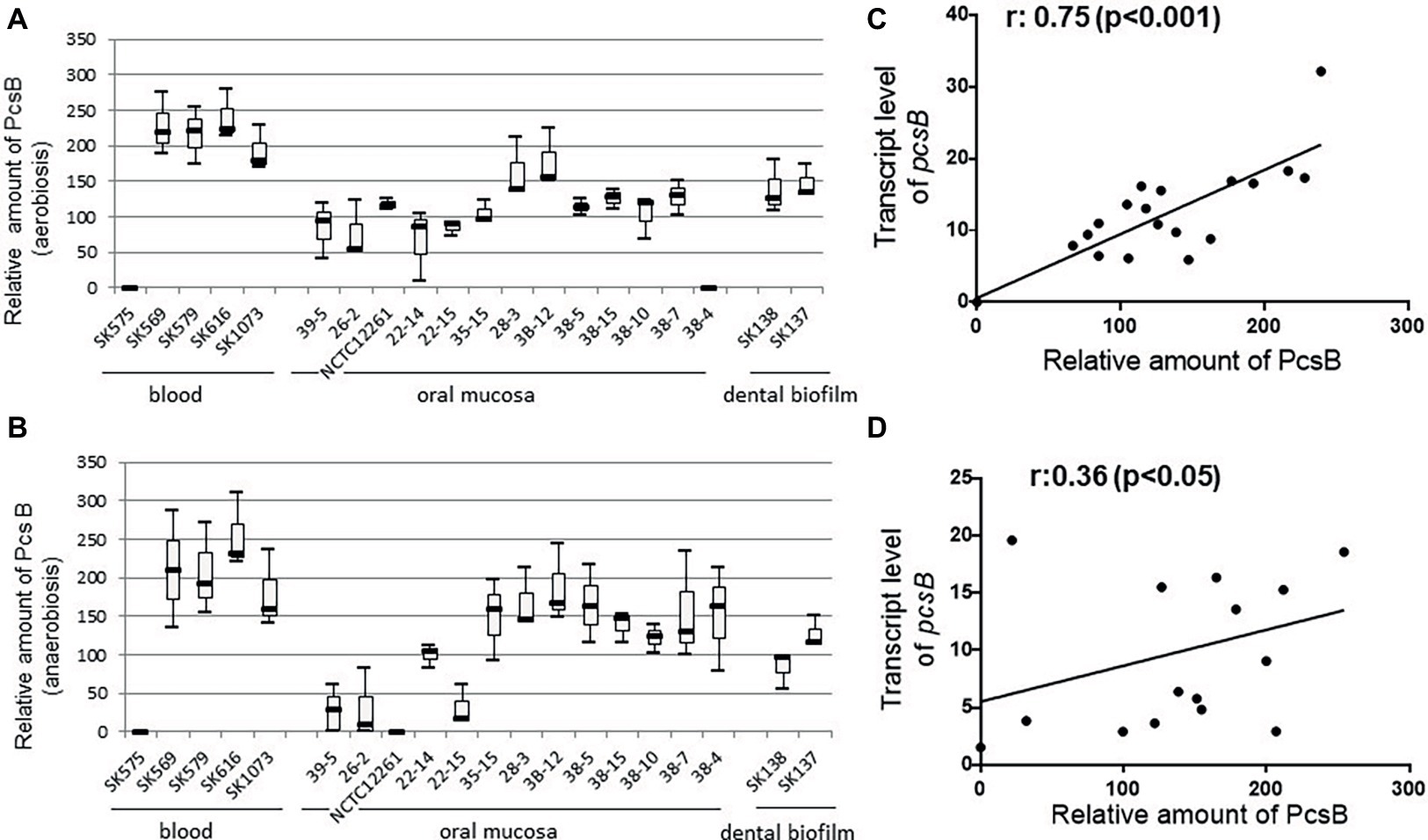
Figure 4. PcsB expression by S. mitis strains isolated from different host sites. Strains grown under aerobic (A) or anaerobic (B) conditions until the mid-log growth phase for collection of bacterial cells and culture supernatants, which were analyzed in immuno dot blot assays with MAbs specific to PcsB. Relative amounts of PcsB produced by each strain were measured by densitometry of the dot blots, under a linear range of PcsB detection. Horizontal lines within box plots indicate mean levels of three independent experiments; bars indicate standard deviations. Strain designations and sites of host isolation are indicated on the X-axis. (C,D) Spearman’s rank correlation analyses between relative amounts of PcsB produced and pcsB transcript levels (determined by RT-qPCR) in strains grown respectively at aerobic and anaerobic conditions.
S. mitis Strains Involved in Systemic Infections Show Increased Expression of PcsB and PcsB-Mediated Phenotypes
In S. mutans, up-regulation of genes involved in binding to sucrose-derived EPS is associated with systemic virulence (Alves et al., 2016). Thus, we compared profiles of PcsB production between S. mitis strains associated with systemic infections (blood strains) and strains isolated from oral sites at commensal states. As shown in Figure 5, blood strains produce increased amounts of PcsB, compared to oral strains, either under aerobic or anaerobic conditions. To further address if PcsB expression influences the strain capacity to bind sucrose-derived EPS, we selected strains with the highest (n = 4; strains SK569, SK579, SK616, SK1073) and lowest (n = 4; strains SK575, 38–4, 35–15, 26–2) levels of PcsB (produced under aerobic conditions) to compare bacterial aggregation mediated by sucrose-derived EPS. Of note, different from S. mutans, S. mitis does not secrete glucosyltransferase enzymes (Gtfs) required for the synthesis of insoluble glucan EPS from sucrose (Xu et al., 2018). Therefore, to supply S. mitis cultures with sucrose-derived EPS, S. mitis aggregation was assessed in filter-sterilized BHI culture supernatants of S. mutans MT8148 (which contain secreted Gtfs: GtfB, GtfC and GtfD) supplemented with sucrose. As controls of Gtf activities, we also supplemented S. mitis cultures with the culture supernatants of the S. mutans gtfBCD triple mutant obtained in MT8148. As shown in Figure 6, S. mutans reference strain UA159 and S. mitis strains show irrelevant aggregation in BHI without sucrose or in S. mutans supernatants in BHI without sucrose, the unique substrate of Gtfs. On the other hand, BHI supplementation with 1% sucrose promoted aggregation of S. mutans UA159, but this phenotype was less evident in most of the S. mitis strains. On the other hand, growth in BHI 1% sucrose medium supplemented with culture supernatants of S. mutans strain MT8148 clearly increased aggregation of S. mitis strains expressing high levels of PcsB, a phenotype not observed in low PcsB-expressing strains (Figure 6). Importantly, the enhanced aggregation phenotypes promoted by the supernatants of S. mutans MT8148 were abolished, when S. mitis strains were grown in BHI 1% sucrose medium with culture supernatants of the gtfBCD-defective strain (BC7s). Of note, aggregation of the gtfBCD-defective S. mutans was restored to parent levels, when this defective mutant was grown in BHI-sucrose medium supplemented with MT8148 culture supernatant.
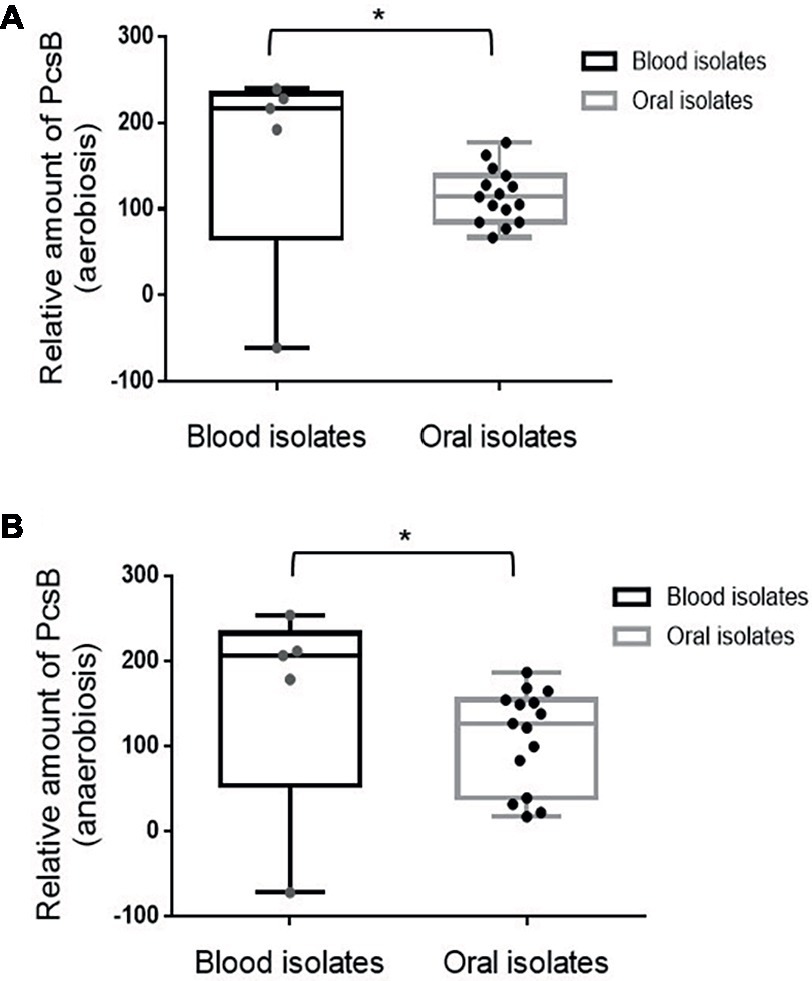
Figure 5. Box plot comparisons of PcsB production between S. mitis strains isolated from the bloodstream or from oral sites. Amounts of PcsB produced were determined using immuno dot blot assays in strains grown under aerobic (A) and anaerobic (B) conditions. Mean levels of PcsB are represented by horizontal lines within boxes. Bars represent standard deviations. Asterisks indicate statistically significant differences between groups in Mann-Whitney U-test (p < 0.05).
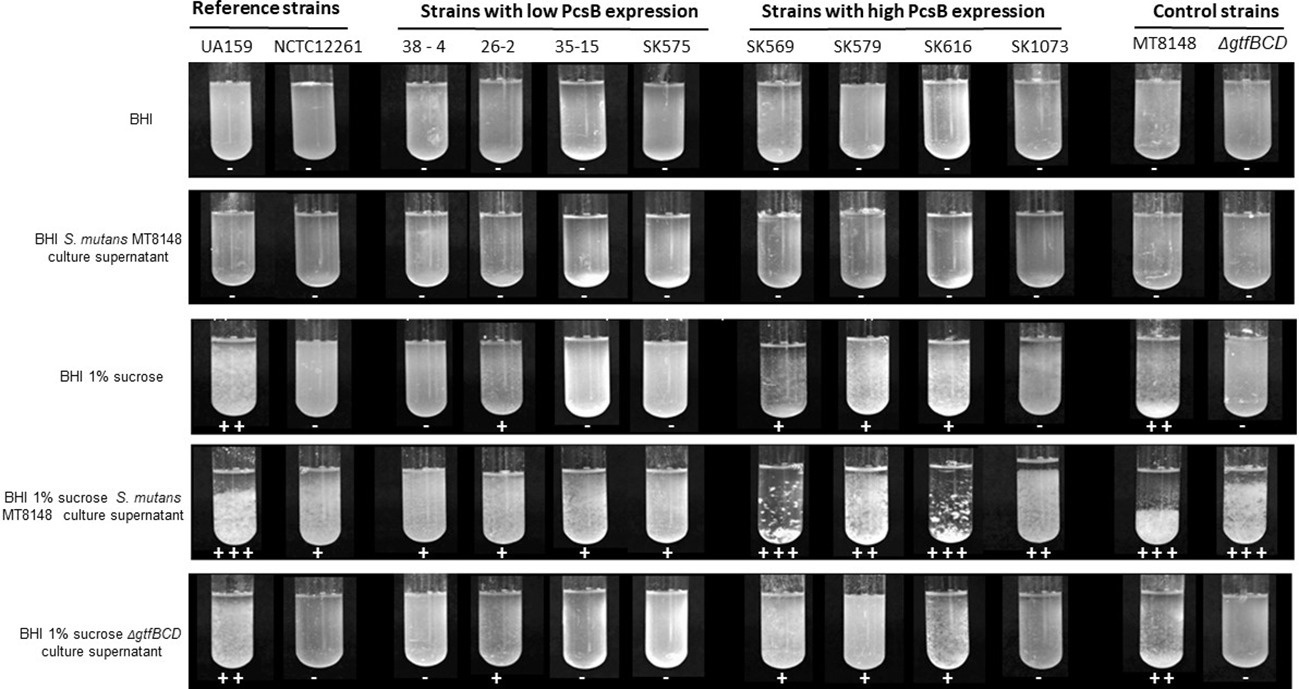
Figure 6. Analysis of bacterial binding to EPS. S. mitis strains with the highest (n = 4) and lowest (n = 4) levels of PcsB produced were grown in BHI or BHI with 1% sucrose supplemented or not with filter-sterilized culture supernatants of S. mutans strains (MT8148 or ΔgtfBCD isogenic mutant). Bacterial aggregation mediated by EPS synthesized from sucrose was then visually examined and the intensities of aggregation scored from 0 (−) to 3 (+++), as indicated below the respective cultures. The reference strains S. mutans UA159 and S. mitis NCTC12261 were also analyzed.
Because expression of gbpB is required for biofilm formation mediated by sucrose-derived EPS in S. mutans (Duque et al., 2011), and GbpB expression influences strain-specific capacities to form biofilms (Mattos-Graner et al., 2001), we compared the amounts of biofilms formed by S. mitis strains under the same culture conditions used to investigate EPS binding. As shown in Figure 7A, significant increases in biofilm biomass were observed in all high PcsB-expressing S. mitis strains grown in BHI-sucrose supplemented with S. mutans MT8148 culture supernatants, compared to biofilms formed in either BHI-sucrose or BHI-sucrose supplemented with ΔgtfBCD mutant culture supernatant. The S. mutans gtfBCD-defective mutant further showed a high increase in biofilm biomass when grown in BHI-sucrose supplemented with culture supernatants of parent strain MT8148. Comparisons of mean biofilm biomasses between S. mitis strains with low versus high PcsB expression further showed increased capacities of high PcsB producers to form biofilms in the presence of BHI-sucrose with MT8148 culture supernatant (Figure 7B). On the other hand, no significant differences between groups were detected when strains were grown in BHI-sucrose (Figure 7C) or BHI-sucrose with culture supernatants of the ΔgtfBCD mutant strain (Figure 7D). Consistently, correlation analysis revealed that PcsB expression levels were significantly associated with the biomasses of biofilms formed in BHI-sucrose supplemented with MT8148 culture supernatant (Figure 7E), but not with the biomasses of biofilms formed in BHI-sucrose medium (Figure 7F) or in BHI-sucrose with culture supernatants of the ΔgtfBCD mutant (Figure 7G). Growth of S. mitis strains in BHI-sucrose medium supplemented with culture supernatants of S. mutans UA159 instead of MT8148 resulted in similar EPS-binding and biofilm phenotypes (data not shown).
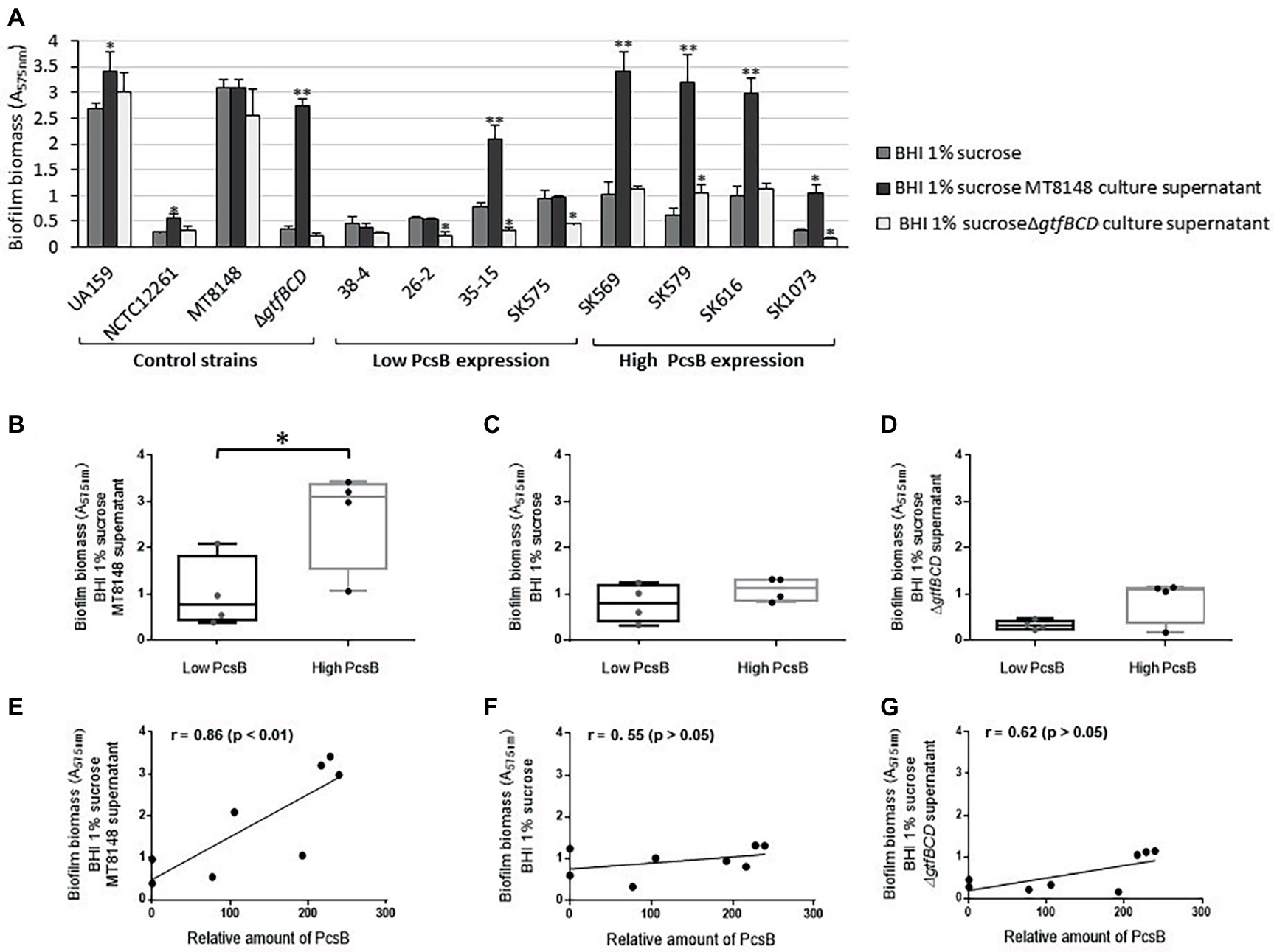
Figure 7. Comparisons of biofilm formation by S. mitis strains differing in PcsB expression levels. (A) Biofilm biomass was assessed in biofilms formed in microtiter plates by S. mitis strains grown in BHI 1% sucrose or in BHI 1% sucrose supplemented with culture supernatants of S. mutans MT8148 or ΔgtfBCD mutant strain. Columns represent means of four replicates of one representative experiment. Asterisks above columns indicate statistically significant differences in relation to the biofilms formed by the same strain in BHI 1% sucrose (Kruskal-Wallis with post hoc Dunn’s multiple comparisons: *p < 0.05; **p < 0.01). (B–D) Box plot comparisons of biofilm biomasses between strains expressing low (n = 4) versus high (n = 4) levels of PcsB, in BHI-sucrose MT8148 culture supernatant (B), BHI-sucrose (C) or BHI-sucrose ΔgtfBCD mutant culture supernatant (D). Asterisk indicates significant differences between groups (Mann-Whitney; *p < 0.05). (E–G) Spearman’s correlation analysis between total levels of PcsB production and biomasses of biofilms formed in either BHI-sucrose MT8148 culture supernatant (E), BHI-sucrose (F), or BHI-sucrose ΔgtfBCD mutant culture supernatant (G).
S. mitis Strains Expressing Increased Levels of PcsB Show Resistance to Complement Deposition
S. mutans binding to sucrose-derived EPS promotes the formation of a capsule-like structure for evasion to complement immunity (Alves et al., 2016). We thus investigated whether S. mitis strains expressing high PcsB levels (SK569, SK579, SK616, SK1073) have resistance to complement deposition compared to low PcsB-producing strains (SK575, 38–4, 35–15, 26–2). These comparisons were performed with strains grown either in BHI, BHI 1% sucrose, or in BHI 1% sucrose supplemented with S. mutans UA159 culture supernatants. In all the tested conditions, high PcsB-expressing strains showed significantly lower levels of C3b binding compared to low PcsB producers (Mann-Whitney, p < 0.05; data not shown). The lowest levels of C3b deposition were observed when strains were grown in BHI 1% sucrose culture supernatants of S. mutans UA159. Figure 8A shows group comparisons of C3b binding at this growth condition. Correlation analysis between levels of C3b deposition on strains grown in BHI-sucrose medium with UA159 culture supernatant and levels of PcsB production further indicates influence of PcsB expression in complement evasion (Figure 8B). Therefore, increased levels of PcsB promote resistance to complement deposition in S. mitis strains at conditions that favor the synthesis of sucrose-derived EPS.
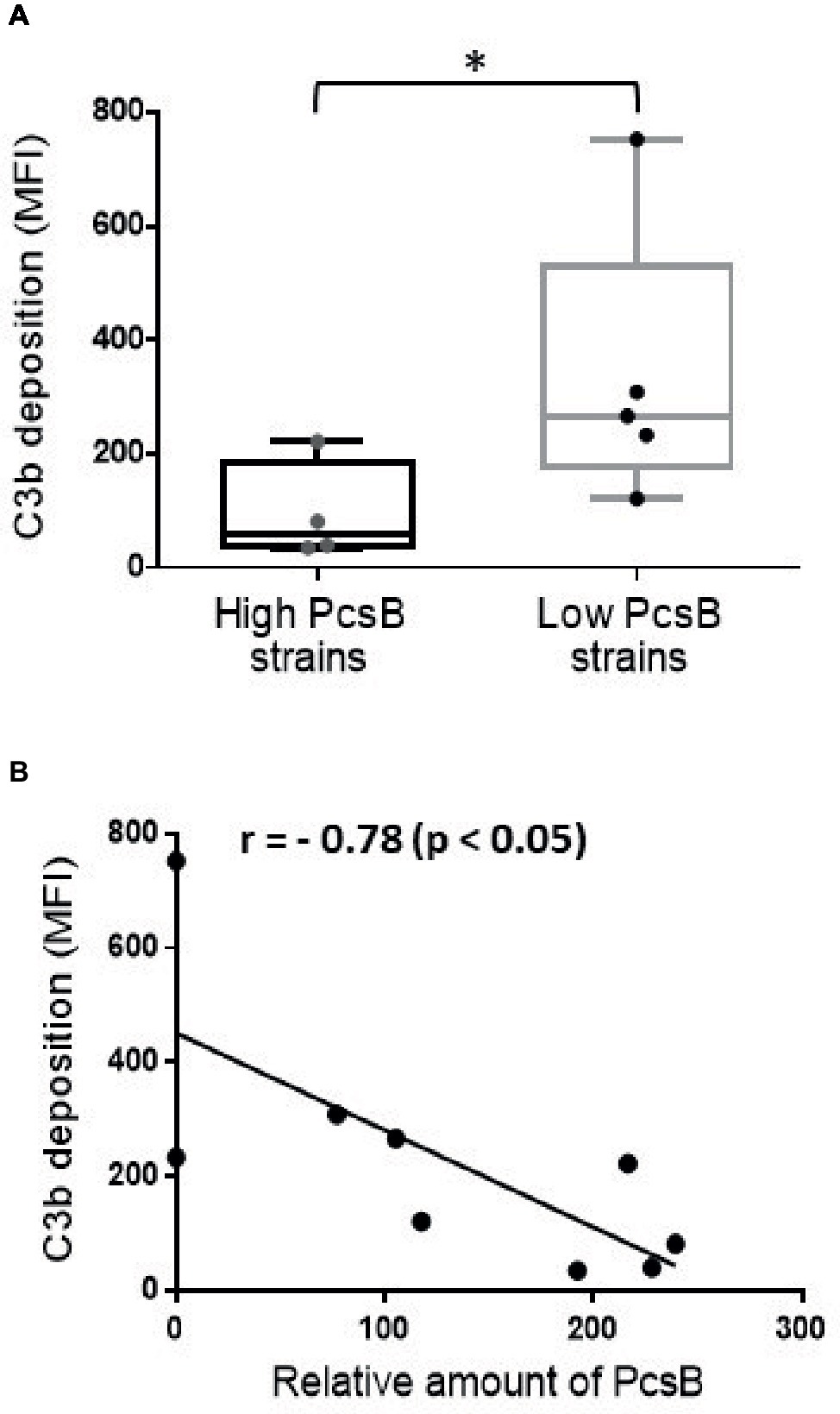
Figure 8. Association between PcsB expression and resistance to C3b deposition in S. mitis strains. Strains grown in S. mutans UA159 BHI culture supernatants supplemented with 1% sucrose (until A757nm 0.3) were treated with 20% human serum, and surface-bound C3b probed with FITC-conjugated anti-human C3 antibody for quantification by flow cytometry; levels of surface-bound C3b were expressed as geometric means of fluorescent intensities (MFI). Asterisks indicate statistically significant differences between groups in Mann-Whitney U-test (p < 0.05). (A) Box plot comparisons of C3b deposition between strains with high (n = 4) and low levels (n = 5) of PcsB production. (B) Spearman’s correlation analysis between C3b deposition and relative amounts of PcsB produced.
Discussion
S. mitis is an abundant member of the oral microbiota of humans from early life to adulthood (Smith et al., 1993; Kononen et al., 2002; Aas et al., 2005; Bek-Thomsen et al., 2008; Human Microbiome Project, 2012), which implies commensal interactions with host immune functions. S. mitis species shows significant genomic and phenotypic diversity, and the genomes of strains isolated from opportunistic systemic infections, and at commensal states, include virulence gene orthologs typical of the pathogenic species S. pneumoniae, a close S. mitis relative (Whatmore et al., 2000; Kilian et al., 2008, 2014; Donati et al., 2010; Johnston et al., 2010). Evolutionary genomic comparisons of S. mitis and S. pneumoniae strains suggest that commensal behavior of S. mitis strains might be associated not simply with the absence of virulence-associated gene clusters, but rather with different profiles of expression of virulence functions (Donati et al., 2010; Kilian et al., 2014; Skov Sorensen et al., 2016). In this study, we show structural and expression diversity of PcsB in S. mitis, an immunodominant surface antigen conserved in streptococci and involved in phenotypes associated with bacterial persistence and virulence, including binding to sucrose-derived EPS, biofilm formation, and resistance to complement immunity. We further report polymorphisms in potential epitopes of MHC class II located at conserved PcsB functional domains, indicating antigenic variation. Finally, we established that most strains isolated from systemic infections not only have increased PcsB expression, but virulence phenotypes associated with PcsB molecular functions, when compared to commensal isolates.
PcsB/GbpB proteins are essential for bacterial viability in S. pneumoniae and S. mutans, likely due to their mureinolytic functions as part of the cell wall divisome (Mattos-Graner et al., 2006; Duque et al., 2011; Sham et al., 2011; Bartual et al., 2014). At least for S. mutans, this surface antigen is further involved in stable bacterial interaction with sucrose-derived glucan EPS (Mattos-Graner et al., 2001; Duque et al., 2011; Stipp et al., 2013), which are major components of the extracellular matrix of cariogenic dental biofilms (Bowen et al., 2018). In the present study, we show that diversity in PcsB production in S. mitis is associated with differences in transcriptional activities of pcsB genes. Different atmospheric conditions of growth (aerobiosis versus anaerobiosis) yield similar profiles of pcsB transcription and protein production, further indicating significant influence of the strain background on PcsB expression. These findings highlight the need for studies addressing mechanisms involved in pcsB transcriptional regulation in S. mitis.
In several streptococci of the oral cavity and pharynx, including S. mutans, S. sanguinis, and S. pneumoniae, gbpB/pcsB are directly regulated by the two-component system (TCS) VicRK (Ng et al., 2005; Senadheera et al., 2005; Stipp et al., 2013; Moraes et al., 2014). In these streptococci, VicRK typically regulates genes involved in virulence-associated functions, e.g., complement evasion, and/or biofilm formation (Ng et al., 2005; Senadheera et al., 2005; Duque et al., 2011; Alves et al., 2017). In S. mutans, several genes of the VicRK regulon, including gtfB, gtfC, and gbpB, are also co-regulated by CovR (Biswas and Biswas, 2006; Stipp et al., 2013), another regulator of virulence. CovR was characterized in more detail in S. pyogenes, as part of the TCS CovRS (Federle and Scott, 2002; Gryllos et al., 2007; Horstmann et al., 2017), but in S. mutans and in other oral species, CovR is an orphan regulator (Mattos-Graner and Duncan, 2017). In S. pyogenes, natural mutations affecting CovR activities were associated with increased expression of virulence-associated functions (Engleberg et al., 2001; Sumby et al., 2006). Orthologs of the TCS VicRK and CovR were found in the genome of S. mitis strain B6 (Mattos-Graner and Duncan, 2017). Thus, analysis of these transcriptional regulators in S. mitis strains might help to understand diversity in pcsB transcription in this species, as well as strain-specific virulence phenotypes.
GtfB, GtfC, and GtfD enzymes secreted by S. mutans account for biofilm enrichment through the synthesis of highly stable EPS (mostly insoluble glucan rich in α-1,3 glycosidic linkages) (Ooshima et al., 2001; Banas and Vickerman, 2003; Bowen and Koo, 2011). These enzymes retain activity once bound to the surface of bacterial species that typically co-habit dental biofilms (Bowen and Koo, 2011). Different from S. mutans and other streptococcal species of dental biofilms, the genomes of S. mitis strains do not harbor genes encoding Gtfs (Xu et al., 2018). This is consistent with the reduced S. mitis aggregation observed in BHI supplemented with sucrose, a phenotype also reported in S. mutans gtfBCD isogenic mutants (Alves et al., 2016). Because S. mitis strains are frequently detected during initial phases of biofilm formation (Diaz et al., 2006; Heller et al., 2016), as well as in cariogenic biofilms, which frequently harbor S. mutans (Nyvad and Kilian, 1990; Aas et al., 2008), we hypothesized that S. mitis expressing PcsB could interact with glucan EPS produced by GtfB/C/D secreted by S. mutans. S. mitis aggregation phenotypes were observed in the presence of S. mutans MT8148-secreted enzymes in BHI-sucrose medium (culture supernatants), but not in the presence of culture supernatants of the ΔgtfBCD isogenic S. mutans strain revealed that S. mitis not only interacts with EPS produced by S. mutans-secreted Gtfs, but that strains expressing increased levels of PcsB have enhanced capacity to bind these EPS and to form biofilms. S. mitis binding to EPS synthesized in the presence of S. mutans Gtfs was further associated with reduced levels of C3b deposition.
We have recently reported that S. mitis strains isolated from systemic infections have increased resistance to complement deposition, compared to oral strains (Alves et al., 2019), but differences in complement resistance could not be associated with the presence of the cps operon for the synthesis of capsule, as most of the strains harbored cps orthologs (Kilian et al., 2014; Skov Sorensen et al., 2016). Capsule expression is a major function of S. pneumoniae involved in complement evasion (Hyams et al., 2011), but differences in capsule composition and structure affect their protective functions (Whatmore et al., 2000; Hyams et al., 2013; Rukke et al., 2014; Lessa et al., 2018). In addition, S. mitis strains may co-express conserved or strain-specific gene orthologs potentially involved in complement evasion (e.g., lytA, pepO, eno, gapdh) (Agarwal et al., 2013; Lessa et al., 2018; Pimenta et al., 2018). Although capsule diversity, as well as expression of potential complement evasion genes remain to be investigated in the studied S. mitis strains, the present findings reveal an additional mechanism of S. mitis complement evasion. The findings that increased expression of PcsB by S. mitis promotes biofilm formation and complement evasion in a fashion dependent on interaction with S. mutans-derived components (i.e., EPS produced by S. mutans secreted enzymes) further exemplify how inter-species interactions could promote virulence phenotypes in commensal streptococci with incomplete panels of virulence-associated genes.
The role of PcsB in microbial interactions with extracellular components of biofilms implies that immune responses to PcsB could affect ecology of multiple species expressing PcsB orthologs. T epitope/ProPed bioinformatic analysis of GbpB/PcsB revealed five potential epitopes (Figure 2A) within PcsB functional domains conserved within several streptococcal species of the oral cavity and pharynx (Figure 3). The significant number of epitopes in the N-terminal part of PcsB is further consistent with prominent salivary IgA reactions with N-terminal linear peptides derived from S. mutans GbpB (Nogueira et al., 2008; Smith and Mattos-Graner, 2008). Cross-reactions of S. mutans and S. mitis antigens with salivary IgA were suggested in previous studies (Cole et al., 1999; Nogueira et al., 2012), although identities of the cross-reactive antigens remained unknown. Interestingly, S. mitis seems to also induce cross-reactive T CD4+ cell effector responses to S. pneumoniae (Engen et al., 2014). Our present report further indicates that pioneer colonization by S. mitis strains may prime cross-reactive adaptive responses to PcsB expressed by later colonizers. All the 12 studied strains isolated from the oral mucosa of young infants produced detectable levels of PcsB, thus would be capable of stimulating PcsB reactive IgA responses. Because several commensal species including S. mitis produce IgA1 proteases, which likely accounts for evasion to IgA-S effector functions (Kilian et al., 1995, 2008), it could be speculated that IgA antibody responses induced by commensal streptococci against PcsB would have a more prominent impact on the establishment of species that do not express these proteases, as is the case of S. mutans. In prospective studies of infants, natural salivary IgA antibody responses to S. mutans GbpB were evident during the first year of life in most children who were not colonized by this species a year later, whereas early colonized infants showed weak IgA responses to GbpB (Nogueira et al., 2005). Of note, S. mutans is more typically detected in the oral cavity by 19–30 months of age when most primary teeth erupt, the major niche of this species (Caufield et al., 1993; Smith et al., 1998), whereas S. mitis is detected shortly during the initial months of life (Smith et al., 1993). Thus, natural immune responses to GbpB/PcsB appear to affect initial colonization by S. mutans (Nogueira et al., 2005). In addition, experimental immunization of rats with GbpB significantly induces antibody responses to the same epitopes recognized by salivary antibodies of children (Nogueira et al., 2008), and protects animals from S. mutans-induced caries development (Smith et al., 2003). The present findings on S. mitis PscB raise further interest in defining the effects of immune responses to PcsB proteins in oral ecology and host-microbiota homeostasis.
Data Availability Statement
The raw data supporting the conclusions of this manuscript will be made available by the authors, without undue reservation, to any qualified researcher.
Ethics Statement
This study was carried out in accordance with the recommendations of the Ethical Committee of the Piracicaba Dental School, State University of Campinas, SP, Brazil. All subjects gave written informed consent in accordance with the Declaration of Helsinki. The protocol was approved by the Ethical Committee of the Piracicaba Dental School, State University of Campinas, SP, Brazil (proc. no. 055/2010 and 153/2014).
Author Contributions
RM-G, DS, EH-C, LA, and WK conceived and designed the experiments. RM-G, EH-C, DS, and WK conceived and performed bioinformatic analyses. EH-C, JT, and MS isolated the S. mitis strains from infants, and performed phenotypic and genotypic analyses. EH-C and LA performed protein and transcriptional analyses. LA performed biofilm and complement deposition assays. RM-G, DS, JH, EH-C, and LA analyzed and interpreted the data. RM-G, EH-C, and LA wrote the manuscript. All the authors revised the manuscript and approved its final version.
Funding
This study was supported by Fundação de Amparo à Pesquisa do Estado de São Paulo (FAPESP; grants no. 2012/50966-6, 2015/12940-3, and 2018/02054-4). This study was also partially funded by the Coordenação de Aperfeiçoamento de Pessoal de Nível Superior – Brasil (CAPES) – Finance Code 001. LA was supported by FAPESP (fellowships no. 2012/04222-5, 2015/07237-1, and 2017/19899-4 to LA). EH-C was supported by FAPESP (fellowship no. 2009/50547-0) and by CAPES (fellowship PNPD – 2013).
Conflict of Interest
The authors declare that the research was conducted in the absence of any commercial or financial relationships that could be construed as a potential conflict of interest.
Acknowledgments
We thank Dr. Mogens Kilian (Aarhus University, Aarhus, Denmark) for providing some of the S. mitis strains analyzed in this study, and Dr. Kazuhiko Nakano (Osaka University, Japan) for providing the S. mutans strains MT8148 and BC7s. We thank Dr. Rafael N. Stipp for his help during construction of PcsB recombinant protein and for helpful discussions. We also thank Sebastien Santini, CNRS/AMU IGS UMR7256, for managing the Phylogeny.fr platform used in these analyses.
Footnotes
1. https://www.ncbi.nlm.nih.gov/genome/genomes/
3. http://www.ebi.ac.uk/Tools/msa/clustalw2/
4. https://embnet.vital-it.ch/software/BOX_form.html
References
Aas, J. A., Griffen, A. L., Dardis, S. R., Lee, A. M., Olsen, I., Dewhirst, F. E., et al. (2008). Bacteria of dental caries in primary and permanent teeth in children and young adults. J. Clin. Microbiol. 46, 1407–1417. doi: 10.1128/JCM.01410-07
Aas, J. A., Paster, B. J., Stokes, L. N., Olsen, I., and Dewhirst, F. E. (2005). Defining the normal bacterial flora of the oral cavity. J. Clin. Microbiol. 43, 5721–5732. doi: 10.1128/JCM.43.11.5721-5732.2005
Agarwal, V., Kuchipudi, A., Fulde, M., Riesbeck, K., Bergmann, S., and Blom, A. M. (2013). Streptococcus pneumoniae endopeptidase O (PepO) is a multifunctional plasminogen- and fibronectin-binding protein, facilitating evasion of innate immunity and invasion of host cells. J. Biol. Chem. 288, 6849–6863. doi: 10.1074/jbc.M112.405530
Ajdić, D., McShan, W. M., McLaughlin, R. E., Savić, G., Chang, J., Carson, M. B., et al. (2002). Genome sequence of Streptococcus mutans UA159, a cariogenic dental pathogen. Proc. Natl. Acad. Sci. USA 99, 14434–14439. doi: 10.1073/pnas.172501299
Alves, L. A., de Carli, T. R., Harth-Chu, E. N., Mariano, F. S., Hofling, J. F., Stipp, R. N., et al. (2019). Oral streptococci show diversity in resistance to complement immunity. J. Med. Microbiol. 68, 600–608. doi: 10.1099/jmm.0.000955
Alves, L. A., Harth-Chu, E. N., Palma, T. H., Stipp, R. N., Mariano, F. S., Hofling, J. F., et al. (2017). The two-component system VicRK regulates functions associated with Streptococcus mutans resistance to complement immunity. Mol Oral Microbiol 32, 419–431. doi: 10.1111/omi.12183
Alves, L. A., Nomura, R., Mariano, F. S., Harth-Chu, E. N., Stipp, R. N., Nakano, K., et al. (2016). CovR regulates Streptococcus mutans susceptibility to complement immunity and survival in blood. Infect. Immun. 84, 3206–3219. doi: 10.1128/IAI.00406-16
Banas, J. A., and Vickerman, M. M. (2003). Glucan-binding proteins of the oral streptococci. Crit. Rev. Oral Biol. Med. 14, 89–99. doi: 10.1177/154411130301400203
Bartual, S. G., Straume, D., Stamsas, G. A., Munoz, I. G., Alfonso, C., Martinez-Ripoll, M., et al. (2014). Structural basis of PcsB-mediated cell separation in Streptococcus pneumoniae. Nat. Commun. 5:3842. doi: 10.1038/ncomms4842
Bek-Thomsen, M., Tettelin, H., Hance, I., Nelson, K. E., and Kilian, M. (2008). Population diversity and dynamics of Streptococcus mitis, Streptococcus oralis, and Streptococcus infantis in the upper respiratory tracts of adults, determined by a nonculture strategy. Infect. Immun. 76, 1889–1896. doi: 10.1128/IAI.01511-07
Biswas, S., and Biswas, I. (2006). Regulation of the glucosyltransferase (gtfBC) operon by CovR in Streptococcus mutans. J. Bacteriol. 188, 988–998. doi: 10.1128/JB.188.3.988-998.2006
Bochud, P. Y., Eggiman, P., Calandra, T., Van Melle, G., Saghafi, L., and Francioli, P. (1994). Bacteremia due to viridans streptococcus in neutropenic patients with cancer: clinical spectrum and risk factors. Clin. Infect. Dis. 18, 25–31. doi: 10.1093/clinids/18.1.25
Bowen, W. H., Burne, R. A., Wu, H., and Koo, H. (2018). Oral biofilms: pathogens, matrix, and polymicrobial interactions in microenvironments. Trends Microbiol. 26, 229–242. doi: 10.1016/j.tim.2017.09.008
Bowen, W. H., and Koo, H. (2011). Biology of Streptococcus mutans-derived glucosyltransferases: role in extracellular matrix formation of cariogenic biofilms. Caries Res. 45, 69–86. doi: 10.1159/000324598
Camargo, T. M., Stipp, R. N., Alves, L. A., Harth-Chu, E. N., Höfling, J. F., and Mattos-Graner, R. O. (2018). Novel two-component system of Streptococcus sanguinis affecting functions associated with viability in saliva and biofilm formation. Infect. Immun. 86, 1–19. doi: 10.1128/IAI.00942-17
Caufield, P. W., Cutter, G. R., and Dasanayake, A. P. (1993). Initial acquisition of mutans streptococci by infants: evidence for a discrete window of infectivity. J. Dent. Res. 72, 37–45. doi: 10.1177/00220345930720010501
Cole, M. F., Bryan, S., Evans, M. K., Pearce, C. L., Sheridan, M. J., Sura, P. A., et al. (1999). Humoral immunity to commensal oral bacteria in human infants: salivary secretory immunoglobulin a antibodies reactive with Streptococcus mitis biovar 1, Streptococcus oralis, Streptococcus mutans, and Enterococcus faecalis during the first two years of life. Infect. Immun. 67, 1878–1886.
Dereeper, A., Audic, S., Claverie, J. M., and Blanc, G. (2010). BLAST-EXPLORER helps you building datasets for phylogenetic analysis. BMC Evol. Biol. 12:8. doi: 10.1186/1471-2148-10-8
Dereeper, A., Guignon, V., Blanc, G., Audic, S., Buffet, S., Chevenet, F., et al. (2008). Phylogeny.fr: robust phylogenetic analysis for the non-specialist. Nucleic Acids Res. 1, W465–W469. doi: 10.1093/nar/gkn180
Diaz, P. I., Chalmers, N. I., Rickard, A. H., Kong, C., Milburn, C. L., Palmer, R. J., et al. (2006). Molecular characterization of subject-specific oral microflora during initial colonization of enamel. Appl. Environ. Microbiol. 72, 2837–2848. doi: 10.1128/AEM.72.4.2837-2848.2006
Donati, C., Hiller, N. L., Tettelin, H., Muzzi, A., Croucher, N. J., Angiuoli, S. V., et al. (2010). Structure and dynamics of the pan-genome of Streptococcus pneumoniae and closely related species. Genome Biol. 11:R107. doi: 10.1186/gb-2010-11-10-r107
Duque, C., Stipp, R. N., Wang, B., Smith, D. J., Hofling, J. F., Kuramitsu, H. K., et al. (2011). Downregulation of GbpB, a component of the VicRK regulon, affects biofilm formation and cell surface characteristics of Streptococcus mutans. Infect. Immun. 79, 786–796. doi: 10.1128/IAI.00725-10
Engen, S. A., Valen, R. H., Becattini, S., Jarrossay, D., Blix, I. J., Petersen, F. C., et al. (2014). The oral commensal Streptococcus mitis shows a mixed memory Th cell signature that is similar to and cross-reactive with Streptococcus pneumoniae. PLoS One 9:e104306. doi: 10.1371/journal.pone.0104306
Engleberg, N. C., Heath, A., Miller, A., Rivera, C., and DiRita, V. J. (2001). Spontaneous mutations in the CsrRS two-component regulatory system of Streptococcus pyogenes result in enhanced virulence in a murine model of skin and soft tissue infection. J. Infect. Dis. 183, 1043–1054. doi: 10.1086/319291
Facklam, R. (2002). What happened to the streptococci: overview of taxonomic and nomenclature changes. Clin. Microbiol. Rev. 15, 613–630. doi: 10.1128/CMR.15.4.613-630.2002
Federle, M. J., and Scott, J. R. (2002). Identification of binding sites for the group a streptococcal global regulator CovR. Mol. Microbiol. 43, 1161–1172. doi: 10.1046/j.1365-2958.2002.02810.x
Garnier, F., Gerbaud, G., Courvalin, P., and Galimand, M. (1997). Identification of clinically relevant viridans group streptococci to the species level by PCR. J. Clin. Microbiol. 35, 2337–2341.
Giefing-Kröll, C., Jelencsics, K. E., Reipert, S., and Nagy, E. (2011). Absence of pneumococcal PcsB is associated with overexpression of LysM domain-containing proteins. Microbiology 157, 1897–1909.
Giefing, C., Meinke, A. L., Hanner, M., Henics, T., Bui, M. D., Gelbmann, D., et al. (2008). Discovery of a novel class of highly conserved vaccine antigens using genomic scale antigenic fingerprinting of pneumococcus with human antibodies. J. Exp. Med. 205, 117–131. doi: 10.1084/jem.20071168
Gryllos, I., Grifantini, R., Colaprico, A., Jiang, S., Deforce, E., Hakansson, A., et al. (2007). Mg(2+) signalling defines the group a streptococcal CsrRS (CovRS) regulon. Mol. Microbiol. 65, 671–683. doi: 10.1111/j.1365-2958.2007.05818.x
Heller, D., Helmerhorst, E. J., Gower, A. C., Siqueira, W. L., Paster, B. J., and Oppenheim, F. G. (2016). Microbial diversity in the early in vivo-formed dental biofilm. Appl. Environ. Microbiol. 82, 1881–1888. doi: 10.1128/AEM.03984-15
Horstmann, N., Sahasrabhojane, P., Yao, H., Su, X., and Shelburne, S. A. (2017). Use of a phosphorylation site mutant to identify distinct modes of gene repression by the control of virulence regulator (CovR) in Streptococcus pyogenes. J. Bacteriol. 199, 1–16. doi: 10.1128/JB.00835-16
Human Microbiome Project (2012). Structure, function and diversity of the healthy human microbiome. Nature 486, 207–214. doi: 10.1038/nature11234
Hyams, C., Opel, S., Hanage, W., Yuste, J., Bax, K., Henriques-Normark, B., et al. (2011). Effects of Streptococcus pneumoniae strain background on complement resistance. PLoS One 6:e24581. doi: 10.1371/journal.pone.0024581
Hyams, C., Trzcinski, K., Camberlein, E., Weinberger, D. M., Chimalapati, S., Noursadeghi, M., et al. (2013). Streptococcus pneumoniae capsular serotype invasiveness correlates with the degree of factor H binding and opsonization with C3b/iC3b. Infect. Immun. 81, 354–363. doi: 10.1128/IAI.00862-12
Johnston, C., Hinds, J., Smith, A., van der Linden, M., Van, E. J., and Mitchell, T. J. (2010). Detection of large numbers of pneumococcal virulence genes in streptococci of the mitis group. J. Clin. Microbiol. 48, 2762–2769. doi: 10.1128/JCM.01746-09
Kilian, M., Husby, S., Host, A., and Halken, S. (1995). Increased proportions of bacteria capable of cleaving IgA1 in the pharynx of infants with atopic disease. Pediatr. Res. 38, 182–186. doi: 10.1203/00006450-199508000-00008
Kilian, M., Poulsen, K., Blomqvist, T., Havarstein, L. S., Bek-Thomsen, M., Tettelin, H., et al. (2008). Evolution of Streptococcus pneumoniae and its close commensal relatives. PLoS One 3:e2683. doi: 10.1371/journal.pone.0002683
Kilian, M., Riley, D. R., Jensen, A., Bruggemann, H., and Tettelin, H. (2014). Parallel evolution of Streptococcus pneumoniae and to pathogenic and mutualistic lifestyles. MBio 5, e01490–e01414. doi: 10.1128/mBio.01490-14
Kononen, E., Jousimies-Somer, H., Bryk, A., Kilp, T., and Kilian, M. (2002). Establishment of streptococci in the upper respiratory tract: longitudinal changes in the mouth and nasopharynx up to 2 years of age. J. Med. Microbiol. 51, 723–730. doi: 10.1099/0022-1317-51-9-723
Lessa, F. C., Milucky, J., Rouphael, N. G., Bennett, N. M., Talbot, H. K., and Harrison, L. H. (2018). Streptococcus mitis expressing pneumococcal serotype 1 capsule. Sci. Rep. 8:17959. doi: 10.1038/s41598-018-35921-3
Mattos-Graner, R. O., and Duncan, M. J. (2017). Two-component signal transduction systems in oral bacteria. J. Oral Microbiol. 9:1400858. doi: 10.1080/20002297.2017.1400858
Mattos-Graner, R. O., Jin, S., King, W. F., Chen, T., Smith, D. J., and Duncan, M. J. (2001). Cloning of the Streptococcus mutans gene encoding glucan binding protein B and analysis of genetic diversity and protein production in clinical isolates. Infect. Immun. 69, 6931–6941. doi: 10.1128/IAI.69.11.6931-6941.2001
Mattos-Graner, R. O., Porter, K. A., Smith, D. J., Hosogi, Y., and Duncan, M. J. (2006). Functional analysis of glucan binding protein B from Streptococcus mutans. J. Bacteriol. 188, 3813–3825. doi: 10.1128/JB.01845-05
Mitchell, J. (2011). Streptococcus mitis: walking the line between commensalism and pathogenesis. Mol Oral Microbiol 26, 89–98. doi: 10.1111/j.2041-1014.2010.00601.x
Moraes, J. J., Stipp, R. N., Harth-Chu, E. N., Camargo, T. M., Hofling, J. F., and Mattos-Graner, R. O. (2014). Two-component system VicRK regulates functions associated with establishment of Streptococcus sanguinis in biofilms. Infect. Immun. 82, 4941–4951. doi: 10.1128/IAI.01850-14
Nakano, K., Matsumura, M., Kawaguchi, M., Fujiwara, T., Sobue, S., Nakagawa, I., et al. (2002). Attenuation of glucan-binding protein C reduces the cariogenicity of Streptococcus mutans: analysis of strains isolated from human blood. J. Dent. Res. 81, 376–379. doi: 10.1177/0810376
Ng, W. L., Robertson, G. T., Kazmierczak, K. M., Zhao, J., Gilmour, R., and Winkler, M. E. (2003). Constitutive expression of PcsB suppresses the requirement for the essential VicR (YycF) response regulator in Streptococcus pneumoniae R6. Mol. Microbiol. 50, 1647–1663. doi: 10.1046/j.1365-2958.2003.03806.x
Ng, W. L., Tsui, H. C., and Winkler, M. E. (2005). Regulation of the pspA virulence factor and essential pcsB murein biosynthetic genes by the phosphorylated VicR (YycF) response regulator in Streptococcus pneumoniae. J. Bacteriol. 187, 7444–7459. doi: 10.1128/JB.187.21.7444-7459.2005
Nogueira, R. D., Alves, A. C., Napimoga, M. H., Smith, D. J., and Mattos-Graner, R. O. (2005). Characterization of salivary immunoglobulin a responses in children heavily exposed to the oral bacterium Streptococcus mutans: influence of specific antigen recognition in infection. Infect. Immun. 73, 5675–5684. doi: 10.1128/IAI.73.9.5675-5684.2005
Nogueira, R. D., King, W. F., Gunda, G., Culshaw, S., Taubman, M. A., Mattos-Graner, R. O., et al. (2008). Mutans streptococcal infection induces salivary antibody to virulence proteins and associated functional domains. Infect. Immun. 76, 3606–3613. doi: 10.1128/IAI.00214-08
Nogueira, R. D., Sesso, M. L., Borges, M. C., Mattos-Graner, R. O., Smith, D. J., and Ferriani, V. P. (2012). Salivary IgA antibody responses to Streptococcus mitis and Streptococcus mutans in preterm and fullterm newborn children. Arch. Oral Biol. 57, 647–653. doi: 10.1016/j.archoralbio.2011.11.011
Nyvad, B., and Kilian, M. (1990). Comparison of the initial streptococcal microflora on dental enamel in caries-active and in caries-inactive individuals. Caries Res. 24, 267–272. doi: 10.1159/000261281
Ooshima, T., Matsumura, M., Hoshino, T., Kawabata, S., Sobue, S., and Fujiwara, T. (2001). Contributions of three glycosyltransferases to sucrose-dependent adherence of Streptococcus mutans. J. Dent. Res. 80, 1672–1677. doi: 10.1177/00220345010800071401
Palma, T. H., Harth-Chu, E. N., Scott, J., Stipp, R. N., Boisvert, H., Salomão, M. F., et al. (2016). Oral cavities of healthy infants harbour high proportions of Streptococcus salivarius strains with phenotypic and genotypic resistance to multiple classes of antibiotics. J. Med. Microbiol. 65, 1456–1464. doi: 10.1099/jmm.0.000377
Pimenta, F., Gertz, R. E. Jr., Park, S. H., Kim, E., Moura, I., Milucky, J., et al. (2018). Streptococcus infantis, Streptococcus mitis, and Streptococcus oralis strains with highly similar cps5 loci and antigenic relatedness to serotype 5 pneumococci. Front. Microbiol. 9:3199. doi: 10.3389/fmicb.2018.03199
Rukke, H. V., Kalluru, R. S., Repnik, U., Gerlini, A., José, R. J., Periselneris, J., et al. (2014). Protective role of the capsule and impact of serotype 4 switching on Streptococcus mitis. Infect. Immun. 82, 3790–3801. doi: 10.1128/IAI.01840-14
Senadheera, M. D., Guggenheim, B., Spatafora, G. A., Huang, Y. C., Choi, J., Hung, D. C., et al. (2005). A VicRK signal transduction system in Streptococcus mutans affects gtfBCD, gbpB, and ftf expression, biofilm formation, and genetic competence development. J. Bacteriol. 187, 4064–4076. doi: 10.1128/JB.187.12.4064-4076.2005
Sham, L. T., Barendt, S. M., Kopecky, K. E., and Winkler, M. E. (2011). Essential PcsB putative peptidoglycan hydrolase interacts with the essential FtsXSpn cell division protein in Streptococcus pneumoniae D39. Proc. Natl. Acad. Sci. USA 108, E1061–E1069. doi: 10.1073/pnas.1108323108
Singh, H., and Raghava, G. P. (2001). ProPred: prediction of HLA-DR binding sites. Bioinformatics 17, 1236–1237.
Skov Sorensen, U. B., Yao, K., Yang, Y., Tettelin, H., and Kilian, M. (2016). Capsular polysaccharide expression in commensal streptococcus species: genetic and antigenic similarities to Streptococcus pneumoniae. MBio 7, 1–17. doi: 10.1128/mBio.01844-16
Smith, D. J., Anderson, J. M., King, W. F., van, H. J., and Taubman, M. A. (1993). Oral streptococcal colonization of infants. Oral Microbiol. Immunol. 8, 1–4. doi: 10.1111/j.1399-302X.1993.tb00535.x
Smith, D. J., King, W. F., Akita, H., and Taubman, M. A. (1998). Association of salivary immunoglobulin a antibody and initial mutans streptococcal infection. Oral Microbiol. Immunol. 13, 278–285. doi: 10.1111/j.1399-302X.1998.tb00708.x
Smith, D. J., King, W. F., Barnes, L. A., Peacock, Z., and Taubman, M. A. (2003). Immunogenicity and protective immunity induced by synthetic peptides associated with putative immunodominant regions of Streptococcus mutans glucan-binding protein B. Infect. Immun. 71, 1179–1184. doi: 10.1128/IAI.71.3.1179-1184.2003
Smith, D. J., and Mattos-Graner, R. O. (2008). Secretory immunity following mutans streptococcal infection or immunization. Curr. Top. Microbiol. Immunol. 319, 131–156. doi: 10.1007/978-3-540-73900-5_6
Stipp, R. N., Boisvert, H., Smith, D. J., Hofling, J. F., Duncan, M. J., and Mattos-Graner, R. O. (2013). CovR and VicRK regulate cell surface biogenesis genes required for biofilm formation in Streptococcus mutans. PLoS One 8:e58271. doi: 10.1371/journal.pone.0058271
Stipp, R. N., Goncalves, R. B., Hofling, J. F., Smith, D. J., and Mattos-Graner, R. O. (2008). Transcriptional analysis of gtfB, gtfC, and gbpB and their putative response regulators in several isolates of Streptococcus mutans. Oral Microbiol. Immunol. 23, 466–473. doi: 10.1111/j.1399-302X.2008.00451.x
Sumby, P., Whitney, A. R., Graviss, E. A., DeLeo, F. R., and Musser, J. M. (2006). Genome-wide analysis of group a streptococci reveals a mutation that modulates global phenotype and disease specificity. PLoS Pathog. 2:e5. doi: 10.1371/journal.ppat.0020005
Whatmore, A. M., Efstratiou, A., Pickerill, A. P., Broughton, K., Woodard, G., Sturgeon, D., et al. (2000). Genetic relationships between clinical isolates of Streptococcus pneumoniae, Streptococcus oralis, and Streptococcus mitis: characterization of “atypical” pneumococci and organisms allied to S. mitis harboring S. pneumoniae virulence factor-encoding genes. Infect. Immun. 68, 1374–1382. doi: 10.1128/IAI.68.3.1374-1382.2000
Keywords: Streptococcus mitis, PcsB, GbpB, biofilm, exopolysaccharides, complement immunity, virulence, microbial ecology
Citation: Harth-Chu EN, Alves LA, Theobaldo JD, Salomão MF, Höfling JF, King WF, Smith DJ and Mattos-Graner RO (2019) PcsB Expression Diversity Influences on Streptococcus mitis Phenotypes Associated With Host Persistence and Virulence. Front. Microbiol. 10:2567. doi: 10.3389/fmicb.2019.02567
Edited by:
Awdhesh Kalia, University of Texas MD Anderson Cancer Center, United StatesReviewed by:
Konstantinos Papadimitriou, Agricultural University of Athens, GreeceKoshy Philip, University of Malaya, Malaysia
Copyright © 2019 Harth-Chu, Alves, Theobaldo, Salomão, Höfling, King, Smith and Mattos-Graner. This is an open-access article distributed under the terms of the Creative Commons Attribution License (CC BY). The use, distribution or reproduction in other forums is permitted, provided the original author(s) and the copyright owner(s) are credited and that the original publication in this journal is cited, in accordance with accepted academic practice. No use, distribution or reproduction is permitted which does not comply with these terms.
*Correspondence: Renata O. Mattos-Graner, cm1ncmFuZXJAZm9wLnVuaWNhbXAuYnI=
†These authors have contributed equally to this work