- 1Genome Center, University of California, Davis, Davis, CA, United States
- 2Department of Evolution and Ecology, University of California, Davis, Davis, CA, United States
- 3Department of Medical Microbiology and Immunology, University of California, Davis, Davis, CA, United States
Seagrasses are globally distributed marine flowering plants that are foundation species in coastal ecosystems. Seagrass beds play essential roles as habitats and hatcheries, in nutrient cycling, and in protecting the coastline from erosion. Although many studies have focused on seagrass ecology, only a limited number have investigated their associated fungi. In terrestrial systems, fungi can have beneficial and detrimental effects on plant fitness. However, not much is known about marine fungi and even less is known about seagrass associated fungi. Here we used culture-independent sequencing of the ribosomal internal transcribed spacer (ITS) region to characterize the taxonomic diversity of fungi associated with the seagrass, Zostera marina. We sampled from two Z. marina beds in Bodega Bay over three time points to investigate fungal diversity within and between plants. Our results indicate that there are many fungal taxa for which a taxonomic assignment cannot be made living on and inside Z. marina leaves, roots and rhizomes and that these plant tissues harbor distinct fungal communities. We also identified differences in the abundances of the orders, Glomerellales, Agaricales and Malasseziales, between seagrass tissues. The most prevalent ITS amplicon sequence variants (ASVs) associated with Z. marina tissues could not initially be confidently assigned to a fungal phylum, but shared significant sequence similarity with Chytridiomycota and Aphelidomycota. To obtain a more definitive taxonomic classification of the most abundant ASV associated with Z. marina leaves, we used PCR with one primer targeting a unique region of this ASV’s ITS2 and a second primer targeting fungal 28S rRNA genes to amplify part of the 28S rRNA gene region corresponding to this ASV. Sequencing and phylogenetic analysis of the resulting partial 28S rRNA gene revealed that the organism that this ASV comes from is a member of Novel Clade SW-I in the order Lobulomycetales in the phylum Chytridiomycota. This clade includes known parasites of freshwater diatoms and algae and it is possible this chytrid is directly infecting Z. marina leaf tissues. This work highlights a need for further studies focusing on marine fungi and the potential importance of these understudied communities to the larger seagrass ecosystem.
Introduction
Seagrasses are fully submerged marine flowering plants (angiosperms) that play essential roles in marine ecosystems as foundation species. Although angiosperms are the most diverse terrestrial plant group with over 250,000 species, there are only around 70 species of seagrasses. There are three main lineages of seagrass in the order Alismatales that separately moved into and adapted to the marine ecosystem through convergent evolution between 70 and 100 million years ago (Les et al., 1997; Wissler et al., 2011). Seagrasses are important keystone species in most coastal environments around the world with ecosystem services comparable to those of tropical rainforests (Costanza et al., 1997). However, seagrass beds are increasingly impacted by climate change, pollution and habitat fragmentation and restoration is expensive and has a low success rate (Orth et al., 2006). Although many studies have focused on the ecological importance of seagrasses, relatively little morphological, or culture-independent work has been performed investigating the fungi associated with these species.
Fungi are known to affect land plant fitness both in detrimental ways (e.g., as pathogens) and beneficial ways [e.g., mycorrhizae are involved in facilitating phosphorus and nitrogen uptake for their plant hosts (Bonfante and Anca, 2009)]. It is estimated that 82–85% of angiosperm species have mycorrhizal fungal associations (Wang and Qiu, 2006; Radhika and Rodrigues, 2007). Mycorrhizae were previously thought to not colonize aquatic environments, but have since been found in wetlands, estuaries, mangrove forests, and freshwater ecosystems (Beck-Nielsen and Madsen, 2001; Bohrer et al., 2004; Šraj-Kržič et al., 2006; Radhika and Rodrigues, 2007; Kohout et al., 2012). Mycorrhizal associations are believed to be at least 400 million years old, critical for plant terrestrialization (Redecker et al., 2000; Humphreys et al., 2010; Wang et al., 2010) and the ancestral state for angiosperms (Brundrett, 2002). Despite their importance to land plants, seagrasses are believed to not form mycorrhizal associations (Nielsen et al., 1999), even though freshwater relatives of seagrasses have been observed to sporadically form mycorrhizal associations (Clayton and Bagyaraj, 1984; Wigand and Court Stevenson, 1994; Khan and Belik, 1995; Beck-Nielsen and Madsen, 2001; Šraj-Kržič et al., 2006; Wang and Qiu, 2006; Radhika and Rodrigues, 2007; Brundrett, 2009).
In contrast to their apparent lack of mycorrhizal associations, some studies of seagrasses have observed associations with novel fungal endophytes similar to dark septate endophytes (DSE) common in land plants (Torta et al., 2015; Vohník et al., 2015, 2016, 2017, 2019; Borovec and Vohník, 2018). DSE are a morphology based type and not a phylogenetic group, and are largely uncharacterized. In some cases, DSEs have been shown to transfer nitrogen and receive carbon from plants as well as increase overall plant nutrient content and growth (Usuki and Narisawa, 2007; Porras-Alfaro and Bayman, 2011). DSEs are not the only fungi that have been observed to form associations with seagrasses. Additional culture based studies have found fungi associated with the leaves, roots and rhizomes of different seagrasses; however, many of these studies often conflict on the prevalence and taxonomic identities of the fungi observed and suffer from the limitations of culture-based methods (Newell, 1981; Kuo, 1984; Cuomo et al., 1985; Devarajan and Suryanarayanan, 2002; Sakayaroj et al., 2010; Mata and Cebrián, 2013; Panno et al., 2013; Shoemaker and Wyllie-Echeverria, 2013; Supaphon et al., 2013, 2017; Gnavi et al., 2014; Phongpaichit and Supaphon, 2014; Kirichuk and Pivkin, 2015; Ling et al., 2015; Torta et al., 2015; Venkatachalam, 2015; Venkatachalam et al., 2015; Vohník et al., 2016). This is compounded by the fact that marine fungi are understudied and that there are only ∼1100 currently accepted species despite estimates that true diversity is much higher, at 10,000 or more species (Jones and Gareth Jones, 2011; Jones et al., 2015; Amend et al., 2019). Here, we use culture-independent methods to characterize the taxonomic diversity of fungi associated with the seagrass, Zostera marina, in order to build a framework from which we can begin to determine the evolutionary, ecological, and functional importance of these associations.
Materials and Methods
Sample Collection
Roots, leaves, and rhizome tissues from individual Z. marina plants and adjacent sediment were collected by coring into the sediment using a 2.375 inch diameter modified PVC pipe (McMaster-Carr, Elmhurst, IL, United States). PVC pipes were modified such that one end of the pipe was cut at an angle to make insertion into the sediment easier. Samples were collected from two sites in Bodega Bay, CA, Gaffney Point (GPS: 38°18′45.05″N, 123° 3′16.66″W) and Westside Point (GPS: 38°19′10.67″N, 123° 3′13.71″W), at three timepoints 2 weeks apart spanning July to August 2016. Samples from GP were collected only at the first timepoint. Samples were dissected using sterile scissors into different bulk sample types (e.g., roots, leaves, and rhizome tissues) in the field, placed into 1.5 mL centrifuge tubes which were immediately placed on dry ice for transport and then kept at −80°C until analyzed. Although samples were obtained from one individual plant, we refer to them here as bulk samples because they represent multiple leaves, roots or rhizome tissues from these plants. Sediment was collected from the 2.375 inch PVC pipe using an 11 mm diameter straw which was placed directly next to the individual seagrass shoot tissue in order to assure sediment was collected as close to the plant as possible. For the third timepoint (T3), multiple samples were collected from the same plant. Individual leaf blades were cut into ∼5 inch long segments moving along the length of the leaf tissue, individual roots were collected, and an additional 7 mm diameter straw core was obtained and then dissected into ∼1.5 cm sections of sediment. Leaf segment lengths are measured in distance from the surface of the sediment (e.g., 10–15 inches indicates that the segment started ∼10 inches above the sediment). Sediment dissections are also measured in distance from the surface of the sediment. Cores of unvegetated sediment from between sites were obtained as a biological control at each time point. For sample sizes, refer to Supplementary Tables S1–S3.
Molecular Methods
DNA was extracted from samples with the PowerSoil DNA Isolation kit (MO BIO Laboratories, Inc., Carlsbad, CA, United States) with minor changes to the manufacturer’s protocol as follows. To improve fungal lysis, samples were heated at 70°C for 10 min between steps 4 and 5 as suggested by the manufacturer. For step 5, samples were bead beaten on the homogenize setting for 1 min using a BioSpec Products mini-bead beater. Samples were placed into randomized blocks prior to extraction using a random number generator.
In order to obtain loosely associated, or epiphytic, fungi, tissue samples were washed in 1 mL of a 1:50 dilution of Redford buffer solution (1 M Tris–HCL, 0.5 M NaEDTA, 1.2% Triton-X) (Kadivar and Stapleton, 2003; Kembel et al., 2014). Samples were left in this solution for 5 min during which they were periodically vortexed. Tissues were then removed and placed into a new microcentrifuge tube for use in endophyte analyses. The epiphyte wash solution was centrifuged at 4000 g for 20 min and the pellets were resuspended in MoBio PowerSoil C1 solution, the first step in the DNA extraction process.
After being washed to obtain epiphytes, a subset of samples collected at the third sampling timepoint were further surface cleaned with the goal of removing any leftover loosely associated fungi. This was done in an effort to obtain samples containing only endophytes, which we define here as being tightly associated to the outside or inside the tissue. Tissues were rinsed with autoclaved nanopure water (30 s), immersed in 95% ethanol (5 s), immersed in 0.5% NaOCl (∼10% bleach) (2 min), immersed in 70% ethanol (2 min), and then rinsed with autoclaved nanopure water (1 min) three times. Tissues were then placed into MoBio PowerSoil C1 solution and crushed using flame sterilized tweezers.
Sequence Generation
Using a random number generator, samples were randomly assigned places in 96-well plates and sent to Zymo Research, Inc. for sequencing via their ZymoBIOMICS Service. We briefly summarize their protocol here. Fungal ITS2 amplicon sequencing was performed using the Quick NGS Library Preparation Kit (Zymo Research, Irvine, CA, United States). The ITS2 region was amplified via PCR using the ITS5.8S_Fun and ITS4_Fun (McHugh and Schwartz, 2015; Taylor et al., 2016) primers which were chosen to try to minimize the amount of host plant amplification. Final PCR products were quantified with qPCR fluorescence readings and pooled together based on equal molarity. The final pooled library was cleaned up with Select-a-Size DNA Clean & ConcentratorTM (Zymo Research, Irvine, CA, United States), then quantified with TapeStation (Agilent, Santa Clara, CA, United States) and Qubit (Invitrogen, Carlsbad, CA, United States). The final library was sequenced on Illumina MiSeq (Illumina, Inc., San Diego, CA, United States) with a v3 reagent kit (600 cycles) to generate 300 bp paired-end reads. The sequencing was performed with >10% PhiX spike-in. Sequence reads were demultiplexed by Zymo Research, Inc. using the MiSeq Reporter. The raw sequence reads generated for this ITS amplicon project were deposited at GenBank under BioProject ID PRJNA515720.
Sequence Processing
Primers were removed using cudadapt (v. 1.14) (Martin, 2011). Forward and reverse reads were then merged with PEAR (v. 0.9.5) (Zhang et al., 2014) using the script run_pear.pl (Comeau et al., 2017) which implements PEAR in parallel.
The resulting merged fastq files were analyzed in R (v. 3.5.1) using dada2 (v. 1.10.0), decontam (v. 1.1.2), phyloseq (v. 1.26.0), vegan (v. 2.5.5), FSA (v. 0.8.25), and ggplot2 (v. 3.1.0) (Dixon, 2003; Wickham, 2009; McMurdie and Holmes, 2013; Callahan et al., 2016; Ogle, 2016; R Core Team, 2016; Davis et al., 2018). For a detailed walkthrough of the following analysis using R, see the R-markdown summary file (Supplementary Data Sheet S2).
Prior to denoising, reads were truncated at the first quality score of 2 and reads with an expected error greater than 2 were removed. Since PEAR alters the quality scores during sequence merging, we inflated the error profile used by dada2 to denoise sequences by a factor of 3. Reads were then denoised and merged using dada2 to generate a table of amplicon sequence variants (ASVs). Chimeric sequences were identified using removeBimeraDenovo and removed prior to downstream analyses (∼0.31% of sequences). Taxonomy was inferred using the RDP Naive Bayesian Classifier algorithm with a modified UNITE (v. 8.0) database resulting in 2953 ASVs (Wang et al., 2007; UNITE Community, 2019). The UNITE database was modified to include a representative ITS amplicon sequence for the host plant, Z. marina (KM051458.1). ASVs were then named by giving each a unique number preceded by “SV” which stands for sequence variant (e.g., SV1, SV2, etc.).
Subsequently, ITS-x (v. 1.1b) was run on the unique ASVs (Bengtsson-Palme et al., 2013). This program, which uses kingdom-specific hidden markov models to identify the ITS region of sequences, could not detect a fungal ITS region in 1064 of the ASVs. These ASVs were thus considered putively non-fungal and were removed from downstream analysis.
Decontam’s prevalence method was used to identify possible contaminants with a threshold of 0.5 which will identify sequences that have a higher prevalence in negative controls than in true samples. This threshold identified 38 possible contaminants which were then removed from the dataset. Negative and positive controls were subsequently removed at this point in the analysis. Remaining ASVs assigned as non-fungal at the domain level (e.g., ASVs assigned to the host plant, Z. marina, or with no domain level classification) were removed from the dataset prior to downstream analysis resulting in a final table of 1850 ASVs. Four samples were represented by zero sequences after these filtering steps and were subsequently removed.
Sequence Analysis and Visualization
For statistical analysis and visualization, the resulting data was then subset without replacement to an even number of sequences per sample depending on the comparisons being made. As a result, when investigating differences between bulk sample types a depth of 10,000 sequences was selected resulting in the inclusion of 49 samples: leaf epiphytes (n = 13), root epiphytes (n = 14), rhizome epiphytes (n = 7), and sediment (n = 15). When investigating differences across leaf length a number of 5,000 sequences was chosen resulting in the inclusion of 50 samples: leaf epiphytes (n = 25) and leaf endophytes (n = 25). These depths were selected after examining rarefaction curves to balance maximizing the number of sequences per sample while also minimizing the number of samples removed from downstream analysis (Supplementary Figures S1, S2).
To assess within-sample (i.e., alpha) diversity, the observed number of ASVs and the Shannon index of samples were calculated using the estimate_richness function in phyloseq. Kruskal–Wallis tests with 9,999 permutations were used to test for significant differences in alpha diversity between bulk sample types (leaf, root, rhizome, and sediment). For metrics in which the Kruskal–Wallis test resulted in a rejected null hypothesis (p < 0.05), Bonferroni corrected post hoc Dunn tests were performed.
To assess between-sample (i.e., beta), diversity, Bray–Curtis (Bray et al., 1957) dissimilarities were calculated using the ordinate function in phyloseq and visualized using principal coordinates analysis. To test for significant differences in mean centroids between sample categories (e.g., sample type), permutational manovas (PERMANOVAs) were performed with 9,999 permutations and to account for multiple comparisons, p-values were adjusted using the Bonferroni correction (Anderson, 2001). PERMANOVA tests are known to be sensitive to differences in dispersion when using abundance-based distance matrices like Bray-Curtis (Warton et al., 2012), but are still more robust than other tests (Anderson and Walsh, 2013). To control for this, we also tested for differences in mean dispersions between different sample categories using the betadisper and permutest functions from the vegan package in R with 9,999 permutations. For betadisper results that resulted in a rejected null hypothesis (p < 0.05), the post hoc Tukey’s honest significant difference (HSD) test was performed to identify which categories had mean dispersions that were significantly different.
To compare fungal community composition, we collapsed ASVs into taxonomic orders using the tax_glom function in phyloseq and then removed orders with a variance of less than one percent when comparing between sample types and a variance of less than 0.1 percent when comparing across leaf lengths. These variance threshold values were chosen to optimize removing rare orders to avoid both false positives and orders that did not vary between samples, while also retaining enough orders that did vary to investigate differences. The average relative abundance of taxonomic orders was compared between sample types using Bonferroni corrected Kruskal–Wallis tests in R. For orders where the Kruskal–Wallis test resulted in a rejected null hypothesis, Bonferroni corrected post hoc Dunn tests were performed to identify which sample comparisons for each taxonomic order were significantly different.
To examine the contribution of specific ASVs to fungal community composition across leaf length, the dataset was filtered to include only ASVs with a mean abundance of greater than two percent. A mean abundance of two percent or greater was chosen because we were most interested in the contribution of the most abundant ASVs to leaf length, as well as to remove rare and spurious ASVs and thus avoid false positives. The resulting ASVs were then compared to each other and those that shared greater than 99% sequence identity were grouped into what we refer to as “complexes.” Complexes were given a name based on the most abundant ASV in the group (e.g., SV8 complex). The average relative abundance of ASVs and ASV complexes were compared between sample types using Bonferroni corrected Kruskal–Wallis tests in R. For ASVs and ASV complexes where the Kruskal–Wallis test resulted in a rejected null hypothesis, Bonferroni corrected post hoc Dunn tests were performed to identify which sample comparisons for each ASV or ASV complex were significantly different.
Sanger Sequencing
The most prevalent sequences associated with Z. marina leaf tissue all come from a single complex (i.e., they all share >99% sequence identity). This complex, which includes SV8, SV11, SV16 and SV56, has been named the SV8 complex because SV8 is the most abundant member of this group. None of the ASVs in the SV8 complex could be classified into a fungal phylum using currently available databases. In order to attempt to improve taxonomic classification of the SV8 complex, we used PCR to obtain sequence data for part of the 28S rRNA gene from representatives of this complex found in our samples. To obtain this sequence, a SV8-specific primer (5′-GGAGCATGTCTGTTTGAGAA-3′) was designed for the ITS2 region using Primer-BLAST (Ye et al., 2012). This primer was then used in PCR along with the reverse fungal 28S rRNA gene primer, LR3 (Vilgalys and Hester, 1990). PCR using this pair of primers should at least in theory amplify the ITS2 and D1/D2 regions of the 28S rRNA gene for the SV8 complex.
Using these primers, we performed PCR on DNA from two leaf epiphyte samples (sample IDs: 108A and 109A) using Taq DNA Polymerase (QIAGEN, Hilden, Germany) with the following conditions: 95°C for 5 min, 35 cycles at 94°C for 30 s, 52°C for 15 s, 72°C for 1 min, and a final extension at 72°C for 8 min (Kress and Erickson, 2012).
PCR products were purified using the Nucleospin Gel and PCR kit (QIAGEN, Hilden, Germany). The resulting amplicon was sequenced using the Sanger method by the College of Biological Sciences UCDNA Sequencing Facility1. The resulting ABI files were viewed and a consensus sequence was produced using seqtrace following the Swabs to Genomes workflow (Dunitz et al., 2015). Consensus sequences for the PCR products were deposited to Genbank under accession numbers MK994004 and MK994005.
Phylogenetic Reconstruction
Closely related sequences to the sequence from the PCR products above were identified using NCBI’s Standard Nucleotide BLAST’s megablast option with default settings. These results were then used to guide a literature search to identify additional sequences for inclusion during phylogenetic reconstruction (Supplementary Table S4; Simmons et al., 2009; Karpov et al., 2014; Hassett et al., 2017; Rad-Menéndez et al., 2018; Van den Wyngaert et al., 2018).
A sequence alignment of all sequences listed in Supplementary Table S4 was generated using MAFFT (v. 7.402) (Katoh, 2002) with default parameters on the CIPRES Science Gateway web server (Miller et al., 2010). The alignment was trimmed using trimAl (v.1.2) with the -gappyout method (Capella-Gutiérrez et al., 2009). The resulting alignment included 22 sequences and 915 alignment positions. JModelTest2 (v. 2.1.6) was run with default parameters on the CIPRES Science Gateway web server to select a best-fit model of nucleotide substitution for use in phylogenetic analyses (Guindon and Gascuel, 2003; Darriba et al., 2012). The best-fit model based on both the Akaike Information Criterion and Bayesian Information Criterion values was the GTR + G + I evolutionary model.
Using the CIPRES Science Gateway web server, phylogenetic trees were inferred from the trimmed alignment using both Bayesian and maximum likelihood approaches and a GTR + G + I model. Bayesian phylogenetic inference was performed using MrBayes (v. 3.2.2) with four incrementally heated simultaneous Monte Carlo Markov Chains (MCMC) run over 40,000 generations, which was the optimal number of generations required to achieve a stop value of 0.01 or less for the convergence diagnostic (Huelsenbeck and Ronquist, 2001). The first 25% of trees generated were discarded as burn-in and for the remaining trees, a majority rule consensus tree was generated and used to calculate the Bayesian Posterior Probabilities. A maximum likelihood approach was undertaken using RAxML (v. 8) using the -autoMRE option which automatically determines the optimal number of bootstrap replicates (Stamatakis, 2014). The resulting phylogenies were then visualized with FigTree (v. 1.4.2) and annotated in Adobe Photoshop CS6 (Rambaut, 2009).
Results
Fungal Alpha Diversity Differs Between Sample Types
Alpha diversity was significantly different between bulk sample types (K–W test; p < 0.01, Supplementary Figure S3 and Supplementary Table S5) for all diversity metrics. Post hoc Dunn tests identified that the alpha diversity for bulk root and rhizome tissues was consistently lower than the alpha diversity of the sediment (p < 0.05, Supplementary Table S6). This is consistent with previous sequence-based studies of seagrass associated fungi (Hurtado-McCormick et al., 2019). The alpha diversity of the Z. marina mycobiome was found to be much lower than that previously seen for the bacterial communities associated with Z. marina (Ettinger et al., 2017).
Alpha diversity was not significantly different between root epiphytes and endophytes for any metrics (K-W test, p > 0.05, Supplementary Figure S4). However, alpha diversity was significantly different between leaf epiphytes and endophytes when using the Shannon diversity index which incorporates evenness (K-W test, p < 0.05), but was not significantly different when looking at the number of observed ASVs (p > 0.05).
Fungal Community Structure Differs Between and Within Plant Parts
Fungal community structure differed significantly between different bulk sample types (PERMANOVA, p < 0.001, Supplementary Table S7 and Supplementary Figure S5). Subsequent pairwise PERMANOVA test results found that community structure was significantly different for all sample type comparisons (p < 0.05, Supplementary Table S8) except sediment vs. roots and leaves vs. roots (p > 0.05).
We note, however, that within group variance, also known as dispersion, also differed significantly between bulk sample types (betadisper, p < 0.01, Supplementary Table S9), with rhizomes having more dispersion than sediment or roots (Tukey HSD, p < 0.01, Supplementary Table S10). PERMANOVA results can be confounded by dispersion differences when not using a balanced design with equal sample numbers for categories being compared. In such instances, a significant PERMANOVA result is unable to distinguish between differences in mean dispersions, and differences in mean centroids. Thus, these results must be interpreted with circumspection.
Fungal community structure also differed significantly between leaf epiphyte and endophyte communities (PERMANOVA, p < 0.001, Supplementary Table S11 and Figure 1) and between leaf length segments (p < 0.05). Pair-wise PERMANOVA test results found that the first five inches of leaves had significantly different community structure from the last fifteen inches (p < 0.01, Supplementary Table S12). No significant dispersion differences were detected between the community structure of epiphytes and endophytes (betadisper, p > 0.05, Supplementary Table S13) and the differences in mean dispersions between leaf length segments was barely non-significant (p = 0.052). The first five inches of leaves had dispersions that differed significantly from the last ten inches (Tukey HSD, p < 0.05, Supplementary Table S14).
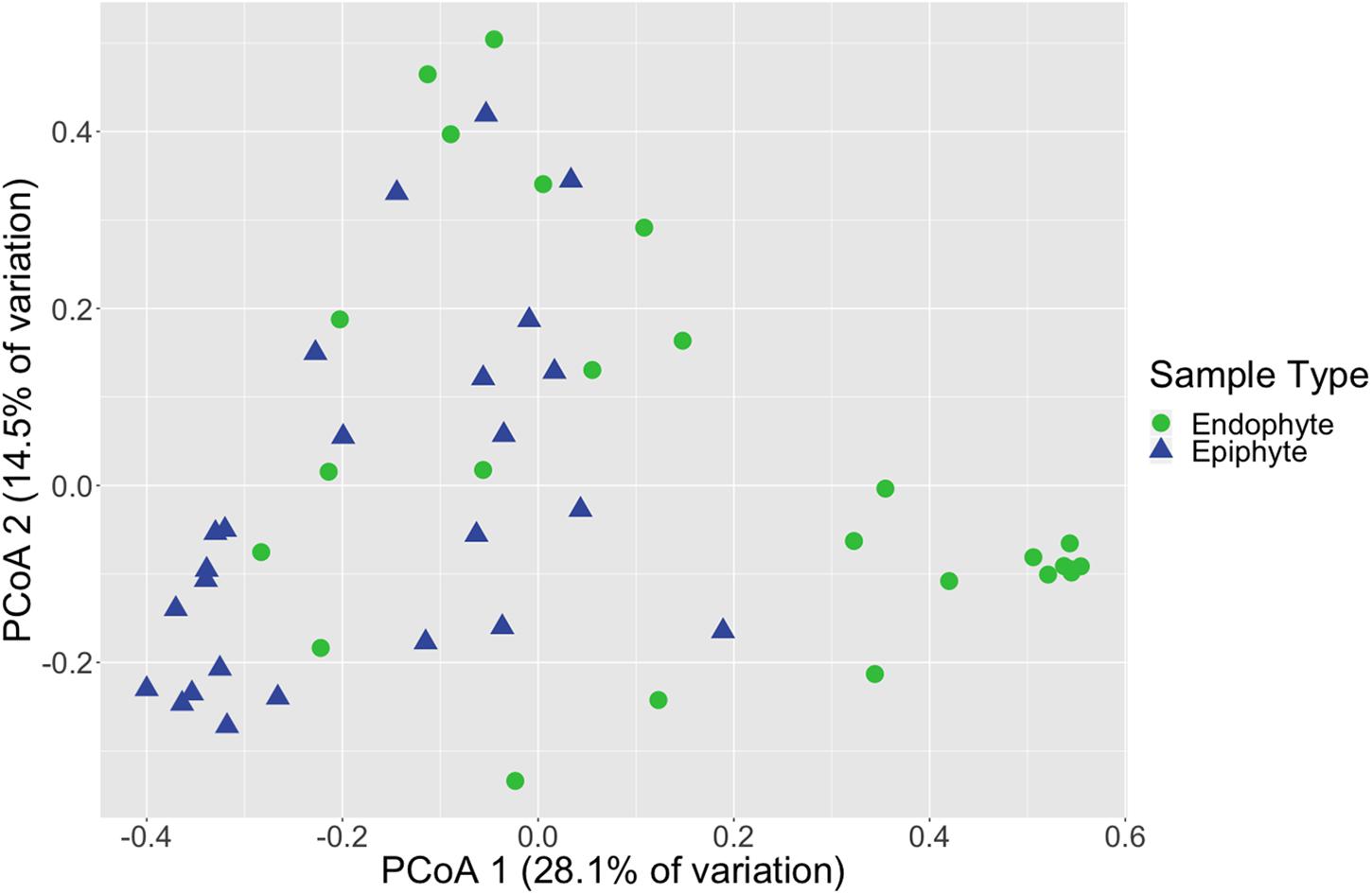
Figure 1. Leaf fungal community structure varies between epiphytes and endophytes. Principal coordinates analysis (PCoA) visualization of Bray-Curtis dissimilarities of fungal communities associated with leaves. Points in the ordination are colored and represented by shapes based on epiphyte (blue triangles; n = 25) or endophyte (green circles; n = 25) status. The dataset was first subset to a depth of 5,000 ITS2 amplicon sequences per sample and then Bray-Curtis dissimilarities were calculated using the ordinate function in phyloseq (McMurdie and Holmes, 2013).
Taxonomic Composition of Mycobiome
The composition of the mycobiome of bulk samples types was generally comprised of members of the taxonomic orders Pleosporales, Helotiales, Saccharomycetales, Coniochaetales, Glomerellales, Agaricales, Cystobasidiales and Malasseziales (Supplementary Table S15 and Figure 2). However, we note that the mycobiome of seagrass tissues was dominated by ASVs that were unable to be classified to a specific fungal phylum using current databases.
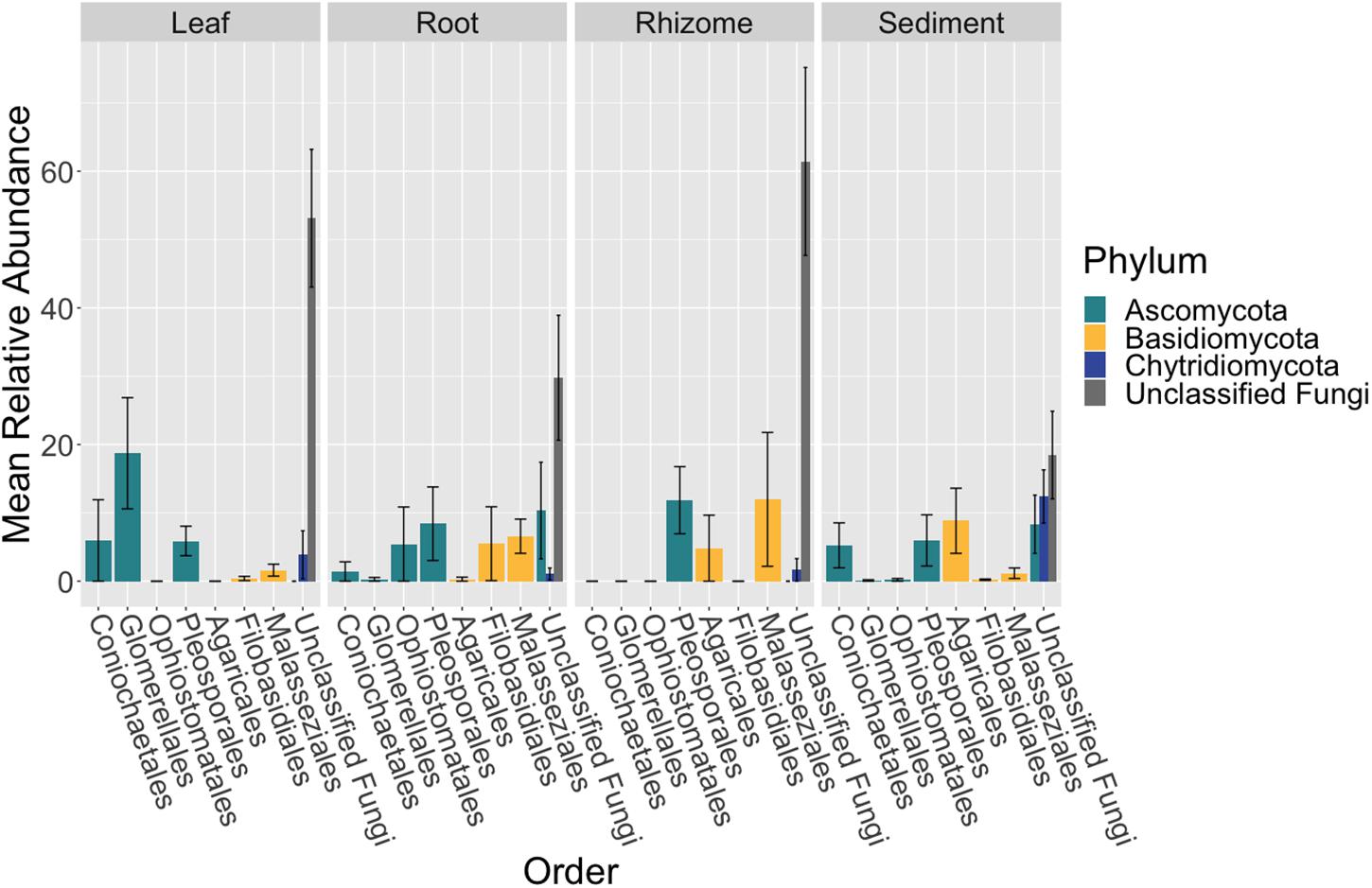
Figure 2. Fungal community composition differs between tissue types. The mean relative abundance of taxonomic orders with a variance of greater than one percent are shown across bulk samples types, leaf (n = 13), root (n = 14), rhizome (n = 7), and sediment (n = 15), with the standard error of the mean represented by error bars and bars colored by taxonomic phylum. The dataset was first subset to a depth of 10,000 sequences per sample, collapsed at the order level using the tax_glom function in phyloseq (McMurdie and Holmes, 2013) and then converted into relative abundance values. Taxonomy was inferred for ITS2 amplicon sequence variants (ASVs) using the RDP Naive Bayesian Classifier algorithm with a modified UNITE (v. 8.0) database (Wang et al., 2007; UNITE Community, 2019).
The order Agaricales had a mean relative abundance that was significantly different between sample types (K-W test, p < 0.05, Supplementary Table S16), while the orders Malasseziales and Glomerellales had mean relative abundances that were only marginally non-significant (p = 0.06). Agaricales was enriched in the sediment relative to root and leaf tissues (Dunn, p < 0.01, Supplementary Table S17). Whereas Malasseziales had a higher mean relative abundance on the roots relative to the leaves and sediment (p < 0.01) and Glomerellales had an increased abundance on the leaves relative to all other sample types (p < 0.05).
Mycobiome Variation in Seagrass Leaves
There were no taxonomic orders with a mean relative abundance that was significantly different between leaf segments (K-W test, p > 0.05, Supplementary Tables S18, S19 and Figure 3). However given the relatively high abundance of ASVs that were unable to be assigned confidently to a fungal order, we investigated whether the mean relative abundance of specific ASVs varied across leaf segments (Supplementary Table S20 and Figure 4). We found that the ASV, SV12, had a significantly higher abundance in leaf segments further from the sediment (K-W test, p < 0.001, Supplementary Table S21). This was further supported by post hoc Dunn test results (p < 0.01, Supplementary Table S22). Based on megablast searches at NCBI, SV12 most closely matches existing ITS2 data from fungal sequences identified as coming from Aphelidomycota. In addition, we were interested in if there were ASVs that had mean relative abundances that varied with epiphyte and endophyte status. We found that the SV8 complex was enriched in epiphyte washes relative to endophyte samples (K-W test, p < 0.01, Supplementary Table S23 and Supplementary Figure S6).
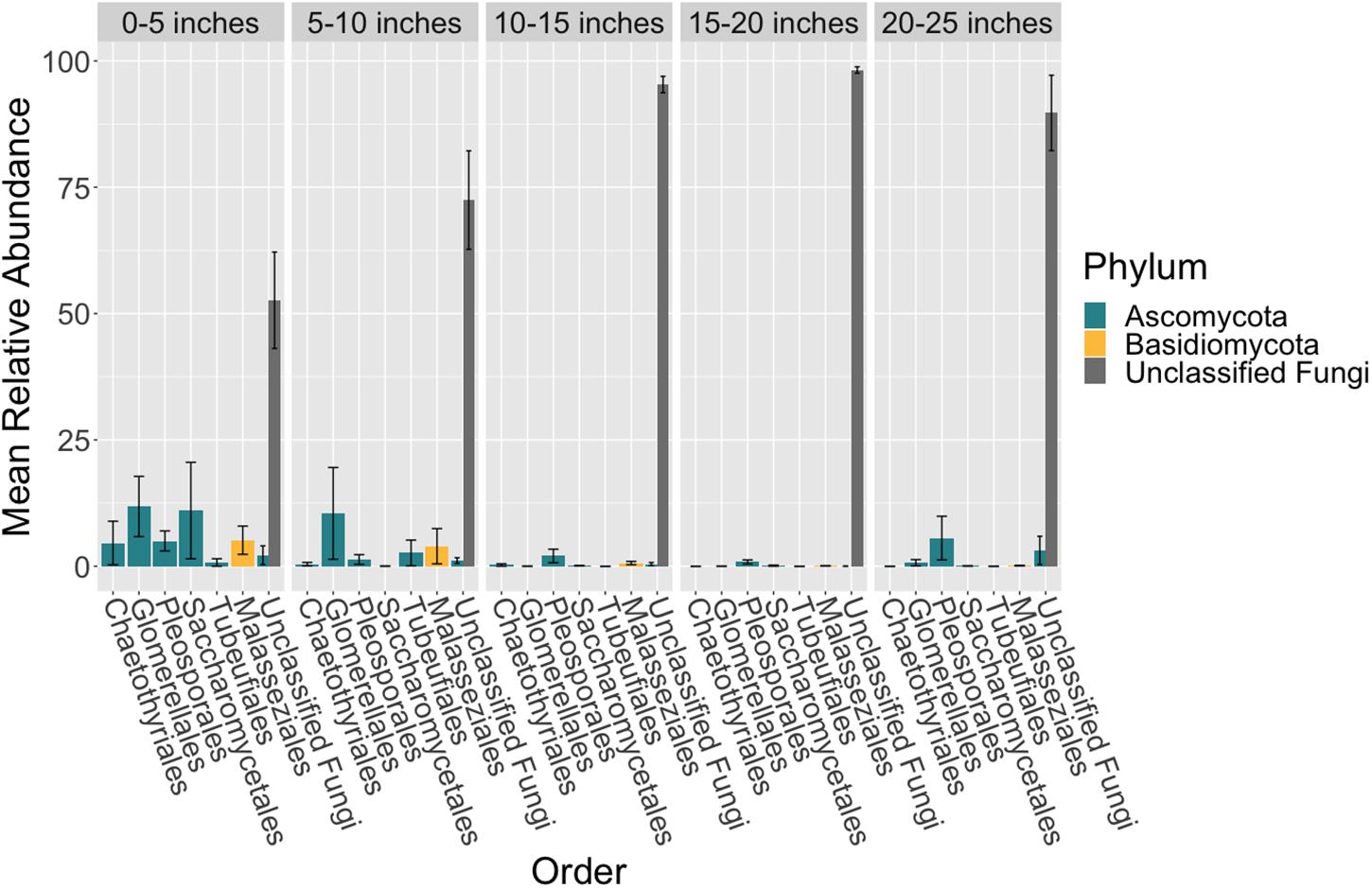
Figure 3. Mean relative abundance of unclassifiable fungi increases across leaf length. The mean relative abundance of taxonomic orders with a variance of greater than 0.1 percent are shown across leaf segments, 0–5 inches (n = 10), 5–10 inches (n = 10), 10–15 inches (n = 10), 15–20 inches (n = 10) and 20–25 inches (n = 10), with the standard error of the mean represented by error bars and bars colored by taxonomic phylum. The dataset was first subset to a depth of 5,000 sequences per sample, collapsed at the order level using the tax_glom function in phyloseq (McMurdie and Holmes, 2013) and then converted into relative abundance values. Taxonomy was inferred for ITS2 ASVs using the RDP Naive Bayesian Classifier algorithm with a modified UNITE (v. 8.0) database (Wang et al., 2007; UNITE Community, 2019).
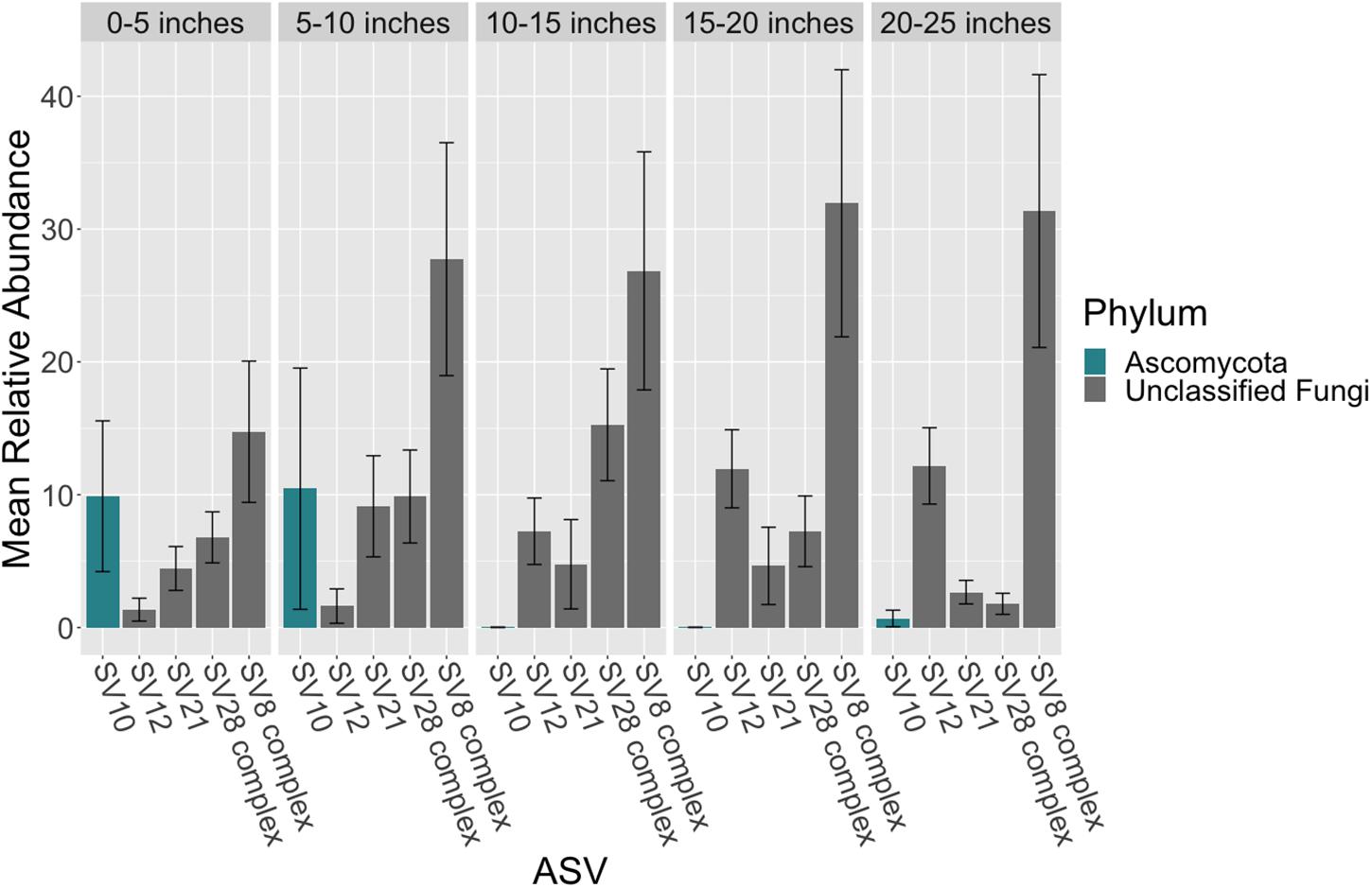
Figure 4. Mean relative abundance of ASVs across leaf length. The mean relative abundance of ASVs with a mean of greater than two percent are shown across leaf segments, 0–5 inches (n = 10), 5–10 inches (n = 10), 10–15 inches (n = 10), 15–20 inches (n = 10) and 20–25 inches (n = 10), with the standard error of the mean represented by error bars and bars colored by taxonomic phylum. The dataset was first subset to a depth of 5,000 sequences per sample and then converted into relative abundance values. ASVs were grouped into complexes if ASVs shared greater than 99% sequence identity. Taxonomy was inferred for ITS2 ASVs using the RDP Naive Bayesian Classifier algorithm with a modified UNITE (v. 8.0) database (Wang et al., 2007; UNITE Community, 2019).
Phylogenetic Placement and Identification of the SV8 Complex
Given the high relative abundance of the SV8 complex in the leaves (Figure 4) and its variable status between epiphyte and endophyte samples, we sought to obtain the sequence of the 28S rRNA gene region for the organism matching this ASV. We designed a primer specific to the ITS2 ASV for the complex using the sequence of SV8 and used a universal fungal 28S rRNA gene primer to obtain a linked ITS2-28S rRNA gene sequence associated with this complex. The resulting ITS2 portion of the resulting PCR products align well with all ASVs from the SV8 complex. Therefore, we believe the 28S rRNA gene sequences obtained accurately represent the flanking region to the taxonomic group represented by the SV8 complex. We then used these 28S rRNA gene sequences to place the organism represented by this ASV complex into a phylogenetic context using both Bayesian and maximum likelihood approaches and then assign it a taxonomic classification. Both approaches produced near-identical topologies (only one branch difference) and the following conclusions are based on the Bayesian topology. The SV8 complex (and thus also all ASVs within this complex) groups within the order Lobulomycetales in the phylum Chytridiomycota (Figure 5). Specifically, the SV8 complex is nested within the recently defined Novel Clade SW-I (Van den Wyngaert et al., 2018) and is sister to several culture independent sequences obtained from the marine ecosystem (Hassett et al., 2017).
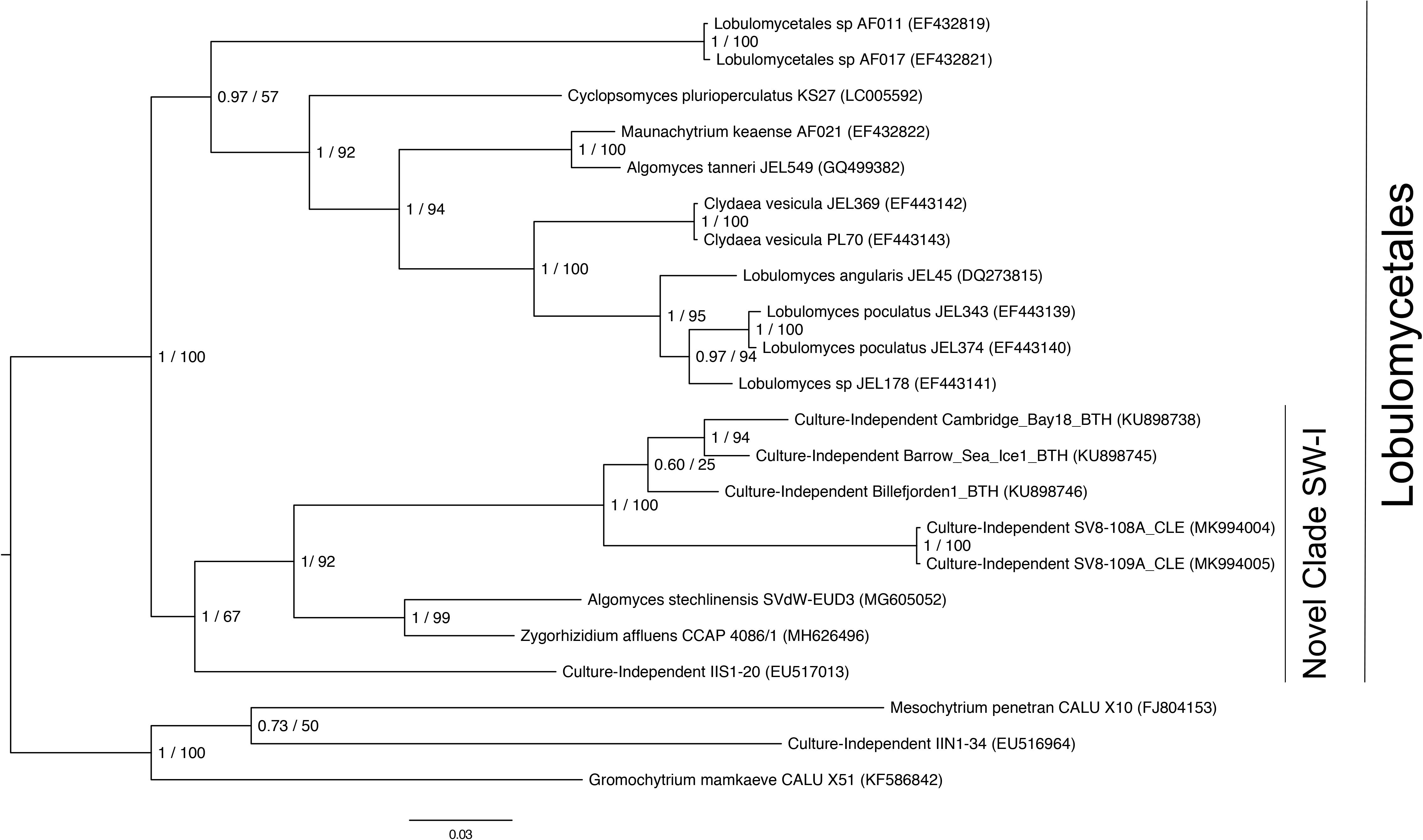
Figure 5. Phylogenetic placement of the SV8 complex in Chytridiomycota. A molecular phylogeny of 28S rRNA genes was constructed using Bayesian inference. Sequence alignments were generated using MAFFT (v. 7.402) on the CIPRES Science Gateway web server, trimmed using trimAl (v.1.2) and a phylogenetic tree was inferred on the trimmed alignment with a GTR + G + I model using MrBayes (v. 3.2.2) (Huelsenbeck and Ronquist, 2001; Katoh, 2002; Miller et al., 2010). Displayed at each node in the tree are the Bayesian posterior probabilities (first number) estimated with MrBayes (v. 3.2.2) and the maximum likelihood bootstrap values (second number) calculated with RAxML (v. 8) (Stamatakis, 2014). The SV8 complex represents a series of four abundant ASVs (SV8, SV11, SV16, and SV56) that are greater than 99% identical. The 28S rRNA gene sequences for the SV8 complex are named SV8-108A_CLE and SV8-109A_CLE in this phylogeny and were amplified from two leaf epiphyte samples (sample IDs: 108A and 109A). The GenBank accession numbers of the sequences used to build this phylogeny can be found in Supplementary Table S4.
Discussion
Here we offer an in depth survey of the fungi associated with Z. marina. We observed that the mycobiome of Z. marina roots and rhizomes had lower alpha diversity than that of rhizosphere sediment and that generally the Z. marina mycobiome had relatively low species diversity. Low levels of fungal colonization as well as decreased alpha diversity compared to nearby sediment have previously been made in culture and clone based studies of seagrasses (Devarajan and Suryanarayanan, 2002; Sakayaroj et al., 2010; Shoemaker and Wyllie-Echeverria, 2013; Ling et al., 2015; Venkatachalam et al., 2015; Van den Wyngaert et al., 2018). Possible reasons for low abundance and/or infrequent colonization of fungi include indirect factors such as the high salinity and low oxygen levels that are reflective of the marine ecosystem (Nielsen et al., 1999) and direct factors such as seagrass-derived phenolic compounds which are known to act as antimicrobials (Zapata and McMillan, 1979). Additionally, seasonal differences in fungal colonization of seagrasses have been observed, adding an additional layer of complexity and thus making fungal diversity and abundance comparisons difficult (Mata and Cebrián, 2013).
Previous sequence-based studies of seagrass associated fungi, observed high abundances of sequences classified as Pleosporales (Hurtado-McCormick et al., 2019) or Eurotiales (Wainwright et al., 2018), where as here the dominant community members appear to be from understudied lineages like the Chytridiomycota. Additionally, Hurtado-McCormick et al. found evidence of Glomeromycota (arbuscular mycorrhizal fungi) in their dataset, whereas we did not. Possible reasons for these different observations include that these studies sampled different seagrass species than we did, had different sampling schema and used different sequencing methods and primer sets. The use of different sequencing primers specifically has been found to have drastic effects on the results and conclusions of mycobiome studies (Frau et al., 2019).
We did see evidence of known DSE associating with Z. marina, including members of the Pleosporales and Glomerellales, both of which include groups that are known to associate with terrestrial plants (Arnold et al., 2007). We found that the Glomerellales, particularly a Colletotrichum species (SV10), was abundant in the lower 10 inches of leaf tissue and enriched on the leaves relative to other sample types. A Colletotrichum spp. was previously isolated as an endophyte from undamaged leaves of the seagrass Thalassia testudinum (Rodriguez, 2008) and this fungal genus is known to include members that can form endophytic or pathogenic associations with land plants (De Silva et al., 2017). Additionally, DSE, particularly members of the Pleosporales, have previously been observed associating with seagrasses (Panno et al., 2013; Gnavi et al., 2014; Torta et al., 2015; Vohník et al., 2015, 2016, 2017, 2019; Borovec and Vohník, 2018). In the mediteranean seagrass, Posidonea, it has been observed that the abundance of the dominant root associated fungi, a Pleosporales species (Posidoniomyces atricolor gen. et sp. nov.), is associated with changes in root hair morphology and can form ecto-mycorrhizal-like structures suggesting a close seagrass-fungi symbiosis (Vohník et al., 2016, 2017, 2019; Borovec and Vohník, 2018).
Although many of the ASVs observed here associated with Z. marina are still unidentified, we were able to phylogenetically place the SV8 complex in Novel Clade SW-I in the Lobulomycetales. Known members of Novel Clade SW-I are parasitic chytrids of freshwater algae and diatoms (Frenken et al., 2017; Rad-Menéndez et al., 2018; Van den Wyngaert et al., 2018). It is possible that the SV8 complex’s high relative abundance both on and in Z. marina leaves may indicate that it is directly infecting host plant tissues and suggests that seagrasses may provide a niche for marine chytrids. However, we note that there is another possible interpretation, which is that the SV8 complex is infecting algae or diatoms that are tightly associated with the leaves of Z. marina which are themselves a part of the larger Z. marina microbiome. This latter interpretation seems to be supported by the observation that the SV8 complex has a higher mean relative abundance in epiphyte washes relative to endophyte washes. However, the closest cultivated relative of the SV8 complex has a gregarious infection pattern and forms sporangia outside of host cells (Van den Wyngaert et al., 2018). Thus, we might expect to find more DNA associated with sporangia and zoospores in the epiphyte washes than predicted for most chytrids. The higher relative abundance of the SV8 complex in the epiphyte washes is consistent with both explanations.
Our observations here are not the first evidence of chytrid associations with seagrasses. A rhizomycelial chytrid was previously observed as the most abundant fungus associating with Thalassia testudinum leaves (Newell and Fell, 1980) and was hypothesized to be a possible symbiont, or weak parasite, of the leaves. In a later study, the authors looked for this same chytrid association in Zostera with little success (Newell, 1981). However the authors only looked at one location at one time and we note that although the SV8 complex was present at low relative abundance in some samples during our first two sampling time points, it was not prevalent. Members of the Lobulomycetales and marine fungi more generally have been observed to exhibit some seasonality in their emergence which has been hypothesized to relate to host-dynamics and environmental conditions (Longcore, 1992; Seto and Degawa, 2015; Hassett and Gradinger, 2016; Rojas-Jimenez et al., 2019). Thus, the high abundance of the SV8 complex during our third sampling time point may be because we observed it during a bloom event.
Another ASV of interest, which was initially unable to be classified into a fungal phylum, is SV12, which most closely matches existing ITS2 data from fungal sequences in the Aphelidomycota. Known Aphelidomycota are intracellular parasites of green algae (Tedersoo et al., 2018). The increased relative abundance of SV12 in the endophyte samples relative to the epiphyte washes is consistent with the possibility that the SV12 containing organisms are directly infecting Z. marina leaf tissue. However, as with the SV8 complex, it is also possible that SV12 containing organisms could be associating with an algae that is itself tightly associated with Z. marina leaves.
The prevalence of unclassifiable ASVs, many of which have closest matches in the Chytridiomycota and Aphelidomycota, with Z. marina makes sense given that these lineages have been previously observed to be the dominant fungal lineages in the marine and aquatic ecosystems (Richards et al., 2012, 2015; Comeau et al., 2016; Picard, 2017; Grossart et al., 2019; Rojas-Jimenez et al., 2019) and have life histories that include associations with green algae (Letcher et al., 2013; Picard et al., 2013; Tedersoo et al., 2018). Thus, it is likely that seagrasses, as marine plants, may be providing these fungal lineages with an ecological niche.
Conclusion
We observed that Z. marina tissues harbor distinct fungal communities and present analyses and speculation regarding the identity and possible functional roles of these species. For example, we identified that the SV8 complex, which represents the most prevalent sequences associated with Z. marina leaf tissue, is nested within Novel Clade SW-I in the order Lobulomycetales and hypothesize that this chytrid may be directly infecting Z. marina leaf tissues. However, despite their abundance and possible importance, as evidenced by the number of novel fungal sequences observed here, we still know relatively little about the diversity and functional roles of marine fungi in the seagrass ecosystem. We propose that seagrass beds may be hotspots of marine fungal diversity, specifically the diversity of understudied lineages that are distantly related from well studied fungi (e.g., the Dikarya). Additionally, seagrasses may be an interesting model with which to look at terrestrial to marine transitions in the context of host-microbe interactions since their evolutionary history (coevolution from a terrestrial ancestor) mirrors the evolutionary history of many lineages of marine fungi, which are thought to have ancestors that secondarily returned to the marine environment multiple times (Spatafora et al., 1998; Schoch et al., 2009; Suetrong et al., 2009; Jones et al., 2015). This work helps lay a foundation for future seagrass-fungal studies and highlights a need for future studies focusing on marine fungi and the potential functional importance of these understudied communities to the larger seagrass ecosystem.
Data Availability Statement
This ITS amplicon sequencing project has been deposited on GenBank under BioProject ID PRJNA515720 and consensus sequences for the SV8 complex were deposited under accession numbers MK994004 and MK994005.
Author Contributions
CE conceived and designed the experiments, performed the sampling, analyzed the data, prepared the figures and/or tables, and wrote and reviewed the drafts of the manuscript. JE advised on the data analysis, and edited and reviewed the drafts of the manuscript.
Funding
This work was supported by grants from the University of California Natural Reserve System and the UC Davis Center for Population Biology to CE. JE time was supported in part by a grant from the Gordon and Betty Moore Foundation on Evolution of Microbiomes in Shallow-Water Marine Organisms Split by the Isthmus of Panama in the Western Atlantic vs. Eastern Pacific. The funders had no role in study design, data collection and analysis, decision to publish, or preparation of the manuscript.
Conflict of Interest
JE is on the Scientific Advisory Board of Zymo Research, Inc. and a discount was received for the sequencing services provided.
The remaining author declares that the research was conducted in the absence of any commercial or financial relationships that could be construed as a potential conflict of interest.
Acknowledgments
We would like to thank Katherine Dynarski (ORCID: 0000-0001-5101-9666) and Sonia Ghose (ORCID: 0000-0001-5667-6876) for their help with sample collection. We would also like to thank John J. Stachowicz for use of his scientific sampling permit, California Department of Fish and Wildlife Scientific Collecting Permit # SC 4874. We are grateful to Marina LaForgia (ORCID: 0000-0003-4377-0841) for her helpful comments on the R code used.
Supplementary Material
The Supplementary Material for this article can be found online at: https://www.frontiersin.org/articles/10.3389/fmicb.2019.02476/full#supplementary-material
Footnotes
References
Amend, A., Burgaud, G., Cunliffe, M., Edgcomb, V. P., Ettinger, C. L., Gutiérrez, M. H., et al. (2019). Fungi in the marine environment: open questions and unsolved problems. mBio 10:e01189-18. doi: 10.1128/mBio.01189-18
Anderson, M. J. (2001). A new method for non-parametric multivariate analysis of variance. Aust. Ecol. 26, 32–46. doi: 10.1111/j.1442-9993.2001.01070.pp.x
Anderson, M. J., and Walsh, D. C. I. (2013). PERMANOVA, ANOSIM, and the Mantel test in the face of heterogeneous dispersions: what null hypothesis are you testing? Ecol. Monogr. 83, 557–574. doi: 10.1890/12-2010.1
Arnold, A. E., Henk, D. A., Eells, R. L., Lutzoni, F., and Vilgalys, R. (2007). Diversity and phylogenetic affinities of foliar fungal endophytes in loblolly pine inferred by culturing and environmental PCR. Mycologia 99, 185–206. doi: 10.3852/mycologia.99.2.185
Beck-Nielsen, D., and Madsen, T. V. (2001). Occurrence of vesicular–arbuscular mycorrhiza in aquatic macrophytes from lakes and streams. Aqua. Bot. 71, 141–148. doi: 10.1016/s0304-3770(01)00180-2
Bengtsson-Palme, J., Ryberg, M., Hartmann, M., Branco, S., Wang, Z., Godhe, A., et al. (2013). Improved software detection and extraction of ITS1 and ITS2 from ribosomal ITS sequences of fungi and other eukaryotes for analysis of environmental sequencing data. Methods Ecol. Evol. 4, 914–919. doi: 10.1111/2041-210x.12073
Bohrer, K. E., Friese, C. F., and Amon, J. P. (2004). Seasonal dynamics of arbuscular mycorrhizal fungi in differing wetland habitats. Mycorrhiza 14, 329–337. doi: 10.1007/s00572-004-0292-7
Bonfante, P., and Anca, I.-A. (2009). Plants, mycorrhizal fungi, and bacteria: a network of interactions. Annu. Rev. Microbiol. 63, 363–383. doi: 10.1146/annurev.micro.091208.073504
Borovec, O., and Vohník, M. (2018). Ontogenetic transition from specialized root hairs to specific root-fungus symbiosis in the dominant Mediterranean seagrass Posidonia oceanica. Sci. Rep. 8:10773. doi: 10.1038/s41598-018-28989-4
Bray, J. R., Roger Bray, J., and Curtis, J. T. (1957). An ordination of the upland forest communities of southern wisconsin. Ecol. Monogr. 27, 325–349. doi: 10.2307/1942268
Brundrett, M. C. (2002). Coevolution of roots and mycorrhizas of land plants. New Phytol. 154, 275–304. doi: 10.1046/j.1469-8137.2002.00397.x
Brundrett, M. C. (2009). Mycorrhizal associations and other means of nutrition of vascular plants: understanding the global diversity of host plants by resolving conflicting information and developing reliable means of diagnosis. Plant Soil 320, 37–77. doi: 10.1007/s11104-008-9877-9
Callahan, B. J., McMurdie, P. J., Rosen, M. J., Han, A. W., Johnson, A. J. A., and Holmes, S. P. (2016). DADA2: high-resolution sample inference from Illumina amplicon data. Nat. Methods 13, 581–583. doi: 10.1038/nmeth.3869
Capella-Gutiérrez, S., Silla-Martínez, J. M., and Gabaldón, T. (2009). trimAl: a tool for automated alignment trimming in large-scale phylogenetic analyses. Bioinformatics 25, 1972–1973. doi: 10.1093/bioinformatics/btp348
Clayton, J. S., and Bagyaraj, D. J. (1984). Vesicular-arbuscular mycorrhizas in submerged aquatic plants of New Zealand. Aqua. Bot. 19, 251–262. doi: 10.1016/0304-3770(84)90043-3
Comeau, A. M., Douglas, G. M., and Langille, M. G. I. (2017). Microbiome helper: a custom and streamlined workflow for microbiome research. mSystems 2:e00127-16. doi: 10.1128/msystems.00127-16
Comeau, A. M., Vincent, W. F., Bernier, L., and Lovejoy, C. (2016). Novel chytrid lineages dominate fungal sequences in diverse marine and freshwater habitats. Sci. Rep. 6:30120. doi: 10.1038/srep30120
Costanza, R., d’Arge, R., de Groot, R., Farber, S., Grasso, M., Hannon, B., et al. (1997). The value of the world’s ecosystem services and natural capital. Nature 387, 253–260. doi: 10.1038/387253a0
Cuomo, V., Vanzanella, F., Fresi, E., Cinelli, F., and Mazzella, L. (1985). Fungal flora of Posidonia oceanica and its ecological significance. Trans. Br. Mycol. Soc. 84, 35–40. doi: 10.1016/s0007-1536(85)80217-5
Darriba, D., Taboada, G. L., Doallo, R., and Posada, D. (2012). jModelTest 2: more models, new heuristics and parallel computing. Nat. Methods 9:772. doi: 10.1038/nmeth.2109
Davis, N. M., Proctor, D. M., Holmes, S. P., Relman, D. A., and Callahan, B. J. (2018). Simple statistical identification and removal of contaminant sequences in marker-gene and metagenomics data. Microbiome 6:226. doi: 10.1186/s40168-018-0605-2
De Silva, D. D., Crous, P. W., Ades, P. K., Hyde, K. D., and Taylor, P. W. (2017). Life styles of Colletotrichum species and implications for plant biosecurity. Fungal Biol. Rev. 31, 155–168. doi: 10.1016/j.fbr.2017.05.001
Devarajan, P. T., and Suryanarayanan, T. (2002). Endophytic fungi associated with the tropical seagrass Halophila ovalis (Hydrocharitaceae). Indian J. Mar. Sci. 31, 73–74.
Dixon, P. (2003). VEGAN, a package of R functions for community ecology. J. Veg. Sci. 14:927. doi: 10.1658/1100-9233(2003)014%5B0927:vaporf%5D2.0.co;2
Dunitz, M. I., Lang, J. M., Jospin, G., Darling, A. E., Eisen, J. A., and Coil, D. A. (2015). Swabs to genomes: a comprehensive workflow. PeerJ 3:e960. doi: 10.7717/peerj.960
Ettinger, C. L., Voerman, S. E., Lang, J. M., Stachowicz, J. J., and Eisen, J. A. (2017). Microbial communities in sediment from patches, but not the leaf or root microbiomes, vary in relation to distance from patch edge. PeerJ 5:e3246. doi: 10.7717/peerj.3246
Frau, A., Kenny, J. G., Lenzi, L., Campbell, B. J., Ijaz, U. Z., Duckworth, C. A., et al. (2019). DNA extraction and amplicon production strategies deeply inf luence the outcome of gut mycobiome studies. Sci. Rep. 9:328. doi: 10.1038/s41598-019-44974-x
Frenken, T., Alacid, E., Berger, S. A., Bourne, E. C., Gerphagnon, M., Grossart, H.-P., et al. (2017). Integrating chytrid fungal parasites into plankton ecology: research gaps and needs. Environ. Microbiol. 19, 3802–3822. doi: 10.1111/1462-2920.13827
Gnavi, G., Ercole, E., Panno, L., Vizzini, A., and Varese, G. C. (2014). Dothideomycetes and Leotiomycetes sterile mycelia isolated from the Italian seagrass Posidonia oceanica based on rDNA data. Springerplus 3:508. doi: 10.1186/2193-1801-3-508
Grossart, H.-P., Van den Wyngaert, S., Kagami, M., Wurzbacher, C., Cunliffe, M., and Rojas-Jimenez, K. (2019). Fungi in aquatic ecosystems. Nat. Rev. Microbiol. 17, 339–354. doi: 10.1038/s41579-019-0175-8
Guindon, S., and Gascuel, O. (2003). A simple, fast, and accurate algorithm to estimate large phylogenies by maximum likelihood. Syst. Biol. 52, 696–704. doi: 10.1080/10635150390235520
Hassett, B. T., Ducluzeau, A.-L. L., Collins, R. E., and Gradinger, R. (2017). Spatial distribution of aquatic marine fungi across the western Arctic and sub-arctic. Environ. Microbiol. 19, 475–484. doi: 10.1111/1462-2920.13371
Hassett, B. T., and Gradinger, R. (2016). Chytrids dominate arctic marine fungal communities. Environ. Microbiol. 18, 2001–2009. doi: 10.1111/1462-2920.13216
Huelsenbeck, J. P., and Ronquist, F. (2001). MRBAYES: bayesian inference of phylogenetic trees. Bioinformatics 17, 754–755. doi: 10.1093/bioinformatics/17.8.754
Humphreys, C. P., Franks, P. J., Rees, M., Bidartondo, M. I., Leake, J. R., and Beerling, D. J. (2010). Mutualistic mycorrhiza-like symbiosis in the most ancient group of land plants. Nat. Commun. 1:103.
Hurtado-McCormick, V., Kahlke, T., Petrou, K., Jeffries, T., Ralph, P. J., and Seymour, J. R. (2019). Regional and microenvironmental scale characterization of the zostera muelleri seagrass microbiome. Front. Microbiol. 10:1011. doi: 10.3389/fmicb.2019.01011
Jones, E. B. G., and Gareth Jones, E. B. (2011). Are there more marine fungi to be described? Bot. Mar. 54, 343–354. doi: 10.1515/bot.2011.043
Jones, E. B. G., Gareth Jones, E. B., Suetrong, S., Sakayaroj, J., Bahkali, A. H., Abdel-Wahab, M. A., et al. (2015). Classification of marine Ascomycota, Basidiomycota, Blastocladiomycota and Chytridiomycota. Fungal Divers. 73, 1–72. doi: 10.1007/s13225-015-0339-4
Kadivar, H., and Stapleton, A. E. (2003). Ultraviolet radiation alters maize phyllosphere bacterial diversity. Microb. Ecol. 45, 353–361. doi: 10.1007/s00248-002-1065-5
Karpov, S. A., Kobseva, A. A., Mamkaeva, M. A., Mamkaeva, K. A., Mikhailov, K. V., Mirzaeva, G. S., et al. (2014). Gromochytrium mamkaevae gen. & sp. nov. and two new orders: Gromochytriales and Mesochytriales (Chytridiomycetes). Persoonia 32, 115–126. doi: 10.3767/003158514x680234
Katoh, K. (2002). MAFFT: a novel method for rapid multiple sequence alignment based on fast Fourier transform. Nucleic Acids Res. 30, 3059–3066. doi: 10.1093/nar/gkf436
Kembel, S. W., O’Connor, T. K., Arnold, H. K., Hubbell, S. P., Wright, S. J., and Green, J. L. (2014). Relationships between phyllosphere bacterial communities and plant functional traits in a neotropical forest. Proc. Natl. Acad. Sci. U.S.A. 111, 13715–13720. doi: 10.1073/pnas.1216057111
Khan, A. G., and Belik, M. (1995). “Occurence and ecological significance of mycorrhizal symbiosis in aquatic plants,” in Mycorrhiza: Structure, Function, Molecular Biology and Biotechnology, eds A. Verma and B. Hock (Heidelberg: Springer-Verlag), 627–666. doi: 10.1007/978-3-662-08897-5_27
Kirichuk, N. N., and Pivkin, M. V. (2015). Filamentous fungi associated with the seagrass Zostera marina Linnaeus, 1753 of Rifovaya Bay (Peter the Great Bay, the Sea of Japan). Russ. J. Mar. Biol. 41, 351–355. doi: 10.1134/s1063074015050053
Kohout, P., Sýkorová, Z., Čtvrtlíková, M., Rydlová, J., Suda, J., Vohník, M., et al. (2012). Surprising spectra of root-associated fungi in submerged aquatic plants. FEMS Microbiol. Ecol. 80, 216–235. doi: 10.1111/j.1574-6941.2011.01291.x
Kress, W. J., and Erickson, D. L. (2012). DNA Barcodes: Methods and Protocols. Totowa, NJ: Humana Press, doi: 10.1007/978-1-61779-591-6_1
Kuo, J. (1984). Structural aspects of apoplast fungal hyphae in a marine angiosperm, Zostera muelleri Irmisch ex Aschers. (Zosteraceae). Protoplasma 121, 1–7. doi: 10.1007/bf01279746
Les, D. H., Cleland, M. A., and Waycott, M. (1997). Phylogenetic studies in alismatidae, ii: evolution of marine angiosperms (Seagrasses) and hydrophily. Syst. Bot. 22:443. doi: 10.2307/2419820
Letcher, P. M., Lopez, S., Schmieder, R., Lee, P. A., Behnke, C., Powell, M. J., et al. (2013). Characterization of Amoeboaphelidium protococcarum, an algal parasite new to the cryptomycota isolated from an outdoor algal pond used for the production of biofuel. PLoS One 8:e56232. doi: 10.1371/journal.pone.0056232
Ling, J., Zhang, Y., Wu, M., Wang, Y., Dong, J., Jiang, Y., et al. (2015). Fungal community successions in Rhizosphere sediment of seagrasses Enhalus acoroides under PAHs stress. Int. J. Mol. Sci. 16, 14039–14055. doi: 10.3390/ijms160614039
Longcore, J. E. (1992). Morphology and zoospore ultrastructure of Chytriomyces angularis sp. nov.(Chytridiales). Mycologia 84, 442–451. doi: 10.1080/00275514.1992.12026158
Martin, M. (2011). Cutadapt removes adapter sequences from high-throughput sequencing reads. EMBnet.J 17:10. doi: 10.14806/ej.17.1.200
Mata, J. L., and Cebrián, J. (2013). Fungal endophytes of the seagrasses Halodule wrightii and Thalassia testudinum in the north-central Gulf of Mexico. Bot. Mar. 56, 541–545. doi: 10.1515/bot-2013-0047
McHugh, T. A., and Schwartz, E. (2015). Changes in plant community composition and reduced precipitation have limited effects on the structure of soil bacterial and fungal communities present in a semiarid grassland. Plant Soil 388, 175–186. doi: 10.1007/s11104-014-2269-4
McMurdie, P. J., and Holmes, S. (2013). phyloseq: an R package for reproducible interactive analysis and graphics of microbiome census data. PLoS One 8:e61217. doi: 10.1371/journal.pone.0061217
Miller, M. A., Pfeiffer, W., and Schwartz, T. (2010). “Creating the CIPRES science gateway for inference of large phylogenetic trees,” in Proceedings of the 2010 Gateway Computing Environments Workshop (GCE), (Piscataway, NJ: IEEE), doi: 10.1109/gce.2010.5676129
Newell, S. (1981). Fungi and bacteria in or on leaves of eelgrass (Zostera marina L.) from Chesapeake Bay. Appl. Environ. Microbiol. 41, 1219–1224.
Newell, S., and Fell, J. (1980). Mycoflora of turtlegrass (Thalassia testudinum Konig) as recorded after seawater incubation. Bot. Mar. 23, 265–275.
Nielsen, S. L., Thingstrup, I., and Wigand, C. (1999). Apparent lack of vesicular–arbuscular mycorrhiza (VAM) in the seagrasses Zostera marina L. and Thalassia testudinum Banks ex König. Aqua. Bot. 63, 261–266. doi: 10.1016/s0304-3770(98)00123-125
Ogle, D. H. (2016). FSA: Fisheries Stock Analysis. Available at: https://cran.r-project.org/web/packages/FSA/index.html
Orth, R. J., Carruthers, T. J. B., Dennison, W. C., Duarte, C. M., Fourqurean, J. W., Heck, K. L., et al. (2006). a global crisis for seagrass ecosystems. Bioscience 56, 987–996.
Panno, L., Bruno, M., Voyron, S., Anastasi, A., Gnavi, G., Miserere, L., et al. (2013). Diversity, ecological role and potential biotechnological applications of marine fungi associated to the seagrass Posidonia oceanica. N. Biotechnol. 30, 685–694. doi: 10.1016/j.nbt.2013.01.010
Phongpaichit, S., and Supaphon, P. (2014). Diversity and antimicrobial activity of endophytic fungi isolated from the seagrass Enhalus acoroides. IJMS 43, 785–797.
Picard, K. T. (2017). Coastal marine habitats harbor novel early-diverging fungal diversity. Fungal Ecol. 25, 1–13. doi: 10.1016/j.funeco.2016.10.006
Picard, K. T., Letcher, P. M., and Powell, M. J. (2013). Evidence for a facultative mutualist nutritional relationship between the green coccoid alga Bracteacoccus sp. (Chlorophyceae) and the zoosporic fungus Rhizidium phycophilum (Chytridiomycota). Fungal Biol. 117, 319–328. doi: 10.1016/j.funbio.2013.03.003
Porras-Alfaro, A., and Bayman, P. (2011). Hidden fungi, emergent properties: endophytes and microbiomes. Annu. Rev. Phytopathol. 49, 291–315. doi: 10.1146/annurev-phyto-080508-081831
Radhika, K. P., and Rodrigues, B. F. (2007). Arbuscular mycorrhizae in association with aquatic and marshy plant species in Goa, India. Aqua. Bot. 86, 291–294. doi: 10.1016/j.aquabot.2006.10.009
Rad-Menéndez, C., Gerphagnon, M., Garvetto, A., Arce, P., Badis, Y., Sime-Ngando, T., et al. (2018). Rediscovering Zygorhizidium affluens canter: molecular taxonomy, infectious cycle, and cryopreservation of a chytrid infecting the bloom-forming diatom Asterionella formosa. Appl. Environ. Microbiol. 84:e01826-18. doi: 10.1128/AEM.01826-18
Rambaut, A. (2009). FigTree, Version 1.4.2. Available at: http://tree.bio.ed.ac.uk/software/figtree/
Redecker, D., Kodner, R., and Graham, L. E. (2000). Glomalean fungi from the Ordovician. Science 289, 1920–1921. doi: 10.1126/science.289.5486.1920
Richards, T. A., Jones, M. D. M., Leonard, G., and Bass, D. (2012). Marine fungi: their ecology and molecular diversity. Annu. Rev. Mar. Sci. 4, 495–522. doi: 10.1146/annurev-marine-120710-100802
Richards, T. A., Leonard, G., Mahé, F., Del Campo, J., Romac, S., Jones, M. D. M., et al. (2015). Molecular diversity and distribution of marine fungi across 130 European environmental samples. Proc. Biol. Sci. 282:20152243. doi: 10.1098/rspb.2015.2243
Rodriguez, G. M. (2008). Potential of fungal endophytes from Thalassia testudinum Bank ex K.D. koenig as producers of bioactive compounds. Master’s thesis, University of Puerto Rico, Mayaguez.
Rojas-Jimenez, K., Rieck, A., Wurzbacher, C., Jürgens, K., Labrenz, M., and Grossart, H.-P. (2019). A salinity threshold separating fungal communities in the Baltic Sea. Front. Microbiol. 10:680. doi: 10.3389/fmicb.2019.00680
Sakayaroj, J., Preedanon, S., Supaphon, O., Jones, E. B. G., and Phongpaichit, S. (2010). Phylogenetic diversity of endophyte assemblages associated with the tropical seagrass Enhalus acoroides in Thailand. Fungal Diversity 42, 27–45. doi: 10.1007/s13225-009-0013-9
Schoch, C. L., Crous, P. W., Groenewald, J. Z., Boehm, E. W. A., Burgess, T. I., de Gruyter, J., et al. (2009). A class-wide phylogenetic assessment of Dothideomycetes. Stud. Mycol. 64, 1S–15S. doi: 10.3114/sim.2009.64.01
Seto, K., and Degawa, Y. (2015). Cyclopsomyces plurioperculatus: a new genus and species of Lobulomycetales (Chytridiomycota. Chytridiomycetes) from Japan. Mycologia 107, 633–640. doi: 10.3852/14-284
Shoemaker, G., and Wyllie-Echeverria, S. (2013). Occurrence of rhizomal endophytes in three temperate northeast pacific seagrasses. Aqua. Bot. 111, 71–73. doi: 10.1016/j.aquabot.2013.05.010
Simmons, D. R., James, T. Y., Meyer, A. F., and Longcore, J. E. (2009). Lobulomycetales, a new order in the Chytridiomycota. Mycol. Res. 113, 450–460. doi: 10.1016/j.mycres.2008.11.019
Spatafora, J. W., Volkmann-Kohlmeyer, B., and Kohlmeyer, J. (1998). Independent terrestrial origins of the Halosphaeriales (marine Ascomycota). Am. J. Bot. 85, 1569–1580. doi: 10.2307/2446483
Šraj-Kržič, N., Pongrac, P., Klemenc, M., Kladnik, A., Regvar, M., and Gaberščik, A. (2006). Mycorrhizal colonisation in plants from intermittent aquatic habitats. Aqua. Bot. 85, 331–336. doi: 10.1016/j.aquabot.2006.07.001
Stamatakis, A. (2014). RAxML version 8: a tool for phylogenetic analysis and post-analysis of large phylogenies. Bioinformatics 30, 1312–1313. doi: 10.1093/bioinformatics/btu033
Suetrong, S., Schoch, C. L., Spatafora, J. W., Kohlmeyer, J., Volkmann-Kohlmeyer, B., Sakayaroj, J., et al. (2009). Molecular systematics of the marine Dothideomycetes. Stud. Mycol. 64, 155S–173S. doi: 10.3114/sim.2009.64.09
Supaphon, P., Phongpaichit, S., Rukachaisirikul, V., and Sakayaroj, J. (2013). Antimicrobial potential of endophytic fungi derived from three seagrass species: Cymodocea serrulata, Halophila ovalis and Thalassia hemprichii. PLoS One 8:e72520. doi: 10.1371/journal.pone.0072520
Supaphon, P., Phongpaichit, S., Sakayaroj, J., Rukachaisirikul, V., Kobmoo, N., and Spatafora, J. W. (2017). Phylogenetic community structure of fungal endophytes in seagrass species. Bot. Mar. 60, 489–501. doi: 10.1515/bot-2016-0089
Taylor, D. L., Walters, W. A., Lennon, N. J., Bochicchio, J., Krohn, A., Caporaso, J. G., et al. (2016). Accurate estimation of fungal diversity and abundance through improved lineage-specific primers optimized for illumina amplicon sequencing. Appl. Environ. Microbiol. 82, 7217–7226. doi: 10.1128/aem.02576-16
Tedersoo, L., Sánchez-Ramírez, S., Kõljalg, U., Bahram, M., Döring, M., Schigel, D., et al. (2018). High-level classification of the Fungi and a tool for evolutionary ecological analyses. Fungal Divers. 90, 135–159. doi: 10.1007/s13225-018-0401-0
Torta, L., Lo Piccolo, S., Piazza, G., Burruano, S., Colombo, P., Ottonello, D., et al. (2015). Lulwoanasp., a dark septate endophyte in roots of Posidonia oceanica(L.) Delile seagrass. Plant Biology 17, 505–511. doi: 10.1111/plb.12246
UNITE Community (2019). UNITE general FASTA release for Fungi 2. Version 18.11.2018. doi: 10.15156/BIO/786353
Usuki, F., and Narisawa, K. (2007). A mutualistic symbiosis between a dark septate endophytic fungus, Heteroconium chaetospira, and a nonmycorrhizal plant, Chinese cabbage. Mycologia 99, 175–184. doi: 10.3852/mycologia.99.2.175
Van den Wyngaert, S., Rojas-Jimenez, K., Seto, K., Kagami, M., and Grossart, H.-P. (2018). Diversity and hidden host specificity of chytrids infecting colonial volvocacean algae. J. Eukaryot. Microbiol. 65, 870–881. doi: 10.1111/jeu.12632
Venkatachalam, A. (2015). Endophytic fungi of marine algae and seagrasses: a novel source of chitin modifying enzymes. Mycosphere 6, 345–355. doi: 10.5943/mycosphere/6/3/10
Venkatachalam, A., Thirunavukkarasu, N., and Suryanarayanan, T. S. (2015). Distribution and diversity of endophytes in seagrasses. Fungal Ecol. 13, 60–65. doi: 10.1016/j.funeco.2014.07.003
Vilgalys, R., and Hester, M. (1990). Rapid genetic identification and mapping of enzymatically amplified ribosomal DNA from several Cryptococcus species. J. Bacteriol. 172, 4238–4246. doi: 10.1128/jb.172.8.4238-4246.1990
Vohník, M., Borovec, O., and Kolařík, M. (2016). Communities of cultivable root mycobionts of the seagrass posidonia oceanica in the northwest mediterranean sea are dominated by a hitherto undescribed pleosporalean dark septate endophyte. Microb. Ecol. 71, 442–451. doi: 10.1007/s00248-015-0640-5
Vohník, M., Borovec, O., Kolaříková, Z., Sudová, R., and Réblová, M. (2019). Extensive sampling and high-throughput sequencing reveal gen. et sp. nov. (Aigialaceae, Pleosporales) as the dominant root mycobiont of the dominant Mediterranean seagrass. Mycokeys 55, 59–86. doi: 10.3897/mycokeys.55.35682
Vohník, M., Borovec, O., Župan, I., Kolarřík, M., and Sudová, R. (2017). Fungal root symbionts of the seagrass Posidonia oceanica in the central Adriatic Sea revealed by microscopy, culturing and 454-pyrosequencing. Mar. Ecol. Prog. Ser. 583, 107–120. doi: 10.3354/meps12337
Vohník, M., Borovec, O., Župan, I., Vondrášek, D., Petrtýl, M., and Sudová, R. (2015). Anatomically and morphologically unique dark septate endophytic association in the roots of the Mediterranean endemic seagrass Posidonia oceanica. Mycorrhiza 25, 663–672. doi: 10.1007/s00572-015-0642-7
Wainwright, B. J., Zahn, G. L., Arlyza, I. S., and Amend, A. S. (2018). Seagrass-associated fungal communities follow Wallace’s line, but host genotype does not structure fungal community. J. Biogeogr. 45, 762–770. doi: 10.1111/jbi.13168
Wang, B., and Qiu, Y.-L. (2006). Phylogenetic distribution and evolution of mycorrhizas in land plants. Mycorrhiza 16, 299–363. doi: 10.1007/s00572-005-0033-6
Wang, B., Yeun, L. H., Xue, J.-Y., Liu, Y., Ané, J.-M., and Qiu, Y.-L. (2010). Presence of three mycorrhizal genes in the common ancestor of land plants suggests a key role of mycorrhizas in the colonization of land by plants. New Phytol. 186, 514–525. doi: 10.1111/j.1469-8137.2009.03137.x
Wang, Q., Garrity, G. M., Tiedje, J. M., and Cole, J. R. (2007). Naive Bayesian classifier for rapid assignment of rRNA sequences into the new bacterial taxonomy. Appl. Environ. Microbiol. 73, 5261–5267. doi: 10.1128/aem.00062-07
Warton, D. I., Wright, S. T., and Wang, Y. (2012). Distance-based multivariate analyses confound location and dispersion effects. Methods Ecol. Evol. 3, 89–101. doi: 10.1111/j.2041-210x.2011.00127.x
Wickham, H. (2009). ggplot2: Elegant Graphics for Data Analysis. Berlin: Springer Science & Business Media.
Wigand, C., and Court Stevenson, J. (1994). The presence and possible ecological significance of mycorrhizae of the submersed macrophyte. Vallisneria americana. Estuaries 17:206. doi: 10.2307/1352570
Wissler, L., Codoñer, F. M., Gu, J., Reusch, T. B. H., Olsen, J. L., Procaccini, G., et al. (2011). Back to the sea twice: identifying candidate plant genes for molecular evolution to marine life. BMC Evol. Biol. 11:8. doi: 10.1186/1471-2148-11-8
Ye, J., Coulouris, G., Zaretskaya, I., Cutcutache, I., Rozen, S., and Madden, T. L. (2012). Primer-BLAST: a tool to design target-specific primers for polymerase chain reaction. BMC Bioinformatics 13:134. doi: 10.1186/1471-2105-13-134
Zapata, O., and McMillan, C. (1979). Phenolic acids in seagrasses. Aqua. Bot. 7, 307–317. doi: 10.1016/0304-3770(79)90032-9
Keywords: seagrass, Zostera marina, mycobiome, marine fungi, internal transcribed spacer, Lobulomycetales, Chytridiomycota
Citation: Ettinger CL and Eisen JA (2019) Characterization of the Mycobiome of the Seagrass, Zostera marina, Reveals Putative Associations With Marine Chytrids. Front. Microbiol. 10:2476. doi: 10.3389/fmicb.2019.02476
Received: 14 August 2019; Accepted: 15 October 2019;
Published: 01 November 2019.
Edited by:
Jonathan P. Zehr, University of California, Santa Cruz, United StatesReviewed by:
Teppo Rämä, Arctic University of Norway, NorwayMaiko Kagami, Yokohama National University, Japan
Copyright © 2019 Ettinger and Eisen. This is an open-access article distributed under the terms of the Creative Commons Attribution License (CC BY). The use, distribution or reproduction in other forums is permitted, provided the original author(s) and the copyright owner(s) are credited and that the original publication in this journal is cited, in accordance with accepted academic practice. No use, distribution or reproduction is permitted which does not comply with these terms.
*Correspondence: Cassandra L. Ettinger, Y2xldHRpbmdlckB1Y2RhdmlzLmVkdQ==