- 1Department of Environmental Engineering, College of Environmental & Resource Sciences, Zhejiang University, Hangzhou, China
- 2Advanced Environmental Biotechnology Centre, Nanyang Environment & Water Research Institute, Nanyang Technological University, Singapore, Singapore
- 3Key Laboratory of Offshore Geotechnics and Material of Zhejiang Province, College of Civil Engineering and Architecture, Zhejiang University, Hangzhou, China
- 4Department of Environmental Science, College of Resources and Environmental Science, Chongqing University, Chongqing, China
Shallow gas is a kind of natural gas buried in shallow strata, generally, with methane as the main component, endowing it a potential energy resource while also a potential risk to the safety of ground engineering and environment. Microbial activity is usually regarded as an important driving force to generate shallow gas via metabolizing the environmental substrates. Therefore, the research on the microbial communities will be helpful to reveal the distribution of shallow gas in the gas-bearing formation. In this study, 30 sediment samples below the seabed in Hangzhou Bay (China) from depths of 1.5 m to 55 m were collected to investigate their microbial community, environmental characteristics and sediment type (clay or sand). It turned out that the presence of shallow gas had a good correlation with the distribution of archaea rather than bacteria, with the dominant microbe of Bathyarchaeota, Thaumarchaeota, and Euryarchaeota in the formation. Methanosarcinaceae and ANME-1a with the capacity of methane metabolism occupied high proportions. The correlation analysis and redundancy analysis (RDA) suggested that ammonium was a key environmental substrate to indicate the microbial community in the formation. The sediment type was proposed to shape environmental substrates in the formation, thus further affecting the microbial communities. The clay strata were demonstrated to have an important role in the generation and distribution of shallow gas, and more attention should be paid in terms of its resource discovery and engineering safety assessment.
Introduction
Shallow gas, with methane as the main component, is a kind of natural gas buried in shallow formation (within the topmost 1000 m of sediment) (Davis, 1992; Roy et al., 2019). Its reserves are plentiful globally. There are many gas reservoirs with exploitation value in the Black Sea (Xing and Spiess, 2015) and Arctic Ocean (Rajan et al., 2013). However, due to the shallow distribution in the formation, shallow gas is easy to be affected by the disturbance of environment and thus release from the sediment, resulting in the risks of eruption and leakage (Gentz et al., 2014; Vielstädte et al., 2015), and engineering safety (Xu et al., 2017). On the other hand, methane is reported as a potent greenhouse gas with the warming potential of 20–40 times that of carbon dioxide (Shindell et al., 2009; Zhang et al., 2019). Therefore, the released shallow gas with high content of methane will potentially intensify the greenhouse effect.
Microorganisms are regarded as an important driving force to form shallow gas, especially in the marginal seas, e.g., estuary and coastal zone, which are characterized by high nutrient inputs from the land (Liu et al., 2015). Nitrogen- and sulfur-bearing organic matter (OM) is typically abundant in estuarine sediments (Wakeham et al., 1997; Burdige, 2005), and could be further converted to shallow gas by microorganisms in the strata. According to previous research, archaea are believed to play an important role in the substrate conversion in the marine sediment. For example, Bathyarchaeota are widespread in marine sediments (Meng et al., 2014), and can metabolize complex OM (Lloyd et al., 2013; Yu et al., 2018). Thaumarchaeota are closely related to nitrogen metabolism, and are more abundant in the marine sediment. Note that, most of the newly reported ammonia oxidizing archaea (AOA) belong to this phylum (Santoro et al., 2015). Euryarchaeota contain the largest group of methanogens, and are capable of converting OM to methane gas (Lang et al., 2015). While the anaerobic methanotrophic (ANME) were reported to couple with sulfate reducing bacteria (SRB), and could anaerobically oxidize methane to low molecular weight OMin marine sediments (Hinrichs et al., 1999; Holler et al., 2011; Wang et al., 2014; Wegener et al., 2015). Additionally, bacteria, such as Proteobacteria, Firmicutes, Actinobacteria, Marinobacter, and Alcanivorax, are also reported to be involved in the transformation of substrates in the marine sediment (Kimes et al., 2014; Kleindienst et al., 2015; Zhang et al., 2015).
Previous studies on microbial communities in sediments usually focused on the shallow surface within a single sediment type (Hu et al., 2014; Yu et al., 2018). However, the deep sediments had been revealed with several different types of sediment layers, where the microbial communities were quietly diverse (Inagaki et al., 2003; Nunoura et al., 2016). The sediment type in the vertical dimension is also an important factor that can affect the distribution of both substrate and shallow gas in the formation, leading to a different microbial structure.
The Hangzhou Bay is the largest estuary in China, and receives a lot of terrestrial substrates from the Yangtze River and the Qiantang River (QR) every year (Xu, 2016). Since the last glaciation, with the rise and fall of sea level, the QR incised valley underwent three stages: (1) deep-cutting stage; (2) rapid-filling stage; and (3) burial stage. Due to the variation of sedimentary environment, quite different rock strata such as sand and clay deposited vertically in the QR incised valley (Lin et al., 2005; Zhang et al., 2013). Because of the rapid deposition rate (2–3 cm/a) (Lin et al., 2005) and rich substrate in the sediment, the gas reservoirs are shallow and widespread, and thus provide a typical case to investigate the relationship among the microbial community, environmental substrates and sediment type.
In the present work, the sediment samples below seafloor in a range of 0–35 m were collected near Yushan Island, Hangzhou Bay, China. Based on the methane content in situ (main gas component with a proportion over 95%), the strata were divided into gas-bearing layer and gas-free layer. The microbial community, environmental substrates and sediment type in both layers were clarified. A comparison analysis among the microbial community, environmental substrate and sediment type in the gas-bearing layer was carried out. The information gathered in the present work demonstrated the core role of microbe in the formation of shallow gas and revealed the unique microbial structure in the gas-bearing layer.
Materials and Methods
Collection of Samples
A total of 30 samples were collected from six sites around the Yushan Island (Hangzhou Bay) of Zhejiang Province, China, in spring 2017. The detail information of sampling sites was shown in Figure 1 and Supplementary Table S1. The samples were collected vertically through drilling, and the drill core at each site was collected. Each sample was divided into two parts, one was stored at 4°C for microbial community analysis and inorganic nitrogen measurement, the other was air-dried for the physicochemical analysis.
Analysis of Environmental Substrates
Ammonium, nitrite, and nitrate were extracted from wet sediments using 2 M KCl (Shen et al., 2013; Hu et al., 2014), and determined according to the standard methods (APHA, 2017). Air-dried sediment samples were sifted for the analysis of other physical and chemical properties (passed through an 18-mesh sieve for the determination of moisture and sulfate, passed through a 100-mesh sieve for the determination of OM and nutrient elements). The moisture of air-dried sediment was determined by the stoving method. The content of soil OM was determined by the K2Cr2O7 oxidation method. Sulfate was extracted by water (the water-soil ratio was 5:1) and was determined by ion chromatography (DIONEX ICS-1000, America). The content of total carbon (TC), nitrogen (TN), and sulfur (TS) was determined using the elemental analyzer (elementar vairo EL CUBE, Germany).
Measurement of Methane Content
The methane content of sediment samples was determined using Static Head Space Method. Intact core was collected immediately using ring-knife when the sediment column was taken out. 3.0 mL sediment samples were put into serum bottles with 20 mL headspace. 6 mL NaOH (1 M) were added into the serum bottle to inhibit methanogenesis. The serum bottles were sealed with butyl rubber stoppers. They were oscillated at 25°C until equilibrium. The methane content in the headspace was determined with Trace GC Ultra gas chromatograph (Thermo Fisher Scientific, United States). The methane content of sediments was calculated using the following equation:
where c(CH4) is the methane concentration in the headspace (in μg/mL), Vheadspace is the volume of the serum bottle headspace (mL) after the adding of sediment and NaOH, and Vsediment is the volume (mL) of sediment added to the serum bottle. The temperature was 25°C, and the pressure in the serum bottle was assume as one bar (Johnson et al., 1990; Lloyd et al., 2011; Li et al., 2012).
DNA Extraction and Sequencing
Total DNA was extracted using a FastDNA SPIN kit for soil (MP biochemicals, United States) according to the manufacturer’s protocol from 0.25 g sediments. Then, 16S rRNA genes were amplified using the bacterial and archaeal primer sets 338F (5′-ACTCCTACGGGAGGCAGCAG-3′)/806R (5′-GGACTACHVGGGTWTCTA AT-3′) and Arch344F (5′-ACGGGGYGCAGCAGGCGCGA-3′)/Arch915R (5′-GTGCTCCCCCGC CAATTCCT-3′). The product of PCR was examined using 2.0% agarose gel electrophoresis, and the purification recovery was conducted on the targeted strips which appeared in the agarose gel using SanPrep Column PCR Product Purification Kit (Sangon Biotech., Shanghai, China). The purified 16S rRNA gene amplicons were sequenced on the Illumina MiSeq platform by MajorBio, Shanghai. Quality control, sequences clustering and OTU taxonomic annotation (Silva database) were completed on I-Sanger Bioinformatics cloud platform1. In addition, α-diversity indexes including Sobs (number of OTU observed), Ace, Chao1; Shannoneven, Simpsoneven (a Shannon/Simpson index-based measure of evenness); Shannon and Simpson were calculated on that platform. The gene sequences obtained in this study are available in NCBI: PRJNA541126 and PRJNA542038.
Statistical Analyses
Strata were divided into gas-bearing layer and gas-free layer based on the methane content. Meanwhile, they were divided into clay stratum and sand stratum according to their texture (Figure 2). T-test (SPSS, 22.0) was used to compare the differences of the microbial community (α-diversity indexes and relative abundance of dominant groups) and environmental factors in different strata. The correlation analysis was completed with the corrplot R package. Redundancy analysis (RDA) was used to explore the relationship between response variables (i.e., relative abundance of archaea at family level or bacteria at genus level) and explanatory variables (methane and environmental substrates such as OM, Ammonia-N). RDA was calculated with the vegan package and plotted using the ggplot2 package. Chi-square test of shallow gas and sediment type was completed with function Mosaic in the vcd package and showed as Mosaic plot. Other plots were completed using software Origin (V 8.0).
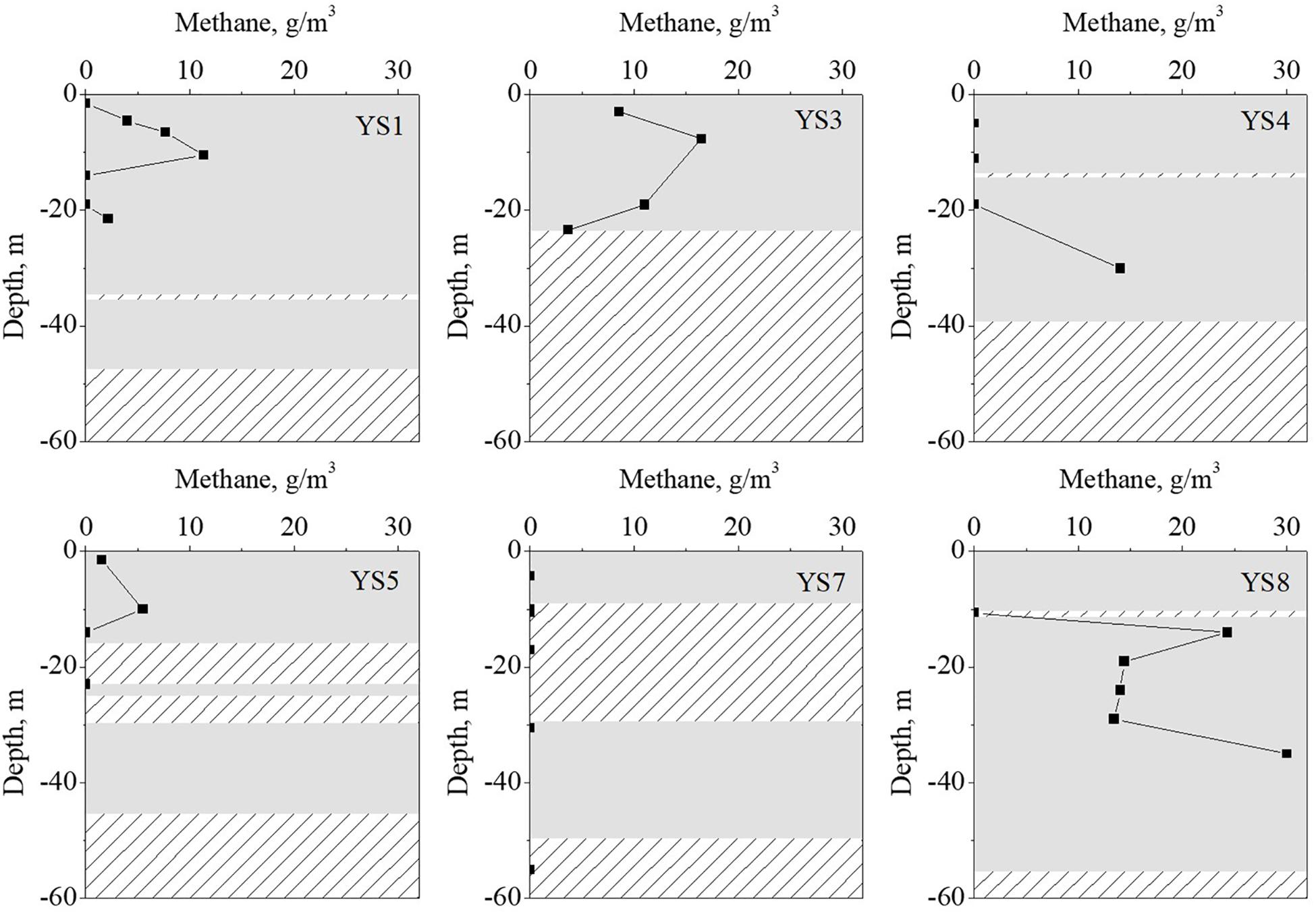
Figure 2. The methane content and sediment type of strata with difference depth. The gray rectangles represent the clay strata, rectangles filled with lines represent the sand strata.
Results
Methane and Environmental Substrates in the Formation
The vertical distribution of the methane in the sediment cores are shown in Figure 2. The methane concentrations were in the range of 0–30.1 g/m3, which were similar to the values reported in previous literature (Lloyd et al., 2011; Li et al., 2012; Hu et al., 2014; Tong et al., 2017). The environmental substrates of the sediment samples were determined, and the results are shown in Figure 3 (detail information can be found in Supplementary Table S2). The OM was the dominant substrate in the sediments, and was in a range of 1.61–10.52 g/kg-DW (dry weight) with 6.45 g/kg-DW on average. Ammonia-N was the main form of inorganic nitrogen with contents of 0.004–0.92 g/kg-WW (wet weight) and 0.23 g/kg-WW on average. The contents of nitrite-N and nitrate-N were much lower than that of ammonia-N, and even below the detect limitation. Hygroscopic water content (moisture) was in a range of 1.85–5.13% with 3.60% on average. Further analysis was carried out to reveal the correlation among the environmental substrates and the results (shown in Supplementary Figure S1) demonstrated a significantly positive correlation between ammonia-N, TC, TN, moisture and OM. It is worth noting that the correlation between TN and OM (r = 0.81) was stronger than that between ammonia-N and OM (r = 0.42). It should be noted that methane was positively correlated with ammonia-N (r = 0.8), but not with OM (r = 0.3).
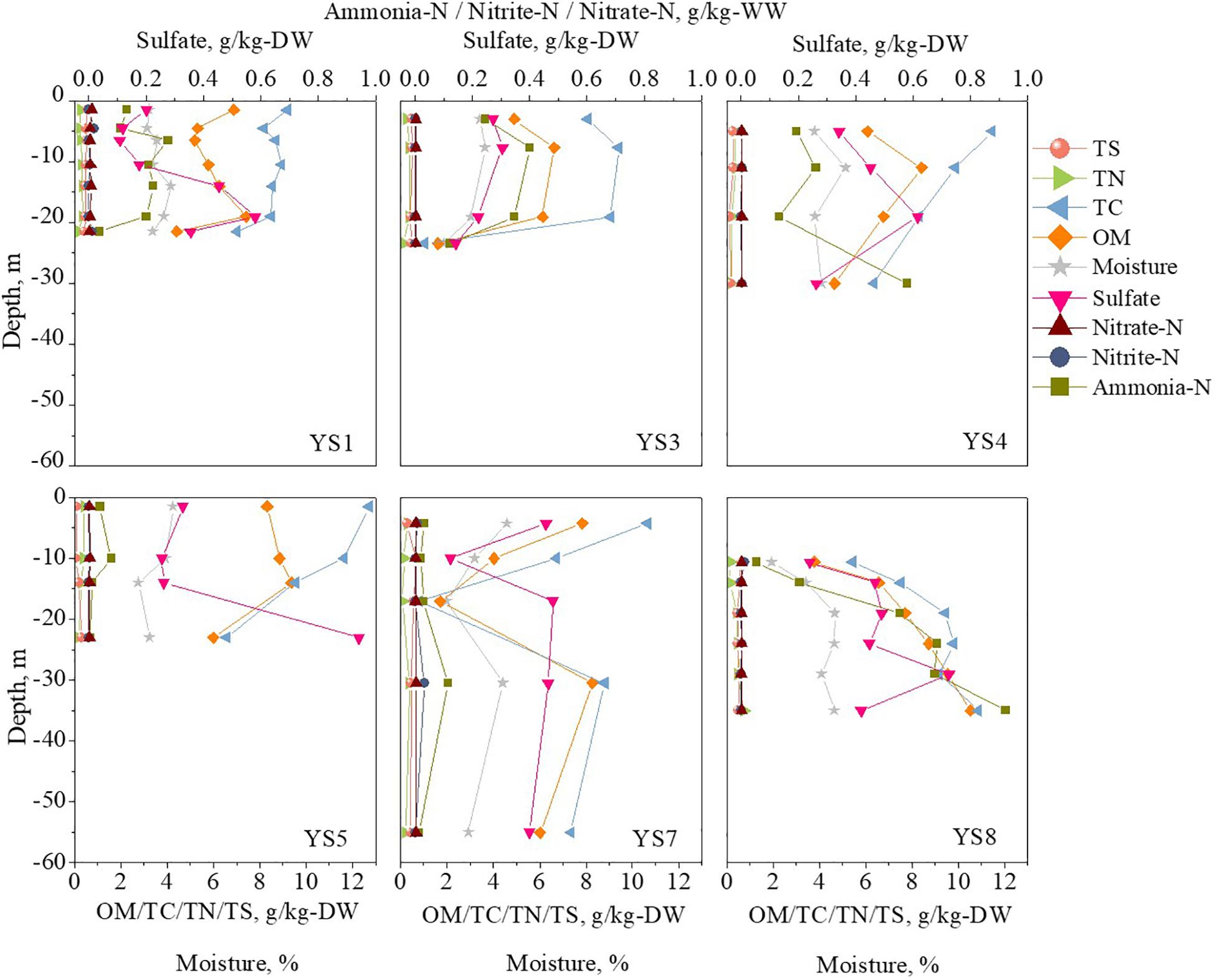
Figure 3. The vertical profiles of environmental factors (Data were shown in Supplementary Table S2).
Microbial Communities in the Formation
The microbial communities of the sediment samples were analyzed using 16S rRNA gene high-throughput sequencing, and the results are showed in Figure 4. In the archaeal domain, 17 phyla were detected, Bathyarchaeota, Thaumarchaeota, and Euryarchaeota were the dominant phyla with average relative abundance of 66.34, 20.37, and 5.29%, respectively (Figure 4A). Bathyarchaeota were the most abundant in 26 samples, while Thaumarchaeota were detected as the dominant microbe in the samples from deep of site YS8 (Supplementary Figure S2a). At the class level, Bathyarchaeota, a novel discovered branch in the archaeal domain, cannot be further identified due to lack of reference. Group C3 were the dominant class in Thaumarchaeota (Supplementary Figure S2a). The distinguishability of Euryarchaeota was better than that of the first two phyla. At the class level, Methanomicrobia or Thermoplasmata were the dominant class, and could be further identified as Marine Benthic Group D (MBGD), ANME-1a, Methanosarcinaceae, MKCST-A3 and ANME-1b at the family level with relative abundances of 29.18, 18.49, 16.43, 11.95, and 8.51%, respectively.
In the bacterial domain, 128 genera affiliated into 44 phyla were detected. Proteobacteria, Bacteroidetes, Firmicutes, Chloroflexi, and Actinobacteria were dominant phyla with average relative abundances of 77.88, 9.13, 5.74, 2.65, and 0.95%, respectively (Figure 4B). At genus level, Pseudomonas, Thioalkalimicrobium, Marinobacter, and Sulfitobacter were the top 4 genera, with average relative abundances of was 7.96, 7.31, 3.96, and 3.94%, respectively. It is worth noting that 16 out of the 20 most abundant genera belong to Proteobacteria.
Relationship Between Microbial Community and Shallow Gas
Microorganisms are regarded as an important driving force to form shallow gas. The characteristics of the microbial community in the gas-bearing and gas-free layers was further analyzed, and the results are showed in Figure 5.
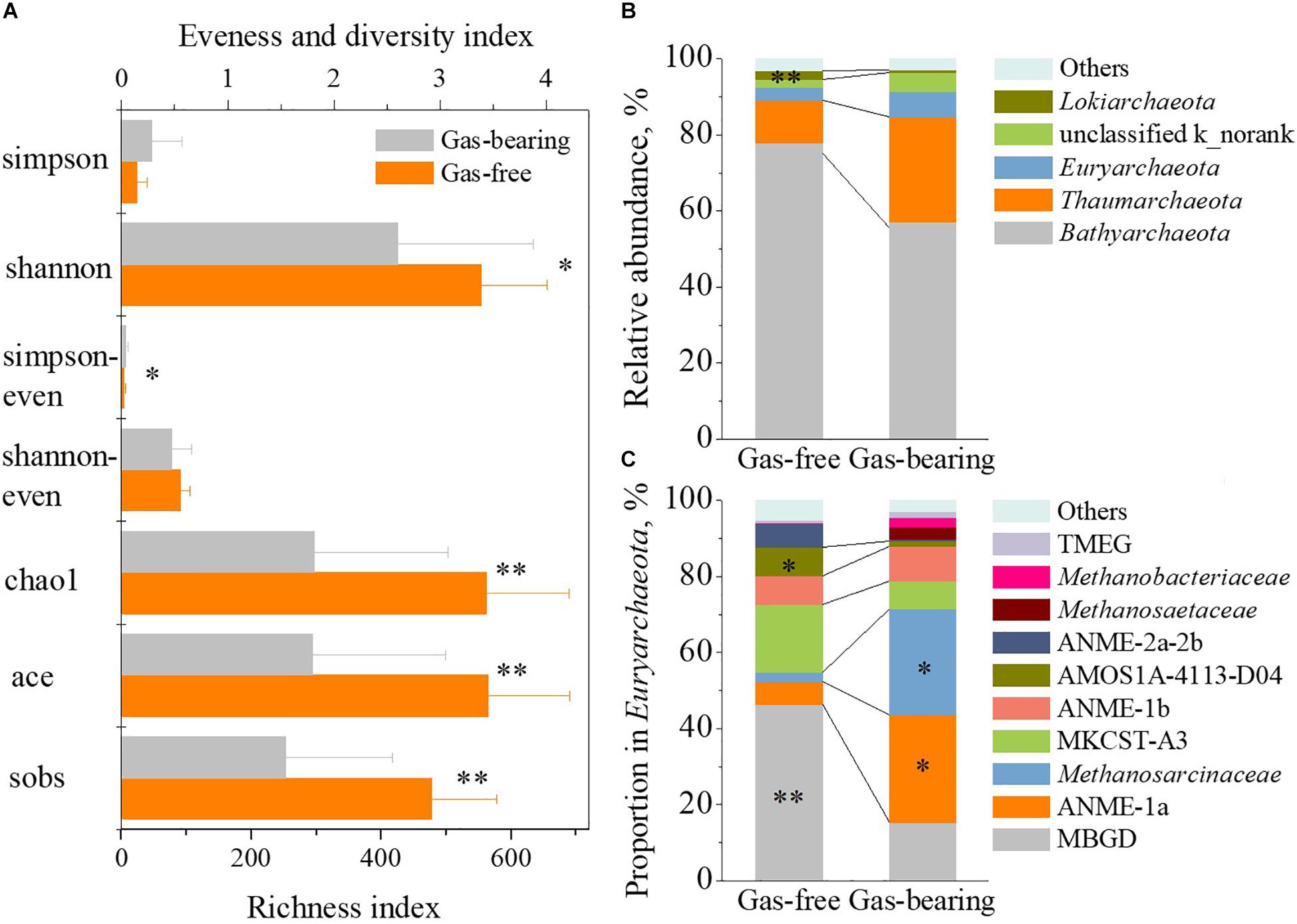
Figure 5. The difference of archaeal community between strata with shallow gas or not. The difference of α-diversity indexes (A), relative abundance of archaeal phylum (B) and proportion in Euryarchaeota at family level (C) between gas-bearing layers and gas-free layers (asterisk: the level of significance. “∗” represents p < 0.05, “∗∗” represents p < 0.01).
In archaeal domain, the results of α-diversity analysis (Figure 5A) showed significant lower species richness (p < 0.01), evenness (p < 0.05) and diversity (p < 0.05) of the archaeal community in the gas-bearing layers than those in the gas-free layers. In detail, no statistically significant differences were observed for Bathyarchaeota, Thaumarchaeota, and Euryarchaeota at the phylum level (Figure 5B). Note that, among the members of Euryarchaeota, the proportion of MBGD in gas-bearing layers was 15.19% on average, which was significantly lower than that in gas-free layers (46.41%, p < 0.01). The proportion of ANME-1a was 28.54% in gas-bearing layers, which was 3.7 times higher than that in gas-free layers (6.12%, p < 0.05). The proportion of Methanosarcinaceae was 27.79% in gas-bearing layers, which was 10.3 times higher than that in gas-free layers (2.46%, p < 0.05) (Figure 5C). Consequently, the statistical analysis results indicated a good correlation between archaeal community and shallow gas.
In the bacterial domain, the results of α-diversity analysis (Supplementary Figure S3a) showed a lower species richness (p < 0.05) of bacterial community in the gas-bearing layers than that in the gas-free layers. In detail, only the relative abundance of Shewanella in the gas-bearing layers (2.30%) at the genus level was significantly different (p < 0.05) from that in the gas-free layers (0.25%) (Supplementary Figure S3b). That is to say, the correlation between shallow gas and bacterial community was poor.
Relationship Between Microbial Community, Methane and Environmental Substrates
Relationship Between Archaea, Methane and Environmental Substrates
It is well-known that environmental substrates have a great influence on the metabolism of microbe, and thus affect the characteristics of microbial communities in the formation. The results showed that the α-diversity of archaea was negatively correlated with multiple environmental substrates (Figure 6). The correlation between α-diversity and ammonia-N was the strongest., whereas the species richness (Sobs, r = −0.83, p < 0.01; Ace, r = −0.81, p < 0.01; Chao1, r = −0.82; p < 0.01), evenness (Shannoneven, r = −0.74, p < 0.01; Simpsoneven, r = 0.56, p < 0.01) and diversity (Shannon, r = −0.80, p < 0.01; Simpson, r = 0.82, p < 0.01) were negatively correlated with ammonia-N. α-diversity and methane had a similar but weaker correlation with α-diversity and ammonia-N. It should be noted that the species richness (Sobs, r = −0.69, p < 0.01; Ace, r = −0.68, p < 0.01; Chao1, r = −0.67; p < 0.01), evenness (Shannoneven, r = −0.42, p < 0.05; Simpsoneven, r = 0.58, p < 0.01) and diversity (Shannon, r = −0.51, p < 0.01; Simpson, r = 0.53, p < 0.01) were negatively correlated with methane.
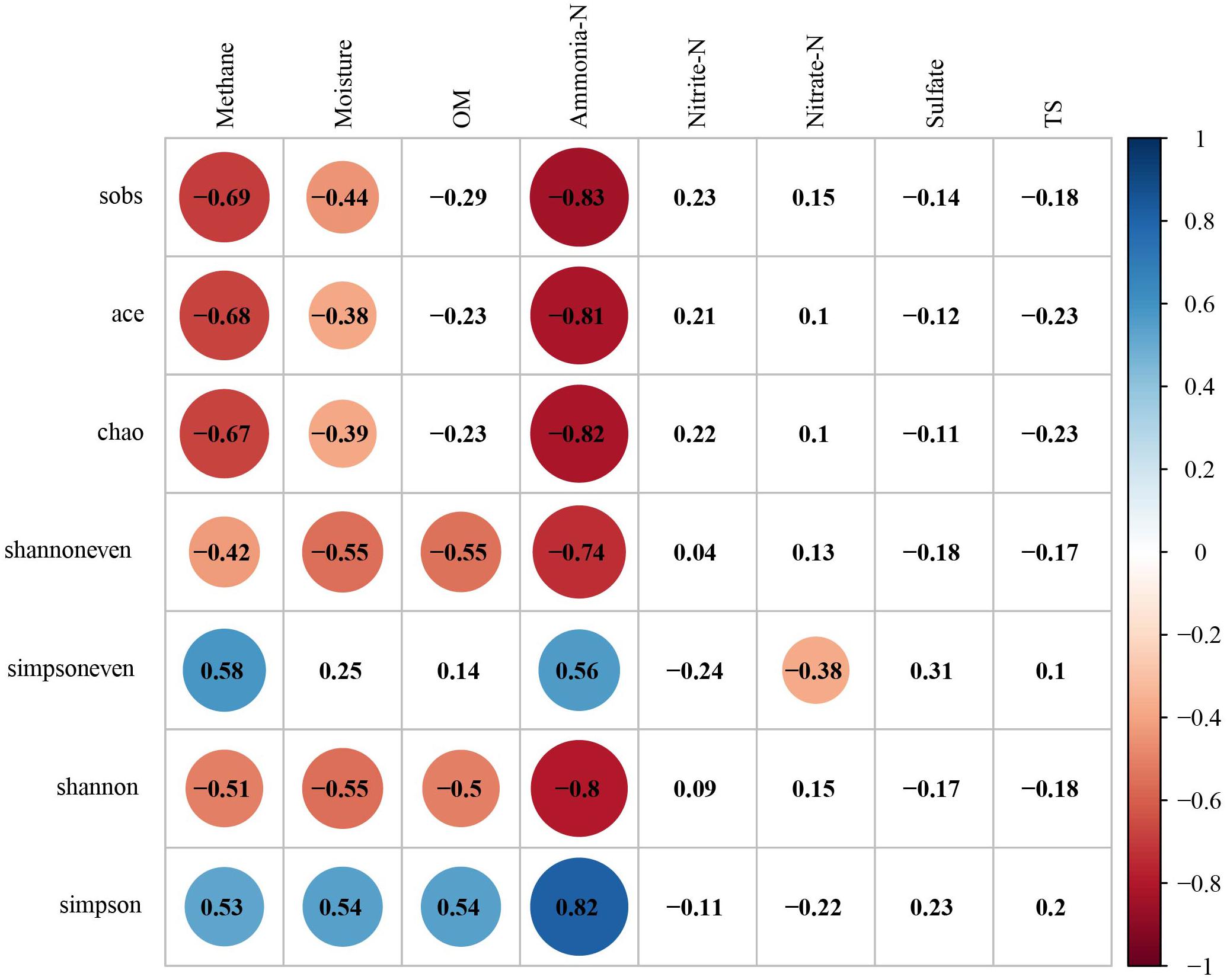
Figure 6. The correlation analysis between the species diversity of archaeal community, methane and environmental factors (Digits represents Pearson correlation coefficients; filled color indicates significant correlation, blue represents positive correlation, red represents negative correlation, shade of color represents strength of correlation).
The top 10 families affiliated to Bathyarchaeota, Thaumarchaeota or Euryarchaeota were used to analyze the variation of relative abundance of archaea in response to methane and environmental substrates by RDA (Figure 7). The Monte Carlo permutation test showed that up to 67.43% of the variance in the relative abundance of dominant archaeal families could be explained by methane and environmental substrates (with 999 permutations, p = 0.002). Among all the explanatory variables, ammonia-N, methane, OM, moisture and nitrate had a significant effect on the species dominance of archaeal community (Supplementary Table S3). The relative abundance of dominant groups had the strongest correlation with ammonia-N, which was consistent with the correlation analysis of α-diversity and environmental factors. As the main methanogens in the formation, Methanosarcinaceae was positively correlated with ammonia-N and methane.
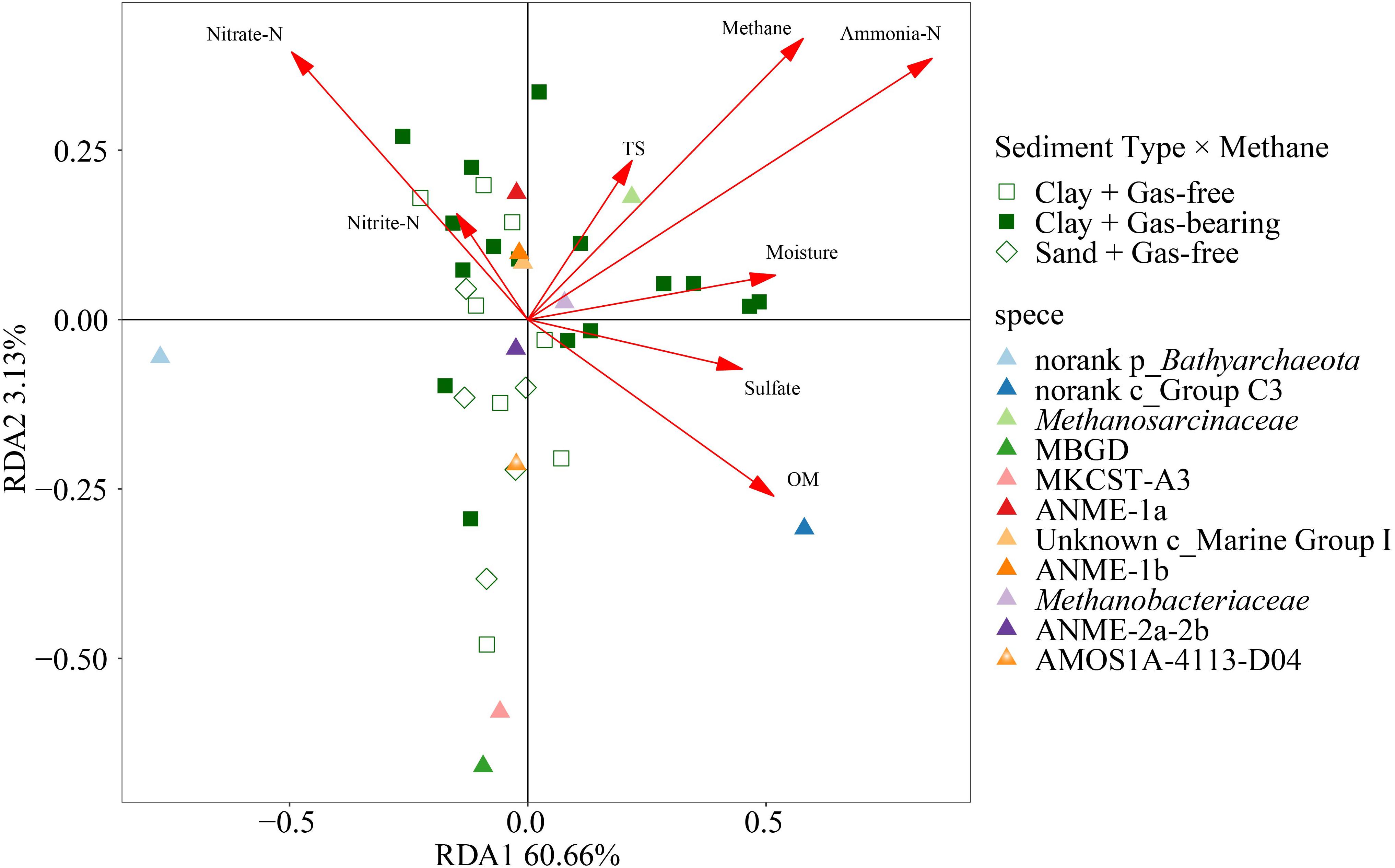
Figure 7. Ordination plot of redundancy analysis (RDA) for the dominant archaeal families with methane and environmental substrates as constraining variables.
Relationship Between Bacteria, Methane and Environmental Substrates
It was found that the environmental substrates had a weak influence on the α-diversity of bacterial community (Supplementary Figure S4). Among all the environmental substrates, the correlation between species diversity and ammonia-N was the strongest. The species richness (sobs, r = −0.57, p < 0.01; ace, r = −0.59, p < 0.01; chao1, r = −0.58; p < 0.01) and diversity (Shannon, r = −0.46, p < 0.05) were negatively correlated with ammonia-N significantly.
The top 20 genera were used to analyze the variation of relative abundance of bacteria in response to methane content and environmental substrates by RDA (Supplementary Figure S5). The Monte Carlo permutation test showed that up to 43.30% of the variance in the relative abundance of dominant bacterial genera could be explained by environmental factors (with 999 permutations, p = 0.007). Among all the explanatory variables, ammonia-N, methane and nitrate had a significant effect on the species dominance of bacterial community (Supplementary Table S4). The relative abundance of dominant groups had a strongest correlation with ammonia-N, which was consistent with the correlation analysis of α-diversity and environmental factors. It is worth noting that the sand samples were clustered into an independent group in the RDA plot.
Relationship Between Microbial Community and Sediment Type
The type of sediment characterizes the physicochemical properties of strata and could affects the distribution of substrates and thus affects the microbial community. Based on soil particle gradation, the strata can be divided into the clay and sand strata. Their α-diversity and dominant species of the microbial community were further analyzed to reveal the influence of texture on microbial community.
As to the archaea, the analysis of α-diversity (Figure 8A) showed that the Sobs, Ace and Chao1 indexes were significantly lower (p < 0.01) in clay strata than those in sand strata, which indicated a lower species richness of archaea in clay strata. A significantly lower Shannoneven index (p < 0.01) suggested a lower species evenness of archaea in the clay strata. Meanwhile, the Shannon index was lower (p < 0.01), while the Simpson index was higher (p < 0.01) in clay strata, implied a lower species diversity of archaea. The analysis of species dominance (Figures 8B,C) showed that, among the members of Euryarchaeota, the proportion of Methanosarcinaceae in clay strata was 19.43% on average, which was 8.57 times higher than that in sand strata (2.03%, p < 0.05).
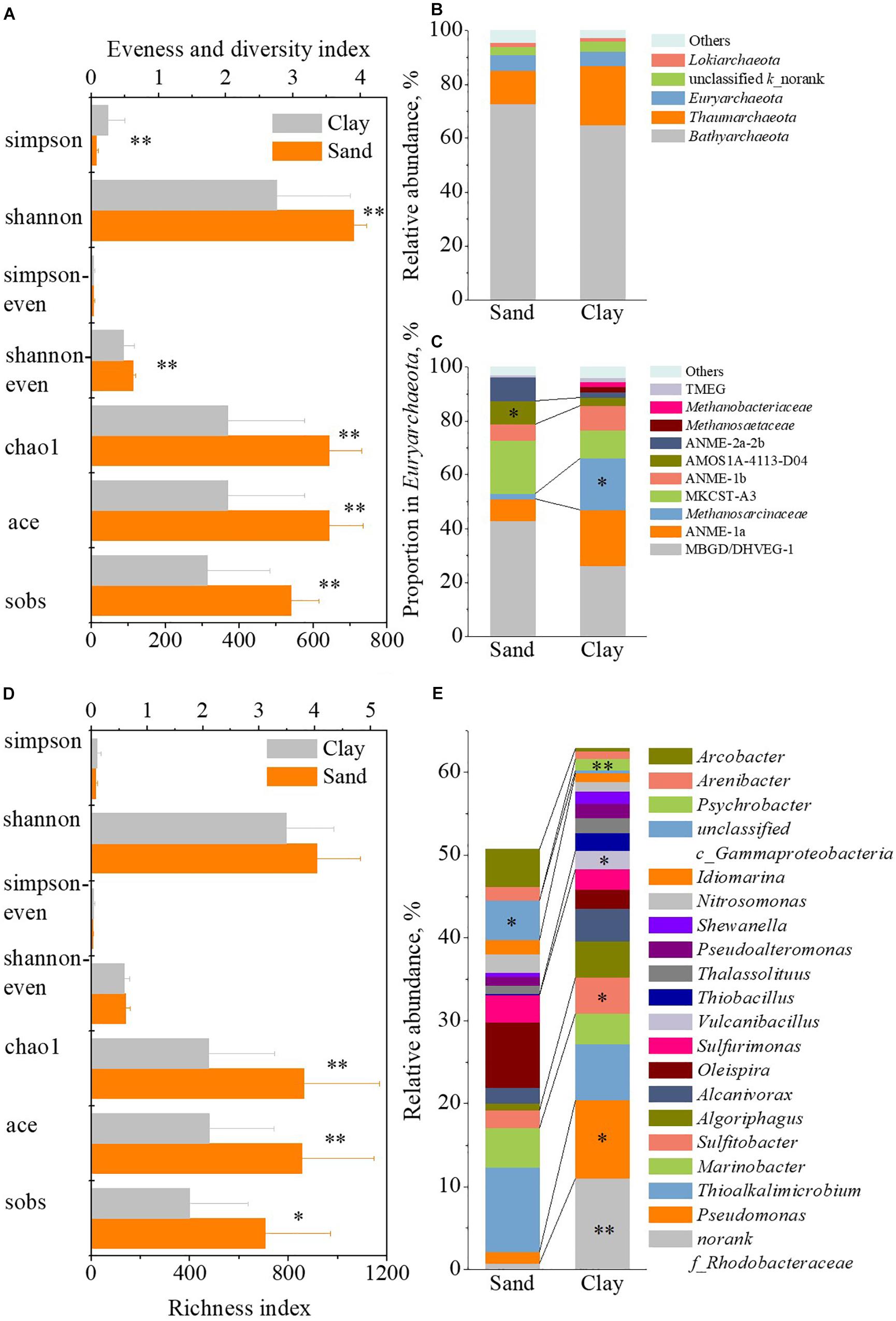
Figure 8. The difference of microbial community between clay strata and sand strata. The difference of α-diversity indexes of archaeal community (A), relative abundance of archaeal phylum (B), proportion in Euryarchaeota at family level (C), α-diversity indexes of bacterial community (D), and relative abundance of bacterial genus (E) between sand strata and clay strata.
As to the bacteria, the analysis of α-diversity (Figure 8D) showed that the Sobs, Ace and Chao1 indexes were significantly lower (p < 0.05 for Sobs index; 0.01 for Ace and Chao1 index) in clay strata than those in sand strata, which suggested the lower species richness of bacteria in clay strata. The analysis of species dominance (Figure 8E) showed that the relative abundance of Pseudomonas (9.47% in average, p < 0.05), Sulfitobacter (4.35% in average, p < 0.05), Vulcanibacillus (2.19% in average, p < 0.05) and Psychrobacter (1.35% in average, p < 0.01) were significantly higher than those in sand strata (0.77, 1.34, 2.14, 0.01, and 0.04%, respectively).
Discussion
Characteristics of Methane and Environmental Substrates Distribution in Hangzhou Bay
The Hangzhou Bay is the largest estuary in China, and receives masses of terrestrial substrates from the Yangtze River and the QR every year (Xu, 2016). Hindered by Zhoushan Islands, the substrates are easily deposited in the bay. In this study, most of the collected sediment samples contained higher OM than the lower limit for potential terrestrial gas sources (3.1 g/kg) (Zhou et al., 1994), and only a few samples contained higher OM than the lower limit for potential marine gas sources (8.6 g/kg) (Dudley and George, 1981) (Supplementary Figure S6). Yushan Island is located at the edge of the QR incised valley, and the formation is mainly consisted of shallow marine sediments. As such, it is difficult to form large-scale shallow gas reservoirs compared with other formation dominated by floodplain sediments (Zhang et al., 2013). The accumulation of ammonium in strata indicated a weak nitrification. TN showed a close relationship with OM in the formation, which was accordant with the fact that organic nitrogen is the main form of soil nitrogen. However, the correlation between ammonia-N and OM was weak (r = 0.42), which might be ascribe to the hypothesis of that ammonium originated from the OMs consumed in geological period. Similarly, methane and ammonia-N (r = 0.8) in the formation have a stronger correlation than that of methane and OM (r = 0.3), which can also contribute to the hypothesis mentioned above.
As shown in Supplementary Figure S7, ammonia-N in gas-bearing layers was 0.34 g/kg-WW on average, which was significantly higher than that in gas-free layers (0.10 g/kg-WW, p < 0.01). Moisture, OM, TC, TN and ammonia-N in clay strata were significantly higher than those in sand strata (p < 0.05 for OM and p < 0.01 for others). These results demonstrated that sediment type had a strong influence on the environmental substrates distribution, which might be overlooked.
Characteristics of Microbial Community in Hangzhou Bay
Bathyarchaeota were the dominant archaea in most of sediment samples in Hangzhou Bay, which was similar with the microbial community in other researches of marine sediments (Sorensen and Teske, 2006; Webster et al., 2014; Meador et al., 2015). Bathyarchaeota were widespread in natural habitats (Meador et al., 2015), especially in marine sediments (Meng et al., 2014). Bathyarchaeota were capable of heterotrophic metabolism (Biddle et al., 2006; Evans et al., 2015), and had been suggested to play an important role in the breakdown of complex OMs such as detrital proteins (Lloyd et al., 2013) and lignin (Yu et al., 2018).
The AOA belonging to Thaumarchaeota are the most well-studied archaeal group (Santoro et al., 2015), which are the main ammonia oxidizers in the marine environment (Zheng et al., 2013). However, AOA were lacking in sampling sites, which explained the accumulation of ammonium. Euryarchaeota contain the largest variety of methane related archaea. As a subgroup of Euryarchaeota, Methanosacinaceae were the most abundant in the formation (Supplementary Figure S2a), which were believed to act methanogenesis from methyl compounds such as methylamines and methyl sulfides. Additionally, it was found that methylotrophic methanogens incorporate the majority of dissolved inorganic carbon into lipids in marine sediment (Yin et al., 2019). By contrast, the relative abundance of hydrogenotrophic methanogens, e.g., Methanobacteriaceae, was negligible in the formation, which is possibly due to the low hydrogen concentration in anaerobic sediment (Kessler et al., 2019) and strong competition of SRB over hydrogen. A lot of AOM were found in the formation, and ANME-1a was the most abundant group. ANME could oxidize methane in anaerobic environment (Wang et al., 2014), which was thought to act sulfate-coupled AOM cooperating with SRB (Wegener et al., 2015).
Proteobacteria was the dominant bacteria in the formation, which has been confirmed in many previous studies (Inagaki et al., 2003). Followed by Bacteroidetes and Firmicutes, which were thought to be the key bacterial communities in the acidogenic process of OM (Li et al., 2019). In this study, the genera with high relative abundance such as Pseudomonas and Algoriphagus were heterotrophic. In addition, many genera, e.g., Algoriphagus (agar) and Arenibacter (alginic acids) are capable of degrading complex OMs to simple compounds. This in turn indicated that the hydrolysis of macromolecular OM might be one of the key steps for the overall carbon mineralization process in the sediment (Flury et al., 2016). SRB, e.g., Desulfuromonas and Desulfuromonas, which could utilize methanogenic substrates such as hydrogen and acetate, were also abundant in the sediment. However, the common syntrophic and fatty acid oxidizing bacteria, e.g., Syntrophobacter and Desulfovibrio, were deficient. More efforts should be devoted on this aspect in future work. Meanwhile, the relative abundance of ammonia oxidizing bacteria (AOB) was low in the formation. Only Nitrosomonas were abundant in several sample, e.g., 7–26 (16.11%), 7–8 (8.21%), and 5–8 (6.82%) (Supplementary Figure S2b). This result confirmed the fact that nitrification is weak in the studied strata.
Generally, the abundance of microbes with methanotrophic function, e.g., Methanosacinaceae, ANME-1a in Euryarchaeota were accordant to the distribution of shallow gas. Although the proportion of methanogen in the Euryarchaeota was significantly positively correlated with methane (Methanosacinaceae, r = 0.42; Methanosaetaceae, r = 0.41; Methanobacteriaceae, r = 0.44), but all were weaker than their respective correlation with ammonia-N (Methanosacinaceae, r = 0.6; Methanosaetaceae, r = 0.46; Methanobacteriaceae, r = 0.64) (Supplementary Figure S8), Meanwhile, weaker than the correlation between ammonia-N and methane (Supplementary Figure S1).
Ammonium-a Key Environmental Substrates to Indicate the Microbial Community in Hangzhou Bay
In this work, ammonium was the most important substrate associated with microbial community in the gas-bearing formation in Hangzhou Bay. In theory, ammonium does not support microbial community directly besides being used as nitrogen source and energy source, but it can indicate the decomposition of nitrogenous OMs. In the formation, nitrogenous OMs are converted into methane, carbon dioxide (biogas) and ammonium by heterotrophic microorganisms. Due to the absence of ammonia oxidizers (see section “Characteristics of Microbial Community in Hangzhou Bay”) and the adsorption of ammonium by clay minerals, the ammonium would accumulate. In this regard, the ammonium concentration in situ could present the degree of heterotrophic degradation of OMs.
Effect of Sediment Type on the Microbial Community
The sediment type may affect the distribution of shallow gas and substrates in the formation. The Chi-square test (Supplementary Figure S9) showed a close relationship between shallow gas and sediment type (p < 0.05), and the shallow gas was revealed to be favorable to present in the clay strata in Hangzhou Bay.
Moreover, the characteristics of sediment would have similar effect on the distribution of environmental substrates. In this regard, the sediment type could greatly affect the microbial communities. Clay strata contained more OMs and other substrates than sand strata, triggering the enrichment of Methanosacinaceae, resulting in a greater methanogenic potential. Additionally, heterotrophic bacteria also occupied high relative abundance in clay strata, e.g., Pseudomonas, Sulfitobacter, Vulcanibacillus, and Psychrobacter. These results illustrated that the clay strata had a great metabolic potential of OMs, and was the main gas producing layer. Therefore, the gas potential in these strata deserved more attention and test for resource discovery and engineering risk assessment.
The Remnants of Geological Microbial Activity in the Gas-Bearing Layers
To sum up, the presence of shallow gas in the gas-bearing layers is ascribed to several factors, i.e., sediment type, environmental substrates and associated microbial organisms. The microbial organism played a key role to convert the environmental substrates to shallow gas via microbial metabolism, while the sediment characteristics could shape the environmental factors in the formation, thus affecting microbial communities and shallow gas production. Methane and ammonium could be produced during the microbial degradation of OMs, where methane could be further consumed or transferred to other strata; while the ammonium was more likely to be stable and stored in situ in this environment. It should be noted that the iron- or manganese-dependent AOM were reported to widely distribute in the sediment (Beal et al., 2009; Sivan et al., 2011; Bar-Or et al., 2017), which could be a potential methane sink to elevate the methane consumption rate in the in situ strata of methane production. More research is proposed to carry out to verify their effects on the correlations of methane with other factors. Consequently, the correlation of methanogen, methane with ammonium were stronger than that between methanogen and methane, and no close relationship was found between microbial community, methane and existing OMs (Figure 9).
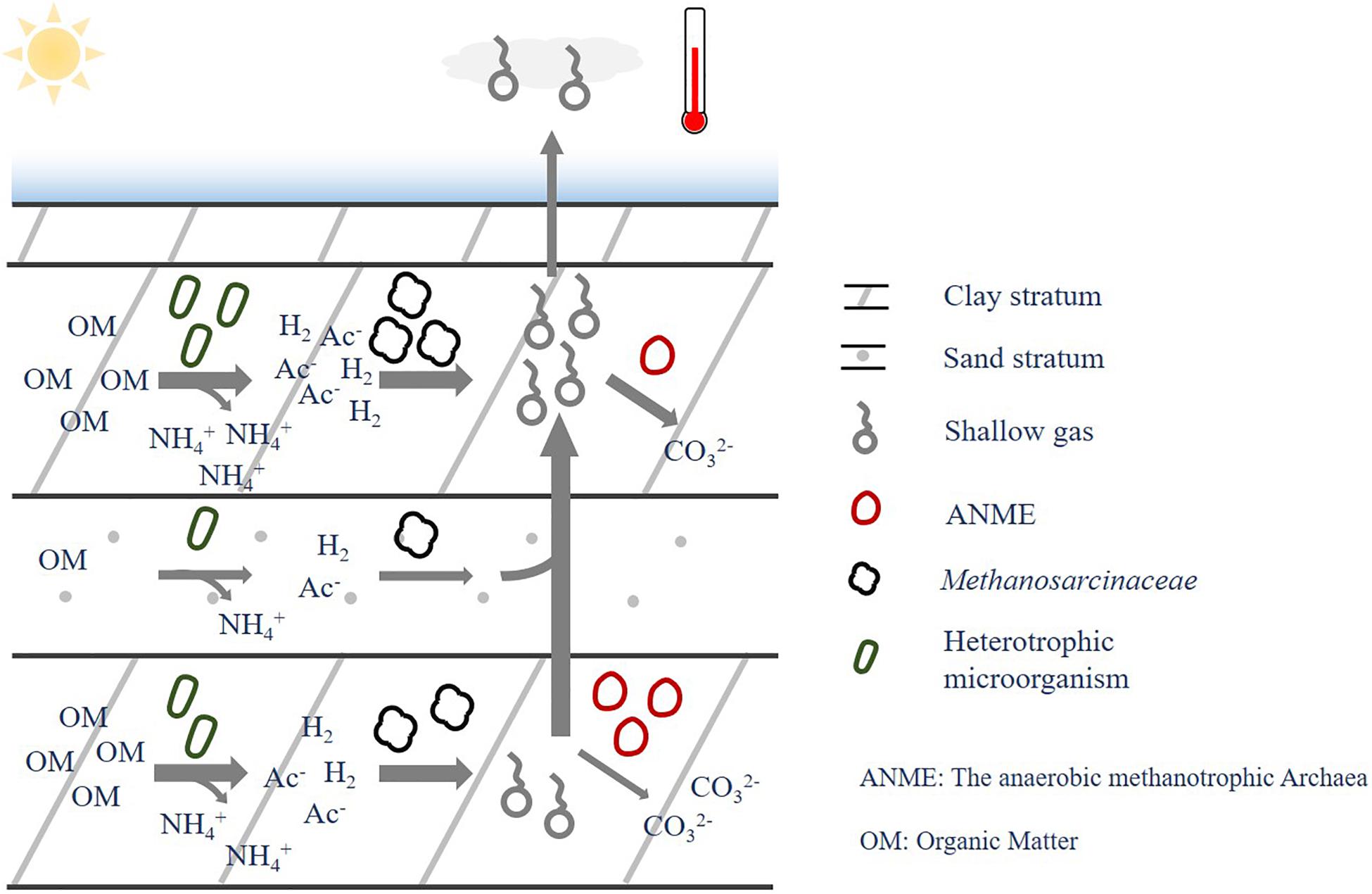
Figure 9. Relationship between sediment type, environmental factors, microbial communities and biogas.
Conclusion
In Hangzhou Bay, it was found that the presence of shallow gas had a good correlation with the distribution of archaea while a weak correlation with bacteria. The dominant archaeal microorganisms were Bathyarchaeota, Thaumarchaeota, and Euryarchaeota. The dominant bacteria were Proteobacteria, Bacteroidetes, Firmicutes, Chloroflexi, and Actinobacteria, and the dominant genera, such as Pseudomonas, were mostly heterotrophic. The gas-bearing layers were revealed with lower species richness, evenness and diversity of archaeal community than those in gas-free layers, while the proportion of Methanosacinaceae and ANME-1a in Euryarchaeota in gas-bearing layers was higher than that in gas-free layers. Ammonium was supposed to be a vital environmental substrate to indicate the characteristics of the microbial community in the formation, and a higher content of ammonium suggested a lower α-diversity of the microbial community and a higher proportion of methanogenic microorganisms. The sediment type could affect the distribution of shallow gas through shaping environmental substrates and the microbial communities. The clay strata were illustrated to have a higher abundance of archaea and be easier for accumulation of shallow gas than sand strata.
Data Availability Statement
The datasets generated for this study can be found in the NCBI, PRJNA541126, PRJNA542038.
Author Contributions
TY and MZ: drafting the work. DK and SZ: acquisition and analysis of data for the work. AD, QL, and DX: revising the manuscript. YH and LW: interpretation of data for the work. PZ: final approval of the version.
Funding
This research was supported by the Key Research and Development Program of Zhejiang Province (2018C03031).
Conflict of Interest
The authors declare that the research was conducted in the absence of any commercial or financial relationships that could be construed as a potential conflict of interest.
Acknowledgments
Jinghui Hu was thanked for providing help on drawing the geographic map. Zhejiang Institute of Hydrogeology and Engineering Geology, Zhejiang Institute of Geology Survey were also thanked for their effort of analyzing stratum lithology and determining methane content in the cores.
Supplementary Material
The Supplementary Material for this article can be found online at: https://www.frontiersin.org/articles/10.3389/fmicb.2019.02421/full#supplementary-material
Footnotes
References
APHA, (2017). Standard Methods for the Examination of Water and Wastewater: Washington, DC: American Public Health Association.
Bar-Or, I., Elvert, M., Eckert, W., Kushmaro, A., Vigderovich, H., Zhu, Q., et al. (2017). Iron-coupled anaerobic oxidation of methane performed by a mixed bacterial-archaeal community based on poorly reactive minerals. Environ. Sci. Technol. 51, 12293–12301. doi: 10.1021/acs.est.7b03126
Beal, E. J., House, C. H., and Orphan, V. J. (2009). Manganese-and iron-dependent marine methane oxidation. Science 325, 184–187. doi: 10.1126/science.1169984
Biddle, J. F., Lipp, J. S., Lever, M. A., Lloyd, K. G., Sorensen, K. B., Anderson, R., et al. (2006). Heterotrophic archaea dominate sedimentary subsurface ecosystems off peru. Proc. Natl. Acad. Sci. U.S.A. 103, 3846–3851. doi: 10.1073/pnas.0600035103
Burdige, D. J. (2005). Burial of terrestrial organic matter in marine sediments: a re-assessment. Glob. Biogeochem. Cycles 19:7.
Davis, A. M. (1992). Shallow gas: an overview. Cont. Shelf Res. 12, 1077–1079. doi: 10.1016/0278-4343(92)90069-v
Dudley, D. R., and George, E. C. (1981). Generation, accumulation, and resource potential of biogenic gas. AAPG Bull. 65, 5–25.
Evans, P. N., Parks, D. H., Chadwick, G. L., Robbins, S. J., Orphan, V. J., Golding, S. D., et al. (2015). Methane metabolism in the archaeal phylum Bathyarchaeota revealed by genome-centric metagenomics. Science 350, 434–438. doi: 10.1126/science.aac7745
Flury, S., Røy, H., Dale, A. W., Fossing, H., Tóth, Z., Spiess, V., et al. (2016). Controls on subsurface methane fluxes and shallow gas formation in baltic sea sediment (aarhus bay, denmark). Geochim. Cosmochim. Ac. 188, 297–309. doi: 10.1016/j.gca.2016.05.037
Gentz, T., Damm, E., Schneider Von Deimling, J., Mau, S., McGinnis, D. F., and Schlüter, M. (2014). A water column study of methane around gas flares located at the west spitsbergen continental margin. Cont. Shelf. Res. 72, 107–118. doi: 10.1016/j.csr.2013.07.013
Hinrichs, K. U., Hayes, J. M., Sylva, S. P., Brewer, P. G., and DeLong, E. F. (1999). Methane-consuming archaebacteria in marine sediments. Nature 398, 802–805. doi: 10.1038/19751
Holler, T., Widdel, F., Knittel, K., Amann, R., Kellermann, M. Y., Hinrichs, K. U., et al. (2011). Thermophilic anaerobic oxidation of methane by marine microbial consortia. ISME J. 5, 1946–1956. doi: 10.1038/ismej.2011.77
Hu, B. L., Shen, L. D., Lian, X., Zhu, Q., Liu, S., Huang, Q., et al. (2014). Evidence for nitrite-dependent anaerobic methane oxidation as a previously overlooked microbial methane sink in wetlands. Proc. Natl. Acad. Sci. U.S.A. 111, 4495–4500. doi: 10.1073/pnas.1318393111
Inagaki, F., Suzuki, M., Takai, K., Oida, H., Sakamoto, T., Aoki, K., et al. (2003). Microbial communities associated with geological horizons in coastal subseafloor sediments from the Sea of Okhotsk. Appl. Environ. Microbiol. 12, 7224–7235. doi: 10.1128/aem.69.12.7224-7235.2003
Johnson, K. M., Hughes, J. E., Donaghay, P. L., and Sieburth, J. M. (1990). Bottle-callbration static head space method for the determination of methane dissolved in seawater. Anal. Chem. 62, 2408–2412. doi: 10.1021/ac00220a030
Kessler, A. J., Chen, Y. J., Waite, D. W., Hutchinson, T., Koh, S., Popa, M. E., et al. (2019). Bacterial fermentation and respiration processes are uncoupled in anoxic permeable sediments. Nat. Microbiol. 6, 1014–1023. doi: 10.1038/s41564-019-0391-z
Kimes, N. E., Callaghan, A. V., Suflita, J. M., and Morris, P. J. (2014). Microbial transformation of the deepwater horizon oil spill–past, present, and future perspectives. Front. Microbiol. 5, 1–11. doi: 10.3389/fmicb.2014.00603
Kleindienst, S., Seidel, M., Ziervogel, K., Grim, S., Loftis, K., Harrison, S., et al. (2015). Chemical dispersants can suppress the activity of natural oil-degrading microorganisms. Proc. Natl. Acad. Sci. U.S.A. 112, 14900–14905. doi: 10.1073/pnas.1507380112
Lang, K., Schuldes, J., Klingl, A., Poehlein, A., Daniel, R., and Brune, A. (2015). New mode of energy metabolism in the seventh order of methanogens as revealed by comparative genome analysis of “candidatus methanoplasma termitum”. Appl. Environ. Microbiol. 81, 1338–1352. doi: 10.1128/aem.03389-14
Li, Q. Q., Wang, F. P., Chen, Z. W., Yin, X. J., and Xiao, X. (2012). Stratified active archaeal communities in the sediments of jiulong river estuary, china. Front. Microbiol. 3, 1–14. doi: 10.3389/fmicb.2012.00311
Li, Y., Zhang, X. D., Xu, H. P., Mu, H., Hua, D. L., and Jin, F. Q. et al. (2019). Acidogenic properties of carbohydrate-rich wasted potato and microbial community analysis: effect of pH. J. Biosci. Bioeng. 1, 50–55. doi: 10.1016/j.jbiosc.2018.12.009
Lin, C., Zhuo, H., and Gao, S. (2005). Sedimentary facies and evolution in the qiantang river incised valley, eastern china. Mar. Geol. 219, 235–259. doi: 10.1016/j.margeo.2005.06.009
Liu, J., Liu, X., Wang, M., Qiao, Y., Zheng, Y., and Zhang, X. (2015). Bacterial and archaeal communities in sediments of the north chinese marginal seas. Microb. Ecol. 70, 105–117. doi: 10.1007/s00248-014-0553-8
Lloyd, K. G., Alperin, M. J., and Teske, A. (2011). Environmental evidence for net methane production and oxidation in putative anaerobic methanotrophic (ANME) archaea. Environ. Microbiol. 13, 2548–2564. doi: 10.1111/j.1462-2920.2011.02526.x
Lloyd, K. G., Schreiber, L., Petersen, D. G., Kjeldsen, K. U., Lever, M. A., Steen, A. D., et al. (2013). Predominant archaea in marine sediments degrade detrital proteins. Nature 496, 215–218. doi: 10.1038/nature12033
Meador, T. B., Bowles, M., Lazar, C. S., Zhu, C., Teske, A., and Hinrichs, K. U. (2015). The archaeal lipidome in estuarine sediment dominated by members of the miscellaneous crenarchaeotal group. Environ. Microbiol. 17, 2441–2458. doi: 10.1111/1462-2920.12716
Meng, J., Xu, J., Qin, D., He, Y., Xiao, X., and Wang, F. (2014). Genetic and functional properties of uncultivated MCG archaea assessed by metagenome and gene expression analyses. ISME J. 8, 650–659. doi: 10.1038/ismej.2013.174
Nunoura, T., Takaki, Y., Shimamura, S., Kakuta, J., Kazama, H., Hirai, M., et al. (2016). Variance and potential niche separation of microbial communities in subseafloor sediments off shimokita peninsula, Japan. Environ. Microbiol. 6, 1889–1906. doi: 10.1111/1462-2920.13096
Rajan, A., Bünz, S., Mienert, J., and Smith, A. J. (2013). Gas hydrate systems in petroleum provinces of the SW-barents sea. Mar. Pet. Geol. 46, 92–106. doi: 10.1016/j.marpetgeo.2013.06.009
Roy, S., Senger, K., Hovland, M., Römer, M., and Braathen, A. (2019). Geological controls on shallow gas distribution and seafloor seepage in an arctic fjord of spitsbergen, norway. Mar. Pet. Geol. 107, 237–254. doi: 10.1016/j.marpetgeo.2019.05.021
Santoro, A. E., Dupont, C. L., Richter, R. A., Craig, M. T., Carini, P., McIlvin, M. R., et al. (2015). Genomic and proteomic characterization of “Candidatus Nitrosopelagicus brevis”: an ammonia-oxidizing archaeon from the open ocean. Proc. Natl. Acad. Sci. U.S.A. 112, 1173–1178. doi: 10.1073/pnas.1416223112
Shen, L., Liu, S., Lou, L., Liu, W., Xu, X., Zheng, P., et al. (2013). Broad distribution of diverse anaerobic ammonium-oxidizing bacteria in chinese agricultural soils. Appl. Environ. Microbiol. 79, 6167–6172. doi: 10.1128/AEM.00884-13
Shindell, D. T., Faluvegi, G., Koch, D. M., Schmidt, G. A., Unger, N., and Bauer, S. E. (2009). Improved attribution of climate forcing to emissions. Scienc. 326, 716–718. doi: 10.1126/science.1174760
Sivan, O., Adler, M., Pearson, A., Gelman, F., Bar-Or, I., John, G. S., et al. (2011). Geochemical evidence for iron-mediated anaerobic oxidation of methane. Limnol. Oceanogr. 56, 1536–1544. doi: 10.1021/es503663z
Sorensen, K. B., and Teske, A. (2006). Stratified communities of active archaea in deep marine subsurface sediments. Appl. Environ. Microbiol. 72, 4596–4603. doi: 10.1128/aem.00562-06
Tong, C., Cadillo-Quiroz, H., Zeng, Z. H., She, C. X., Yang, P., and Huang, J. F. (2017). Changes of community structure and abundance of methanogens in soils along a freshwater–brackish water gradient in subtropical estuarine marshes. Geoderma 299, 101–110. doi: 10.1016/j.geoderma.2017.03.026
Vielstädte, L., Karstens, J., Haeckel, M., Schmidt, M., Linke, P., Reimann, S., et al. (2015). Quantification of methane emissions at abandoned gas wells in the central north Sea. Mar. Pet. Geol. 68, 848–860. doi: 10.1016/j.marpetgeo.2015.07.030
Wakeham, S. G., Lee, C., Hedges, J. I., Hernes, P. J., and Peterson, M. J. (1997). Molecular indicators of diagenetic status in marine organic matter. Geochim. Cosmochim. Ac. 61, 5363–5369. doi: 10.1016/s0016-7037(97)00312-8
Wang, F. P., Zhang, Y., Chen, Y., He, Y., Qi, J., Hinrichs, K. U., et al. (2014). Methanotrophic archaea possessing diverging methane-oxidizing and electron-transporting pathways. ISME J. 8, 1069–1078. doi: 10.1038/ismej.2013.212
Webster, G., O’Sullivan, L. A., Meng, Y., Williams, A. S., Sass, A. M., Watkins, A. J., et al. (2014). Archaeal community diversity and abundance changes along a natural salinity gradient in estuarine sediments. FEMS Microbiol. 91, 1–18. doi: 10.1093/femsec/fiu025
Wegener, G., Krukenberg, V., Riedel, D., Tegetmeyer, H. E., and Boetius, A. (2015). Intercellular wiring enables electron transfer between methanotrophic archaea and bacteria. Nature 526, 587–590. doi: 10.1038/nature15733
Xing, J., and Spiess, V. (2015). Shallow gas transport and reservoirs in the vicinity of deeply rooted mud volcanoes in the central black Sea. Mar. Geol. 369, 67–78. doi: 10.1016/j.margeo.2015.08.005
Xu, F. L. (2016). The biogeochemistry of sedimentary organic matter in marginal seas. Master. thesis, Zhejiang
Xu, Y., Wu, H., Shen, J. S., and Zhang, N. (2017). Risk and impacts on the environment of free-phase biogas in quaternary deposits along the coastal region of shanghai. Ocean Eng. 137, 129–137. doi: 10.1016/j.oceaneng.2017.03.051
Yin, X., Wu, W., Maeke, M., Richter-Heitmann, T., Kulkarni, A. C., Oni, O. E., et al. (2019). CO2 conversion to methane and biomass in obligate methylotrophic methanogens in marine sediments. ISME J. 13, 2107–2119. doi: 10.1038/s41396-019-0425-9
Yu, T. T., Wu, W. C., Liang, W. Y., Lever, M. A., Hinrichs, K., and Wang, F. P. (2018). Growth of sedimentary Bathyarchaeota on lignin as an energy source. Proc. Natl. Acad. Sci. U.S.A. 115, 6022–6027. doi: 10.1073/pnas.1718854115
Zhang, J., Yang, Y., Zhao, L., Li, Y., Xie, S., and Liu, Y. (2015). Distribution of sediment bacterial and archaeal communities in plateau freshwater lakes. Appl. Microbiol. Biotechnol. 99, 3291–3302. doi: 10.1007/s00253-014-6262-x
Zhang, M., Gu, J., and Liu, Y. (2019). Engineering feasibility, economic viability and environmental sustainability of energy recovery from nitrous oxide in biological wastewater treatment plant. Bioresour. Technol. 282, 514–519. doi: 10.1016/j.biortech.2019.03.040
Zhang, X., Lin, C., Li, Y., Qu, C., and Wang, S. (2013). Sealing mechanism for cap beds of shallow-biogenic gas reservoirs in the Qiantang River incised valley, china. Cont. Shelf. Res. 69, 155–167. doi: 10.1016/j.csr.2013.09.006
Zheng, Y., Hou, L., Newell, S., Liu, M., Zhou, J., Zhao, H., et al. (2013). Community dynamics and activity of ammonia-oxidizing prokaryotes in intertidal sediments of the yangtze estuary. Appl. Environ. Microbiol. 80, 408–419. doi: 10.1128/AEM.03035-13
Keywords: shallow gas, gas bearing formation, microbial community, environmental substrates, sediment type
Citation: Yu T, Zhang M, Kang D, Zhao S, Ding A, Lin Q, Xu D, Hong Y, Wang L and Zheng P (2019) Characteristics of Microbial Communities and Their Correlation With Environmental Substrates and Sediment Type in the Gas-Bearing Formation of Hangzhou Bay, China. Front. Microbiol. 10:2421. doi: 10.3389/fmicb.2019.02421
Received: 20 May 2019; Accepted: 07 October 2019;
Published: 23 October 2019.
Edited by:
Mustafa Yucel, Middle East Technical University, TurkeyReviewed by:
Amy Michele Grunden, North Carolina State University, United StatesJeffrey M. Dick, Central South University, China
Copyright © 2019 Yu, Zhang, Kang, Zhao, Ding, Lin, Xu, Hong, Wang and Zheng. This is an open-access article distributed under the terms of the Creative Commons Attribution License (CC BY). The use, distribution or reproduction in other forums is permitted, provided the original author(s) and the copyright owner(s) are credited and that the original publication in this journal is cited, in accordance with accepted academic practice. No use, distribution or reproduction is permitted which does not comply with these terms.
*Correspondence: Ping Zheng, cHpoZW5nQHpqdS5lZHUuY24=
†These authors have contributed equally to this work