- 1Jiangsu Key Laboratory of Anaerobic Biotechnology, School of Environmental and Civil Engineering, Jiangnan University, Wuxi, China
- 2Department of Biogeochemistry, Max Planck Institute for Terrestrial Microbiology, Marburg, Germany
- 3Jiangsu Collaborative Innovation Center of Technology and Material of Water Treatment, Suzhou, China
Anaerobic digestion is a widely applied technology for sewage sludge treatment. Hydrogen and CO2 are important degradation products, which serve as substrates for both hydrogenotrophic methanogenesis and chemolithotrophic acetogenesis. In order to understand the competition between these processes for H2/CO2, sludge samples were incubated under H2/CO2 headspace at different temperatures, and analyzed with respect to turnover of H2, CO2, CH4 and acetate including their δ13C values. At 15°C, 13C-depleted acetate (δ13C of −41 to −43‰) and transient acetate accumulation were observed under H2/CO2, and CH4 accumulated with δ13C values increasing from −53 to −33‰. The copy numbers of the fhs gene, which is characteristic for acetogenic bacteria, were at 15°C one order of magnitude higher in the H2/CO2 incubations than the N2 control. At 30°C, however, acetate did not accumulate in the H2/CO2 incubation and the δ13C of CH4 was very low (−100 to −77‰). At 50°C, isotopically enriched acetate was transiently formed and subsequently consumed followed by the production of 13C-depleted CH4. Collectively, the results indicate a high contribution of chemolithotrophic acetogenesis to H2/CO2 utilization at 15°C and 50°C, while H2/CO2 was mainly consumed by hydrogenotrophic methanogenesis at 30°C. Fermentative production and methanogenic consumption of acetate were active at 50°C.
Introduction
Anaerobic digestion has been widely used for stabilization and energy recovery of sewage sludge (Kelessidis and Stasinakis, 2012). Anaerobic digestion of organic matter is achieved in four steps: hydrolysis, fermentation, acetogenesis, and methanogenesis (Adekunle and Okolie, 2015). Acetate and CH4 are the respective products of chemolithotrophic acetogensis (4 H2 + 2 CO2 → CH3COOH + 2 H2O) and hydrogenotrophic methanogenesis (4 H2 + CO2 → CH4 + 2 H2O). Chemolithotrophic acetogenic bacteria normally compete directly with hydrogenotrophic methanogens for H2/CO2 as substrates (Lopes et al., 2015; Liu et al., 2016). Meanwhile, the emission of CO2 and CH4 during anaerobic digestion of sewage sludge has received attention because of the greenhouse effect (Niu et al., 2013). The generation of acetate instead of CH4 from sewage sludge is a promising technology for waste recycling and reduction of greenhouse gas emission (Agler et al., 2011).
Temperature is one of the key variables in anaerobic sludge digestion and has an important effect on H2/CO2 utilization (Conrad and Wetter, 1990; Kotsyurbenko et al., 2001; Shanmugam et al., 2014). Studies on rice field soils indicate that acetogenic bacteria can outcompete methanogens for H2 at low temperature (Conrad et al., 1989; Liu and Conrad, 2011). Thermophilic anaerobic digestion processes offer kinetic advantages when compared with mesophilic conditions. Compared to 35°C, rates of methanogenesis increase at 55°C, but the methanogenic pathway also changes by replacing acetoclastic methanogesis with syntrophic acetate oxidation coupled to hydrogenotrophic methanogenesis (Zábranská et al., 2000; Hao et al., 2011; Ho et al., 2013). Their respective contribution to the overall anaerobic degradation of organic matter in sewage sludge may be different due to different temperatures. Some studies reported the competition between acetogenic bacteria and methanogens in lake sediments and rice field soils (Chin and Conrad, 2010; Liu and Conrad, 2011; Olivier, 2016), however, the effect of temperature on the contribution of acetogenesis and methanogenesis to chemolithotrophic H2/CO2 utilization in anaerobic digested sludge is not well understood.
However, the differentiation of chemolithotrophic acetogenesis and hydrogenotrophic methanogenesis in H2/CO2 utilization is complex. Acetate is not only produced by chemolithotrophic acetogenesis but also by fermentation and heterotrophic acetogenesis. Methane is the end product of both acetoclastic methanogenesis and hydrogenotrophic methanogenesis. Isotope technique is a reasonable approach, since studies have shown that the stable carbon isotope fractionation of chemolithotrophic acetogenesis (−38 to −68‰) and hydrogenotrophic methanogenesis (−21 to −71‰) is strong (Galand et al., 2010; Blaser et al., 2013; Gehring et al., 2015; Ji et al., 2018), which imprints a signature on the stable carbon isotope composition (13C/12C) of acetate and CH4.
In this study, we aimed to specify the competition between chemolithotrophic acetogenesis and hydrogenotrophic methanogenesis for H2/CO2 in anaerobic digested sludge. Incubation under H2/CO2 at different temperatures served for determining the potential of the chemolithotrophic acetogenesis and hydrogenotrophic methanogenesis. Incubation in the presence of bromoethanesulfonate (BES) was used to inhibit methanogenesis.
Materials and Methods
Sewage Sludge Incubation
Sewage sludge was obtained from secondary settling tank sludge of Wuxi Shuofang sewage treatment plant. The physicochemical characteristics of sewage sludge were: pH (7.65); dry weight (DW; 14.3%); volatile substances (72g/L); water content (85.6%); total N (15.8 mg g–1 DW); and total phosphorus (17.0 mg g–1 DW). Sludge slurries were prepared in 26-mL pressure tubes by mixing 3.9 g sewage sludge and 6.1 mL of anoxic sterile water. The tubes were closed with black rubber stoppers, flushed with N2, pressurized to 0.5 bar overpressure, and then pre-incubated at 25°C for about 5 days to deplete alternative electron acceptors and initiate methanogenesis. After pre-incubation, three treatments were all incubated under 15°C, 30°C, 50°C: (1) control, the sludge slurry was incubated under N2 headspace; (2) H2/CO2 treatment, the sludge slurry was incubated under H2/CO2 (80/20, v/v) headspace to stimulate both chemolithotrophic acetogenesis and hydrogenotrophic methanogenesis; and (3) H2/CO2 + BES treatment, the sludge slurry was incubated under H2/CO2 (80/20, v/v) headspace and methanogenesis was inhibited by 100 mM BES. The headspace pressures of the three treatments were all adjusted to 1.5 bar. The tubes with sewage sludge slurry were prepared in numerous parallels (about 108 tubes), of which triplicates were sacrificed for chemical analyses of liquid samples and molecular analyses. Gas samples were taken from 27 tubes during the incubation at few days’ intervals to measure the concentrations of CH4, CO2, H2 and the δ13C values of CH4 and CO2. The other tubes were opened to retrieve liquid samples for analysis of volatile fatty acids (VFAs) concentration and the δ13C of acetate, and were stored frozen at −20°C for later molecular analyses. The δ13C of the organic carbon in the sewage sludge was −29.8‰.
Chemical Analysis
Analytical methods for CH4, CO2, H2 in gas samples and acetate in liquid samples were as described before (Fu et al., 2018). Simply, the partial pressures of CH4 and CO2 were analyzed by gas chromatography (GC). The partial pressures were converted into molar quantities by using the ideal gas volume formula at different temperatures. The small amount of dissolved CH4 was neglected, and the amount of dissolved CO2 was calculated from the Henry constants at different temperatures. The concentrations of bicarbonate were calculated from the CO2 partial pressures and the pH using the equations listed in Stumm and Morgan (1981). The 13C content of CH4 and CO2 was measured using a Finnigan Gas Chromatography Combustion Isotope Ratio Mass Spectrometry System. Concentrations of acetate and other VFAs were analyzed by high-pressure liquid chromatography (HPLC). An HPLC system (Spectra System P1000, Thermo Fisher Scientific, San Jose, CA, United States; Mistral, Spark, Emmen, Netherlands) equipped with an ion-exclusion column (Aminex HPX-87-H) and a Finnigan LC IsoLink (Thermo Fisher Scientific, Bremen, Germany) was used to measure the δ13C values of acetate.
DNA Extraction and Quantification of Gene Copy
DNA was extracted from the sewage sludge sample using the PowerSoil® DNA Isolation kit. Frozen sewage sludge samples were thawed at 4°C. In order to ensure homogeneity, sludge samples were vortexed prior to DNA extraction. Quality and concentration of the extracted DNA were detected by UV spectrophotometer (NanoDrop ND 2000).
All the oligonucleotide primers were synthesized by Shanghai Bio-Engineering Co., Ltd. (China), and all the qPCR reaction components were purchased from Shanghai Bio-Engineering Co., Ltd. (China). The qPCR was conducted in a Rotor-Gene Q fluorescence quantitative PCR instrument. For all assays, the standard was a sample containing known numbers of DNA copies of the target gene. Standards were continuously diluted and used in each reaction to construct calibration curves. Methanogenic archaea and acetogenic bacteria were quantified by amplification of the mcrA and fhs genes, respectively using primers listed in fhs-f/fhs-r Table 1 (Angel et al., 2011; Xu et al., 2015). The mcrA and fhs gene qPCR conditions included an initial denaturation at 94°C for 4 min, followed 30 cycles at 94°C for 30s at the specific annealing temperature shown in Table 1. In order to know the relative abundance of acetogenic bacteria, we also used the universal primers 519f/907r to quantify the 16S rRNA gene copies of the domain Bacteria (Table 1) (Imachi et al., 2008).
Results
H2/CO2 Utilization at Low Temperature
The time courses of accumulation of CH4, CO2, acetate and H2, as well as the temporal change of δ13C values of CH4, acetate, and CO2 of the treatments control, H2/CO2, and H2/CO2 + BES are shown in Figures 1–3 for the incubation temperatures 15, 30, and 50°C, respectively. At 15°C CH4 concentrations increased with time in the control and H2/CO2 treatments but not in the presence of BES, which inhibited CH4 production completely (Figure 1A). At the same time, CO2 (Figure 1E) and H2 (Figure 1G) concentrations decreased in the H2/CO2 treatments both in the presence and absence of BES. Later on, CO2 slightly increased in the absence of BES presumably because of the conversion of acetate to CO2 and CH4 (Figure 1E). In the N2 incubations H2 transiently accumulated to 104 μmol/gDW and then decreased to very low concentration at low temperature (Figure 1G).
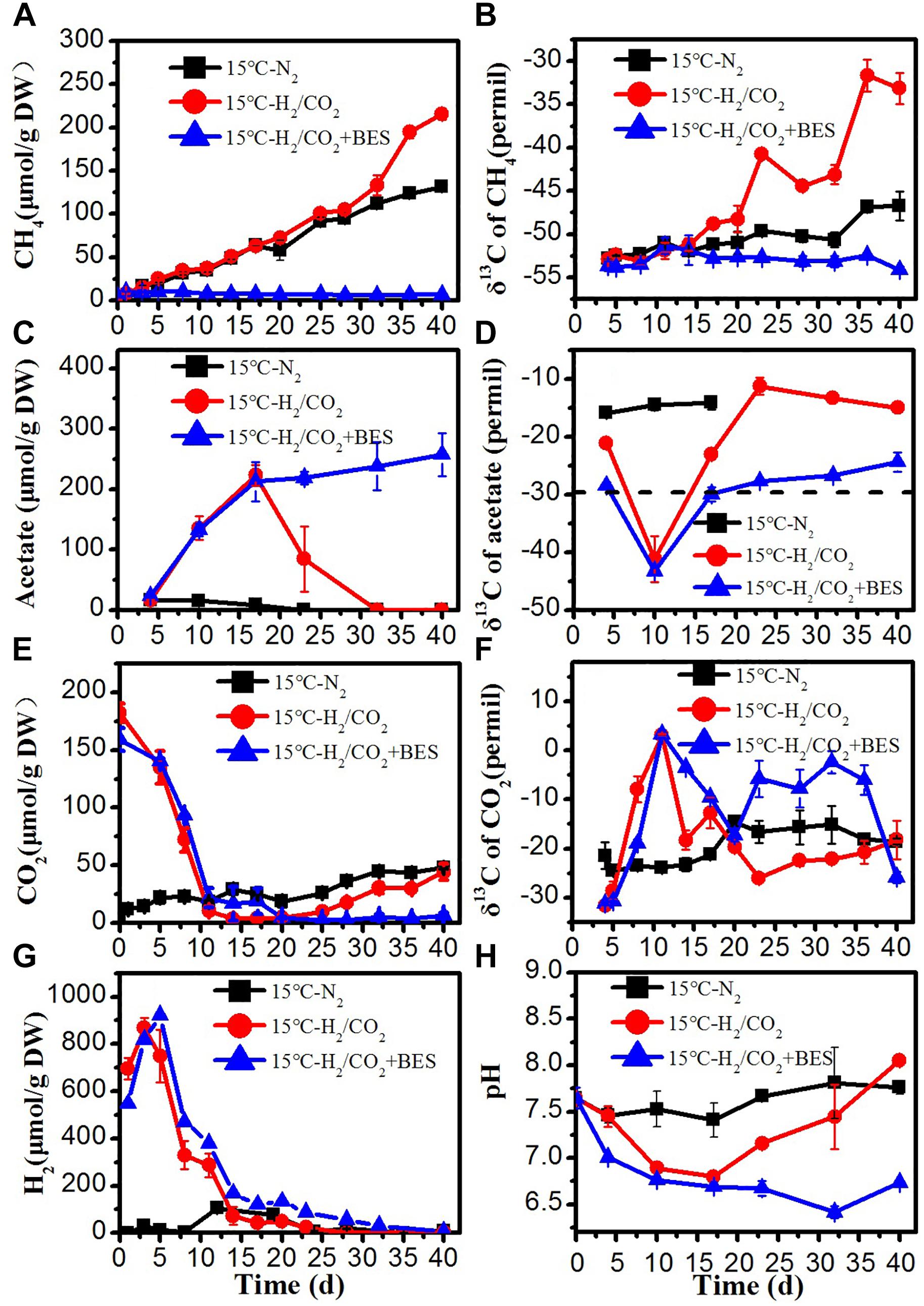
Figure 1. Time course of accumulated (A) CH4, (B) δ13C of CH4, (C) acetate, (D) δ13C of acetate, (E) CO2, (F) δ13C of CO2, (G) H2 concentration, and (H) pH during the treatment of sewage sludge at 15°C, BES as an inhibiter of methanogenesis. The δ13C of the sludge organic matter (−29.8‰) was represented by dotted line. Mean ± SD, n = 3.
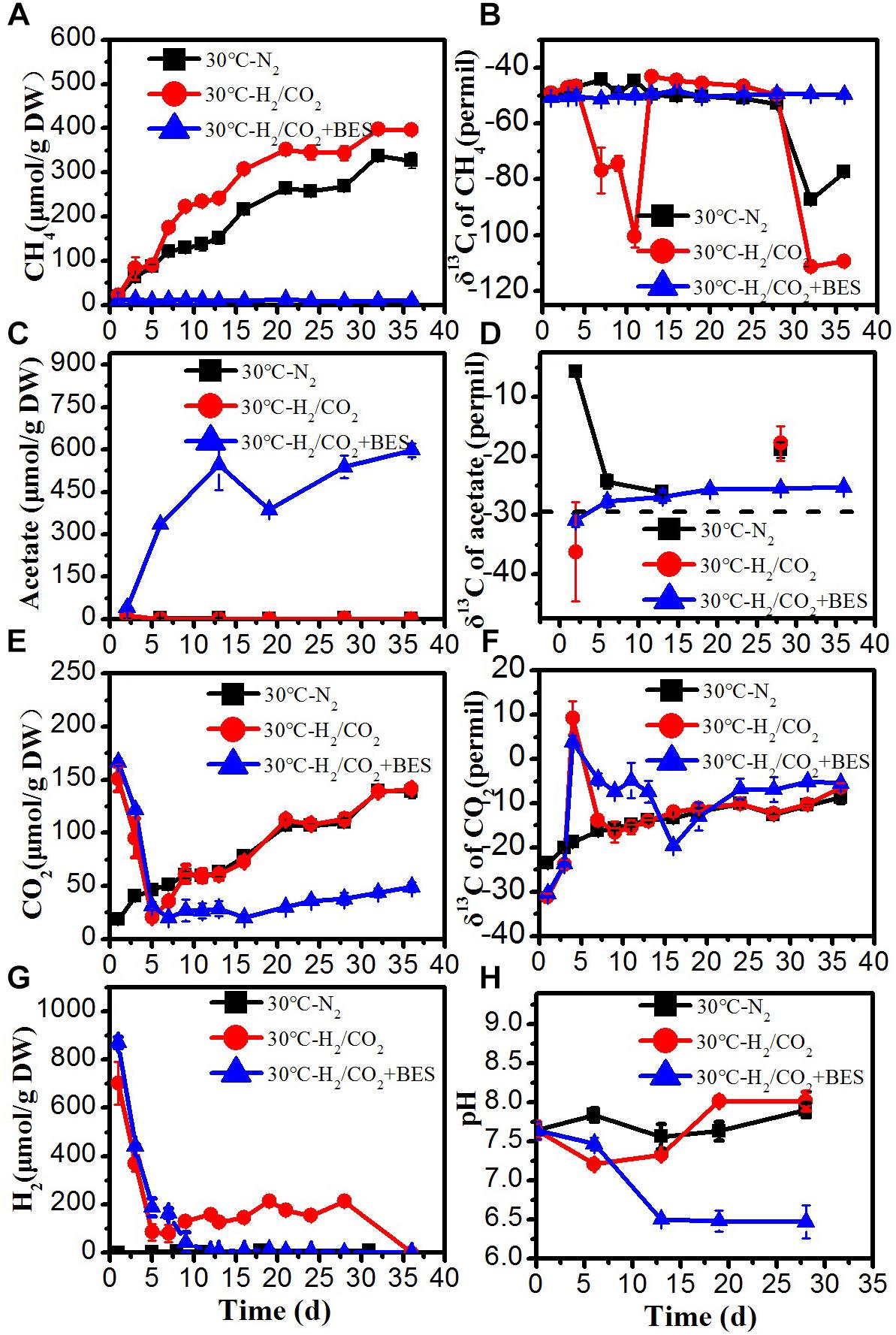
Figure 2. Time course of accumulated (A) CH4, (B) δ13C of CH4, (C) acetate, (D) δ13C of acetate, (E) CO2, (F) δ13C of CO2, (G) H2 concentration, and (H) pH during the treatment of sewage sludge at 30°C, BES as an inhibiter of methanogenesis. The δ13C of the sludge organic matter (−29.8‰) was represented by dotted line. Mean ± SD, n = 3.
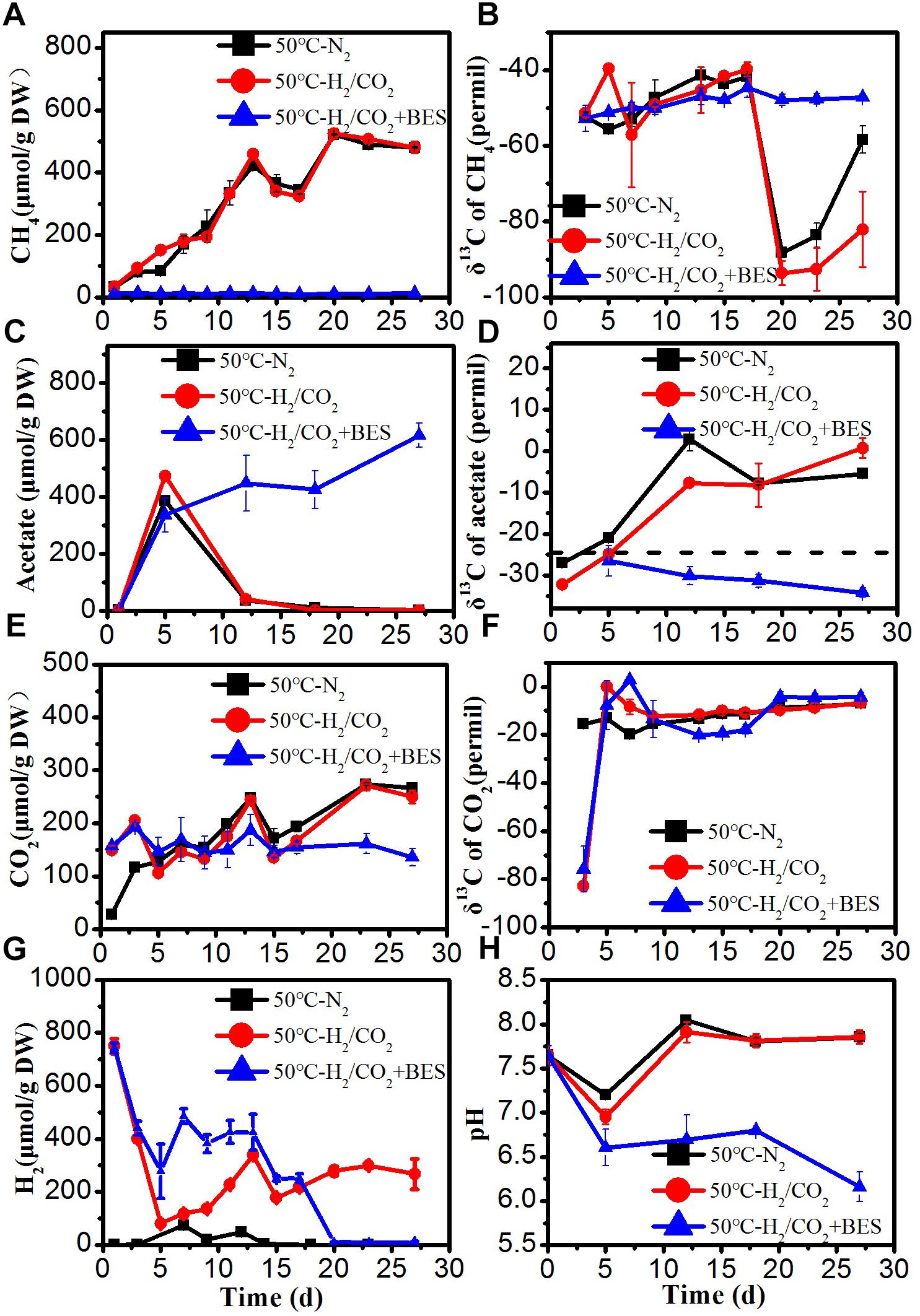
Figure 3. Time course of accumulated (A) CH4, (B) δ13C of CH4, (C) acetate, (D) δ13C of acetate, (E) CO2, (F) δ13C of CO2, (G) H2 concentration, and (H) pH during the treatment of sewage sludge at 50°C, BES as an inhibiter of methanogenesis. The δ13C of the sludge organic matter (−29.8‰) was represented by dotted line. Mean ± SD, n = 3.
The two major products of consumption of H2 and CO2 were CH4 (Figure 1A) and acetate (Figure 1B). In the H2/CO2 incubations, acetate concentrations accumulated to a maximum on day 17, and then gradually decreased to nearly zero with time (Figure 1B). Acetate was then presumably converted to CH4, which was inhibited in the BES-treated samples (Figure 1A). There was almost no acetate accumulation in the N2 controls (Figure 1C). Formate, propionate, and butyrate concentrations were always lower than 14, 21, and 23 μmol/g DW, respectively (Supplementary Figure S1).
The amounts of consumed H2 and produced acetate and CH4 are summarized in Table 2. With exogenous H2/CO2 and the methanogenic inhibitor BES, about 800–916 μmol/g DW of H2 were consumed and about 212–258 μmol/g DW acetate were produced, indicating a stoichiometry of 4 to 1 as expected for chemolithotrophic acetogenesis. Without BES, the transiently accumulated acetate was finally converted to less than 215 μmol/g DW CH4, taking into account that CH4 was also produced from the sewage sludge without exogenous H2/CO2.
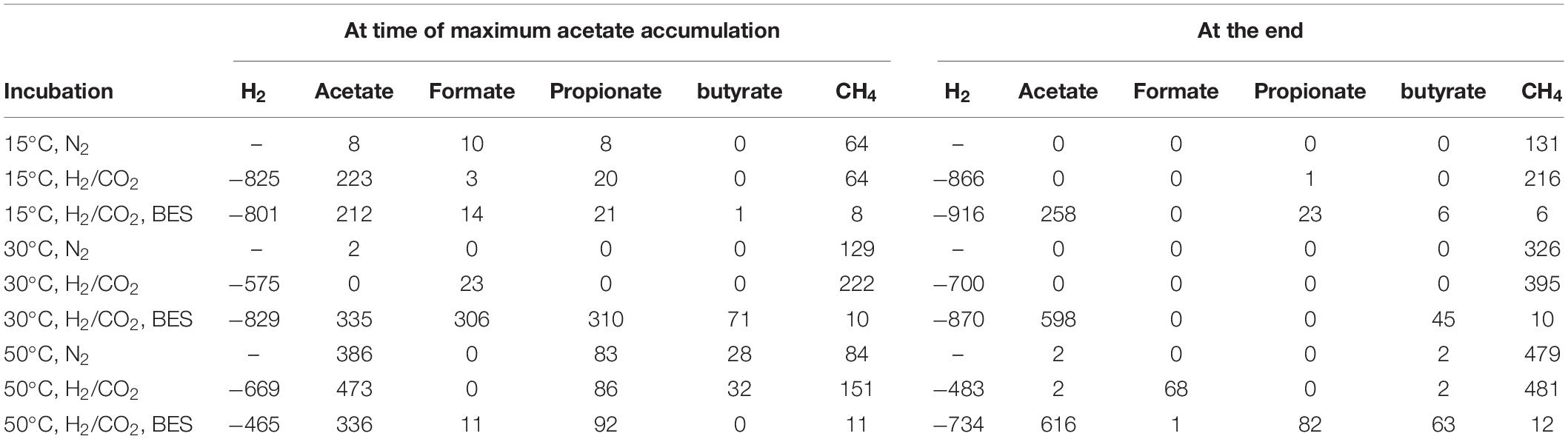
Table 2. Accumulated (positive μmol/g DW) or consumed (negative μmol/g DW) metabolites in the different incubations.
The δ13C values of acetate under H2/CO2 treatments showed transiently very low values (<−40‰) on day 10 (Figure 1D). Based on the isotopic signature of acetogenic pure cultures, this 13C-depleted acetate was apparently produced from chemolithotrophic acetogenesis (Blaser et al., 2013). These values were much lower than the δ13C of sludge organic matter (−29.8‰), indicating that acetate was produced by chemolithotrophic acetogenesis. Later on, δ13C values of acetate increased to values >−30‰, especially in the absence of BES, indicating conversion by acetoclastic methanogenesis (Figure 1D). Only little CH4 (8–18 μmol/g DW) with a δ13C of about −54‰ was observed in in the presence of BES due to the inhibition of methanogenesis. In the absence of BES, the δ13C values of CH4 under H2/CO2 increased to about −33‰, but in the N2 controls only to about − 47‰ (Figure 1B). In the N2 control, the δ13C values of CO2 accordingly increased from initially −31‰ to about −18.6‰ (Figure 1F). However, in the H2/CO2 treatments, the δ13C values of CO2 initially increased to about 0‰, irrespectively of the presence of BES. This increase is consistent with the conversion of CO2 to either CH4 or acetate. Later on, the δ13C values of CO2 decreased again, especially in the absence of BES, presumably due to methanogenic consumption of acetate (Figure 1F).
H2/CO2 Utilization at Mesophilic Temperature
At 30°C, the time courses of accumulation of CH4, CO2, acetate and H2 are shown in Figure 2. The time courses were similar as at 15°C with the following remarkable exceptions: Methane production rates were larger. Acetate only accumulated in the BES treatment, when CH4 production was inhibited by BES (Figure 2B). Similarly, formate, propionate and butyrate accumulated in the H2/CO2 incubations transiently but only in the presence of BES (Supplementary Figure S2). These observations indicate that any produced VFA was instantaneously consumed and did not accumulate when acetoclastic methanogenesis was operating in the absence of BES. In the N2 controls only traces of H2 (<7 μmol/g DW) were detected (Figure 2G). The concentrations of H2 and CO2 both decreased initially in the H2/CO2 treatments. Although H2 and CO2 later on gradually increased again but slightly increased H2 was completely consumed after Day 28 in the absence of BES (Figure 2E). Initially, H2 and CO2 was consumed by hydrogenotrophic methanogenesis to produce CH4, as indicated by the very low δ13C value of CH4 (−100.6‰) (Figure 2B). At the end of the incubation, δ13C of CH4 again decreased to −111.3‰ and δ13C of CO2 gradually and slightly increased indicating dominance of hydrogenotrophic methanogenesis. The slight increase of H2 and CO2 concentration in the middle of incubation could be due to the fermentation of organic matter in the sludge, which is consistent with a similar trend and similar values in the N2 controls and the decrease of δ13C of CO2 and the absence of acetate accumulation (Figures 2C,E–G).
The amounts of acetate production (about 500–550 μmol/g DW) were larger than expected from the amounts of H2 consumed (about 870 μmol/g DW) and the assumed stoichiometry of 1:4 (Table 2). Accumulation of CH4 in the presence of exogenous H2/CO2 was not much larger (396 μmol/g DW) than in the absence (326 μmol/g DW) (Table 2). Therefore, it is likely that both CH4 and acetate were to a large extent produced from the sewage sludge rather than from the exogenous H2/CO2, which would imply a stoichiometry of 4:1 as characteristic for hydrogenotrophic methanogenesis.
H2/CO2 Utilization at Thermophilic Temperature
At 50°C, the rates of CH4 production were higher than at 30 and 15°C (Figure 3). The added H2 was only slowly consumed when BES was present. The concentrations of H2 decreased initially in the H2/CO2 treatments, but H2 later on gradually increased again to the final concentrations of about 280 μmol/g DW, which were higher than at the other temperatures (Figure 3G). The added CO2 was also hardly consumed at 50°C, and in the N2 control CO2 eventually increased to a similar concentration (Figure 3E). The detected H2 concentrations in the N2 controls were generally lower than 73 μmol/gDW (Figure 3G). Acetate, however, was transiently produced in all the treatments including the N2 control, but was later on consumed again except when CH4 production was inhibited by BES (Figure 3C). Accumulated formate of 173–206 μmol/g was finally consumed to very low concentration except in the H2/CO2 treatments where finally about 68 μmol/g DW formate remained (Supplementary Figure S3). In the N2 controls and the H2/CO2 treatments, propionate and butyrate were transiently accumulated to about 87 and 32 μmol/g DW and subsequently consumed (Supplementary Figure S3). Propionate and butyrate concentrations reached 82 and 63 μmol/g DW in the BES treatments (Supplementary Figure S3). The δ13C of acetate substantially increased to about − 7‰ due to the consumption, except in the presence of BES (Figure 3D). The δ13C of CO2 initially increased and then stayed relatively constant at about −15 to −5‰ (Figure 3F), and that of CH4 was about −50‰, but decreased significantly at the end of incubation, except in the presence of BES (Figure 3B).
The produced amounts of both acetate and CH4 were much larger than the amounts of exogenous H2 consumed, assuming a stoichiometry of 1:4 as characteristic for chemolithotrophic acetogenesis and hydrogenotrophic methanogenesis (Table 2). Consequently, it is likely that most of the acetogenic and methanogenic substrates were produced from the anaerobic sewage sludge.
Quantification of Methanogens and Acetogenic Bacteria
The copy numbers of the mcrA gene, coding for a subunit of the methyl coenzyme M reductase, was measured as equivalent for the number of methanogens in the sewage sludge (Figure 4). In BES treatments mcrA was not quantified. The copy numbers of mcrA gene at 50 and 30°C were one order of magnitude higher than those of 15°C during the whole incubation (Figure 4A). At 15°C, the final copy numbers of mcrA gene under H2/CO2 were one order of magnitude higher than that of the controls, which indicated H2/CO2 stimulated the growth of methanogens (Figure 4). The copy numbers of mcrA gene at 30°C were always one order of magnitude higher than those of the N2 control during the whole incubation. However, the copy numbers of mcrA gene in the H2/CO2 incubation at 50°C were at a similar level than those of the N2 controls (Figure 4).
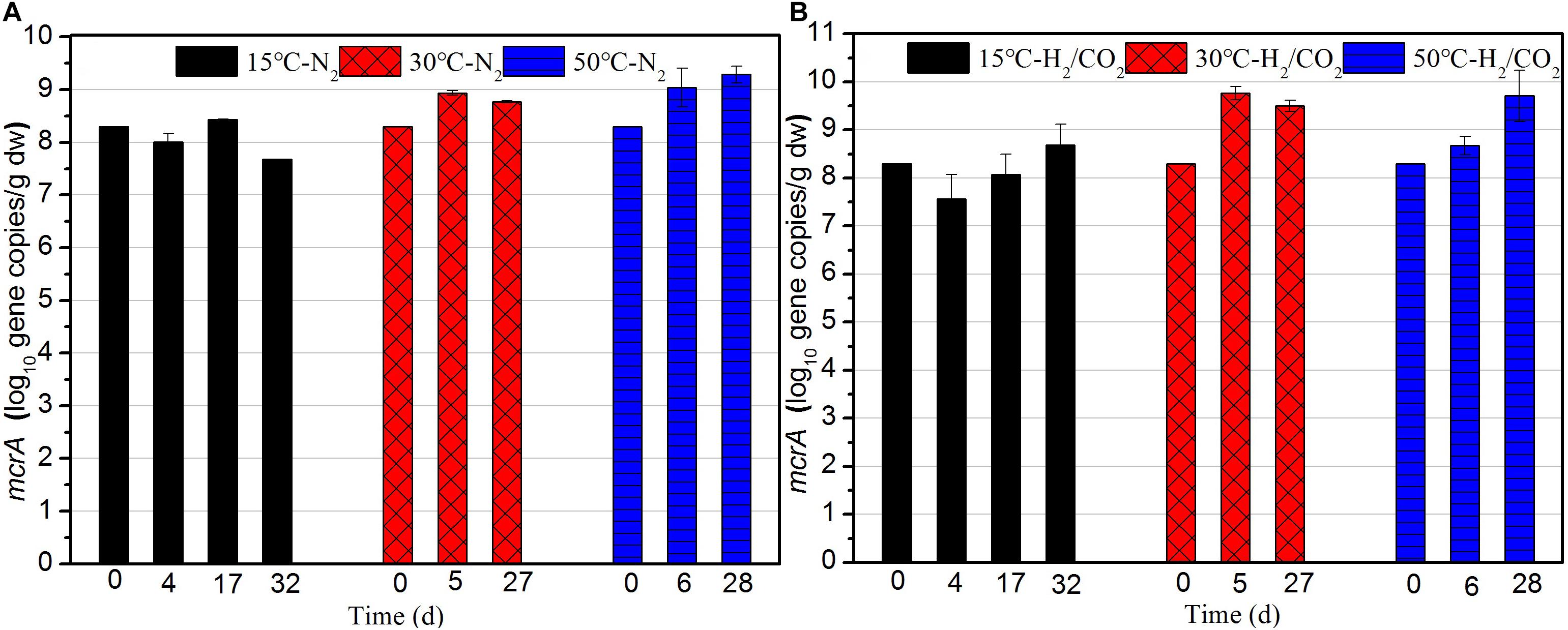
Figure 4. Copy numbers of mcrA gene during the (A) N2 controls and (B) H2/CO2 treatments of sewage sludge at 15, 30, and 50°C. Mean ± SD, n = 3.
The fhs gene, coding for the formyl tetrahydrofolate synthetase, was quantified as equivalent of the number of acetogens, and compared to the number of bacterial 16S rRNA gene copies. At low temperature, the initial copy numbers of fhs gene in the H2/CO2 incubations were one order of magnitude higher than those of the N2 control (Figure 5). The copy numbers of fhs gene at 30°C showed a same trend as at 15°C and the relative abundance under H2/CO2 was 19–40 times higher than that of the N2 control (Figure 5). At 50°C, addition of H2/CO2 did not affect the copy numbers and abundance of fhs gene (Figure 5).
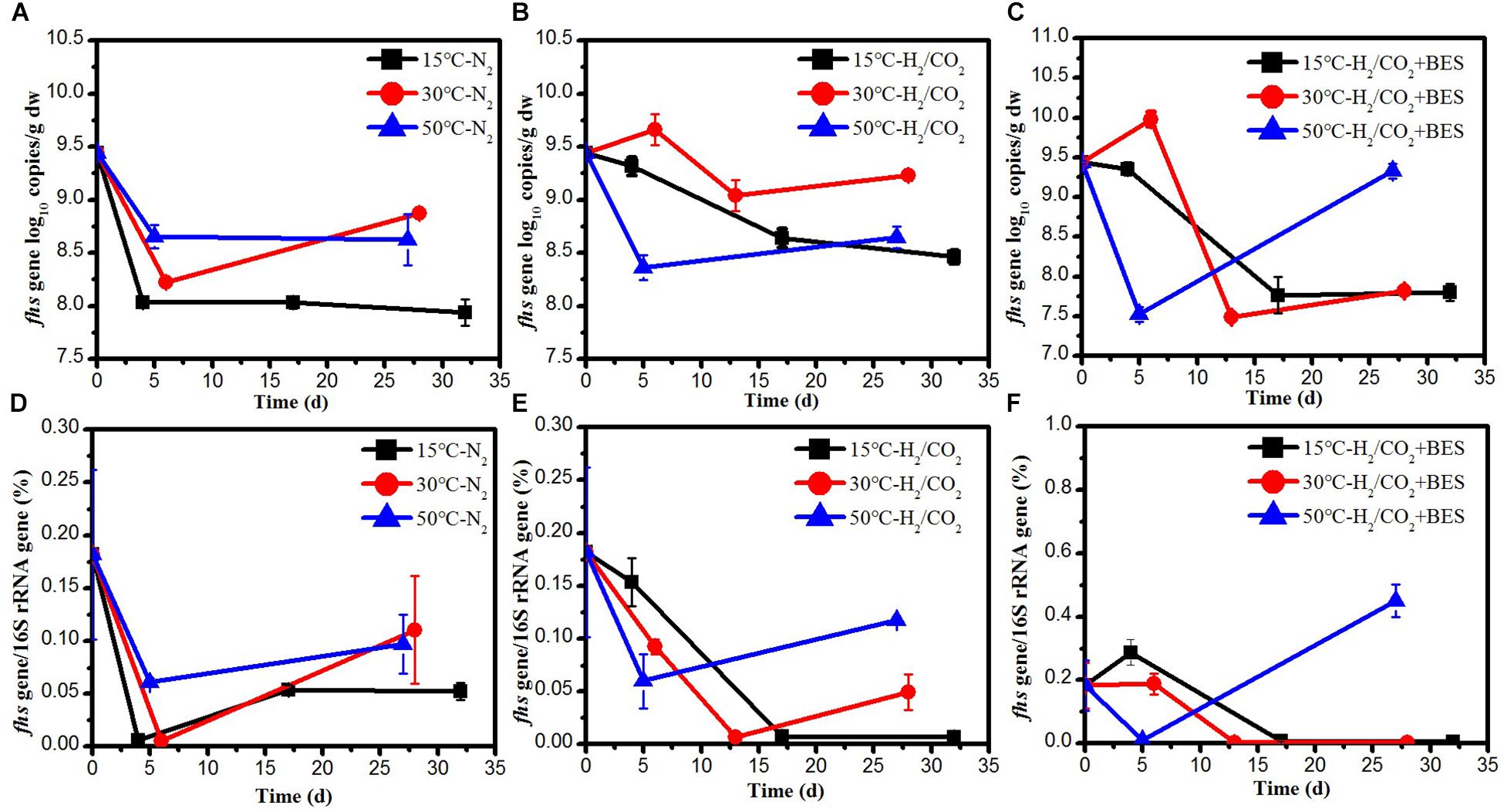
Figure 5. Copy numbers of (A–C) fhs gene and (D–F) fhs gene/16S gene during the treatment of sewage sludge at 15, 30, and 50°C, BES as an inhibiter of methanogenesis. Mean ± SD, n = 3.
Chemolithotrophic Acetogenesis Versus Hydrogenotrophic Methanogenesis Under Elevated H2/CO2 Concentration at Different Temperature
In order to interpret the competition for H2/CO2 between acetogens and methanogens at different temperatures, we determined the percentage of methane to the total products (methane + acetate) at the time of maximum acetate accumulation (Table 3). Methanogenesis contributed only marginally (3–4%) in presence of BES due to the inhibition of methanogenesis. However, hydrogenotrophic methanogenesis may has been the exclusive process (98–100%) for H2/CO2 consumption at 30°C, especially in the treatment with H2/CO2 (Table 3), which was also indicated by the initial and transient decrease of the δ13C of CH4 to values of −100.6‰ and final decrease again to −111.3‰ (Figure 2B). By contrast, acetogenesis contributed substantially at 15 and 50°C (Table 3). At 15°C, acetogenesis contributed only in the H2/CO2 treatment (78%), but at 50°C it also contributed much (82%) without exogenous H2/CO2 (Table 3). The transient accumulation of acetate at 50°C (especially in the N2 control) indicates that at the beginning of the incubation fermentative acetate production (in addition to chemolithotrophic acetogenesis) was faster than the consumption of acetate.
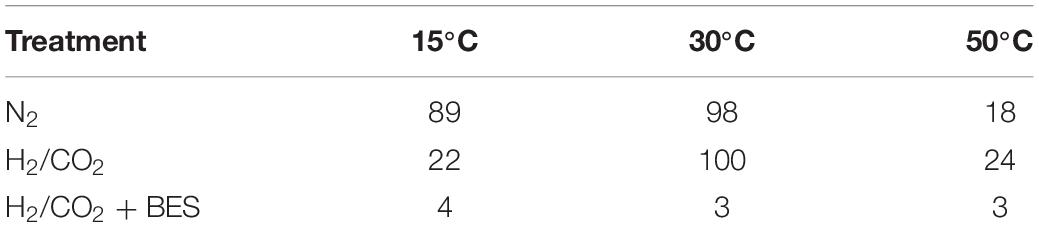
Table 3. Percentage of methane relative to total products (acetate + methane) formed at the time of maximum acetate accumulation.
Discussion
The Effect of Temperature on Competition Between Chemolithotrophic Acetogenesis and Hydrogenotrophic Methanogenesis
The competition of acetogens and methanogens for H2 is of great importance in many anoxic systems. However, the investigation of the competition between them is very complex. As the product of acetogens, acetate is also produced by fermentation and consumed by different metabolic pathways at the same time. Isotope technique is a reasonable approach to study the competition between acetogens and methanogens for H2 since chemolithotrophic acetogenesis and hydrogenotrophic methanogenesis result in a distinct 13C depletion of acetate and methane, respectively (Conrad, 2005; Ho et al., 2014; Gehring et al., 2016). Unfortunately, a complication arises from the fact that acetate concentrations in the anoxic environment are often too low for detection and isotopic analysis. Stimulation of chemolithotrophic acetogenesis and hydrogenotrophic methanogenesis by addition of H2 apparently allowed determination of reasonable 13C values of acetate and methane. Although the experiment set-up of exogenous H2 addition may not represent in situ condition, it still provides a maximum of further insight into the potential competition between acetogens and methanogens for H2.
The results of our study showed that the outcome of the competition between chemolithotrophic acetogenesis and hydrogenotrophic methanogenesis strongly depended on the incubation temperature. Collectively, our results suggest the following pathways for consumption of H2/CO2 (Figure 6). Chemolithotrophic acetogenesis consumed most of the added H2/CO2 at low temperature (15°C) and high (50°C) temperature. Hydrogenotrophic methanogenesis was the dominant pathway at middle (30°C) temperature. At high temperature, acetate was not only produced from H2/CO2 but also greatly from organic matter. Subsequently, the acetate was probably degraded by thermotolerant acetoclastic methanogens. A conversion of acetate to H2/CO2 (by the reversal of chemolithotrophic acetogenesis) was unlikely due to the relatively high H2 concentrations in the 50°C treatment, rendering this reaction thermodynamically endergonic.
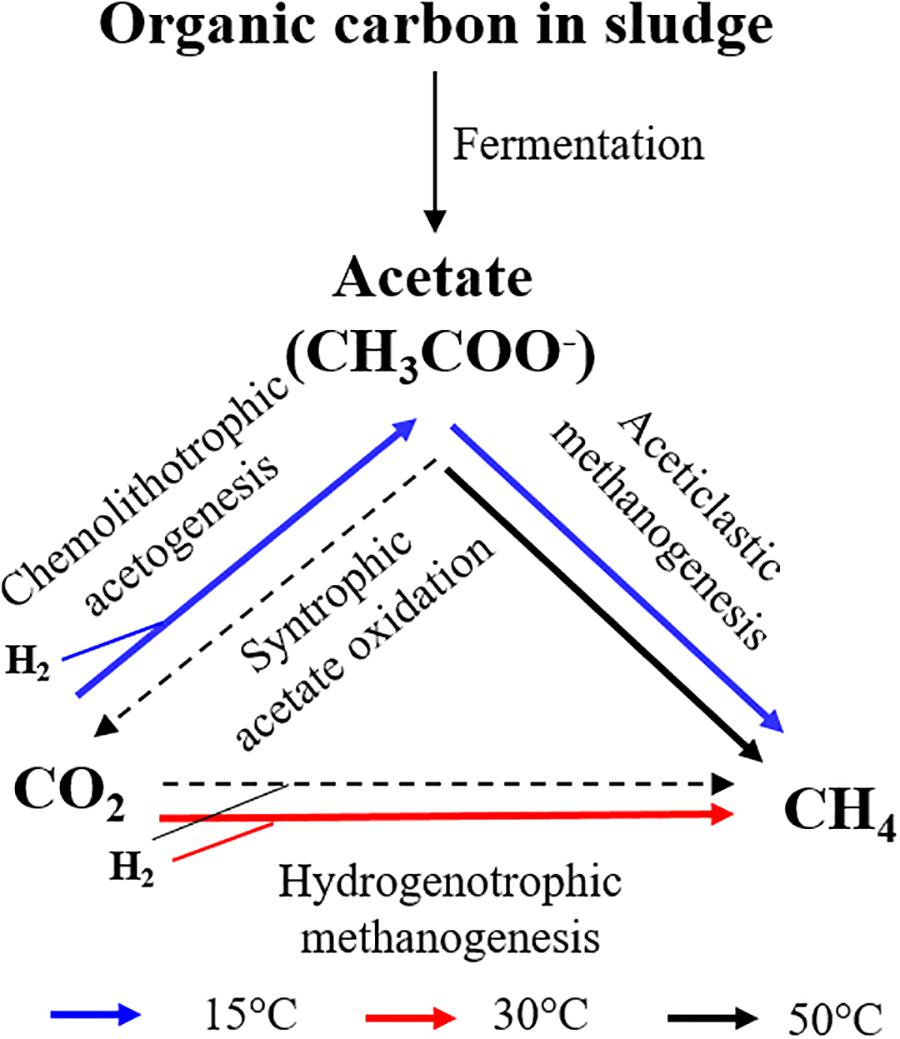
Figure 6. The pathways for consumption of H2/CO2 during the anaerobic digestion of sewage sludge at 15, 30, and 50°C.
At 15°C, the addition of H2/CO2 stimulated the production of acetate with isotopically low value (−41.1 to −43.3‰) indicating the operation of chemolithotrophic acetogenesis (Figure 1D). Furthermore, the decrease in δ13C values of acetate was paralleled by an increase of copy numbers of the fhs gene (Figure 5). The accumulated acetate was gradually exhausted, accompanied by a significant increase of δ13C-enriched CH4 and an increase of δ13Cacetate value (Figures 1B,D). Typically, the acetate-derived CH4 shows a smaller fractionation than the CO2-derived CH4 (Conrad, 2005; Gehring et al., 2015). Hence, the formed acetate from chemolithotrophic acetogenesis was mainly consumed by acetoclastic methanogens to produce CH4.
At 30°C, the ratios of methane to the total products in the treatments with H2/CO2 and the N2 controls were almost 100% (Table 3). The methane production under H2/CO2 was accompanied by very low δ13C values (−100.5 to −76.8‰) and increased copy numbers of the mcrA gene (Figures 2B, 4). This indicated that elevated H2/CO2 exclusively stimulated the formation of methane via hydrogenotrophic methanogenesis at mesophilic temperature.
At 50°C, the ratios of methane to the total products in the H2/CO2 incubations and N2 controls were only 24 and 18%, respectively. Hence much of the H2/CO2 was converted to acetate similarly as at 15°C. However, the stoichiometry of acetate production indicated that an additional part was produced from fermentation of organic matters (Heuer et al., 2010). The copy numbers of the fhs gene were similar with those in the N2 controls. The acetate was transiently produced and paralleled by an increase in δ13C values of acetate due to acetate consumption (Figures 3C,D). The isotopically enriched acetate was eventually and completely consumed, followed by the production of 13C-depleted CH4, which was produced after day 16 until the end of incubation (Figure 3B). Collectively, these observations can be explained by chemolithotrophic acetogenesis from H2/CO2, followed by aceticlastic methanogenesis. However, the relatively high and constant H2 concentrations during the latter incubation are not easily explained. Perhaps, they were caused by small H2 production from aceticlastic methanogens (Kulkarni et al., 2018).
Compared to the N2 controls, the presence of exogenous H2 significantly affected the percentage of methane relative to the total products formed only at 15°C (Table 3), which indicated that chemolithotrophic acetogenesis was more favored at low than at medium and high temperatures. This has also been shown in our previous study of rice field soils (Liu and Conrad, 2011; Fu et al., 2018). Acetogens have at low temperatures higher growth rates than most methanogens (Kotsyurbenko et al., 2001). Under mesophilic conditions, however, methanogenesis is generally energetically more beneficial than acetogenesis, and also exhibits a higher cell-specific affinity for substrate, resulting in much stronger H2/CO2 utilization via hydrogenotrophic methanogenesis than via homoacetogenesis (Hoehler et al., 2002; Conrad et al., 2008). At thermophilic temperatures, acetate production from H2/CO2 was augmented by heterotrophic acetate production.
Implication for Sludge Digestion Operation
This study illuminates the carbon flow in sludge anaerobic digestion under elevated H2/CO2 concentrations at different temperatures. This understanding deepens our knowledge of methanogenesis pathways involved in anaerobic digestion of sewage sludge, which are fundamental for improvement or regulation of the anaerobic digestion process. Temperature regulation strategy may be used for sludge digestion operation. Thermophilic digestion facilitates syntrophic acetate oxidization, which helps relieve methanogens from substrate inhibition such as high ammonia and high acetate concentration (Hao et al., 2011; Wang et al., 2015; Westerholm et al., 2019). As such, thermophilic digestion could potentially apply to ammonia-rich wastes such as cattle and pig manure or easily degradable wastes such as food waste for methane production.
Methane has a low monetary value. Therefore, more and more attention has been paid to the promises and challenges of an undefined-mixed-culture process to generate a mixture of carboxylates as intermediate platform chemicals toward generation of complex fuels from wastes (Agler et al., 2011). As useful chemical, acetate can be generated from fermentation and homoacetogenesis during anaerobic digestion. Thermophilic digestion enables high hydrolysis and fermentation efficiencies, which could allow efficient acetate accumulation from organic wastes. Additionally, elevated H2/CO2 concentrations at low temperatures is beneficial to homoacetogenesis, enabling higher production of acetate than methane. Our previous study has reported a novel system coupling glucose fermentation and homoacetogenesis for elevated acetate production (Nie et al., 2008; Ni et al., 2010). When aiming at higher acetate production from sludge, a two-stage thermophilic-psychrophilic AD process coupling fermentation and homoacetogenesis is an alternative approach, with the first stage operated at high temperature (50–55°C) to enable fast hydrolysis and fermentation, and the second stage at 10–15°C under elevated H2/CO2 concentration derived from the first stage to enable efficient acetogenesis.
Data Availability Statement
The datasets generated for this study are available on request to the corresponding author.
Author Contributions
BF planned, designed, and performed the experiments as well as revised the manuscript. XJ participated in performing the experiments and wrote the manuscript. RC designed the experiments and analyzed the results as well as revised the manuscript. HoL assisted in the performance of experiments and revisions of the final manuscript. HeL conceived and coordinated the study, and revised the final manuscript. All authors read and approved the final version of the manuscript.
Funding
This research was supported by the Natural Science Foundation of Jiangsu Province (BK20181344), the National Natural Science Foundation of China (51678280), and the Jiangsu Key Laboratory of Anaerobic Biotechnology (JKLAB201711).
Conflict of Interest
The authors declare that the research was conducted in the absence of any commercial or financial relationships that could be construed as a potential conflict of interest.
Supplementary Material
The Supplementary Material for this article can be found online at: https://www.frontiersin.org/articles/10.3389/fmicb.2019.02418/full#supplementary-material
References
Adekunle, F. K., and Okolie, A. J. (2015). A review of biochemical process of anaerobic digestion. Adv. Biosci. Biotechnol. 6, 205–212. doi: 10.4236/abb.2015.63020
Agler, T. M., Wrenn, A. B., Zinder, H. S., and Angenent, T. L. (2011). Waste to bioproduct conversion with undefined mixed cultures: the carboxylate platform. Trends Biotechnol. 29, 70–78. doi: 10.1016/j.tibtech.2010.11.006
Angel, R., Claus, P., and Conrad, R. (2011). Methanogenic archaea are globally ubiquitous in aerated soils and become active under wet anoxic conditions. ISME J. 6, 847–862. doi: 10.1038/ismej.2011.141
Blaser, M. B., Dreisbach, L. K., and Conrad, R. (2013). Carbon isotope fractionation of 11 acetogenic strains grown on H2 and CO2. Appl. Environ. Microb. 79, 1787–1794. doi: 10.1128/AEM.03203-12
Chin, K. J., and Conrad, R. (2010). Intermediary metabolism in methanogenic paddy soil and the influence of temperature. FEMS Microbiol. Ecol. 18, 85–102. doi: 10.1016/0168-6496(95)00042-9
Conrad, R. (2005). Quantification of methanogenic pathways using stable carbon isotopic signatures: a review and a proposal. Org. Geochem. 36, 739–752. doi: 10.1016/j.orggeochem.2004.09.006
Conrad, R., Bak, F., Seitz, H. J., Thebrath, B., Mayer, H. P., and Schütz, H. (1989). Hydrogen turnover by psychrotrophic homoacetogenic and mesophilic methanogenic bacteria in anoxic paddy soil and lake sediment. FEMS Mocrobiol. Ecol. 62, 285–294. doi: 10.1016/0378-1097(89)90010-4
Conrad, R., Liu, S., and Drake, H. (2008). “Temperature effects on methanogenic microbial communities,” in Microbes and the Environment: Perspectives and Challenges, eds S. J. Liu, and H. L. Drake, (Beijing: Science Press), 35–40.
Conrad, R., and Wetter, B. (1990). Influence of temperature on energetics of hydrogen metabolism in homoacetogenic, methanogenic, and other anaerobic bacteria. Arch. Microbiol. 155, 94–98. doi: 10.1007/bf00291281
Fu, B., Conrad, R., and Blaser, M. (2018). Potential contribution of acetogenesis to anaerobic degradation in methanogenic rice field soils. Soil Biol. Biochem. 119, 1–10. doi: 10.1016/j.soilbio.2017.10.034
Galand, P., Yrjälä, K., and Conrad, R. (2010). Stable carbon isotope fractionation during methanogenesis in three boreal peatland ecosystems. Biogeosciences 7, 3893–3900. doi: 10.5194/bg-7-3893-2010
Gehring, T., Klang, J., Niedermayr, A., Berzio, S., Immenhauser, A., Klocke, M., et al. (2015). Determination of methanogenic pathways through carbon isotope (δ13C) analysis for the two-stage anaerobic digestion of high-solids substrates. Environ. Sci. Technol. 49, 4705–4714. doi: 10.1021/es505665z
Gehring, T., Niedermayr, A., Berzio, S., Immenhauser, A., Wichern, M., and Lübken, M. (2016). Determination of the fractions of syntrophically oxidized acetate in a mesophilic methanogenic reactor through an 12C and 13C isotope based kinetic model. Water Res. 102, 362–373. doi: 10.1016/j.watres.2016.06.038
Hao, L. P., Lü, F., He, P. J., Li, L., and Shao, L. M. (2011). Predominant contribution of syntrophic acetate oxidation to thermophilic methane formation at high acetate concentrations. Environ. Sci. Technol. 45, 508–513. doi: 10.1021/es102228v
Heuer, V. B., Krüger, M., Elvert, M., and Hinrichs, K. U. (2010). Experimental studies on the stable carbon isotope biogeochemistry of acetate in lake sediments. Org. Geochem. 41, 22–30. doi: 10.1016/j.orggeochem.2009.07.004
Ho, D. P., Jensen, P. D., and Batstone, D. J. (2013). Methanosarcinaceae and acetate-oxidizing pathways dominate in high-rate thermophilic anaerobic digestion of waste-activated sludge. Appl. Environ. Microb. 79, 6491–6500. doi: 10.1128/AEM.01730-13
Ho, D., Jensen, P., and Batstone, D. (2014). Effects of temperature and hydraulic retention time on acetotrophic pathways and performance in high-rate sludge digestion. Environ. Sci. Technol. 48, 6468–6476. doi: 10.1021/es500074j
Hoehler, T. M., Albert, D. B., Alperin, M. J., Bebout, B. M., Martens, C. S., and Des Marais, D. J. (2002). Comparative ecology of H2 cycling in sedimentary and phototrophic ecosystems. Antonie Van Leeuwenhoek 81, 575–585.
Imachi, H., Sakai, S., Sekiguchi, Y., Hanada, S., Kamagata, Y., Ohashi, A., et al. (2008). Methanolinea tarda gen. nov., sp. nov., a methane-producing archaeon isolated from a methanogenic digester sludge. Int. J. Syst. Evol. Microbiol. 58, 294–301. doi: 10.1099/ijs.0.65394-0
Ji, Y., Liu, P., and Conrad, R. (2018). Change of the pathway of methane production with progressing anoxic incubation of paddy soil. Soil Biol. Biochem. 121, 177–184. doi: 10.1016/j.soilbio.2018.03.014
Kelessidis, A., and Stasinakis, S. A. (2012). Comparative study of the methods used for treatment and final disposal of sewage sludge in European countries. Waste Manage. 32, 1186–1195. doi: 10.1016/j.wasman.2012.01.012
Kotsyurbenko, O. R., Glagolev, M. V., Nozhevnikova, A. N., and Conrad, R. (2001). Competition between homoacetogenic bacteria and methanogenic archaea for hydrogen at low temperature. FEMS Microbiol. Ecol. 38, 153–159. doi: 10.1016/s0168-6496(01)00179-9
Kulkarni, G., Mand, D. T., and Metcalf, W. W. (2018). Energy conservation via hydrogen cycling in the methanogenic archaeon Methanosarcina barkeri. Mbio 9, e01256-18. doi: 10.1128/mBio.01256-18
Liu, F., and Conrad, R. (2011). Chemolithotrophic acetogenic H2/CO2 utilization in Italian rice field soil. ISME J. 5, 1526–1539. doi: 10.1038/ismej.2011.17
Liu, R., Hao, X., and Wei, J. (2016). Function of homoacetogenesis on the heterotrophic methane production with exogenous H2/CO2 involved. Chem. Eng. J. 284, 1196–1203. doi: 10.1016/j.cej.2015.09.081
Lopes, M., Alves, J. I., Arantes, A. L., Belo, I., Sousa, D. Z., and Alves, M. M. (2015). Hydrogenotrophic activity under increased H2/CO2 pressure: effect on methane production and microbial community. J. Biotechnol. 208:S57.
Ni, B. J., He, L., Nie, Y. Q., Zeng, R. J., Du, G. C., Chen, J., et al. (2010). Coupling glucose fermentation and homoacetogenesis for elevated acetate production: experimental and mathematical approaches. Biotechnol. Bioeng. 108, 345–353. doi: 10.1002/bit.22908
Nie, Y. Q., Liu, H., Du, G. C., and Chen, J. (2008). Acetate yield increased by gas circulation and fed-batch fermentation in a novel syntrophic acetogenesis and homoacetogenesis coupling system. Bioresour. Technol. 99, 2989–2995.
Niu, D. J., Hui, H., Dai, X. H., and Zhao, Y. C. (2013). Greenhouse gases emissions accounting for typical sewage sludge digestion with energy utilization and residue land application in China. Waste Manage. 33, 123–128. doi: 10.1016/j.wasman.2012.06.024
Olivier, M. F. (2016). Temperature and Salinity Controls on Methanogenesis in an Artificial Freshwater Lake. Cardiff Bay: Cardiff University.
Shanmugam, S. R., Chaganti, S. R., Lalman, J. A., and Heath, D. D. (2014). Statistical optimization of conditions for minimum H2 consumption in mixed anaerobic cultures: effect on homoacetogenesis and methanogenesis. Int. J. Hydrogen Energy 39, 15433–15445.
Stumm, W., and Morgan, J. J. (1981). Aquatic Chemistry: An Introduction Emphasizing Chemical Equilibria in Natural Waters. New York: Wiley.
Wang, H., Fotidis, A. I., and Angelidaki, I. (2015). Ammonia effect on hydrogenotrophic methanogens and syntrophic acetate-oxidizing bacteria. FEMS Microbiol. Ecol. 91, 1–8. doi: 10.1093/femsec/fiv130
Westerholm, M., Dolfing, J., and Schnürer, A. (2019). Growth characteristics and thermodynamics of syntrophic acetate oxidizers. Environ. Sci. Technol. 53, 5512–5520. doi: 10.1093/femsec/fiv130
Xu, S., Fu, B., Zhang, L., and Liu, H. (2015). Bioconversion of H2/CO2 by acetogen enriched cultures for acetate and ethanol production: the impact of pH. World J. Microbiol. Biotechn. 31, 941–950. doi: 10.1007/s11274-015-1848-8
Xu, K., Liu, H., Du, G., and Chen, J. (2009). Real-time PCR assays targeting formyltetrahydrofolate synthetase gene to enumerate acetogens in natural and engineered environments. Anaerobe 15, 204–213. doi: 10.1016/j.anaerobe.2009.03.005
Keywords: methanogenesis, acetogenesis, carbon isotope, temperature, H2/CO2 utilization
Citation: Fu B, Jin X, Conrad R, Liu H and Liu H (2019) Competition Between Chemolithotrophic Acetogenesis and Hydrogenotrophic Methanogenesis for Exogenous H2/CO2 in Anaerobically Digested Sludge: Impact of Temperature. Front. Microbiol. 10:2418. doi: 10.3389/fmicb.2019.02418
Received: 12 July 2019; Accepted: 07 October 2019;
Published: 23 October 2019.
Edited by:
Mirko Basen, University of Rostock, GermanyReviewed by:
Oliver Schmidt, University of Bayreuth, GermanyAlfons Stams, Wageningen University & Research, Netherlands
Copyright © 2019 Fu, Jin, Conrad, Liu and Liu. This is an open-access article distributed under the terms of the Creative Commons Attribution License (CC BY). The use, distribution or reproduction in other forums is permitted, provided the original author(s) and the copyright owner(s) are credited and that the original publication in this journal is cited, in accordance with accepted academic practice. No use, distribution or reproduction is permitted which does not comply with these terms.
*Correspondence: He Liu, bGl1aGVAamlhbmduYW4uZWR1LmNu