- 1Brazilian Synchrotron Light Laboratory, Brazilian Center for Research in Energy and Materials, Campinas, Brazil
- 2Institute of Physics of São Carlos, University of São Paulo, São Paulo, Brazil
- 3Interunits Graduate Program in Biotechnology, University of São Paulo, São Paulo, Brazil
- 4Fundamental Chemistry Department, University of São Paulo, São Paulo, Brazil
The biogenicity problem of geological materials is one of the most challenging ones in the field of paleo and astrobiology. As one goes deeper in time, the traces of life become feeble and ambiguous, blending with the surrounding geology. Well-preserved metasedimentary rocks from the Archaean are relatively rare, and in very few cases contain structures resembling biological traces or fossils. These putative biosignatures have been studied for decades and many biogenicity criteria have been developed, but there is still no consensus for many of the proposed structures. Synchrotron-based techniques, especially on new generation sources, have the potential for contributing to this field of research, providing high sensitivity and resolution that can be advantageous for different scientific problems. Exploring the X-ray and matter interactions on a range of geological materials can provide insights on morphology, elemental composition, oxidation states, crystalline structure, magnetic properties, and others, which can measurably contribute to the investigation of biogenicity of putative biosignatures. Here, we provide an overview of selected synchrotron-based techniques that have the potential to be applied in different types of questions on the study of biosignatures preserved in the geological record. The development of 3rd and recently 4th generation synchrotron sources will favor a deeper understanding of the earliest records of life on Earth and also bring up potential analytical approaches to be applied for the search of biosignatures in meteorites and samples returned from Mars in the near future.
Introduction
Elucidating the origin and evolution of life on Earth, as well as the possibilities of its occurrence and preservation outside the planet are topics of primordial interest to the astrobiological community. For the search of life in extraterrestrial worlds, distinguishing and understanding the preservation of the earliest records of life on Earth is a step of fundamental importance. Although evidences of life have been reported in rocks with more than 3.7 billion years (Ohtomo et al., 2014), several descriptions of very ancient chemical or morphological biosignatures have been debated by the scientific community over the past decades (Brocks et al., 1999; Brasier et al., 2015; Nutman et al., 2016; Dodd et al., 2017; Schopf et al., 2018). The challenge becomes even greater when the putative biosignatures are from outside Earth (whether in meteorites, or chemical or morphological signals measured in situ or that will be collected in future missions to Mars). The Viking missions on the 1970’s found chemical traces that could be related to metabolism on the surface of Mars (Levin and Straat, 1977), which were later contested. The same happened in the 1990’s, when McKay et al. (1996) described supposed biological microscopic structures on the ALH84001 meteorite. More recently, Noffke (2015) presented sedimentary structures resembling terrestrial microbially-induced sedimentary structures (MISS) on Mars, based on morphological analysis of images from the rover Curiosity, which instigated the astrobiological community in the hope of finding chemical biosignatures or other evidences that could support their biogenicity.
The application of analytical techniques in paleo and astrobiology has contributed to the advancement of knowledge about the indicatives of past or present biological activity from macroscopic to nanometric scales. Techniques such as Raman Spectroscopy, X-ray Diffraction, X-ray Tomography, Electron Microscopy and nanoscale secondary ion mass spectrometry (Nano SIMS) are now being routinely applied on Earth sciences. Meanwhile, techniques based on synchrotron light are gaining more space as promising tools for the non-destructive investigation of paleobiological samples, giving rise to an emerging field known as paleometry (Gomes et al., 2019). Some of these techniques are summarized in Table 1.
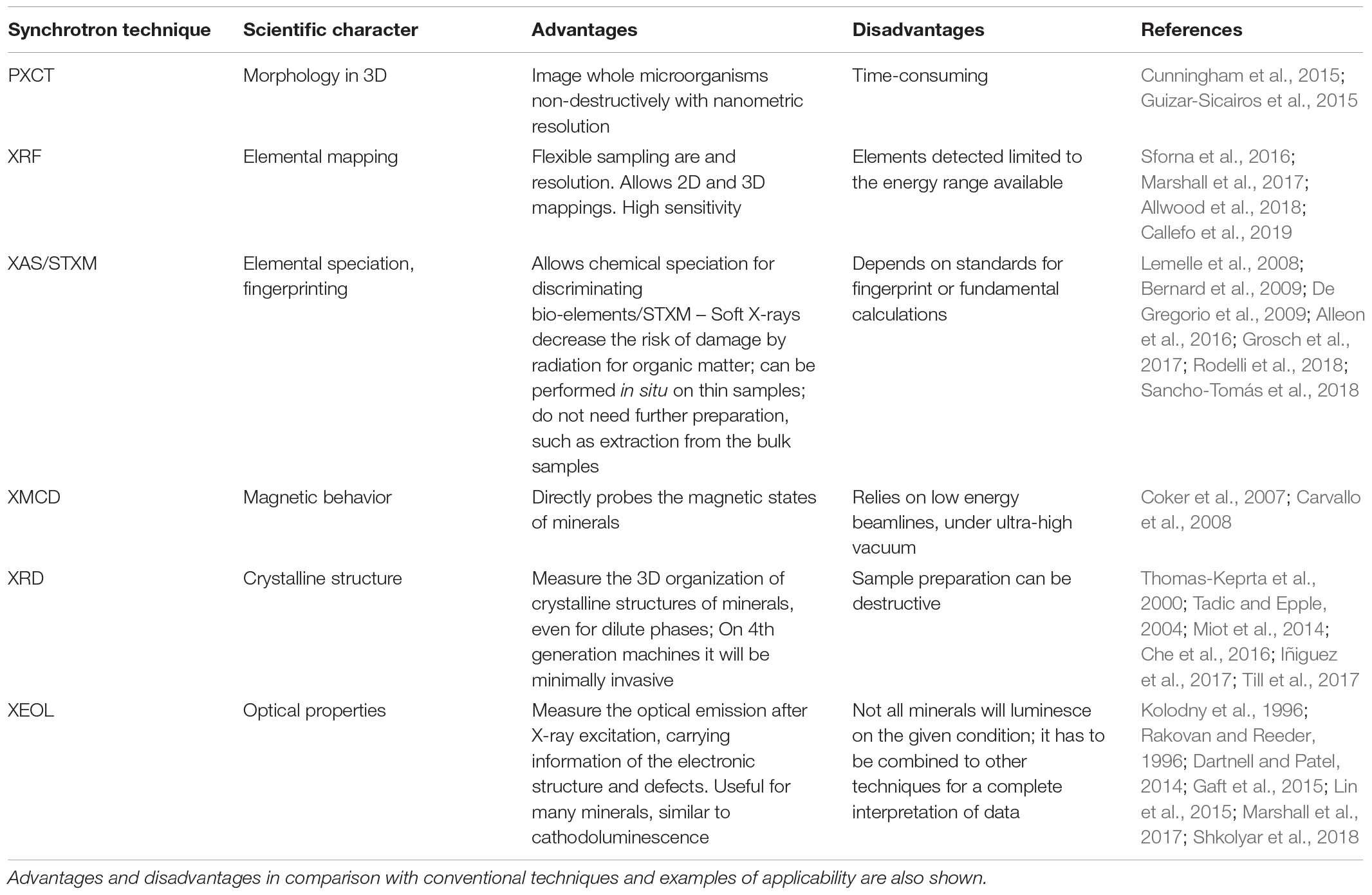
Table 1. List of selected synchrotron-based techniques for assessing scientific characters related to biogenicity.
Synchrotron accelerators produce light with unique qualities, such as high brilliance (flux of photons per unit area), broad, continuous energy range, and high coherence, which are unattainable by conventional light sources, such as lasers, lamps or X-ray tubes. These characteristics allow the use of multiple physical phenomena to unveil the original elemental and molecular composition, mineralogy and morphology of samples, with higher sensitivity, penetrability and resolution than conventional techniques applied for the same purposes. In the present review, we will discuss recent applications and potentials of some synchrotron-based techniques for evaluating biosignatures in the geological record.
Biosignatures and Implications
By definition, biosignatures are any signs, objects, substances, and/or patterns generated by biological activity (Des Marais et al., 2003). The definition of biosignature, its applicability for exoplanets and how it can be used as analogs for the search of life outside Earth have been the focus of several studies such as White (1974), Walsh (1992), Schopf (1993), Mojzsis et al. (1996), Des Marais and Walter (1999), Brocks et al. (1999), Westall et al. (2000), Banfield et al. (2001), Javaux et al. (2001), Des Marais et al. (2002), Gorbushina et al. (2002), Thomas-Keprta et al. (2002), van Zuilen et al. (2002), Garcia-Ruiz et al. (2003), Lindsay et al. (2005), Douglas et al. (2008), Summons et al. (2008), Westall (2008), Westall (2009), Westall and Cavalazzi (2011), Cockell (2014), Brasier et al. (2015), Meadows (2017), Catling et al. (2018), Meadows et al. (2018), Schwieterman et al. (2018), Walker et al. (2018), Westall et al. (2019), among several others.
Biosignatures include, among others, (i) morphological features, such as preserved cells or extracellular components, as fossilized extracellular polymeric substance (EPS); (ii) biogenic minerals, such as those biologically-induced (i.e., biogenic dolomite precipitated in microbialites and microbial mats) or biologically-controlled (i.e., endoskeletons and hard mineralized parts of metazoans), which sometimes present crystallographic characteristics and/or physical properties that can be distinguished for those abiotic minerals (i.e., biogenic magnetite, such as magnetites produced inside the cells of magnetotactic bacteria (magnetosomes); (iii) specific textures or biogenic fabrics in rocks originated by microbial activity, such as fenestral fabrics in microbialites; (iv) molecular vestiges linked to biological activity, such as organic ligands, lipids, organic macromolecules, etc; (v) specific chemical characteristics of bioprocessing, such as chirality in organic molecules; (vi) stable isotopes favorable to biogenic patterns and bioprocessing. Interpreting these signals as biosignatures is not always trivial, especially if these samples are from deep time context (Javaux, 2019). One example is the distribution pattern of bio-elements (trace or major) in the samples of interest. The interpretation of signals of past life in these distributions may be complex and others aspects need to be considered, such as the co-occurrence of other types of biosignatures, such as morphological and chemical (isotopes, co-localization of elements, combinations with organic signatures, etc.), or characteristics of the authigenic mineralogy and properties of the original geological context.
The complexity of interpretation also increase considering the possibilities of posterior contaminations and the several changes in the original characteristics of mineralogy and geological features, the action of metamorphism and changes of temperature which can degrade or modify the organic biosignatures, besides several other alterations during the post-burial processes over time. Thus, it is important to do critical interpretations of the signs of early life in order to avoid misinterpretations and/or equivocal biosignatures. There are several examples in the literature regarding the contestation or reassessment of alleged evidence of ancient life on Earth and outside it (McKay et al., 1996; Golden et al., 2000; Thomas-Keprta et al., 2002, 2009; Steele et al., 2012). Some will be discussed in the following specific sessions.
Imaging Morphological Biosignatures
Morphological traces of microorganisms are important biosignatures for providing direct insights into ancient life ultrastructure, evolution, paleo-environment and also to help to understand the physico-chemical conditions that allowed their long-term preservation. High resolution microscopies applied so far, such as Scanning Electron Microscopy (SEM) or Transmission Electron Microscopy (TEM) have allowed significative advances on the understanding of the earliest fossilized microbes. Their application, however, is limited by the low penetration depth of electrons, thus requiring destructive sample preparation for exposing the structures from within the rock matrix (potentially introducing artifacts), or by preparing ultra thin (<100 nm) sections that can sample only a small fraction of the specimen. 3D images can be achieved by using a focused ion beam (FIB) coupled to a SEM (FIB-SEM), and combining sequential milling with concurrent Energy Dispersisve Spectroscopy (EDS-SEM) imaging. Although being a destructive approach, it has allowed even some of the most ancient microfossils to be investigated at the nanoscale, providing insights into their chemistry, ultrastructure, taphonomy and investigating the biogenicity of these structures (Wacey et al., 2012; Brasier et al., 2015).
X-rays can penetrate objects and provide information of their interior non-destructively, therefore having the potential of overcoming the limitations of electron microscopy. Conventional X-ray imaging, such as the widely known Computed Tomography (CT), is based in the absorption of X-rays, a physical interaction which relies on the densities and/or atomic numbers of the materials comprising the specimens. For mineralized structures such as some fossils, this can represent a limitation in contrast. Synchrotron sources allow other contrast modalities to be explored, such as the phase contrast. Phase-contrast μ-CT has become critical for paleobiological studies in the last decades (Tafforeau et al., 2006; Cunningham et al., 2012; Maldanis et al., 2016), due to its capacity of extracting 3D information from these homogeneously dense specimens non-invasively, revealing even the preservation of soft tissues. Recently, the limit of resolution of X-ray microscopy has been pushed forward by the development of techniques based on Coherent Diffraction Imaging (CDI), specially Ptychography. This lensless method allows specimens of tens of microns to be imaged with nanometric resolution, and can also be applied in 3D, receiving the name of Ptychographic X-Ray Computed Tomography, or PXCT (Holler et al., 2014). This imaging approach is based in the collection in the far-field of diffraction patterns partially superposed while scanning the sample. This redundancy of measurements allows the reconstruction of the specimen’s complex refraction index (both absorption and phase components) using iterative algorithms instead of X-ray lenses. PXCT has been only briefly applied to the study of microfossils (Cunningham et al., 2015; Guizar-Sicairos et al., 2015), and its potential for evaluating the biogenicity of morphological biosignatures has still to be further explored. Nevertheless, its potential for unveiling whole fossilized microbes within their geological context also configures it as a potential methodology to be used for searching morphological biosignatures potentially preserved in rocks returned from Mars in the near-future.
Elemental Mapping of Chemical Biosignatures
Metabolic processes of biological systems can generate traces or patterns uncommon to abiotic systems. For geobiological materials, these biosignatures can be present in the form of biominerals with different crystalline organizations or specific distributions, mineral assemblages in association with organic matter and modifications on the mineral surface (Banfield et al., 2001). Some elements are considered to be bio-essential and bio-functional (i.e., P, S, Ti, V, Cr, Mn, Fe, Co, Ni, Cu, Zn, Mo, As, and Pb) (Williams and Frausto da Silva, 1996), and when co-localized and/or associated to specific morphological patterns, such as layered distribution or in consonance with organic matter, they could represent biosignatures, including results of biological processes. Moreover, the geochemical composition and growth processes of microbial mats favor the binding of some metals, which can be reorganized during the development of the community, forming biominerals or being adsorbed on the surface of minerals.
Identifying and mapping the chemical elements present in geobiological structures can provide means of supporting their biogenicity and also understanding their ecological interactions and modes of preservation. Nevertheless, identifying these elements and distinguishing patterns generated by biotic from abiotic processes require approaches with high-spatial resolution, sensitivity to a broad range of elements and to trace element concentrations. In questions about biogenicity studies, in particular for elemental distribution analyzes such as X-ray fluorescence mapping, it is important to consider the co-location of elements with morphological structures of interest, original lithology and its possible changes, the co-occurrence of biogeochemical elements of interest and also their abundance limits taking into account the geological and preservational context. The combination of as much evidence as possible that can explore the depositional history, and especially the diagenetic alterations that may have exerted any changes in the preservation of authigenic characteristics, it is important to minimize any misinterpretation, especially in deep time rocks.
Synchrotron-based X-ray fluorescence (SR-XRF) allows the identification, mapping and semi-quantification of chemical elements even in concentrations of parts per billion (ppb), and has a spatial resolution primarily dependent on the size of the X-ray beam, which, in the case of the new generation synchrotrons, can reach nanometric dimensions. The range of elements that can be detected with this approach depends on the energy of the X-rays, once the fluorescence phenomenon relies on the removal of strongly-binded inner-shell electrons, creating vacancies that need to be filled by external electrons. The difference of energy between the electrons involved in this process is emitted as photons, generating a fluorescence pattern which is specific to each element. One example of case study using SR-μ-XRF was applied to discuss the biogenicity of pyrites in a microbial context in order to understand the role of bacteria in the tafonomical process of well-preserved fossilized organisms in Crato Basin, Brazil (Osés et al., 2017). The authors combined morphological biosignatures, such as putative fossilized bacterial EPS observed in SEM with framboidal pyrites, using the SR-μ-XRF mapping to identify some metals (i.e., Fe, Cu, and Zn) which could be incorporated in the system by microbial activity (Figure 1).
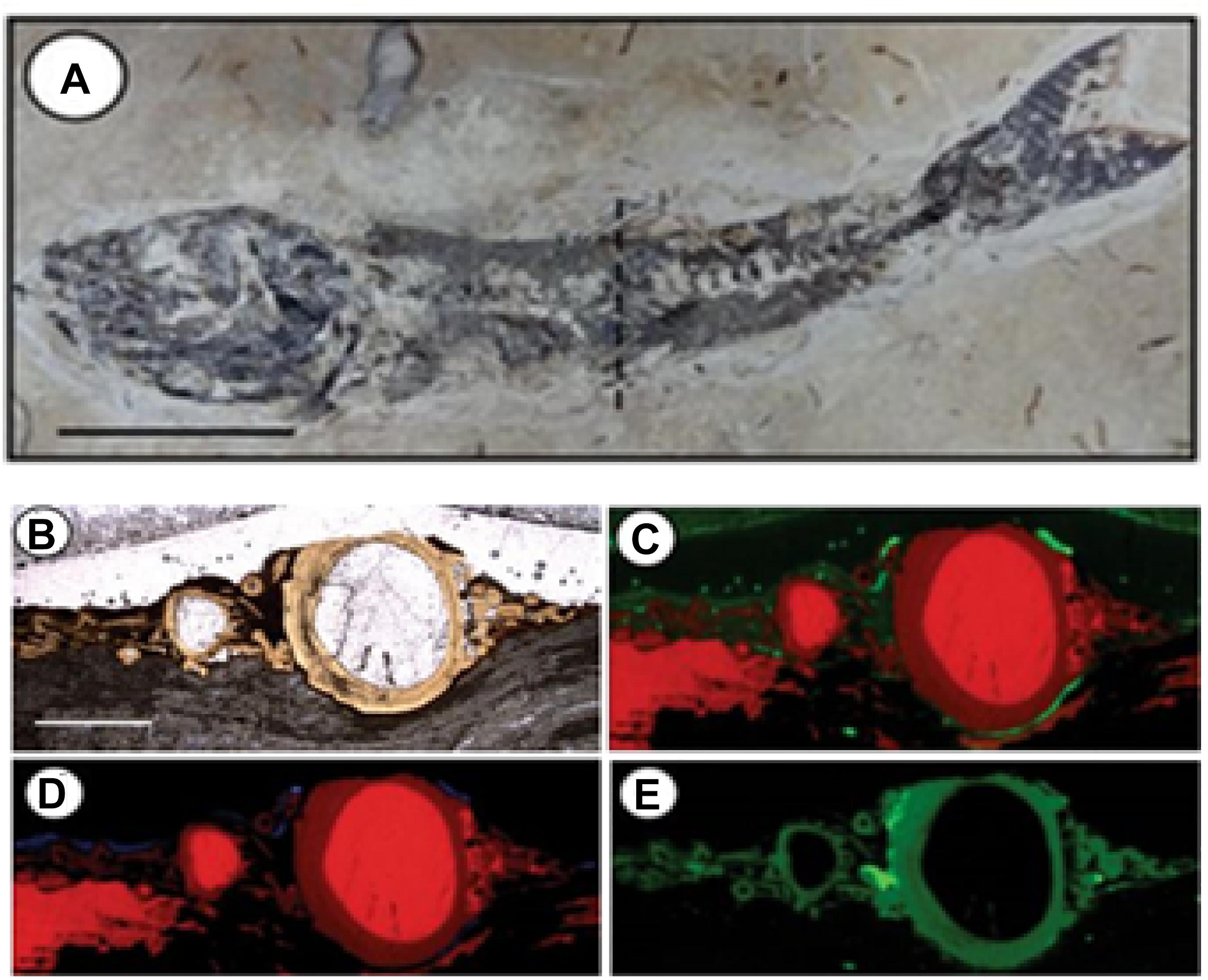
Figure 1. Application of synchrotron-based X-ray microfluorescence in fossil fish from Crato Member, Brazil (Osés et al., 2017), showing the potential of elemental mapping in providing information about the original elemental composition (interpreted as biosignatures) and diagenetic processes (interpreted as secondary incorporation). In this case, the authors used the elemental mapping to identify some metals (i.e., Fe, Cu, and Zn) related to the incorporation by microbial activity, in order to discuss the biogenicity of pyrites and understand the role of bacteria in the tafonomical process. (A) Mapped specimen (mapped area in a cross section in the dashed line), scale: 1.5 cm; (B) area mapped by X-ray microfluorescence, scale: 2 mm; (C) Ca-red, Fe-green; (D) Ca-red, Cu-blue; (E) Zn (modified from Osés et al., 2017). Reprinted by permission from Scientific Reports (open access), Creative Commons CC BY, Copyright (2017), Springer Nature.
Sforna et al. (2016) used SR-μXRF and complementary approaches to assess the metal incorporation in living microbialites and their remobilization during a simulated diagenetic processes. They mapped the distribution of metals triggered by secondary abiotic processes and provided a basis of comparison for evaluating ancient Precambrian microbialites. Recently, SR-μ-XRF was also applied in combination with conventional techniques and magnetic analysis by Callefo et al. (2019), in order to evaluate the biogenicity of iron minerals in carboniferous rhythmites (periodic sedimentary depositions). The distribution pattern of iron in co-occurrence with putative MISS and organic matter, plus the magnetic signal compatible with biogenic magnetite, indicated a biological origin for the iron minerals, allowing a reassessment the depositional history of the geological site.
Studying organic-walled microfossils, Marshall et al. (2017) reported the application of XRF for mapping V, an element present in chlorophyll and heme porphyrin pigments. The authors propose that the co-localization of this element within microfossil-like morphologies and carbonaceous composition can be used as a biosignature for putative microfossils, and could also be applied in samples returned from Mars in the near future.
Allwood et al. (2018), in order to reassess the biogenicity of putative microbialites from 3.7 Ga Isua supracrustal belt published by Nutman et al. (2016), used the distribution of trace elements relative to minerals in the putative structures (Figure 2). In combination of other techniques to reveal the three-dimensional, morphology and orientation of the structures, the X-ray fluorescence showed the distribution of major and trace elements inside the morphology of the putative stromatolites, revealing an alternative explanation for the origin of the structures. For the authors, it is plausible a non-biological formation of the structures, consisting of a feature caused after burial process by deformations in a carbonate-altered metasediments. The authors argue that in the previous interpretation (from Nutman et al., 2016) for the trace-elements in the three-dimensional structure as evidence of primary marine carbonate sedimentation, in fact was result as a mixture of samples, including micas, not only dolomite. Once micas can carry oligoelements in these rocks along the geological history, the biogenicity of the structures become questionable. This case may illustrate the need for further analysis and other evidences to complement the use of elemental biosignatures.
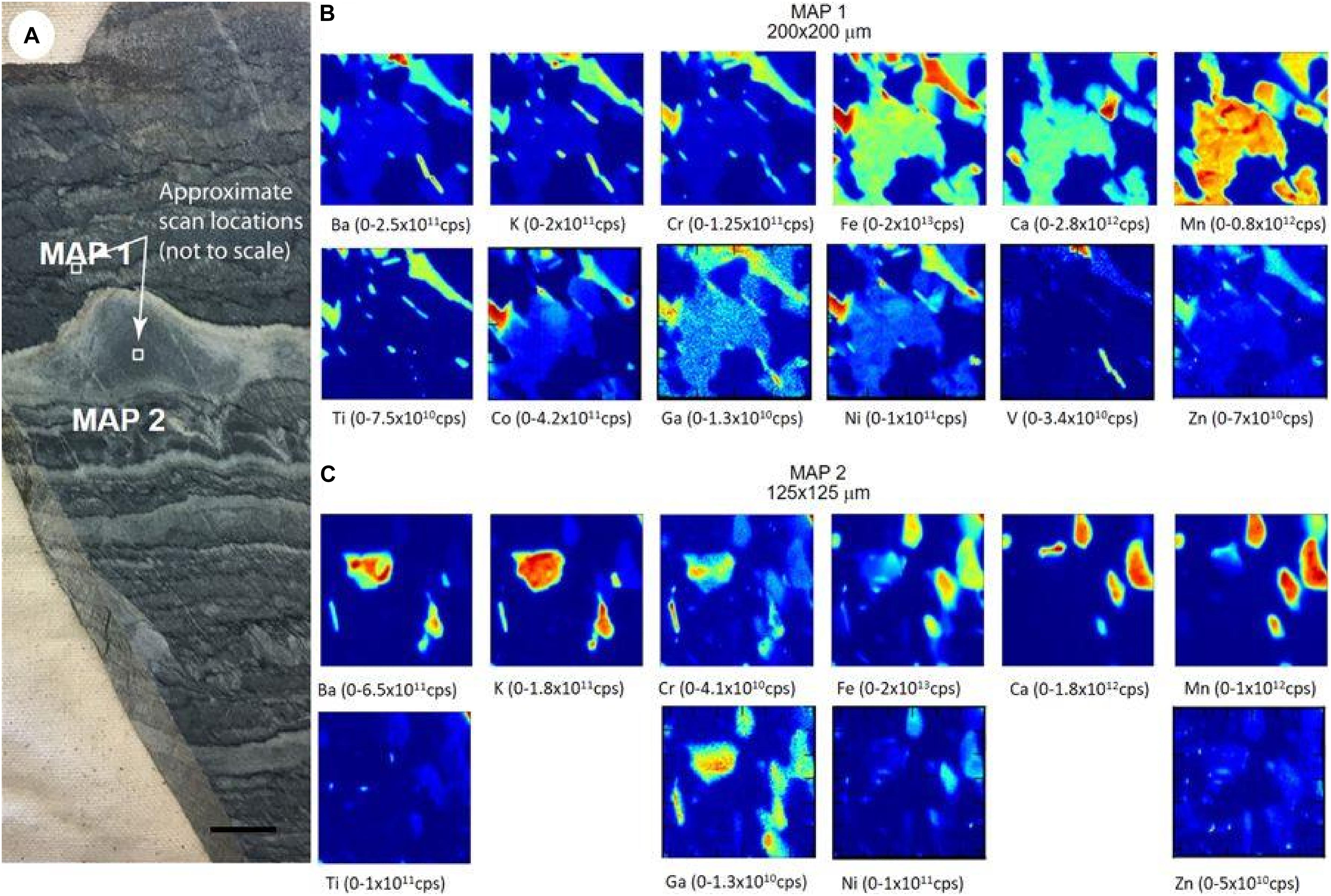
Figure 2. Distribution of trace elements in putative microbialites from 3.7 Ga Isua supracrustal belt, Greenland, originally published by Nutman et al. (2016) as putative stromatolites. The elemental maps were made with the application of synchrotron-based X-ray fluorescence, and the elemental maps makes part of the discussion of a plausible non-biological origin of the structures. (A) Photograph of the sample with the mapped areas (white squares, scale: 10 mm); (B) map of area 1; (C) map of area 2. For both maps the blue shows the highest X-ray intensity while the red shows the lowest intensity (counts per second); the maps are for K-shell X-rays except for Ba, which was detected using L-shell X-rays (Allwood et al., 2018). Reprinted by permission from Springer Nature.
Differentiating Biotic and Abiotic Elements Species
One of the main challenges in the search of chemical biosignatures associated to microfossils and microbialites is the fact that the original biochemical components of microorganisms are degraded and altered over time, while other non-biogenic elements are incorporated in the course of diagenetic processes (Lemelle et al., 2008). The metabolic activity of microorganisms, however, generates redox heterogeneities, which, sometimes in association to organic compounds, can constitute biosignatures (Miot et al., 2014). The speciation of elements provides information about oxidation state and chemical neighboring, which can provide valuable insights into microorganism-minerals interactions and ancient metabolisms.
The possibility to tune the energy of X-rays in synchrotron sources allows the application of X-ray Absorption Spectroscopy (XAS), an approach based on the analysis of the absorption profile of a given element prior to the core electrons removal with the incidence of X-rays (the same phenomenon described above for the generation of the fluorescence signal). The different regions analyzed on the absorption spectra gives rise to two different techniques, X-ray Absorption Near Edge Structure (XANES), and Extended X-ray Absorption Fine Structure (EXAFS). These techniques are strongly sensitive to the oxidation state of the elements and can also provide information about the coordination of the absorbing ions. When associated to the small beams available at scanning X-ray microscopes, speciation maps with nanometric resolution can be achieved. These techniques have already been applied at lower concentrations and at less-than-perfect sample conditions (Newville, 2014), as the ones usually present in natural geological samples.
There are several examples of the use of these techniques as a complement both in reinforcing and in questioning the biogenicity of ancient microbialites and microfossils. Lemelle et al. (2008) found biomarkers related to organic S associated to microfossils by evaluating the sulfur absorption K-edge. Grosch et al. (2017) used XANES to question the biogenicity of 3.47 Ga filament-shaped titanite microtextures in early Archean samples from Barberton Greenstone Belt, South Africa, which was considered the oldest microbial trace-fossil on Earth. The authors used temperature maps combined with μ-XANES for Fe speciation profiles in chlorites present in metabasalts that contained the filaments. The data pointed to metamorphic constraints that indicated incompatibility with the biogenicity of the structures. In contrast, De Gregorio et al. (2009) used XANES with Scanning-Transmission X-ray Microscopy (STXM) and complementary techniques in order to reinforce the biogenicity of 3.5 Ga putative microfossils from Apex Chert, Western Australia. The authors found similar characteristics on these specimens with biogenic kerogen from the ca. 1.9 Ga Gunflint Formation. In this study, XANES provided information about the chemical complexity of kerogen, which presented aromatic carbon and oxygenated functional groups. Sancho-Tomás et al. (2018) applied XANES and μ-XRF in combination with conventional analysis and showed the relationship between the biological processing of As with the mineralogy in recent hypersaline microbial mats, by evaluating the mineral occurrence, the As speciation and the elemental distribution.
With the intense photon beams of 3rd and 4th generation synchrotron sources, radiation damage of the samples should also be considered. Potentially preserved organic molecules are the most fragile ones, and can suffer from photooxidation and breakup depending on the measuring condition. However, even inorganic signatures can be altered, as X-rays can change the oxidation state of elements (e.g., photo-reduction of sulfur as reported by Moussallam et al., 2014), or produce defects on the crystal lattice of minerals. It is possible to use the advantage of the brilliant beams while minimizing exposition by performing very fast scans, both in energy (for spectroscopy) and in space (for imaging). This should be taken in consideration on the design of new beamlines that intend to be used on radiation-sensitive materials. Theoretical calculations and test measurements with standards can optimize the systems before the measurement, ir order to collect enough signal to allow the study, but delivering the minimum dose possible. Different strategies of analysis for mitigating and monitoring these effects for ancient materials have been reviewed by Bertrand et al. (2014).
It is possible to use the chemical information obtained with XAS as a contrast for an imaging approach, called STXM is a type of X-ray microscopy which uses XANES as its contrast mechanism (Ade and Urquhart, 2002). This approach works usually in the soft X-ray energy range (130–2.500 eV), and can reach nanometric scale of spatial resolution. This range of energy can interact with almost all elements, besides allow to map chemical species based on bonding structure. The use of soft X-rays also reduce the risks of damage for radiation, in comparison with the electron beam techniques (Lawrence et al., 2003). One advantage is the possibility of working with bulk samples, as long as it is transparent to the beam, and the possibility of imaging a number of key-elements of interest in the same sample. This technique has been utilized for determining the speciation of elements such as carbon and nitrogen in microfossils at submicrometric scale, such as organic microfossils from 1.88 Ga Gunflint Formation (Alleon et al., 2016), refining the knowledge about the degradation of organic biosignatures along the time, especially by the effects of temperature changes along the diagenetic processes.
Infrared techniques can also be powerful tools for studies of detection and study of the preservation or alteration of organic biosignatures. It possible to retrieve infrared spectra in absorption or emission of samples in gas, liquid or solid state, with high spectral resolutions and in a wide spectral range. Although there are several studies using conventional infrared techniques for the study of biosignatures (Guido et al., 2012; Preston et al., 2014; Gordon and Septhon, 2016; Gaboyer et al., 2017; Igisu et al., 2018; Stevens et al., 2019), there is still a lack of reference in the use of synchrotron-based FTIR (SR-FTIR) techniques for this purpose. The synchrotron-based approach has the advantage of the high flux and broad range of energy, which allows the acquisition of fast spectra with high signal-noise ratio. For biological signatures, this can mean a decrease in the risk of degradation of biosignatures during the measurement time.
An example of application of SR-FTIR on the evaluation of chemical biosignatures’ behavior during the fossilization process was presented by Benning et al. (2004), in which SR-FTIR micro-spectroscopy was applied to determine the response of the organic structure of live cyanobacterial cells. The vibration of the original components of the microbial cells (specific functional groups related to the cells), together with the characteristic vibrations for silica was analyzed during the progressive silicification. This is especially relevant, as silicification is one of the most common fossilization processes and present high capability of biosignature preservation during the geological time (Konhauser et al., 2004; Wacey et al., 2011; Campbell et al., 2015; Sugitani et al., 2015; Manning-Berg et al., 2019).
Investigating the Structure, Optical and Magnetic Properties of Biominerals
The advantage of biominerals (Dove et al., 2003; Perry et al., 2007; Dupraz et al., 2009) in their use as biosignatures is due to they are more resistant to deep-time geological processes and to several alterations that can be caused by the diagenesis. In comparison with organic biomarkers, for instance, the biominerals are more persistent in nature over the time (Jimenez-Lopez et al., 2010). Biominerals are very important for the study of past life on the planet, as they can represent life records present in rocks of very old ages. In the case of magnetofossils, it can be a good biomarker for the presence of past life on Earth and beyond (McKay et al., 1996). However, in order to be able to use them as biosignatures, it is first necessary to know the intrinsic characteristics that differ them from minerals of abiotic origin, and to compare them in order to establish biogenicity parameters. Biominerals can be differentiated from minerals of abiotic origin due to some intrinsic characteristics that they present. It is known that the biomineralization processes can influence the organization of minerals, allowing one to differentiate biogenic and abiogenic minerals regarding, for instance, their crystalline structure and physical properties (Chang and Kirschvink, 1989; Bazylinski et al., 1995; Thomas-Keprta et al., 2000; Egli, 2004). In the biologically-controlled mineralization, the genetics of the organisms/microorganisms can control intrinsically the mineral nucleation according some physiological or morphological need (Mann, 2001; Dupraz et al., 2009). This intrinsic control can originate characteristics that can be distinguishable from those inorganic minerals, such as some properties and organizations observed in internal and external skeletons (i.e., Ma et al., 2016; Rao et al., 2016) or observed in different origins of magnetites (Bazylinski et al., 1995; Thomas-Keprta et al., 2000; Körnig et al., 2014). These biominerals (or organominerals, such as suggested by Mann (2001) when the mineral is genetically controlled during its formation) can be considered direct evidence of life, consisting, when properly detected, a consistent biosignature. For instance, there are at least six specific characteristics that can distinguish the intracellular magnetite from detrital magnetite (Thomas-Keprta et al., 2000). They are: small size of the crystals to until a few dozen nanometers (single domain – SD), controlled mainly by the EPS; chemical purity and crystallographic perfection (less contaminants or exogenous elements between the atoms chains which forms the crystal lattices); organization in chains (when consists of magnetofossils or fresh cells), uncommon shapes of particles (such as bullet-shaped or elongated crystals which can not be mimicked by inorganic processes); and a trendy to elongation of the crystals when they are organized in chains toward the crystallographic direction [111].
X-Ray Diffraction (XRD) is a potential technique to be used in the study of mineral biosignatures due to its capability of identifying crystalline phases such as inorganic and organic ordered structures preserved and/or secreted by living organisms. This kind of technique is selective and can identify synthetic or natural minerals (Tadic and Epple, 2004). Even if a material presents mixed crystal structures, the characteristic peaks produced from different planes of reflection may allow the identification of specific crystalline phases. Thus, XRD is a very important tool for evaluating the biogenicity of minerals (Che et al., 2016; Iñiguez et al., 2017), allowing the detection of past life evidence in ancient rocks, fossils and even rocks from Mars in the future.
The detection limit of XRD depends on the measurement geometry, incident photon energy, spot size, and flux. Compared to conventional X-ray diffractometers, synchrotron measurements allow the study of very dilute phases with high angular resolution (2θ 10–4∘), considering the Bragg-Brentano geometry and the measurement in 2θ, which can be used to deconvolute very close peaks. This can be used to distinguish biotic and abiotic crystals, for example, magnetic compounds like greigite and magnetites produced by bacteria (Miot et al., 2014; Till et al., 2017).
Still exploring the possibility of detecting biominerals, another technique applicable to biogenicity problems of minerals, the X-ray Magnetic Circular Dichroism (XMCD), is able to give information about the orbital magnetic moment and the spin of the material. The technique provides information about the 3d electronic states in transition metals, such as Fe, Ni, Co, etc., which is responsible for the magnetic properties of the minerals (Stöhr, 1999; Rogalev et al., 2006). XMCD has been increasingly used to provide detailed information about the electronic and magnetic structure of nanoparticles (van der Laan and Figueroa, 2014), and this can be interesting for the study of biogenicity, especially for the investigation of ferromagnetic biominerals, such as biogenic magnetite and greigite. The technique is based on the dichroic effect, which occurs when left and right polarized light passes through a material showing differences in the absorption coefficients. Dichroism can be caused by the spin or by the anisotropy of the material, such as the magnetic anisotropy of certain minerals (Stöhr, 1999). That is, depending on the crystallographic direction of the mineral, the absorption of the light will be different, generating different spectra. Bacteria can produce extracellular nanoparticles of magnetite by different metabolic pathways, such as the iron oxidation under aerobic conditions. Also, another group, the magnetotactic bacteria, can originate intracellular magnetite in chains, the magnetosomes. These biominerals can be part of the fossil record or can be part of rocks with dubious origin. The XMCD technique has been shown to be a good tool in some biogenicity problems, especially regarding ferromagnetic minerals, as it can provide information about the ratio of iron species in magnetites of different origins (biogenic and inorganic), crystallinity, mineralogical structure and purity of the crystal. The crystallographic and magnetic characteristics of biogenic magnetites that allows to differentiate them from the inorganic minerals (Thomas-Keprta et al., 2000), could also be detectable with XMCD. For example, Carvallo et al. (2008), combining data from TEM analysis, used XMCD to demonstrate the high purity and crystallinity of biogenic nanomagnetite, showing that these particles contained higher amount of Fe2+ than the abiogenic nanomagnetite. The authors utilized the technique to compare the ratio of Fe2+ and Fe3+ in biogenic and inorganic magnetite nanoparticles synthesized, concluding that biogenic ones have higher crystallinity and higher amount of Fe2+ when measured in comparison with the inorganic nanoparticles. The authors concluded that the difference signal between the biogenic and abiogenic XMCD spectra is bigger than any systematic instrumental error.
Also using XMCD, Coker et al. (2007) compared magnetosome crystals with extracellular magnetite and inorganic magnetite, showing the similarity of the magnetosomes with the stoichiometric magnetite and their higher chemical purity in comparison with the other non-intracellular crystals. The works of Coker et al. (2007) and Carvallo et al. (2008) can be useful by presenting parameters to differentiate biogenic and abiogenic magnetites, taking into account the high crystallinity and high Fe2+ content in the intracellular magnetites. The exceptional reducing power of bacteria such as Shewanella putrefaciens probably explains the high concentration of Fe2+ in comparison to nanoparticles of abiotic origin.
The optical activity from organic and inorganic compounds has also been proposed as a tool for the detection and characterization of biosignatures. For example, kerogen (Marshall et al., 2017; Shkolyar et al., 2018), proteins (Dartnell and Patel, 2014; Lin et al., 2015) and minerals can be associated with the past presence of life in an environment. Gaft et al. (2015) showed several minerals in which the optical activity may be associated with rocks formed in different contexts. The optical channels in these materials, i.e., the ions and/or defects responsible for the luminescence, are described as in function of oxidation state and their optical transition (wavelength emission, time decay) when stimulated with ultraviolet (UV), visible and infrared (IR) light. X-ray Excited Optical Luminescence (XEOL) can be used for the same purpose. However, the excitation with X-rays allows the observation of all optical active channels due to their capability of exciting core levels, making the optical process dependent of the lattice relaxation. Defects may be probed and explored, as well as their characteristics such as oxidation state, origin (intrinsically or extrinsically formed) (Teixeira et al., 2014; Finch et al., 2016; Rezende et al., 2016), and the environment they were formed, for example, when a living organism has started its fossilization process or even why a precious gemstone presents determined color (Tao, 2016).
XEOL is a photon-in/photon out technique in which X-rays are used to excite core levels and getting light emitted in the range from the UV to IR (Sham, 2002). It is site-selective and can be used with variable X-ray photon energy, deeply penetrating a structure to excite its optical channels to explore their origins. XEOL combined with techniques such as XRF and XRD can be a powerful tool to describe a whole picture about composition and elements distribution in natural materials. Beamlines of 4th generations synchrotrons, such as the Carnaúba beamline of the Sirius light source (Tolentino et al., 2017), in Brazil, will have specific setups for the application of multi-technique analysis (XRF, XAS, XRD, and XEOL) of environmental samples, such as rocks and fossils. It will be possible to map optical active channels with a micro/nanosized and high resolution probe that will allow to explore the presence, for example, of an ion inside of minerals from the bones of a fossil and their characteristics (Kolodny et al., 1996; Rakovan and Reeder, 1996).
Conclusion
The complexity of attestations of biogenicity on geological and paleobiological materials makes it essential to explore multiple and complementary approaches in different length and sensitivity scales. For the micron- and nanoscales synchrotron-based techniques represent the forefront of the application of photons for the inspection of a wide range of materials, allowing complex and heterogeneous samples to be studied at an unprecedented level of detail. Synchrotron approaches are been consolidated as important tools for the deeper understanding of the records of ancient life on Earth and for the non-destructive investigation of extremely rare samples, such as meteorites and rocks that will be retrieved from Mars in the near-future sample return missions.
The recent developments in synchrotron sources also brings good perspectives for the study of biosignatures. The novel 4th generation sources, such as MAX IV in Sweden, Sirius in Brazil, and the upgraded sources ESRF-II in France, APS-U in the United States and Spring8-II in Japan are opening up new avenues for the nanoscale investigation of different types of materials. For geobiological specimens, these machines will allow the achievement of nanometric spatial resolution for resolving preserved morphological fossils and also microbial-mineral interactions with different chemical and morphological contrast information, high energy and high spectral resolution for probing, mapping and speciating heavy Z elements and high sensitivity to elements in trace concentrations. These advances will allow complex and important questions on the early chemical and morphological biosignatures to be attacked, likely consolidating synchrotron paleometry and nanopalebiology within biogeosciences and astrobiology.
Author Contributions
All authors contributed to the literature revision and manuscript writing.
Funding
FAPESP (2015/21810-6 and 2016/25681-9), CNPq (projects 152915/2018-4, 301263/2017-5, and 424367/2016-5), Capes (Finance Code 001), and Serrapilheira (project G-1709-20205) grants are responsible for the maintenance of the research team activities. The Research Unit in Astrobiology (NAP/Astrobio – PRP/USP) was acknowledged for the institutional support.
Conflict of Interest
The authors declare that the research was conducted in the absence of any commercial or financial relationships that could be construed as a potential conflict of interest.
Acknowledgments
The authors thank São Paulo Research Foundation (FAPESP grants #2015/21810-6 and #2016/25681-9), CNPq (projects 152915/2018-4, 301263/2017-5 and 424367/2016-5) and Capes (Finance Code 001) for the financial support and the Research Unit in Astrobiology (NAP/Astrobio – PRP/USP) for the institutional support. The authors also thank the Serrapilheira Project (No. G-1709-20205).
References
Ade, H., and Urquhart, S. G. (2002). “NEXAFS spectroscopy and microscopy of natural and synthetic polymers,” in Chemical Applications of Synchrotron Radiation, ed. T. K. Sham (River Edge, NJ: World Scientific Publishing), 285–355.
Alleon, J., Bernard, S., Guillou, C. L., Carbonne, J. M., Pont, S., Beyssac, O., et al. (2016). Molecular preservation of 1.88Ga Gunflint organic microfossils as a function of temperature and mineralogy. Nat. Commun. 7:11977. doi: 10.1038/ncomms11977
Allwood, A. C., Rosing, M. T., Flannery, D. T., Hurowitz, J. A., and Heirwegh, C. M. (2018). Reassessing evidence of life in 3,700-million-year-old rocks of Greenland. Nature 563, 241–244. doi: 10.1038/s41586-018-0610-4
Banfield, J. F., Moreau, J. W., Chan, C. S., Welch, S. A., and Little, B. (2001). Mineralogical biosignatures and the search for life on Mars. Astrobiology 1, 447–465. doi: 10.1089/153110701753593856
Bazylinski, D. A., Frankel, R. B., Heywood, B. R., Mann, S., King, J. W., Donaghay, P. L., et al. (1995). Controlled biomineralization of magnetite (Fe3O4) and greigite (Fe3S4) in a magnetotactic bacterium. Appl. Environ. Microbiol. 61, 3232–3239.
Benning, L. G., Phoenix, V. R., Yee, N., and Tobin, M. J. (2004). Molecular characterization of cyanobacterial silicification using synchrotron infrared micro-spectroscopy. Geochim. Cosmochim. Acta 68, 729–741. doi: 10.1016/s0016-7037(03)00489-7
Bernard, S., Benzerara, K., Beyssac, O., Brown, G. E. Jr., Grauvogel, L., Stamm, S., et al. (2009). Ultrastructural and chemical study of modern and fossil sporoderms by scanning transmission X-ray microscopy (STXM). Rev. Palaeobot. Palynol. 156, 248–261. doi: 10.1016/j.revpalbo.2008.09.002
Bertrand, L., Schoeder, S., Anglos, D., Breese, M., Janssens, K., Moini, M., et al. (2014). Mitigation strategies for radiation damage in the analysis of ancient materials. Trends Anal. Chem. 66, 128–145. doi: 10.1016/j.trac.2014.10.005
Brasier, M. D., Antcliffe, J., Saunders, M., and Wacey, D. (2015). Changing the picture of Earth’s earliest fossils (3.5– 1.9 Ga) with new approaches and new discoveries. Proc. Natl. Acad. Sci. U.S.A. 112, 4859–4864. doi: 10.1073/pnas.1405338111
Brocks, J. J., Logan, G. A., Buick, R., and Summons, R. E. (1999). Archean molecular fossils and the early rise of eukaryotes. Science 285, 1033–1036. doi: 10.1126/science.285.5430.1033
Callefo, F., Ricardi-Branco, F., Hartmann, G. A., Galante, D., Rodrigues, F., Maldanis, L., et al. (2019). Evaluating iron as a biomarker of rhythmites - an example from the last Paleozoic ice age of Gondwana. Sediment. Geol. 383, 1–15. doi: 10.1016/j.sedgeo.2019.02.002
Campbell, K. A., Lyanne, B. Y., Handley, K. M., Jordan, S., Farmer, J. D., Guido, D. M., et al. (2015). Tracing biosignature preservation of geothermally silicified microbial textures into the geological record. Astrobiology 15, 858–882. doi: 10.1089/ast.2015.1307
Carvallo, C., Sainctavit, P., Arrio, M. A., Menghy, N., Wang, Y., Nguema, G. O., et al. (2008). Biogenic vs. abiogenic magnetite nanoparticles: A XMCD study. American Mineralogist 93, 880–885. doi: 10.2138/am.2008.2713
Catling, D. C., Krissansen-Totton, J., Kiang, N. Y., Crisp, D., Robinson, T. D., DasSarma, S., et al. (2018). Exoplanet biosignatures: a framework for their assessment. Astrobiology 18, 709–738. doi: 10.1089/ast.2017.1737
Chang, S. B. R., and Kirschvink, J. L. (1989). Magnetofossils, the magnetization of sediments, and the evolution of magnetite biomineralization. Annual Review of Earth and Planetary Sciences 17, 169–195. doi: 10.1146/annurev.earth.17.1.169
Che, C., Parvez, S., and Glotch, T. D. (2016). “Spectroscopic Study of Biosignatures in Clay-Rich Sediments: Implication for Martian Astrobiological Exploration,” in Proceedings of the 47 Lunar and Planetary Science Conference, (The Woodlands, TX).
Cockell, C. S. (2014). Habitable worlds with no signs of life. Philos Trans A Math Phys Eng Sci 372, 20130082. doi: 10.1098/rsta.2013.0082
Coker, V. S., Pearce, C. I., Lang, C., van der Laan, G., Patrick, A. D., Telling, N. D., et al. (2007). Cation site occupancy of biogenic magnetite compared to polygenic ferrite spinels determined by X-ray magnetic circular dichroism. European Journal of Mineralogy 19, 707–716. doi: 10.1127/0935-1221/2007/0019-1758
Cunningham, J. A., Thomas, C. W., Bengtson, S., Kearns, S. L., Xiao, S., Marone, F., et al. (2012). Distinguishing geology from biology in the Ediacaran Doushantuo biota relaxes constraints on the timing of the origin of bilaterians. Proceedings of the Royal Society B: Biological Science 279, 2369–2376. doi: 10.1098/rspb.2011.2280
Cunningham, J. A., Vargas, K., Pengju, L., Belivanova, V., Marone, F., Martínez-Pérez, C., et al. (2015). Critical appraisal of tubular putative eumetazoans from the Ediacaran Weng’an Doushantuo biota. Proceedings of the Royal Society B: Biological Sciences 282, 20151169. doi: 10.1098/rspb.2015.1169
Dartnell, L. R., and Patel, M. R. (2014). Degradation of microbial fluorescence biosignatures by solar ultraviolet radiation on Mars. International Journal of Astrobiology 13, 112–123. doi: 10.1017/s1473550413000335
De Gregorio, B. T., Sharp, T. G., Flynn, G. J., Wirick, S., and Hervig, R. L. (2009). Biogenic origin for Earth’s oldest putative microfossils. Geology 37, 631–634. doi: 10.1130/g25683a.1
Des Marais, D. J., Allamandola, L. J., Benner, S. A., Boss, A. P., Deamer, D., Falkowski, P. G., et al. (2003). The NASA astrobiology roadmap. Astrobiology 3, 219–235. doi: 10.1089/153110703769016299
Des Marais, D. J., Harwit, M. O., Jucks, K. W., Kasting, J. F., Lin, D. N. C., Lunine, J. I., et al. (2002). Remote sensing of planetary properties and biosignatures on extrasolar terrestrial planets. Astrobiology 2, 153–181. doi: 10.1089/15311070260192246
Des Marais, D. J., and Walter, M. R. (1999). Astrobiology: exploring the origins, evolution, and distribution of life in the universe. Annu Rev Ecol Syst 30, 397–420. doi: 10.1146/annurev.ecolsys.30.1.397
Dodd, M. S., Papineau, D., Grenne, T., Slack, J. F., Rittner, M., Pirajno, F., et al. (2017). Evidence for Early Life in Earth’s Oldest Hydrothermal Vent Precipitates. Nature 543, 60–64. doi: 10.1038/nature21377
Douglas, S., Abbey, W., Mielke, R., Conrad, P., and Kanik, I. (2008). Textural and mineralogical biosignatures in an unusual microbialite from Death Valley, California. Icarus 193, 620–636. doi: 10.1016/j.icarus.2007.08.019
Dove, P. M., De Yoreo, J. J., and Weiner, S. (2003). “Biomineralization,” in Reviews in Mineralogy and Geochemistry, (Washington, DC: Mineralogical Society of America), 54.
Dupraz, C., Reid, R. P., Braissant, O., Decho, A. W., Norman, R. S., and Visscher, P. T. (2009). Processes of carbonate precipitation in modern microbial mats. Earth Sci. Rev. 96, 141–162. doi: 10.1016/j.earscirev.2008.10.005
Egli, R. (2004). Characterization of individual rock magnetic components by analysis of remanence curves, 1. unmixing natural sediments. Studia Geophysica et Geodaetica 48, 391–446. doi: 10.1023/b:sgeg.0000020839.45304.6d
Finch, A. A., Friis, H., and Maghrabi, M. (2016). Defects in sodalite-group minerals determined from X-ray-induced luminescence. Physics and Chemistry of Minerals 43, 481–491. doi: 10.1007/s00269-016-0816-7
Gaboyer, F., Milbeau, C. L., Schwender, P., Vannier, P., Beblo-Vranesevic, K., Rabbow, E., et al. (2017). Mineralization and Preservation of an extremotolerant Bacterium Isolated from an Early Mars Analog Environment. Scientific Reports 7, 8775. doi: 10.1038/s41598-017-08929-4
Gaft, M., Reisfeld, R., and Panczer, G. (2015). Modern Luminescence Spectroscopy of Minerals and Materials. Cham: Springer International Publishing.
Garcia-Ruiz, J. M., Hyde, S. T., Carnerup, A. M., Christy, A. G., Van Kranendonk, M. J., and Welham, N. J. (2003). Self assembled silica-carbonate structures and detection of ancient microfossils. Science 302, 1194–1197. doi: 10.1126/science.1090163
Golden, D. C., Ming, D. W., Schwandt, C. S., Morris, R. V., Yang, S. V., and Lofgren, G. E. (2000). An experimental study on kinetically-driven precipitation of Ca-Mg-Fe carbonates from solution: implications for the low temperature formation of carbonates in Martian meteorite ALH84001. Meteoritics and Planetary Sciences 35, 457–465. doi: 10.1111/j.1945-5100.2000.tb01428.x
Gomes, A. L. S., Becker-Kerber, B., Osés, G. L., Prado, G. M. E. M., Kerber, P. B., Barros, G. B. B., et al. (2019). Paleometry as a key tool to deal with paleobiological and astrobiological issues: some contributions and reflections on the Brazilian fossil record. International Journal of Astrobiology 19, 1–15. doi: 10.1017/s1473550418000538
Gorbushina, A. A., Krumbein, W. E., and Volkmann, M. (2002). Rock surfaces as life indicators: new ways to demonstrate life and traces on former life. Astrobiology 2, 203–213. doi: 10.1089/15311070260192273
Gordon, P. R., and Septhon, M. A. (2016). Organic Matter Detection on Mars by Pyrolysis-FTIR: An Analysis of Sensitivity and Mineral Matrix Effects. Astrobiology 16, 831–845. doi: 10.1089/ast.2016.1485
Grosch, E. G., Muñoz, M., Mathon, O., and McLoughlin, N. (2017). “Earliest microbial trace fossils in Archaean pillow lavas under scrutiny: new micro-X-ray absorption near-edge spectroscopy, metamorphic and morphological constraints,” in Earth System Evolution and Early Life: A Celebration of the Work of Martin Brasier, Vol. 448, eds A. T. Brasier, D. McIlroy, and N. McLoughlin (London: Geological Society), 57–70. doi: 10.1144/sp448.8
Guido, A., Mastandrea, A., Tosti, F., Demasi, F., Blanco, A., Elia, M. D., et al. (2012). Characterization of fossil organic matter with Fourier-Transform Infrared (FTIR) Spectroscopy: an attempt to record extraterrestrial life. Memorie della Societa Astronomica Italiana Supplement 20, 64.
Guizar-Sicairos, M., Boon, J. J., Mader, K., Diaz, A., Menzel, A., and Bunk, O. (2015). Quantitative interior x-ray nanotomography by a hybrid imaging technique. Optica 2, 259–266.
Holler, M., Diaz, A., Guizar-Sicairos, M., Karvinen, P., Färm, E., Härkönen, E., et al. (2014). X-ray ptychographic computed tomography at 16 nm isotropic 3D resolution. Scientific Reports 4, 3857. doi: 10.1038/srep03857
Igisu, M., Ueno, Y., and Takai, K. (2018). FTIR microspectroscopy of carbonaceous matter in ∼ 3.5 Ga seafloor hydrothermal deposits in the North Pole area, Western Australia. Progress in Earth and Planetary Science 5, 85.
Iñiguez, E., Mendoza-Lavaniegos, V., and Kretzschmar, T. G. (2017). Potential of hydrothermal altered rocks as potential scenario for search of biosignatures in exoplanets. Procedia Earth and Planetary Science 17, 901–904. doi: 10.1016/j.proeps.2017.01.012
Javaux, E. J. (2019). Challenges in evidencing the earliest traces of life. Nature 572, 451. doi: 10.1038/s41586-019-1436-4
Javaux, E. J., Knoll, A. H., and Walter, M. R. (2001). Morphological and ecological complexity in early eukaryotic ecosystems. Nature 412, 66–69. doi: 10.1038/35083562
Jimenez-Lopez, C., Romanek, C. S., and Bazylinski, D. A. (2010). Magnetite as a prokaryotic biomarker: A review. Journal of Geophysical Research 115, G00G03.
Kolodny, Y., Luz, B., Sander, M., and Clemens, W. A. (1996). Dinosaur bones: fossils or pseudomorphs? The pitfalls of physiology reconstruction from apatitic fossils. Palaeogeography, Palaeoclimatology, Palaeoecology 126, 161–171. doi: 10.1016/s0031-0182(96)00112-5
Konhauser, K. O., Jones, B., Phoenix, V. R., Ferris, G., and Renaut, R. W. (2004). The microbial role in hot spring silicification. AMBIO: A Journal of the Human Environment 33, 552–558. doi: 10.1579/0044-7447-33.8.552
Körnig, A., Winklhofer, M., Baumgartner, J., Gonzalez, T. P., Fratz, P., and Faivre, D. (2014). Magnetite crystal orientation in magnetosome chains. Advanced Functional Materials 24, 3926–3932. doi: 10.1002/adfm.201303737
Lawrence, J. R., Swerhone, G. D. W., Leppard, G. G., Araki, T., Zhang, X., West, M. M., et al. (2003). Scanning Transmission X-Ray, Laser Scanning, and Transmission Electron Microscopy Mapping of the Exopolymeric Matrix of Microbial Biofilms. Applied and Environmental Microbiology 69, 5543–5554. doi: 10.1128/aem.69.9.5543-5554.2003
Lemelle, L., Labrot, P., Salomé, M., Simionovici, A., Viso, M., and Westall, F. (2008). In situ imaging of organic sulfur in 700–800 My-old Neoproterozoic microfossils using X-ray spectromicroscopy at the S K-edge. Org. Geochem. 39, 188–202. doi: 10.1016/j.orggeochem.2007.10.008
Levin, G. V., and Straat, P. A. (1977). Life on Mars? The Viking labeled release experiment. Biosystems 9, 165–174. doi: 10.1016/0303-2647(77)90026-0
Lin, S., Gao, W., Tian, Z., Yang, C., Lu, L., Mergny, J. L., et al. (2015). Luminescence switch-on detection of protein tyrosine kinase-7 using a G-quadruplex-selective probe. Chemical Science 7, 4284–4290. doi: 10.1039/c5sc01320h
Lindsay, J. F., Brasier, M. D., McLoughlin, N., Green, O. R., Fogel, M., Steele, A., et al. (2005). The problem of deep carbon - an Archean paradox. Precambrian Research 143, 19–22.
Ma, S., Boughton, O., Karunaratne, A., Jin, A., Cobb, J., Hansen, U., et al. (2016). Synchrotron imaging assessment of bone quality. Clinical Reviews in Bone and Mineral Metabolism 3, 150–160. doi: 10.1007/s12018-016-9223-3
Maldanis, L., Carvalho, M., Almeida, M. R., Freitas, F. I., Andrade, J. A. F. G., Nunes, R. S., et al. (2016). Heart Fossilization Is Possible and Informs the Evolution of Cardiac Outflow Tract in Vertebrates. ELife 5, 1–12. doi: 10.7554/eLife.14698
Mann, S. (2001). Biomineralization: principles and concepts in bioinorganic materials chemistry. Oxford: Oxford University Press, 198.
Manning-Berg, A. R., Wood, R. S., Williford, K. H., Czaja, A. D., and Kah, L. C. (2019). The taphonomy of proterozoic microbial mats and implications for early diagenetic silicification. Geosciences 9, 40. doi: 10.3390/geosciences9010040
Marshall, C. P., Oltcott, M. A., Aitken, J. B., Lai, B., Vogt, S., Breuer, P., et al. (2017). Imaging of Vanadium in Microfossils: A New Potential Biosignature. Astrobiology 17, 1069–1076. doi: 10.1089/ast.2017.1709
McKay, D. S., Gibson, E. K. Jr., Thomas-Keprta, K. L., Vali, H., Romanek, C. S., Chillier, X. D. F., et al. (1996). Search for Past Life on Mars: Possible Relic Biogenic Activity in Martian Meteorite ALH84001. Science 273, 924–930. doi: 10.1126/science.273.5277.924
Meadows, V. S. (2017). Reflections on O2 as a biosignature in exoplanetary atmospheres. Astrobiology 17, 1022–1052. doi: 10.1089/ast.2016.1578
Meadows, V. S., Reinhard, C. T., Arney, G. N., Parenteau, M. N., Schwieterman, E. W., Domagal-Goldman, S. D., et al. (2018). Exoplanet biosignatures: understanding oxygen as a biosignature in the context of its environment. Astrobiology 18, 630–662. doi: 10.1089/ast.2017.1727
Miot, J., Li, J., Benzerara, K., Sougrati, M. T., Nguema, G. O., Bernard, S., et al. (2014). Formation of single domain magnetite by green rust oxidation promoted by microbial anaerobic nitrate-dependent iron oxidation. Geochimica et Cosmochimica Acta 139, 327–343. doi: 10.1016/j.gca.2014.04.047
Mojzsis, S. J., Arrhenius, G., McKeegan, K. D., Harrison, T. M., Nutman, A. P., and Friend, C. R. L. (1996). Evidence for life on Earth before 3.800 million years ago. Nature 384, 55–59. doi: 10.1038/384055a0
Moussallam, Y., Oppenheimer, C., Scaillet, B., Gaillard, F., Kyle, P. R., Peters, N., et al. (2014). Tracking the changing oxidation state of Erebus magmas, from mantle to surface, driven by magma ascent and degassing. Earth and Planetary Science Letters, Elsevier 393, 200–209. doi: 10.1016/j.epsl.2014.02.055
Newville, M. (2014). Fundamentals of XAFS. Reviews in Mineralogy and Geochemistry 78, 33–74. doi: 10.2138/rmg.2014.78.2
Noffke, N. (2015). Ancient sedimentary structures in the <3.7 Ga Gillespie Lake Member, Mars, that resemble macroscopic morphology, spatial associations, and temporal succession in terrestrial microbialites. Astrobiology 15, 169–192. doi: 10.1089/ast.2014.1218
Nutman, A. P., Bennett, V. C., Friend, C. R. L., Kranendonk, M., and Chivas, A. R. (2016). Rapid Emergence of Life Shown by Discovery of 3,700-Million-Year-Old Microbial Structures. Nature 537, 535–538. doi: 10.1038/nature19355
Ohtomo, Y., Kakegawa, T., Ishida, A., Nagase, T., and Rosing, M. T. (2014). Evidence for biogenic graphite in early Archaean Isua metasedimentary rocks. Nature Geoscience 7, 25–28. doi: 10.1038/ngeo2025
Osés, G. L., Setembrino, P., Voltani, C. G., Prado, G. M. E. M., Galante, D., Rizzutto, M. A., et al. (2017). Deciphering pyritization-kerogenization gradient for fish soft-tissue preservation. Scientific Reports 7, 1468. doi: 10.1038/s41598-017-01563-0
Perry, R. S., Mcloughlin, N., Lynne, B. Y., Sephton, M. A., Oliver, J. D., Perry, C. C., et al. (2007). Defining biominerals and organominerals: Direct and indirect indicators of life. Sedimentary Geology 201, 157–179. doi: 10.1016/j.sedgeo.2007.05.014
Preston, L. J., Melim, L., Polyak, V. J., Asmerom, Y., and Southam, G. (2014). Infrared Spectroscopic Biosignatures from Hidden Cave, New Mexico: Possible Applications for Remote Life Detection. Geomicrobiology Journal 31, 929–941. doi: 10.1080/01490451.2014.913096
Rakovan, J., and Reeder, R. J. (1996). Intracrystalline rare earth element distributions in apatite: Surface structural influences on incorporation during growth. Geochimica et Cosmochimica Acta 60, 4435–4445. doi: 10.1016/s0016-7037(96)00244-x
Rao, D. V., Gigante, D. E., Kumar, Y. M., Ceasreo, R., Bruneti, A., Schiavon, N., et al. (2016). Synchrotron-based crystal structure, associated morphology of snail and bivalve shells by X-ray diffraction. Radiation Physics and Chemistry 127, 155–164. doi: 10.1016/j.radphyschem.2016.06.024
Rezende, M. V. S., Montes, P. J. R., Andrade, A. B., Macedo, Z. S., and Valerio, M. E. G. (2016). Mechanism of X-ray excited optical luminescence (XEOL) in europium doped BaAl2O4 phosphor. Physical Chemistry Chemical Physics 18, 17646–17654. doi: 10.1039/c6cp01183g
Rodelli, D., Jovane, L., Roberts, A. P., Cypriano, J., Abreu, F., and Lins, U. (2018). Fingerprints of partial oxidation of biogenic magnetite from cultivated and natural marine magnetotactic bacteria using synchrotron radiation. Environmental Microbiology Reports 10, 337–343. doi: 10.1111/1758-2229.12644
Rogalev, A., Wilhelm, F., Jaouen, N., Goulon, J., and Kappler, J. P. (2006). “X-ray Magnetic Circular Dichroism: Historical Perspective and Recent Highlights,” in Magnetism: A Synchrotron Radiation Approach, Lecture Notes in Physics, eds E. Beaurepaire, H. Bulou, F. Scheurer, and J. P. Kappler (Heidelberg: Springer), 697.
Sancho-Tomás, M., Somogyi, A., Medjoubi, K., Bergamaschi, A., Visscher, P. T., Van Driessche, A. E. S., et al. (2018). Distribution, redox state and (bio)geochemical implications of arsenic in present day microbialites of Laguna Brava, Salar de Atacama. Chemical Geology 490, 13–21. doi: 10.1016/j.chemgeo.2018.04.029
Schopf, J. W. (1993). Microfossils of the Early Archean Apex chert: new evidence of the antiquity of life. Science 260, 640–646. doi: 10.1126/science.260.5108.640
Schopf, J. W., Kitajima, K., Spicuzza, M. J., Kudryavtsev, A. B., and Valley, J. W. (2018). SIMS Analyses of the Oldest Known Assemblage of Microfossils Document Their Taxon-Correlated Carbon Isotope Compositions. Proceedings of the National Academy of Sciences 115, 53–58. doi: 10.1073/pnas.1718063115
Schwieterman, E. W., Kiang, N. Y., Parenteau, M. N., Harman, C. E., DasSarma, S., Fisher, T. M., et al. (2018). Exoplanet Biosignatures: A Review of Remotely Detectable Signs of Life. Astrobiology 18, 663–708. doi: 10.1089/ast.2017.1729
Sforna, M. C., Daye, M., Phillippot, P., Somogyi, A., van Zuilen, M. A., Medjoubi, K., et al. (2016). Patterns of metal distribution in hypersaline microbialites during early diagenesis: Implications for the fossil record. Geobiology 15, 259–279. doi: 10.1111/gbi.12218
Sham, T.-K. (2002). Chemical Applications of Synchrotron Radiation, Vol. 12. Singapore: World Scientific.
Shkolyar, S., Eshelman, E. J., Farmer, J. D., Hamilton, D., Daly, M. G., and Youngbull, C. (2018). Detecting Kerogen as a Biosignature Using Colocated UV Time-Gated Raman and Fluorescence Spectroscopy. Astrobiology 18, 431–453. doi: 10.1089/ast.2017.1716
Steele, A., McGubbin, F. M., Fries, M., Kater, L., Boctor, N. Z., Fogel, M. L., et al. (2012). A reduced organic carbon component to Martian Basalts. Science 337, 212–215. doi: 10.1126/science.1220715
Stevens, A. H., McDonald, A., Koning, C., Riedo, A., Preston, L. J., Ehrenfreund, P., et al. (2019). Detectability of biosignatures in a low-biomass simulation of martian sediments. Scientific Reports 9, 9706. doi: 10.1038/s41598-019-46239-z
Stöhr, J. (1999). Exploring the microscopic origin of magnetic anisotropies with X-ray magnetic circular dichroism (XMCD) spectroscopy. J. Magn. Magn. Mater. 200, 470–497. doi: 10.1016/s0304-8853(99)00407-2
Sugitani, K., Mimura, K., Takeuchi, M., Yamaguchi, T., Suzuki, K., Senda, R., et al. (2015). A Paleoarchean coastal hydrothermal field inhabited by diverse microbial communities: The Strelley Pool Formation, Pilbara Craton, Western Australia. Geobiology 13, 522–545. doi: 10.1111/gbi.12150
Summons, R. E., Albrecht, P., McDonald, G., and Moldowan, J. M. (2008). Molecular biosignatures. Space Science Reviews 135, 115–132.
Tadic, D., and Epple, M. (2004). A thorough physicochemical characterisation of 14 calcium phosphate-based bone substitution materials in comparison to natural bone. Biomaterials 25, 987–994. doi: 10.1016/s0142-9612(03)00621-5
Tafforeau, P., Boistel, R., Boller, E., Bravin, A., Brunet, M., Chaimanee, Y., et al. (2006). Applications of X-Ray Synchrotron Microtomography for Non-Destructive 3D Studies of Paleontological Specimen. Applied Physics A 83, 195–202. doi: 10.1007/s00339-006-3507-2
Tao, H. (2016). “The Applications of Ultraviolet Visible Absorption Spectrum Detection Technology in Gemstone Identification,” in Proceedings of the 5th International Conference on Materials Engineering and Advanced Technologies (ICMEAT).” DEStech Transactions on Materials Science and Engineering Meet, (Quebec City).
Teixeira, V. C., Montes, P. J. R., and Valerio, M. E. G. (2014). Structural and optical characterizations of Ca2Al2SiO7: Ce3+, Mn2+ nanoparticles produced via a hybrid route. Optical Materials 36, 1580–1590. doi: 10.1002/bio.3025
Thomas-Keprta, K. L., Bazylinski, D. A., Kirschvink, J. L., Clemett, S. J., Mckay, D. S., Wentworth, S. J., et al. (2000). Elongated prismatic magnetite crystals in ALH84001 carbonate globules: Potential Martian magnetofossils. Geochimica et Cosmochimica Acta 64, 4049–4081. doi: 10.1016/s0016-7037(00)00481-6
Thomas-Keprta, K. L., Clemett, S. J., Bazylinski, D. A., Kirschvink, J. L., McKay, D. S., Wentworth, S. J., et al. (2002). Magnetofossils from ancient Mars: a robust biosignature in the martian meteorite ALH84001. Applied and Environmental Microbiology 68, 3663–3672. doi: 10.1128/aem.68.8.3663-3672.2002
Thomas-Keprta, K. L., Clemett, S. J., McKay, D. S., Gibson, E. K. Jr., and Wentworth, S. J. (2009). Origins of magnetite nanocrystals in Martian meteorite ALH84001. Geochimica et Cosmochima Acta 73, 6631–6677. doi: 10.1016/j.gca.2009.05.064
Till, J., Guyodo, Y., Lagroix, F., Morin, G., Menguy, N., and Nguema, G. O. (2017). Presumed magnetic biosignatures observed in magnetite derived from abiotic reductive alteration of nano goethite. Comptes Rendus Géoscience 349, 63–70. doi: 10.1016/j.crte.2017.02.001
Tolentino, H. C. N., Soares, M. M., Perez, C. A., Vicentin, F. C., Abdala, D. B., Galante, D., et al. (2017). Carnaúba: the coherent x-ray nanoprobe beamline for the Brazilian Synchrotron Sirius/LNLS. Journal of Physics: Conference Series 849, 012057. doi: 10.1088/1742-6596/849/1/012057
van der Laan, G., and Figueroa, A. I. (2014). X-ray magnetic dichroism – A versatile tool to study magnetism. Coord. Chem. Rev. 277-278, 95–129. doi: 10.1016/j.ccr.2014.03.018
van Zuilen, M., Lepland, A., and Arrhenius, G. (2002). Reassessing the evidence for the earliests traces of life. Nature 418, 627–630. doi: 10.1038/nature00934
Wacey, D., Kilburn, M. R., Saunders, M., Cliff, J., and Brasier, M. D. (2011). Microfossils of sulphur-metabolizing cells in 3.4-billion-year-old rocks of Western Australia. Nature Geosciences 4, 698. doi: 10.1038/ngeo1238
Wacey, D., Menon, S., Green, L., Gerstmann, D., Kong, C., Mcloughlin, N., et al. (2012). Taphonomy of very ancient microfossils from the 3400Ma Strelley Pool Formation and 1900Ma Gunflint Formation: New insights using a focused ion beam. Precambrian Research. 220–221, 234–250. doi: 10.1016/j.precamres.2012.08.005
Walker, S. I., Bains, W., Cronin, L., DasSarma, S., Danielache, S., Domagal-Goldman, S., et al. (2018). Exoplanet biosignatures: future directions. Astrobiology 18, 779–824. doi: 10.1089/ast.2017.1738
Walsh, M. M. (1992). Microfossils and possible microfossils from the Early Archean Onverwacht Group, Barberton Mountain Land, South Africa. Precambrian Research 54, 271–293. doi: 10.1016/0301-9268(92)90074-x
Westall, F. (2008). Morphological biosignatures in terrestrial and extraterrestrial materials. Space Science Reviews 135, 95–114. doi: 10.1007/978-0-387-77516-6_9
Westall, F., and Cavalazzi, B. (2011). “Biosignatures in Rocks,” in Encyclopedia of Geobiology. Encyclopedia of Earth Sciences Series, eds J. Reitner, and V. Thiel (Dordrecht: Springer).
Westall, F., Hickmann-Lewis, K., and Cavalazzi, B. (2019). “Biosignatures in deep time,” in Biosignatures for Astrobiology, Advances in Astrobiology and Biogeophysics, eds B. Cavalazzi, and F. Westall (Cham: Springer Nature Switzerland AG).
Westall, F., Steele, A., Toporski, J., Walsh, M., Allen, C., Guidry, S., et al. (2000). Polymeric substances and biofilms as biomarkers in terrestrial materials: implications for extraterrestrial samples. Journal of Geophysical Research 105, 24511–24527. doi: 10.1029/2000je001250
White, B. (1974). Microfossils from the Late Precambrian Altyn Formation of Montana. Nature 247, 452–453. doi: 10.1038/247452a0
Keywords: synchrotron, biogenicity, spectroscopy, imaging, biostructures
Citation: Callefo F, Maldanis L, Teixeira VC, Abans RAdO, Monfredini T, Rodrigues F and Galante D (2019) Evaluating Biogenicity on the Geological Record With Synchrotron-Based Techniques. Front. Microbiol. 10:2358. doi: 10.3389/fmicb.2019.02358
Received: 17 October 2018; Accepted: 27 September 2019;
Published: 11 October 2019.
Edited by:
Nancy Hinman, University of Montana, United StatesReviewed by:
Peter Croot, National University of Ireland Galway, IrelandMichael Tice, Texas A&M University, United States
Copyright © 2019 Callefo, Maldanis, Teixeira, Abans, Monfredini, Rodrigues and Galante. This is an open-access article distributed under the terms of the Creative Commons Attribution License (CC BY). The use, distribution or reproduction in other forums is permitted, provided the original author(s) and the copyright owner(s) are credited and that the original publication in this journal is cited, in accordance with accepted academic practice. No use, distribution or reproduction is permitted which does not comply with these terms.
*Correspondence: Douglas Galante, ZG91Z2xhcy5nYWxhbnRlQGxubHMuYnI=
†Deceased June 6, 2019