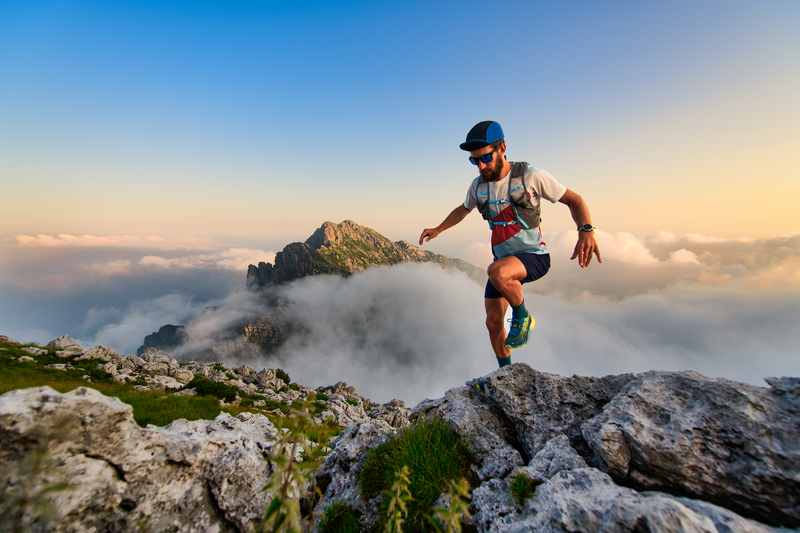
94% of researchers rate our articles as excellent or good
Learn more about the work of our research integrity team to safeguard the quality of each article we publish.
Find out more
ORIGINAL RESEARCH article
Front. Microbiol. , 15 October 2019
Sec. Microbial Immunology
Volume 10 - 2019 | https://doi.org/10.3389/fmicb.2019.02320
This article is part of the Research Topic Harnessing Innate Trained Immunity To Eliminate Persistent, Drug-Resistant And Subsequent Infections View all 7 articles
Trained immunity is the enhanced response of the innate immune system to a secondary infection after an initial encounter with a microorganism. This non-specific response to reinfection is a primitive form of adaptation that has been shown to be conserved from plants to mammals. Insects lack an acquired immune component and rely solely on an innate one, and they have expanded it upon traits of plasticity and adaptation against pathogens in the form of immune priming. The recent discoveries of the role of Saccharomyces cerevisiae in the insect’s ecology and the ability of this yeast to induce trained immunity in different organisms suggest that insects could have developed mechanisms of adaptation and immune enhancing. Here, we report that two yeast strains of S. cerevisiae, previously shown to induce trained immunity in mammals, enhance resistance to Escherichia coli infection in the paper wasp Polistes dominula. The reduction of injected E. coli load by S. cerevisiae strains was statistically significant in future foundresses but not in workers, and this occurs before and after hibernation. We thus investigated if the effect on E. coli was mirrored by variation in the gut microbiota composition. Foundresses, showing immune enhancing, had statistically significant changes in composition and diversity of gut bacterial communities but not in the fungal communities. Our results demonstrate that S. cerevisiae can prime insect responses against bacterial infections, providing an advantage to future foundress wasps to carry these microorganisms. Understanding the mechanisms involved in the generation of specific and long-lasting immune response against pathogenic infections in insects and the influence of the induction of trained immunity on the commensal gut microbiota could have a major impact on modern immunology.
Immunological memory is an important evolutionary trait that improves host survival upon secondary infection (Netea et al., 2011, 2019). Trained immunity is a newly discovered mechanism of innate immune memory, causing metabolic and epigenetic reprograming of innate immune cells [monocytes/macrophages, natural killer (NK) cells] (Netea et al., 2011, 2019). This non-specific response describes an inflammatory protection against a microorganism upon a second encounter, independently from adaptive immunity, and it has been shown to be present not only in mammals but also in plants (Durrant and Dong, 2004) and insects (Pham et al., 2007; Rodrigues et al., 2010; Norouzitallab et al., 2016). This discovery represents a paradigm change in the biology of immunity with respect to the rigorous division between innate and adaptive immune responses.
Viruses, parasites, and many bacterial or fungal cells or components of their cell wall [lipopolysaccharide (LPS), β-glucan, and chitin] represent strong stimuli of innate immune memory (Mulder et al., 2019). In mice models deficient for functional T and B lymphocytes, β-glucans from fungal cell walls induce trained immunity against non-pathogenic Candida albicans via functional reprograming of monocytes, through histone methylation, leading to enhanced cytokine production in vivo and in vitro (Quintin et al., 2012).
Recent experiments from our group showed that human monocytes stimulated by chitin from Saccharomyces cerevisiae lead to enhanced ability to eliminate a wide range of microorganisms, such as C. albicans (an opportunistic fungus), Staphylococcus aureus (Gram-positive bacterium), or Escherichia coli (Gram-negative bacterium) (Rizzetto et al., 2016).
The study of the occurrence of trained immunity in different organisms can be a leading approach to unravel the robustness and plasticity of this phenomenon. An insect model of immune memory is important for a deeper understanding of host defense and thus identifying the most effective approaches to modulate it. Insects evolved a complex innate immune system allowing the rapid elimination of unwanted microorganisms (Rosengaus et al., 1999). Defensive mechanisms against pathogens are mainly related to the activity of phagocytic cells present in the hemolymph and to the production of antimicrobial peptides (Gillespie et al., 1997; Lehrer and Ganz, 1999). Indeed, the activation of innate immunity in vertebrates and invertebrates showed shared mechanisms in the defense against microbial pathogens (Hoffmann et al., 1999), such as the observed activation of nuclear factor (NF)-κB family in Toll-like receptor (TLR) signaling pathways in Drosophila melanogaster after fungal interaction (Silverman and Maniatis, 2001). Although lacking elaborate immune-specific reactions based on the “memory” of previously encountered pathogens, as in vertebrates, insects can show an increased resistance upon a secondary exposure to a microorganism (Little and Kraaijeveld, 2004; Sadd and Schmid-Hempel, 2006).
To date, the plasticity of the immune response in invertebrates has been mainly associated with a species-specific response against the pathogen responsible for the primary infection (immune priming) (Milutinović and Kurtz, 2016; Cooper and Eleftherianos, 2017). This immunization can last for days or weeks after the primary infection (Sadd and Schmid-Hempel, 2006) and can be vertically transferred across generation (Sadd et al., 2005; Cooper and Eleftherianos, 2017; Bordoni et al., 2018). Recently, it has been shown that immune priming in D. melanogaster is related to the activation of the phagocytes acting as effectors of the response against E. coli (Elrod-erickson et al., 2000). This finding suggests that immune priming determines an increase of immune activity with a reduced probability of reinfection through an improved clearance of pathogens. However, many aspects related to trained immunity in invertebrates remain unclear.
Recent reports have shown that the yeast S. cerevisiae exploits social wasps as vectors and natural reservoir for winter survival and summer dispersal (Stefanini et al., 2012). Polistes dominula paper wasps, in particular, preserve yeasts in their guts and presumably spread them in the environment but also favor hybridization in their intestinal environment, allowing the formation of genetically recombined strains of S. cerevisiae, and other yeasts (Stefanini et al., 2016). It is known that gut microbial communities locally influence immune response (Cerf-Bensussan and Gaboriau-Routhiau, 2010; Arnolds and Lozupone, 2016). Less clear is if the yeasts determine physiological responses in the insects, also through modulation of gut microbiota.
According to present knowledge (Stefanini et al., 2012), S. cerevisiae is a natural component of paper wasps’ gut microbiota, and it plays a crucial role in the insects’ ecology. The occurrence of a non-pathogenic microorganism in the gut could have the potential to alter the immunocompetence and gut microbial balance of its host. Here, we investigated if two strains of S. cerevisiae, previously demonstrated able to induce trained immunity in mammals (Rizzetto et al., 2016), elicit an immunization in P. dominula against bacterial infections, and promote gut microbiota modulation. These findings allow us to elucidate the complex relationships between host immunocompetence and its microbiota in the context of wasp natural history.
Wasps of the species P. dominula were sampled from the “insect open lab,” an experimental wasp orchard grass located in a field at the university campus in Florence (Sesto Fiorentino, Central Italy, 43°50′7″N, 11°11′46″E). Two different wasp castes, workers (collected before sexual emergence and at the beginning of summer) and future foundresses (female wasps that had not yet found a nest), were considered for immunological trials. The foundresses were tested before and after the winter diapause, a life condition in which different hormonal balance and fluctuation of insect immune system occur (Kubrak et al., 2014).
After sampling, the wasps were pooled and maintained in cohousing under controlled conditions (according to environmental photoperiod and room temperature) in sterile plastic cages and fed with autoclaved water and 40% D-glucose solution, referred to as sugar solution (SS) ad libitum for 7 days. After this period, each wasp was randomly assigned to yeast treatment (SS plus yeast) or to a control group (only SS), split, and kept separately in sterile 35-mm Petri dishes for the entire trial period, in order to avoid trophallaxis or any wasp–wasp interactive behavior.
Two Saccharomyces cerevisiae strains (YP4 and YH1) belonging to a clinical yeast collection of isolates from human fecal samples (Rizzetto et al., 2016; Ramazzotti et al., 2019) were tested as enhancers of resistance to bacterial infection in paper wasp P. dominula.
The two strains were previously proven for their ability to induce training immunity in human cells and mice (Rizzetto et al., 2016) and for different T-polarizing cytokine production in humans (Ramazzotti et al., 2019). These strains also differ in genetic background, as observed by previous analysis of S. cerevisiae population structure (Ramazzotti et al., 2019). YP4 strain belongs to the “Human Gut 2” cluster (referred to as HG2), which includes strains isolated from wasps and human intestine, with a common ancestral lineage, whereas YH1 belongs to the HG3 cluster, which includes wasps and human gut strains and other strains isolated from bakery, wine, and other fermentations, descending from a common ancestor, previously identified as West Africa (Liti et al., 2009).
Table 1 summarizes the different genetic, phenotypic, and immunophenotypic characteristics of the two tested strains, as reported by our previous studies (Rizzetto et al., 2016; Ramazzotti et al., 2019).
Table 1. Summary of genetic, phenotypic, and immunomodulatory traits of the two tested Saccharomyces cerevisiae strains.
The Saccharomyces cerevisiae strains were grown on yeast peptone dextrose (YPD) broth medium (2% yeast extract, 1% peptone, and 2% D-glucose) at 30°C. Yeast cells were counted with a Bürker chamber to determine the title and washed using sterile phosphate-buffered saline (PBS) solution and then were resuspended in SS and orally administered with sterile tips at a concentration of 108 cells per wasp (Stefanini et al., 2016).
For bacterial infection, non-pathogenic E. coli, an immune elicitor commonly used to test immune competence in insects (Cappa et al., 2015; Cini et al., 2018), was used. E. coli XL-1 Blue, tetracycline resistant {chromosomal genotype: mutant alleles: recA1 endA1 gyrA96 thi-1 hsdR17 supE44 relA1 lac [F’ proAB lacIqZΔM15 Tn10 (Tetr)]}, was grown on Luria–Bertani (LB) medium (1% tryptone, 0.5% yeast extract, and 1% NaCl), added with tetracycline 10 μg/ml in order to exclude any other microbial contaminants. This microorganism was selected because it is not naturally found in P. dominula; thus, we can exclude its presence in our wasps prior to infection.
In order to observe the effect of administration of S. cerevisiae strain on bacterial clearance in an insect model, the first group of autumn foundresses (N = 40 pre-hibernation foundresses) was preliminary tested. The wasps were divided into two subgroups: 20 foundresses fed with SS to which yeast strain YP4 was added, while the remaining 20 wasps were fed with SS without any yeast added, as a control group (Supplementary Figure S1).
A second experiment (Figure 1) was conducted with wasps of different castes and in different seasons. A group of autumn foundresses (pre-hibernation foundresses) was divided into three subgroups (N = 62): 19 foundresses were fed with SS added with YP4 strain, 20 with SS and YH1 strain, and the remaining 23 with SS without any yeast added (control group). A group of spring foundresses (N = 84) was collected after the winter diapause and similarly divided into three subgroups: 31 were fed with SS added with YP4 strain, 33 with SS and YH1 strain, and 20 with SS only.
Figure 1. Schematic representation of experimental workflow. After environmental sampling and cohousing for 7 days, Saccharomyces cerevisiae strains (YP4 and YH1) were administered in foundresses of Polistes dominula wasps collected in different seasons (autumn and spring) and life stages (pre- and post-diapause) and workers collected in summer. At the 11th day, bacterial clearance was compared among treated and control groups. In the pre-diapause foundresses collected in autumn (in blue), gut microbiota analysis was also performed. SS, sterile sugar solution (40% D-glucose). Details were reported in the section “Materials and Methods.”
Workers (n = 53) were collected in summer from 10 colonies (at least five workers per colony) and treated as follows: 18 workers were fed with SS and YP4 strain, 19 workers with SS and YH1 strain, and 16 workers with SS without yeast (control group).
After feeding, foundresses were maintained at 8°C in the dark and fed ad libitum with sterile SS for 10 days (Stefanini et al., 2016). Workers were maintained in similar conditions to foundresses except for temperature, which was kept around 20°C, according to the seasonal temperature in which the workers emerge in the colony. After 11 days of feeding, bacterial infection with E. coli was performed. Furthermore, in order to exclude the presence of naturally occurring tetracycline-resistant microorganisms, five wasps were randomly taken from the same collection groups. Their whole body, gut included, was dissected and plated on LB agar, added with tetracycline 10 μg/ml, and incubated for 72 h at 37°C.
Escherichia coli cells were grown aerobically in LB medium plus tetracycline 10 μg/ml at 37°C. Cells were counted on a Bürker chamber, washed using PBS solution, and resuspended in PBS. Then, E. coli (105 cells) was injected in each wasp with a HamiltonTM micro syringe through the intersegmental membrane between the second and third abdominal tergites (Cini et al., 2018).
After injection, wasps were maintained in the dark at room temperature (20°C) for 24 h. The dead wasps were removed. Each wasp was then dissected under sterile conditions and homogenized in 1 ml of PBS solution using a sterile pestle. Before homogenization, the gut was collected for subsequent metagenomic analysis while the sting and the venom sac were removed in order to avoid possible reduction of the bacterial count due to the presence of antimicrobial peptides in the wasp venom. The homogenate was serially diluted and plated on LB solid medium plus tetracycline 10 μg/ml and then incubated at 37°C overnight.
The day after infection (12th day), bacterial clearance was evaluated by counting bacterial colony-forming units (CFUs) per milliliter per wasp. We performed the bacterial clearance test as a good proxy of insect immunity, since injection of live bacteria provides an integrative view of the activation of the organismal immune system (Charles and Killian, 2015). Bacterial clearance was compared among wasps belonging to different castes (foundresses and workers), treatments (control and YP4 and YH1 strains), and seasons for foundresses (autumn and spring), by using generalized linear mixed models (GLMMs) where the experiments (three for foundresses and one for workers) were included as a random effect. The effect of fixed factors and their interaction have been tested, obtaining a type III analysis of variance table, by using the Anova function of the car R package (Fox and Weisberg, 2019). Moreover, the effect of individual yeast strains compared to the control has been also assessed by using the summary function of the stat R package. The generalized linear models (GLMs) have been carried out, grouped and separated for the two experiments as well as for the two castes (foundresses and workers).
The wasp guts were collected and stored in RNAlater Stabilization Solution (Invitrogen, Thermo Fisher Scientific) in sterile microcentrifuge tubes at −20°C until DNA extraction. DNA extraction from gut samples was performed using DNeasy PowerLyzer PowerSoil Kit (QIAGEN) following the manufacturer’s protocol. The total DNA was quantified by a Tecan quantification device (Life Sciences). The sequencing was carried out by an Illumina MiSeq platform (BMR Genomics sequencing service of the University of Padova, Italy1). DNA sequencing on bacterial communities was performed on the V3–V4 region of the 16S ribosomal RNA (rRNA) genes by using the primers Pro341F (5′-CCTACGGGNBGCASCAG-3′) and Pro805R (5′-GACTACNVGGGTATCTAATCC-3′) (Takahashi et al., 2014). The same guidelines for fungal communities were carried out on internal transcribed spacer (ITS) 2 rRNA genes by using the primers ITS3 (5′-GCATCGATGAAGAACGCAGC-3′) and ITS4 (5′-TCCTCCGCTTATTGATATGC-3′) (White et al., 1990). Illumina sequencing reads are available at the European Nucleotide Archive2 under accession study PRJEB32390.
Demultiplexed sample libraries were visually inspected with the FastQC program (Andrews, 2010); low-quality ends of forward and reverse reads were trimmed using Sickle (Joshi and Fass, 2011) with a quality cutoff of 20 and a length threshold after trimming of 200. MICCA pipeline v. 1.7.2 (Albanese et al., 2015) was used for operational taxonomic unit (OTU)/sequence variant (SV) picking as follows: forward and reverse reads were joined with micca mergepairs command, and reads with N bases were discarded with command micca filter. OTU/SV picking and chimera checking were performed with the miccaotu command and the UNOISE3 protocol as a picking algorithm. Taxonomy was assigned to the representative sequences of the identified OTUs/SVs classified using the RDP classifier v. 2.11 (Wang et al., 2007).
Subsequent analyses were performed in R (v.3.42; R Core Team, 2018), employing package phyloseq v.1.22.3 (McMurdie and Holmes, 2013) to import data [as biological observation matrix (BIOM) files], to perform PCoA ordination analysis (using the Bray–Curtis distance measure), and to plot microbiota composition as a bar plot. Alpha diversity indices were calculated with the microbiome package (Lahti et al., 2017), and overall differences were tested with ANOVA, while species accumulation curves were calculated with the ranacapa package (Kandlikar et al., 2018). Prior to any analysis, count data were scaled with CSS transform as implemented in the metagenomeSeq (Paulson et al., 2013). Heatmaps reporting OTU distribution in different samples were created with the pheatmap package (Kolde, 2012). For heatmap construction, rows (i.e., OTUs/SVs) were ordered based on the results of the plot_heatmap command in the phyloseq package, using PCoA as the ordination method and Bray–Curtis as the distance measure, while columns (i.e., samples) were ordered based on Euclidean distance (as implemented in the pheatmap package). Color maps of OTU/SV abundance were scaled on the rows. Association between gut microbial community diversity and bacterial CFUs found in the hemocele post infection (a proxy for immune response priming and activation) were evaluated with distance-based permutational multivariate analysis of variance (PERMANOVA; Bray–Curtis distance; 9,999 permutations) using the adonis function in the vegan v.2.5-2 (Oksanen et al., 2018) package, as well as employing multivariate-structure-based GLMs (negative binomial), as implemented by the manyglm command in the package mvabund (Wang et al., 2012). STAMP (Parks et al., 2014) software was used to compare relative abundance of OTUs at different taxonomic levels and to create plots. We used Welch’s t test (with no correction for multiple comparison) and displayed only OTUs with a p < 0.05.
In order to test the ability of S. cerevisiae, a generally recognized as safe (GRAS) yeast, to enhance resistance to bacterial infection, we selected two S. cerevisiae strains based on their previously observed ability to induce training immunity in human cells and mice (Rizzetto et al., 2016).
In a first experiment, bacterial clearance was preliminarily tested on one group of autumn foundresses after administration of the YP4 yeast strain (Supplementary Figure S1). Subsequently, an immune trial was performed on two different wasp castes (future foundresses and workers) in three different seasons (summer workers and autumn and winter foundresses, see section “Materials and Methods”). The experimental design is depicted in Figure 1. By immune trials performed on foundress wasps, we observed that the bacterial clearance was lower in the control group than in the treated groups (Figure 2, Supplementary Datasheet S2, and Supplementary Figure S4). Firstly, data of the bacterial clearance from the two different experiments (including the preliminary test with the sole YP4 strain administration, as reported in Supplementary Figure S1) were analyzed separately, showing a statistically significant effect of yeast treatment on the reduction of E. coli load (Table 2). Then, we have extended the analysis to all groups by integrating all variables (treatments, caste state, and season) into a GLMM (Table 3; see section “Materials and Methods”). The GLMM showed that the treatment with S. cerevisiae decreased the infection burden (measured as E. coli CFUs per milliliter as reported in Table 3 and shown in Figure 2). The treatment effect was significant in the reduction of E. coli load regardless the two different trials, also when the foundresses from the preliminary experiment were added in an overall analysis (Table 3).
Figure 2. Bacterial clearance in P. dominula in foundresses and workers after yeast administration. Mean and standard deviation of residual load of Escherichia coli colony-forming units (CFUs) per milliliter in control (C) and treated groups (YH1 strain and YP4 strain administration) in foundresses and workers, at 24 h after bacterial infection. Data are representative of the bacterial clearance measure, reported as z-score transformation (residual z-score of CFUs per milliliter).
The experiments and analyses have been repeated for foundresses and workers separately (Table 3), revealing a caste-dependent effect, statistically significant in foundresses but not in workers (Figure 2). Furthermore, we showed a significant interaction effect for the sole strain YH1 (p = 0.019, Table 3) that increased bacterial clearance in foundresses but decreased resistance to the infection in workers (higher CFU-per-milliliter counts; Figure 2).
The experiments on the foundresses were performed in autumn (entry into diapause) and spring (exit to the diapause). We found that the levels of CFUs per milliliter were significantly higher in the spring than in the autumn (p < 0.001; Table 3). However, the treatment affects the CFU-per-milliliter levels regardless of the season, as shown by the lack of significance of the interaction effect in Table 3 (p = 0.785 for YH1 ∗ season and p = 0.251 for YP4 ∗ season).
In order to evaluate the effect of S. cerevisiae administration on the gut microbial communities, either via direct interaction or as a consequence of the immune training, we performed characterization of gut microbiota on the autumn foundress group, in which the effect of yeast administration on bacterial clearance was more evident. Autumn was also preferred because it is the season with a higher isolation rate of S. cerevisiae strains from wasp guts (Stefanini et al., 2012).
We selected nine gut samples of foundresses per group (treatments and control), pooling them together in a total of three gut samples per group, as representative gut microbiota collection of all tested samples of the respective groups (Supplementary Figure S2). The criteria for sample selection were in accordance with the coefficient-of-variation (CV) analysis calculated by comparison of intragroup and intergroup (intragroup CV%: control group = 15.4; YP4-treated group = 38.2; YH1-treated group = 36.2; intergroup CV%: control vs. YP4 = 46.9; control vs. YH1 = 48; as reported in Supplementary Table S1).
By 16S rRNA V3–V4 region sequencing, a total of 214,089 sequence reads were obtained after quality filtering, clustering in a total of 495 OTUs. The number of 16S rRNA sequences per sample ranged from 1,607 to 51,340. Although the control group showed a low amount of sequence reads, no statistical differences in read number were observed among groups (Supplementary Figure S5).
By comparison of the alpha diversity indices among the three groups (control and YH1- and YP4-treated groups), we observed a reduction in richness (Figure 3A) and diversity (i.e., Shannon and inverse Simpson indices; Figures 3B,C) of gut bacterial communities in the control group, with respect to the two treated groups (Figure 3; ANOVA test; richness: F = 16.91, p = 0.0034; Shannon’s index: F = 17.6, p = 0.0031; inverse Simpson index: F = 18.32, p = 0.0027; a pairwise t test is reported for every comparison in Figure 3). Moreover, as indicated by a higher evenness index, the microbial community of the control group had more homogeneous abundance distribution than the treated groups (Figure 3D).
Figure 3. Alpha and beta diversity of gut microbial communities. (A–D) Alpha diversity measures: (A) richness, (B) Shannon diversity index, (C) Simpson index, and (D) evenness of control and treated (YH1 and YP4) groups. Statistically significant comparisons by t test (∗p < 0.05, ∗∗p < 0.01, and ns = not statistically significant). (E) Principal coordinate analysis (PCoA) ordination based on the Bray–Curtis distance. (F) Heatmap reported the frequency of operational taxonomic unit (OTU) abundance within treatment and control groups.
PCoA ordination analysis based on Bray–Curtis distances showed a clear separation of gut samples, along the first ordination axis (explaining 35.1% of the variability), according to the type of treatment (Figure 3E). In particular, samples from the YH1-treated group showed a clear separation and a more defined grouping than did the control and YP4 groups (Figure 3E). PERMANOVA was used to test the different distributions of samples, as observed in ordination analysis (Supplementary Table S2). Statistical analysis showed that S. cerevisiae treatment has an effect, as a whole, on microbiota composition, when compared to the control group (control vs. treatment: R2 = 0.3, p = 0.01), and the treatments with the two S. cerevisiae strains differentially affect the microbiota composition (control vs. YH1 treatment vs. YP4 treatment: R2 = 0.4, p = 0.03). In Figure 3F, the heatmap displays the OTU frequencies and abundance distribution among control and treated samples. We observed different trends of OTU pattern distribution between control and treated groups (separated branches in hierarchical clustering as reported in Figure 3F), as well as between the two different yeast-treated groups.
Generalized linear models analysis (mvabund R package) was performed in order to assess the multivariate association between residual CFUs per milliliter (following the bacterial clearance) and gut bacterial community composition, showing significant association (likelihood ratio statistic: 857.4, p = 0.002). We found 75 OTUs (Supplementary Datasheet S1) with a significant univariate association to (log)CFUs per milliliter of bacterial infectious agent, regardless of treatments or control. When correction for multiple comparison was applied, Campylobacter was still significantly associated to the count of infectious agent (adjusted p = 0.013; Supplementary Datasheet S1).
To evaluate the overall effect of S. cerevisiae administration and the subsequent resistance to E. coli infection on the wasp gut microbiota composition, we compared the microbial community’s relative abundance (at phylum and genus levels) among treated and control groups, as shown in Figure 4 and Supplementary Figure S3. At the phylum level, an increase of Bacteroidetes and Fusobacteria was observed in treated groups (Figure 4A). At the genus level, 17 genera showed significant different abundances between control and treatment groups (Figure 4B; Welch’s t test). Among them, Staphylococcus (Firmicutes phylum) and Morganella (Proteobacteria phylum) were significantly enriched in the control group. Interestingly, Escherichia/Shigella genus was significantly enriched in the gut community of the controls compared to the treated groups (Figure 4C; ANOVA p = 0.025).
Figure 4. Comparison of statistically significant taxa in treated and control groups. Mean proportion and confidence intervals (%) in control vs. treated (YH1 and YP4) groups explored at phylum (A) and genus (B) levels (p-values by Welch’s t test). (C) Relative abundance (%) of Escherichia/Shigella genus distribution between control and treated groups (ANOVA p = 0.025).
In treated groups, Prevotella (Bacteroidetes), Veillonella (Firmicutes), Capnocytophaga (Bacteroidetes), Fusobacterium (Fusobacteria), Haemophilus (Proteobacteria), and Leptotrichia (Fusobacteria) were found to be significantly abundant (Figure 4B; Welch’s t test).
The two yeast strains highly differed in their ability to change the microbial profiles (Figure 5). While administration of both S. cerevisiae strains reduced the levels of Staphylococcus and Morganella, when compared to the control group (Figures 5A,B), the single comparison between strain administration and control (YH1 vs. control in Figure 5A and YP4 vs. control in Figure 5B) showed a strain-dependent effect on gut microbiota composition. To note, YH1 treatment (Figure 5A) was associated to an enrichment of a higher number of bacterial genera, such as Fusobacterium, Veillonella, Alloprevotella, Capnocytophaga, Porphyromonas, and Campylobacter, compared to YP4 treatment (Figure 5B; Welch’s t test).
Figure 5. Comparison of statistically significant taxa in YH1- and YP4-treated and control groups. Mean proportion and confidence intervals (%) in (A) control vs. treated YH1 and (B) control vs. YP4, explored at genus level (p-values by Welch’s t test).
For the fungal ITS2 region sequencing, we obtained a total of 14,646 sequences after quality filtering, clustering in a total of 63 OTUs. The number of sequence reads per sample ranged from 202 to 3,024. Contrary to the gut bacterial community, the mycobiota composition did not show significant differences in richness and biodiversity between control and treated groups, as observed by alpha diversity and PCoA ordination analysis based on the Bray–Curtis distance (Figure 6). Accordingly, PERMANOVA analysis used to test the differences in fungal community composition among groups showed no effect of treatment on gut mycobiota (Supplementary Table S3). In Figure 7A, we reported an overview of relative abundances of fungal taxa (>1%) annotated at the species level in the three groups. No significant association between fungal community composition and bacterial infection load (CFUs per milliliter of E. coli) have been observed. The fungal community abundances (Figure 7A) appeared unaffected by the S. cerevisiae administration and by bacterial infection in hemocele. Only Metschnikowia sp. was found significantly enriched in the control group compared to both treated groups (Figures 7B,C; ANOVA p < 0.001).
Figure 6. Alpha and beta diversity of gut mycobiota. (A–D) Comparison of alpha diversity measures: (A) richness, (B) Shannon diversity index, (C) Simpson index, and (D) evenness among treated (YH1 and YP4) and control groups. Statistically significant comparisons by t test (∗p < 0.05, ∗∗p < 0.01, and ns = not statistically significant). (E) Principal coordinate analysis (PCoA) ordination based on the Bray–Curtis distance among fungal communities between control and treated groups.
Figure 7. Gut mycobiota community abundance. (A) Comparison of gut mycobiota composition in treated and control groups. Bar plot showed the taxa with >1% of relative abundance annotated at species level. (B) Focus on Saccharomycetes fungal class composition reporting species taxa with >1% of relative abundance. (C) Relative abundance (%) of Metschnikowia sp. distribution between control and treated groups.
In recent years, the physiological and ecological relationships between hymenopterans, in particular social wasps, and the yeast S. cerevisiae have become evident (Stefanini, 2018). The wasps likely act as natural vectors, hosting and transporting S. cerevisiae between different environmental niches and favoring mating among strains (Stefanini et al., 2012, 2016; Ramazzotti et al., 2019). While the advantage provided to the yeast is well understood, the benefit for the wasp to carry S. cerevisiae remains unclear. In this study, we tested the hypothesis that S. cerevisiae could help the insect to resist infections during hibernation. This hypothesis is supported by previous studies (Quintin et al., 2012; Rizzetto et al., 2016; Rusek et al., 2018; Mulder et al., 2019) showing that fungal cells or components of cell wall (i.e., chitin) are potent stimuli of innate immune memory in mammals. To this aim, we investigated whether two well-characterized S. cerevisiae strains, by genetic, phenotypic, and immunophenotypic assays (Ramazzotti et al., 2019), previously proved to induce trained immunity in mammals Rizzetto et al. (2016), are also able to enhance resistance of the invertebrate immune system against bacterial infection and shape the gut microbial communities. A few studies have focused on the nature of the insect immune system, revealing that it can be efficiently primed upon exposure to microorganisms, acquiring a protective effect upon subsequent challenge with the same, and/or different microbes (Cooper and Eleftherianos, 2017).
We used the social wasp P. dominula as an animal model for innate immune trials. They evolved mechanisms to tolerate and benefit from the presence of this GRAS microorganism, providing protection against pathogens and maintaining health status, through the development and boosting of the immune system and providing protection against pathogens. Our results showed that S. cerevisiae induces in foundresses, but not in workers, a higher response against bacterial infection compared to the controls, possibly via the activation of strain-dependent innate immunization. In fact, following YH1 administration when compared to YP4 strain, we observed significant differences in immune enhancing, as well as in the changes of the gut microbiota.
The data presented here, alongside the immune training induced by the same yeast strains in mouse models and human monocytes (Rizzetto et al., 2016), show that the yeast strains can provide protective mechanisms shared between different subphyla, potentially through analog immune training mechanisms occurring in mammals, and insects. The yeast-mediated protection against pathogens could be important to protect hibernating foundresses during overwintering, simultaneously providing yeasts with the environmental niche where they mate and survive, thus suggesting the existence of a complex symbiotic interaction. Complex communication systems have been evolved between the host immune system and the intestinal microbiota (Belkaid and Hand, 2014). Evidence is mounting in support of a dominant and decisive role of gut in shaping and modulating immune responses in the prevention of disease (Hand, 2016). However, the effect of trained immunity on commensal microbial communities, that is, the microbiota, has not been shown yet. The gut microbiota plays a fundamental role in the education and functional tuning of the host immune system, thereby acting as adjuvant to the host immune system and continually driving the nature of immune responses, providing protection against pathogens. In turn, the host immune system has evolved multiple means by which to maintain its symbiotic relationship with the microbiota (Dethlefsen et al., 2007; Cho and Blaser, 2012; Lynch and Pedersen, 2016; Gupta et al., 2018).
Hymenoptera’s gut contains a relatively simplified microbiota, with lesser microbial species as compared to that of mammals, yet social insects harbor microbial communities with highly specialized and beneficial functions in nutrition, protection from parasites and pathogens, and modulation of immune responses (Engel and Moran, 2013).
In both yeast-fed groups, we also observed reduction in potential pathogenic bacterial genera, such as Staphylococcus, Morganella, and Escherichia/Shigella, compared to the control group. The observed reduction of Staphylococcus load, following yeast feeding, may be also associated with the ability of S. cerevisiae to counteract and inhibit Staphylococcus biofilm making, as previously observed (Walencka et al., 2007).
The two yeast strains showed different abilities to change the bacterial microbiota composition. The YH1 strain displays the strongest caste-dependent immunomodulatory effect, to the detriment of the resistance to E. coli infection in the workers, and shows increased ability in changing the gut microbiota, suggesting that the changes in the bacterial communities are related to strain-specific immunomodulation. While this effect is evident for bacterial communities, S. cerevisiae administration does not alter the diversity of the fungal communities. In the latter, only the Metschnikowia genus was reduced. Yeast–yeast competition for nutrients are well-known, especially regarding S. cerevisiae and Metschnikowia growing in fermentative processes (Alexandre et al., 2004; Fleet, 2008; Albergaria and Arneborg, 2016; Ciani et al., 2016; Sadoudi et al., 2017). Our results suggest that the competition between these two yeasts is not limited to grape must but may occur also in the insect gut.
It is unlikely that the observed effects are influenced by a role of S. cerevisiae as nutrient supplementation: (i) it is known that in P. dominula, the green fluorescent protein (GFP)-labeled S. cerevisiae, ingested by wasps, populate the gut for at least 4 months (Stefanini et al., 2012, 2016) (thus, the microorganism is still alive after treatment and survives after passage through the wasps’ digestive tract); (ii) immune training occurred only in foundresses and not in workers, showing a caste-dependent effect in insects that received the same amount of S. cerevisiae cells (if the effect would have been related to nutrition, one would expect this to be equal regardless of the caste); (iii) the two strains showed significant differences in the ability to improve the bacterial clearance (a strain-dependent immune-mediated event excludes a general nutritional effect of the yeast); and (iv) the differences in immune enhancing are mirrored by differences in the changes of the microbiota composition (this finding corroborates our conclusion that the effect is mediated by immune responses and not just by feeding).
Overall, this study provides a preliminary indication of the complex interactions existing between insects’ immune responses and their symbiotic gut communities. Future studies using insects as models promise stimulating information that will potentially uncover the relationship between immune priming and physiological functions, as well as their effect on gut microbiota. Our results suggest that yeasts and social insects possess key synergistic elements and that a deeper understanding of these interactions, including the molecular determinants of the priming and trained immunity, may provide significant insights on the host immune system–microbe evolution.
Data are available as electronic Supplementary Material. Illumina sequencing data are available at the European Nucleotide Archive (http://www.ebi.ac.uk/ena/data/view/PRJEB32390) under accession study PRJEB32390.
DC, ST, and NM conceived the study. NM, DR, FC, FT, and AG carried out the experiment. LD and NM performed the statistical analyses. FV performed the metagenomic and data analysis. NM, MP, LB, and DC drafted the manuscript. All authors critically revised the manuscript, approved the final version of the manuscript, and agreed to be held accountable for the content therein.
This study was funded by the University of Florence, Regione Toscana POR FSE 2014–2020, progetto VESPATER and Jay Pritzker’s Foundation.
The authors declare that the research was conducted in the absence of any commercial or financial relationships that could be construed as a potential conflict of interest.
The Supplementary Material for this article can be found online at: https://www.frontiersin.org/articles/10.3389/fmicb.2019.02320/full#supplementary-material
Albanese, D., Fontana, P., De Filippo, C., Cavalieri, D., and Donati, C. (2015). MICCA: a complete and accurate software for taxonomic profiling of metagenomic data. Sci. Rep. 5:9743. doi: 10.1038/srep09743
Albergaria, H., and Arneborg, N. (2016). Dominance of Saccharomyces cerevisiae in alcoholic fermentation processes: role of physiological fitness and microbial interactions. Appl. Microbiol. Biotechnol. 100, 2035–2046. doi: 10.1007/s00253-015-7255-0
Alexandre, G., Greer-Phillips, S., and Zhulin, I. B. (2004). Ecological role of energy taxis in microorganisms. FEMS Microbiol. Rev. 28, 113–126.
Andrews, S. (2010). FastQC: A Quality Control Tool for high Throughput Sequence data. In: Babraham Bioinformatics (version 0.11.3). Available at: http://www.bioinformatics.babraham.ac.uk/projects/fastqc (accessed March 25, 2015).
Arnolds, K. L., and Lozupone, C. A. (2016). Striking a balance with help from our little friends—how the gut microbiota contributes to immune homeostasis. Yale J. Biol. Med. 89, 389–395.
Belkaid, Y., and Hand, T. (2014). Role of the microbiota in immunity and inflammation. Cell 157, 121–141. doi: 10.1016/j.cell.2014.03.011
Bordoni, A., Dapporto, L., Tatini, I., Celli, M., Bercigli, M., Ressurrección Barrufet, S., et al. (2018). Trans-generational immunization in the acrobat ant Crematogaster scutellaris. Biol. Lett. 14:20170761. doi: 10.1098/rsbl.2017.0761
Cappa, F., Beani, L., Cervo, R., Grozinger, C., and Manfredini, F. (2015). Testing male immunocompetence in two hymenopterans with different levels of social organization: “live hard, die young?”. Biol. J. Linn. Soc. 114, 274–278. doi: 10.1111/bij.12427
Cerf-Bensussan, N., and Gaboriau-Routhiau, V. (2010). The immune system and the gut microbiota: friends or foes? Nat. Rev. Immunol. 10, 735–744. doi: 10.1038/nri2850
Charles, H. M., and Killian, K. A. (2015). Response of the insect immune system to three different immune challenges. J. Insect. Physiol. 81, 97–108. doi: 10.1016/j.jinsphys.2015.07.005
Cho, I., and Blaser, M. J. (2012). The human microbiome: at the interface of health and disease. Nat. Rev. Genet. 13, 260–270. doi: 10.1038/nrg3182
Ciani, M., Capece, A., Comitini, F., Canonico, L., Siesto, G., and Romano, P. (2016). Yeast interactions in inoculated wine fermentation. Front. Microbiol. 7:555. doi: 10.3389/fmicb.2016.00555
Cini, A., Cappa, F., Petrocelli, I., Pepiciello, I., Bortolotti, L., and Cervo, R. (2018). Competition between the native and the introduced hornets Vespa crabro and Vespa velutina: a comparison of potentially relevant life-history traits. Ecol. Entomol. 43, 351–362. doi: 10.1111/een.12507
Cooper, D., and Eleftherianos, I. (2017). Memory and specificity in the insect immune system: current perspectives and future challenges. Front. Immunol. 8:539. doi: 10.3389/fimmu.2017.00539
Dethlefsen, L., McFall-Ngai, M., and Relman, D. A. (2007). An ecological and evolutionary perspective on human–microbe mutualism and disease. Nature 449, 811–818. doi: 10.1038/nature06245
Durrant, W. E., and Dong, X. (2004). Systemic acquired resistance. Annu. Rev. Phytopathol. 42, 185–209. doi: 10.1146/annurev.phyto.42.040803.140421
Elrod-erickson, M., Mishra, S., and Schneider, D. (2000). Interactions between the cellular and humoral immune responses in Drosophila. Curr. Biol. 10, 781–784. doi: 10.1016/S0960-9822(00)00569-8
Engel, P., and Moran, N. A. (2013). The gut microbiota of insects—diversity in structure and function. FEMS Microbiol. Rev. 37, 699–735. doi: 10.1111/1574-6976.12025
Fleet, G. H. (2008). Wine yeasts for the future. FEMS Yeast Res. 8, 979–995. doi: 10.1111/j.1567-1364.2008.00427.x
Fox, J., and Weisberg, S. (2019). An R Companion to Applied Regression, 3rd Edn, Thousand Oaks, CA: Sage. Available at: https://socialsciences.mcmaster.ca/jfox/Books/Companion/
Gillespie, J. P., Kanost, M. R., and Trenczek, T. (1997). Biological mediators of insect immunity. Annu. Rev. Entomol. 42, 611–643. doi: 10.1146/annurev.ento.42.1.611
Gupta, N., Kumar, R., and Agrawal, B. (2018). New players in immunity to tuberculosis: the host microbiome, lung epithelium, and innate immune cells. Front. Immunol. 9:709. doi: 10.3389/fimmu.2018.00709
Hand, T. W. (2016). The role of the microbiota in shaping infectious immunity. Trends Immunol. 37, 647–658. doi: 10.1016/j.it.2016.08.007
Hoffmann, J. A., Kafatos, F. C., Janeway, C. A., and Ezekowitz, R. A. (1999). Phylogenetic perspectives in innate immunity. Science 284, 1313–1318.
Joshi, N. A., and Fass, J. N. (2011). Sickle: a Sliding-Window, Adaptive, Quality-Based Trimming Tool for FastQ files (version 1.33) [software].
Kandlikar, G. S., Gold, Z. J., Cowen, M. C., Meyer, R. S., Freise, A. C., Kraft, N. J. B., et al. (2018). ranacapa: an R package and Shiny web app to explore environmental DNA data with exploratory statistics and interactive visualizations. F1000Res 7:1734. doi: 10.12688/f1000research.16680.1
Kolde, R. (2012). pheatmap: pretty heatmaps. R package version 1.0.12. Available at: https://CRAN.R-project.org/package=pheatmap (accessed December 26, 2018).
Kubrak, O. I., Kučerová, L., Theopold, U., and Nässel, D. R. (2014). The sleeping beauty: how reproductive diapause affects hormone signaling, metabolism, immune response and somatic maintenance in Drosophila melanogaster. PLoS One 9:e113051. doi: 10.1371/journal.pone.0113051
Lahti, L., Shetty, S. et al. (Bioconductor, 2017). Tools for Microbiome Analysis in R. Microbiome Package Version 1.7.21. Available at: http://microbiome.github.com/microbiome
Lehrer, R. I., and Ganz, T. (1999). Antimicrobial peptides in mammalian and insect host defence. Curr. Opin. Immunol. 11, 23–27. doi: 10.1016/S0952-7915(99)80005-3
Liti, G., Carter, M. D., Moses, A. M., Warringer, J., Parts, L., James, S. A., et al. (2009). Population genomics of domestic and wild yeasts. Nature 458, 337–341. doi: 10.1038/nature07743
Little, T. J., and Kraaijeveld, A. R. (2004). Ecological and evolutionary implications of immunological priming in invertebrates. Trends Ecol. Evol. 19, 58–60. doi: 10.1016/j.tree.2003.11.011
Lynch, S. V., and Pedersen, O. (2016). The human intestinal microbiome in health and disease. N. Engl. J. Med. 375, 2369–2379. doi: 10.1056/NEJMra1600266
McMurdie, P. J., and Holmes, S. (2013). phyloseq: an R package for reproducible interactive analysis and graphics of microbiome census data. PLoS One 8:e61217. doi: 10.1371/journal.pone.0061217
Milutinović, B., and Kurtz, J. (2016). Immune memory in invertebrates. Semin. Immunol. 28, 328–342. doi: 10.1016/j.smim.2016.05.004
Mulder, W. J. M., Ochando, J., Joosten, L. A. B., Fayad, Z. A., and Netea, M. G. (2019). Therapeutic targeting of trained immunity. Nat. Rev. Drug Discov. 18, ages553–ages566. doi: 10.1038/s41573-019-0025-4
Netea, M. G., Quintin, J., and van der Meer, J. W. (2011). Trained immunity: a memory for innate host defense. Cell Host Microbe. 9, 355–361. doi: 10.1016/j.chom.2011.04.006
Netea, M. G., Schlitzer, A., Placek, K., Joosten, L. A. B., and Schultze, J. L. (2019). Innate and adaptive immune memory: an evolutionary continuum in the host’s response to pathogens. Cell Host Microbe. 25, 13–26. doi: 10.1016/j.chom.2018.12.006
Norouzitallab, P., Baruah, K., Biswas, P., Vanrompay, D., and Bossier, P. (2016). Probing the phenomenon of trained immunity in invertebrates during a transgenerational study, using brine shrimp Artemia as a model system. Sci. Rep. 6:21166. doi: 10.1038/srep21166
Oksanen, J., Guillaume Blanchet, F., Friendly, M., Kindt, R., Legendre, P., McGlinn, D., et al. (2018). Vegan: Community Ecology Package. Available at: https://CRAN.R-project.org/package=vegan
Parks, H. D., Tyson, W. G., Hugenholtz, P., and Beiko, G. R. (2014). STAMP: statistical analysis of taxonomic and functional profiles. Bioinformatics 30, 3123–3124. doi: 10.1093/bioinformatics/btu494
Paulson, J. N., Talukder, H., Pop, M., and Bravo, H. C. (2013). metagenomeSeq: Statistical Analysis for Sparse High-Throughput Sequencing. Bioconductor package: 1.24.1. Available at: http://cbcb.umd.edu/software/metagenomeSeq
Pham, L. N., Dionne, M. S., Shirasu-Hiza, M., and Schneider, D. S. (2007). A specific primed immune response in Drosophila is dependent on phagocytes. PLoS Pathog. 3:e26. doi: 10.1371/journal.ppat.0030026
Quintin, J., Saeed, S., Martens, J. H. A., Giamarellos-Bourboulis, E. J., Ifrim, D. C., Logie, C., et al. (2012). Candida albicans infection affords protection against reinfection via functional reprogramming of monocytes. Cell Host Microbe. 12, 223–232. doi: 10.1016/j.chom.2012.06.006
R Core Team (2018). R: A Language and Environment for Statistical Computing. Vienna: R Foundation for Statistical Computing.
Ramazzotti, M., Stefanini, I., Di Paola, M., De Filippo, C., Rizzetto, L., Berná, L., et al. (2019). Population genomics reveals evolution and variation of Saccharomyces cerevisiae in the human and insects gut. Environ Microbiol. 21, 50–71. doi: 10.1111/1462-2920.14422
Rizzetto, L., Ifrim, D. C., Moretti, S., Tocci, N., Cheng, S. C., Quintin, J., et al. (2016). Fungal chitin induces trained immunity in human monocytes during cross-talk of the host with Saccharomyces. J. Biol. Chem. 291, 7961–7972. doi: 10.1074/jbc.M115.699645
Rodrigues, J., Brayner, F. A., Alves, L. C., Dixit, R., and Barillas-Mury, C. (2010). Hemocyte differentiation mediates innate immune memory in Anopheles gambiae mosquitoes. Science 329, 1353–1355. doi: 10.1126/science.1190689
Rosengaus, R. B., Traniello, J. F. A., Chen, T., Brown, J. J., and Karp, R. D. (1999). Immunity in a social insect. Naturwissenschaften 86, 588–591. doi: 10.1007/s001140050679
Rusek, P., Wala, M., Druszczyńska, M., and Fol, M. (2018). Infectious agents as stimuli of trained innate immunity. Int. J. Mol. Sci. 19:E456. doi: 10.3390/ijms19020456
Sadd, B. M., Kleinlogel, Y., Schmid-Hempel, R., and Schmid-Hempel, P. (2005). Trans-generational immune priming in a social insect. Biol. Lett. 1, 386–388. doi: 10.1098/rsbl.2005.0369
Sadd, B. M., and Schmid-Hempel, P. (2006). Insect immunity shows specificity in protection upon secondary pathogen exposure. Curr. Biol. 16, 1206–1210. doi: 10.1016/j.cub.2006.04.047
Sadoudi, M., Rousseaux, S., David, V., Alexandre, H., and Tourdot-Maréchal, R. (2017). Metschnikowia pulcherrima influences the expression of genes involved in PDH bypass and glyceropyruvic fermentation in Saccharomyces cerevisiae. Front. Microbiol. 8:1137. doi: 10.3389/fmicb.2017.01137
Silverman, N., and Maniatis, T. (2001). NF-κB signaling pathways in mammalian and insect innate immunity. Genes Dev. 15, 2321–2342. doi: 10.1101/gad.909001
Stefanini, I. (2018). Yeast–insect associations: it takes guts. Yeast 35, 315–330. doi: 10.1002/yea.3309
Stefanini, I., Dapporto, L., Berná, L., Polsinelli, M., Turillazzi, S., and Cavalieri, D. (2016). Social wasps are a Saccharomyces mating nest. Proc. Natl. Acad. Sci. U.S.A. 113, 2247–2251. doi: 10.1073/pnas.1516453113
Stefanini, I., Dapporto, L., Legras, J., Calabretta, A., Di Paola, M., De Filippo, C., et al. (2012). Role of social wasps in Saccharomyces cerevisiae ecology and evolution. Proc. Natl. Acad. Sci. U.S.A. 109, 13398–13403. doi: 10.1073/pnas.1208362109
Takahashi, S., Tomita, J., Nishioka, K., Hisada, T., and Nishijima, M. (2014). Development of a prokaryotic universal primer for simultaneous analysis of bacteria and archaea using next-generation sequencing. PLoS One 9:e105592. doi: 10.1371/journal.pone.0105592
Walencka, E., Wieckowska-Szakiela, M., Rozalskab, S., Sadowska, B., and Rozalska, B. (2007). A surface-active agent from Saccharomyces cerevisiae influences staphylococcal adhesion and biofilm development. Z. Naturforsch. C. 62, 433–438.
Wang, Q., Garrity, G. M., Tiedje, J. M., and Cole, J. R. (2007). Naive Bayesian classifier for rapid assignment of rRNA sequences into the new bacterial taxonomy. Appl. Environ. Microbiol. 73, 5261–5267.
Wang, Y. I., Naumann, U., Wright, S. T., and Warton, D. I. (2012). mvabund—an R package for model-based analysis of multivariate abundance data. Methods Ecol. Evol. 3, 471–474. doi: 10.1111/j.2041-210X.2012.00190.x
White, T. J., Bruns, T. D., Lee, S. B. and Taylor, J. W. (1990) Amplification and direct sequencing of fungal ribosomal RNA genes for phylogenetics, in PCR Protocols: A Guide to Methods and Applications, eds M. A. Innis, D. H. Gelfand, J. J. Sninsky, and T. J. White, New York, NY: Academic Press, 315-322.
Keywords: innate immunity, immune training, Saccharomyces cerevisiae, gut microbiota, Polistes dominula
Citation: Meriggi N, Di Paola M, Vitali F, Rivero D, Cappa F, Turillazzi F, Gori A, Dapporto L, Beani L, Turillazzi S and Cavalieri D (2019) Saccharomyces cerevisiae Induces Immune Enhancing and Shapes Gut Microbiota in Social Wasps. Front. Microbiol. 10:2320. doi: 10.3389/fmicb.2019.02320
Received: 10 June 2019; Accepted: 23 September 2019;
Published: 15 October 2019.
Edited by:
Lia Danelishvili, Oregon State University, United StatesReviewed by:
Nathan T. Mortimer, Illinois State University, United StatesCopyright © 2019 Meriggi, Di Paola, Vitali, Rivero, Cappa, Turillazzi, Gori, Dapporto, Beani, Turillazzi and Cavalieri. This is an open-access article distributed under the terms of the Creative Commons Attribution License (CC BY). The use, distribution or reproduction in other forums is permitted, provided the original author(s) and the copyright owner(s) are credited and that the original publication in this journal is cited, in accordance with accepted academic practice. No use, distribution or reproduction is permitted which does not comply with these terms.
*Correspondence: Stefano Turillazzi, c3RlZmFuby50dXJpbGxhenppQHVuaWZpLml0; Duccio Cavalieri, Y2F2YWxpZXJpLnVuaWZpQGdtYWlsLmNvbQ==; ZHVjY2lvLmNhdmFsaWVyaUB1bmlmaS5pdA==
Disclaimer: All claims expressed in this article are solely those of the authors and do not necessarily represent those of their affiliated organizations, or those of the publisher, the editors and the reviewers. Any product that may be evaluated in this article or claim that may be made by its manufacturer is not guaranteed or endorsed by the publisher.
Research integrity at Frontiers
Learn more about the work of our research integrity team to safeguard the quality of each article we publish.