- Department of Pharmacy, Qilu Children’s Hospital, Shandong University, Jinan, China
Several studies have demonstrated the significant antiviral, antimicrobial, antiplasmodial, antioxidative, antifungal, antimutagenic, and antitumor properties of harmine hydrochloride (HMH). The main objective of the present study was to investigate the antifungal effects and underlying mechanisms of HMH when combined with azoles to determine whether such combinations act in a synergistic manner. As a result, we found that HMH exhibits synergistic antifungal effects in combination with azoles against resistant Candida albicans (C. albicans) planktonic cells, as well as resistant C. albicans biofilm in the early stage. Antifungal potential of HMH with fluconazole was also explored in vivo using an invertebrate model. Our results suggest that HMH combined with azoles is synergistic against resistant C. albicans in vitro and in vivo. No synergy is seen with azole sensitive C. albicans strains nor with other Candida species. Such synergistic mechanisms on resistance C. albicans are involved in increasing intracellular azoles, inhibiting hyphal growth, disturbing cytosolic calcium concentration, and inducing apoptosis of resistant C. albicans cells.
Introduction
Despite therapeutic advances, candidiasis remains the most common fungal infection, 50% of which is caused by Candida albicans (C. albicans) (Yapar, 2014; Pu et al., 2017). C. albicans can usually be associated with high mortality (Wisplinghoff et al., 2004). The choice of appropriate antifungals is very important for patients with the C. albicans infection treatment. Therapies aimed to treat these infections are limited to azoles, polyenes, and echinocandins. Among them, azoles are the most commonly used antifungals due to their better efficacy and higher safety levels. Increases in resistant C. albicans complicate the therapy for C. albicans infections. Therefore, managing resistant fungi and discovering drug alternatives against resistant C. albicans are significant challenges for clinical therapy and antifungal drug development. Importantly, understanding the inner mechanisms associated with fungal resistance is particularly important for overcoming drug resistance and developing new antifungal agents. In recent years, the concept of combination antifungal therapy has become a research hotspot (Marr, 2004; Liu et al., 2014). Different drugs or compounds in combination with antifungal are recommended to inhibit C. albicans, especially resistant C. albicans.
Harmine hydrochloride (HMH) is an abundant alkaloid in nature and several studies have demonstrated its multiple pharmacological activities, such as antiviral, antibacterial, antiplasmodial, antioxidative, antifungal, antimutagenic, and anticancer properties (Zaker et al., 2007; Egusa et al., 2011; Frost et al., 2011; Hamsa and Kuttan, 2011; Yonezawa et al., 2011; Onishi et al., 2012; Reus et al., 2012; Chen et al., 2015). Although reports have been published on the antifungal effects of HMH (Patel et al., 2012), its synergistic effect with antifungal agents is yet to be reported.
Therefore, our aim was to determine the antifungal potential of HMH in combination with azoles in vitro and in vivo. Furthermore, we aimed to elucidate the antifungal mechanisms of these drug combinations. In this study, the microdilution method was used to determine the in vitro antifungal effects of HMH in combination with azoles on planktonic cells and biofilms. G. mellonella infection model was used to evaluate the in vitro antifungal effects of these drug combinations. In addition, changes in the biofilm formation, hyphal growth, cytosolic calcium concentration ([Ca2+]i) and apoptosis markers were measured to elucidate the anti-resistance mechanisms of these drug combinations.
Materials and Methods
Isolates and Media
As shown in Table 1, 12 Candida spp. isolates were used in this study. These isolates and the quality control isolate, ATCC 10231, were obtained from Shandong Provincial Qianfoshan Hospital (Jinan, China). Before each experiment, each isolate was revived twice on Sabouraud’s Dextrose Agar (SDA) solid medium for 24 h. RPMI (Roswell Park Memorial Institute) 1640 medium was used in the experiments.
Antifungal Agents and G. mellonella Larvae
All reagents in this study were purchased from Dingguo Changsheng Biotech Co., Ltd. (Beijing, China). All stock solutions, including HMH (5,120 μg/mL in sterile distilled water), fluconazole (FLC, 2,560 μg/mL in sterile distilled water), itraconazole (ITR, 2,560 μg/mL in DMSO), voriconazole (VRC, 2,560 μg/mL in DMSO) and oxacillin sodium (2,560 μg/mL in sterile distilled water), were stored at −20°C. G. mellonella larvae were purchased from Tianjin Huiyude Biotech Co., Ltd. (Tianjin, China). Weighing (0.25 ± 0.03 g) of larvae was performed in all assays.
Determination of Minimum Inhibitory Concentrations (MICs) of Planktonic Cells in vitro
Minimum inhibitory concentrations of antifungal agents were tested based on CLSI standard reference method (M27-A3), using 96-well microtiter plates. The concentrations of azoles and HMH were adjusted to 0.125 μg/mL to 64 μg/mL, and 16 μg/mL to 1,024 μg/mL, respectively. Meanwhile, Candida spp. cell suspensions with a final concentration of 1.0 × 103 cells/mL were added to the 96-well microtiter plates. Plates containing FLC were incubated for 24 h and plates containing ITR or VRC were incubated for 48 h at 35°C. After incubation, XTT assay was used for the detection of cell growth at 492 nm by the microplate reader. MIC endpoints were read as previously described (Pfaller et al., 2008; Li et al., 2017). Experiments were performed in triplicate. The MIC of the control strain ATCC10231 was within the prescript of CLSI M27-A3.
Determination of Sessile Minimum Inhibitory Concentrations (SMICs) of Biofilms in vitro
Candida albicans biofilm formation was performed as described elsewhere with some modifications (Vila et al., 2013; Wang et al., 2015). The biofilms of CA10 and CA16 were formed over 90 min or 24 h at 35°C by adding 200 μL of the standardized cell suspension (5.0 × 106 cells/mL) into selected wells of 96-well plates. After 90 min or 24 h, the wells were gently washed three times with sterile PBS to remove any remaining non-adhering cells. HMH and azoles were then added to the wells. After incubation for 48 h at 35°C, XTT assay was used for detection at 492 nm by the microplate reader. The drug concentration that reduces the absorption value by 50% relative to control was regarded as the SMIC endpoint. Experiments were performed in triplicate.
Drug Interaction Interpretation
Interactions of the drug combination were interpreted according to the fractional inhibitory concentration index (FICI) model based on Loewe Additivity (LA) theory as previously described (Odds, 2003; Li et al., 2017).
Determination of in vivo Antifungal Effects
Survival Assay
Galleria mellonella survival assays were carried out according to the previously described method with few modifications (Mylonakis et al., 2005; Scorzoni et al., 2013). The larvae weighing (0.25 ± 0.03 g) were employed in all assays. A resistant isolate, CA10, was used in this experiment. Larvae were stored in wood chips without light at 25°C before the start of the experiments. Each group contained 20 randomly chosen larvae. C. albicans cells were incubated in liquid SDA medium for 24 h. Cell suspensions of C. albicans 5 × 108 cells/mL were prepared in PBS and 20 μg/mL of oxacillin sodium was added to prevent bacterial infection. Larvae were inoculated in C. albicans suspensions (final inoculated concentration: 5 × 106 cells/larva) as previously described (Li et al., 2017). At 2.5 h after post-infection, infected larvae were treated with FLC (final concentration in vivo: 1.6 μg/larva) and HMH (final concentration in vivo: 1.6 μg/larva) alone or in combination. A group of infected larvae without drug treatment was regarded as control. Larvae were kept on petri dishes at 35°C and monitored daily for survival. Death was identified when there was no movement after touching with metal tweezers. All assays were repeated on three separate occasions.
Histological Study
Galleria mellonella larvae were treated with CA10 and drugs as described above. Three days after incubation, one larva from each group was collected, washed and fixed in 4% paraformaldehyde for 24 h. Subsequently, larvae were embedded using optimal cutting temperature compound and 8-μm tissue slices of larvae were made using a freezing microtome. Each tissue slice was processed as previously described (Li et al., 2017). All assays were repeated on three separate occasions.
Synergistic Mechanism Explorations
Assay for the Uptake and Efflux of Rhodamine 6G (R6G)
R6G was used to specifically detect whether HMH affects the intracellular concentration of azoles in resistant C. albicans isolate (CA10). The experiment was conducted as previously described (Li et al., 2017), with minor modifications. The C. albicans cells were incubated by shaking at 180 rpm in SDA liquid medium at 35°C for 18 h and harvested at mid-log phase by centrifugation at 3000 rpm and 4°C for 10 min. The samples were washed twice with PBS and re-suspended in PBS.
For the R6G uptake assay, R6G (10 μM) and HMH (128 μg/mL) were simultaneously added to the prepared cells. Cells treated with R6G alone were regarded as control. The BD FACSAria II flow cytometer (excitation at 488 nm, emission at 530 nm) was used to determine mean fluorescent intensity (MFI) every 10 min. Each assay was performed in triplicate.
For the R6G efflux assay, cells were prepared in the same manner described above and starved for 2 h at 35°C. The starved cells were harvested at 3000 rpm and 4°C for 10 min and resuspended in PBS. Subsequently, 1.0 × 107 cells/mL was incubated in the presence of R6G (10 μM) in 5 mL PBS at 35°C for 60 min to preload the cells with the R6G. Preloaded cells were harvested and treated in an ice bath for 15 min to stop preloading. Cells were pelleted by centrifugation at 3000 rpm and 4°C for 10 min, washed twice with PBS, and resuspended in PBS. Finally, HMH (128 μg/mL) was added to 5% glucose-PBS. Another group without HMH treatment was regarded as control. The MFI was determined with a flow cytometer every 10 min. Each assay was performed in triplicate.
Hypha Growth Assay
Two hundred μL suspension of CA10 cells (1.0 × 105 cells/mL) treated with FLC (1 μg/mL) and HMH (32 μg/mL) alone or in combination was incubated in 96-well microtiter plates at 35°C for 4 h. Sample without drug treatment was regarded as control. Plates were shaken at 80 rpm for cell attachment to the surfaces of the disks. Unattached cells were subsequently removed, and the disks were washed twice with PBS. All samples were observed under a Leica DMI3000 microscope with the 10× objective and the 10× eyepiece. The experiment was performed in triplicate.
Cytosolic Calcium Concentration ([Ca2+]i) Measurement
The fluorescent probe Fluo-3/AM (3 mM) was used to determine the changes of [Ca2+]i with different drug treatment. The drug free control was set with only Fluo-3/AM. The intensity of Fluo-3/AM fluorescence in C. albicans cells was measured in detail as R6G above and calculated with the following formula:
where Kd is the calcium dissociation constant of the Fluo-3/AM combination (204 nmol/L at 37°C), F is the MFI of each sample, and Fmin and Fmax are the minimum and maximum fluorescence intensity of the measured values, respectively. The excitation and emission wavelengths for flow cytometer (Becton Dickinson, United States) were 488 and 530 nm, respectively. The fluorescence intensity of each sample was measured every 10 min until 60 min. Each experiment was performed three times.
ROS Production Assay
Candida albicans cells in the exponential phase were collected and washed thrice with PBS. thrice, cells (1 × 108 cells/mL) were treated or untreated with HMH (32 μg/mL) and/or FLC (1 μg/mL) for 3 h. After incubation, cells were added to 2, 7-dichlorofluorescin diacetate (DCFH-DA, Sigma, United States) and incubated for 30 min in the dark. Before the detection, cells were washed with PBS for three times to remove the residual DCFH-DA in darkness conditions. MFI values were instantly detected on the BD FACSAria II flow cytometer (excitation at 488 nm, emission at 530 nm).
Metacaspase Activity Detection
Detection of activated metacaspases was performed using the CaspACE, FITC-VAD-FMK In Situ Marker (Promega, Madison, WI, United States). Briefly, CA10 cells treated with HMH (32 μg/mL) and/or FLC (1 μg/mL) for 12 h were collected, washed in PBS, and incubated with 10 μM of FITC-VAD- FMK for 1 h at 30°C in the dark. After incubation, cells were washed once and resuspended in PBS. Microscope and image acquisitions were performed with a Leica DMi8 fluorescence microscope.
Statistics
Survival curves of G. mellonella were analyzed by the IBM SPSS statistics v.20.0 (IBM SPSS Inc., Chicago, IL, United States). Graphs of R6G and [Ca2+]i were are presented as with Graph Pad Prism 7 (GraphPad Software Inc., California, CA, United States). P < 0.05 was considered statistically significant.
Results
Planktonic Cell Assay
The effects of HMH and azoles on Candida spp. were interpreted using the FICI model as shown in Table 1 and Supplementary Table S1. HMH exerted antifungal effects against six strains (CA10, CA16, CG1, CG8, CK2, and CK3) when used alone at 24 h. For the remaining six strains (CA4, CA8, CG2, CG3, CK9, and CK10) no antifungal effects were found when HMH was used alone. For resistant C. albicans strains (CA10, CA16), evident synergism of HMH in combination with three azoles was found. With 8 μg/mL of HMH, the MIC of FLC was reduced 2048-fold and 1024-fold for CA10 and CA16, respectively. With 16 or 32 μg/mL of HMH, the MIC of ITR was reduced 512-fold and 128-fold for CA10 and CA16, respectively. Besides, the MIC of VRC was reduced by 512-fold for both CA10 and CA16 with 32 μg/mL of HMH. No synergism was however observed for azole-sensitive C. albicans strains and azole resistant or sensitive strains of other Candida species.
Biofilms Assay
The antibiofilm activity of HMH combined with three azoles on resistant C. albicans (CA10 and CA16) was interpreted by FICI. The antibiofilm results at 90 min and 24 h are shown in Tables 2, 3, respectively. Synergies were observed for the HMH and azole combinations against 90 min biofilms (Table 2), all with FICI < 0.5. However, no synergism was observed on the 24 h biofilms as shown in Table 3.
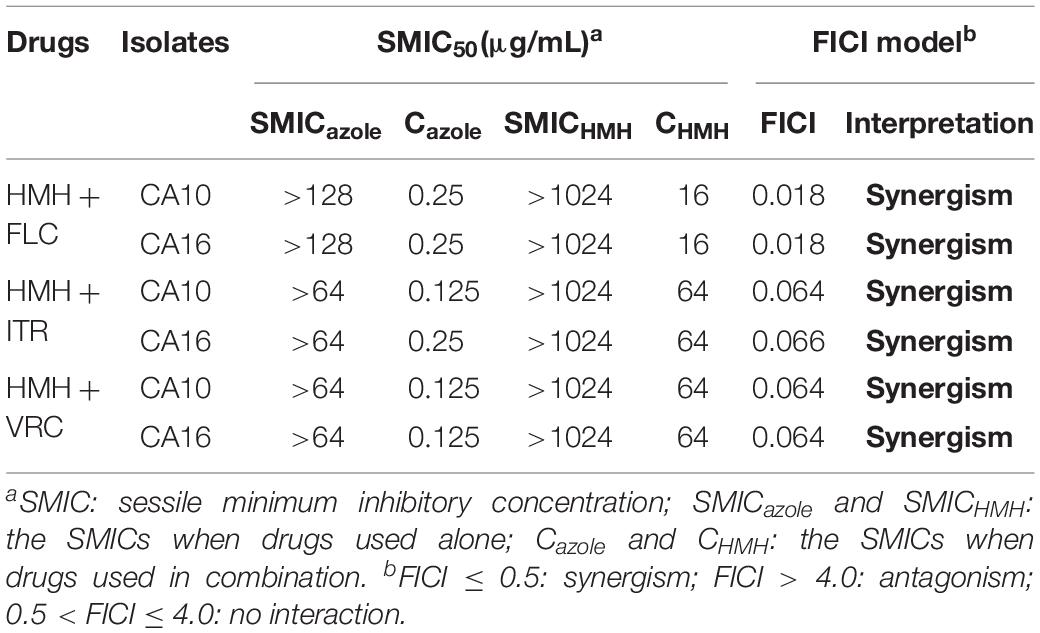
Table 2. Synergistic effects of HMH alone and in combination with azoles against 90 min biofilms of resistant C. albicans.
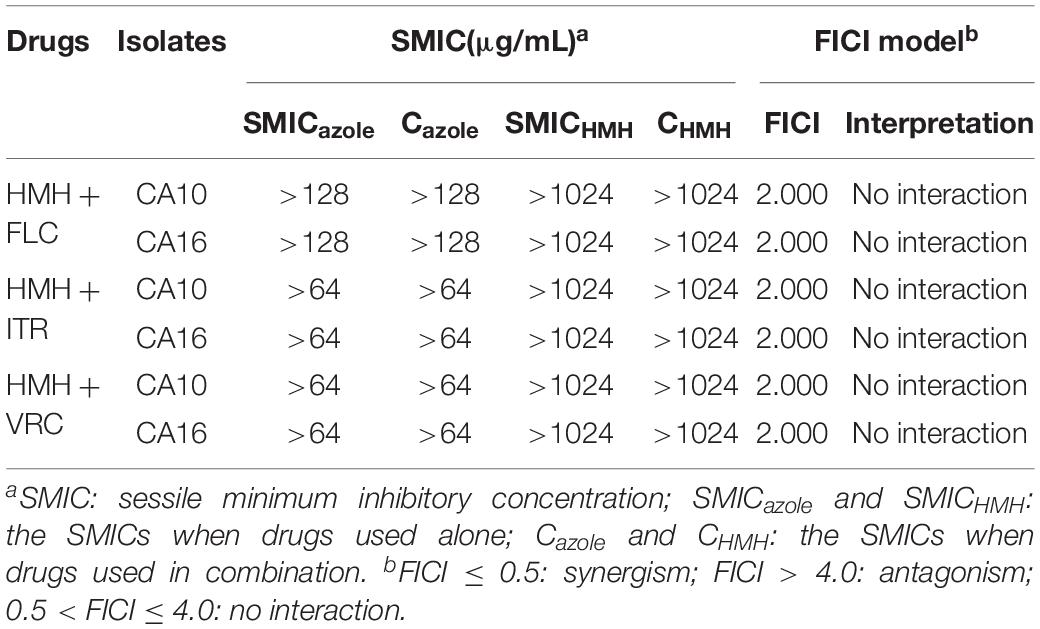
Table 3. Synergistic effects of HMH alone and in combination with azoles against 24 h biofilms of resistant C. albicans.
Survival Rate and Tissue Damage Assay
In the in vivo experiment, the resistant isolate CA10 was used, and FLC was selected as the representative of the three azoles. First, we determined whether this drug combination had an influence on the survival rates of infected G. mellonella. The combination of HMH and FLC leads to prolonged survival compared to the drug alone or control group. The difference in survival rates was statistically significant between the combination group and the other three groups (Figure 1), indicating the potential in vivo efficacy of this drug combination on resistant C. albicans.
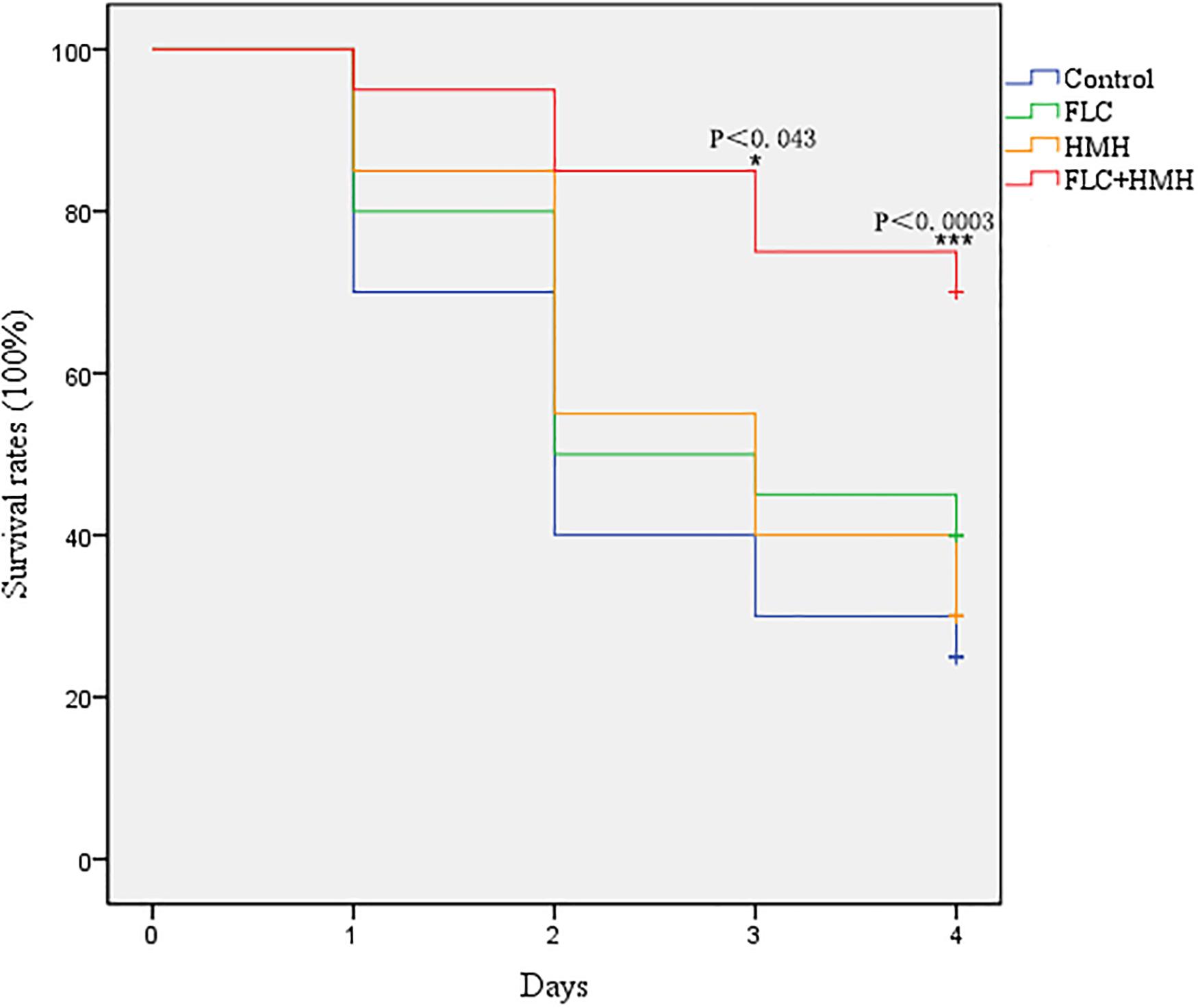
Figure 1. Effect of HMH alone or in combination with FLC on the survival rates of infected G. mellonella. The concentration of CA10 cells was 5 × 106 CFU/larva. Treatments consisted of PBS, FLC (1.6 μg/larva) alone, HMH (1.6 μg/larva) alone, or a combination of FLC (1.6 μg/larva) with HMH (1.6 μg/larva). The IBM SPSS statistics v.20.0 software was used to analyze the data.
Microscopic analysis was performed to determine the figures of infected G. mellonella tissues. In the control and drug monotherapy groups, abundant melanized nodules were observed in the tissues, which consisted of hyphae and yeast cells of C. albicans. However, the larvae that received drug combination treatment had a significant decrease in the number of melanized nodules compared to larvae in the other three groups (Figure 2).
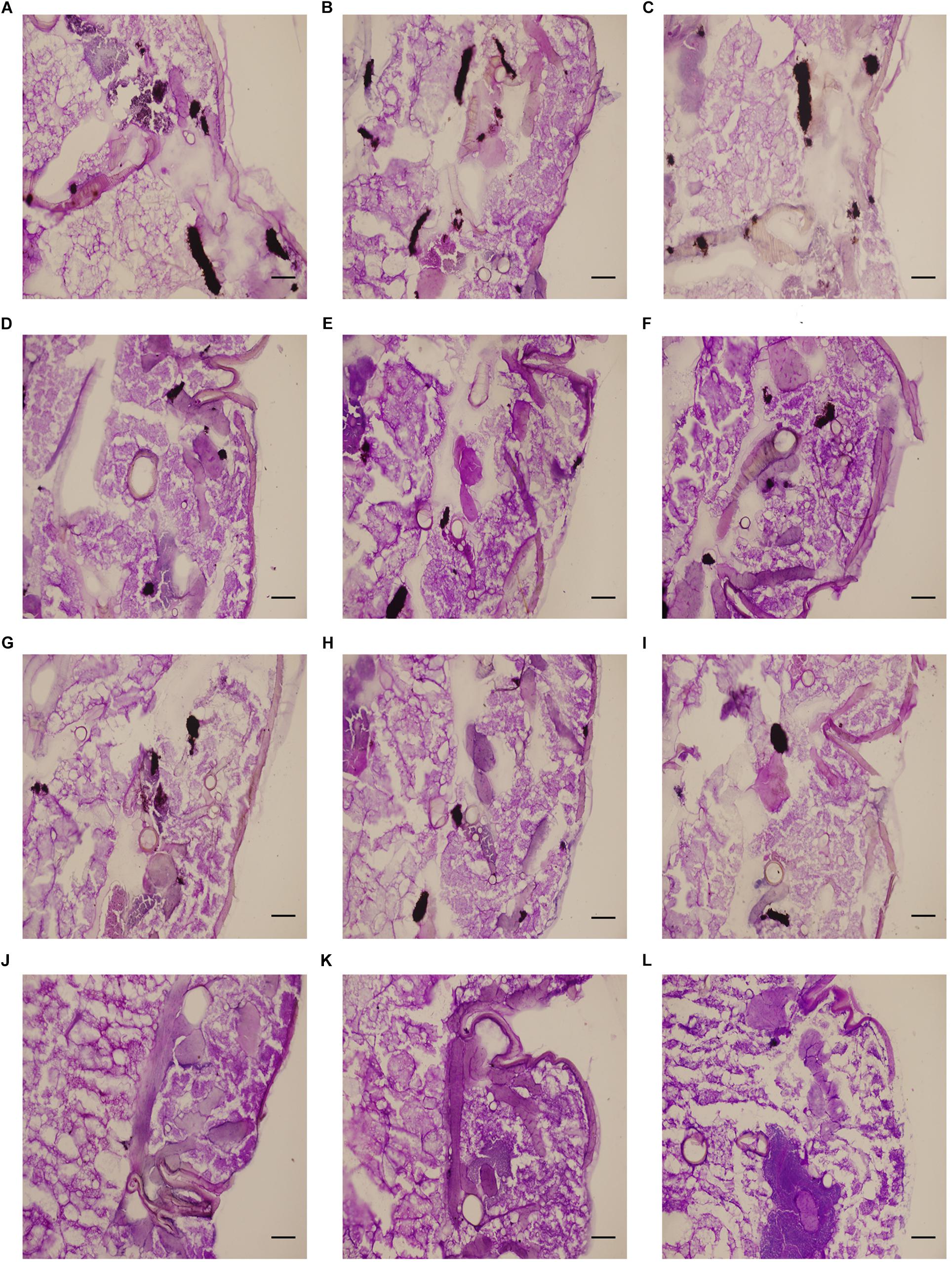
Figure 2. Effect of HMH alone or in combination with FLC on the histopathology of infected G. mellonella. The concentration of CA10 cells was 5 × 106 CFU/larva. Treatments consisted of PBS (A–C), FLC (1.6 μg/larva) alone (D–F), HMH (1.6 μg/larva) alone (G–I), or a combination (J–L) of FLC (1.6 μg/larva) with HMH (1.6 μg/larva). Melanized nodules consisted of mixtures of yeast cells and filaments. Bar = 200 μm.
Uptake and Efflux of R6G Assay
To clarify the influence of HMH on the uptake and efflux of azoles, resistant isolate CA10 was used. R6G was selected as the alternative for azoles. No difference was found in the intracellular R6G uptake of HMH-treated group and control group (Figure 3A). As shown in Figure 3B, the MFI in the HMH group was significantly higher than that in the control group at 150 min (P < 0.05), 200 min (P < 0.01), and 250 min (P < 0.01), indicating that HMH can inhibit the efflux of R6G in resistant C. albicans.
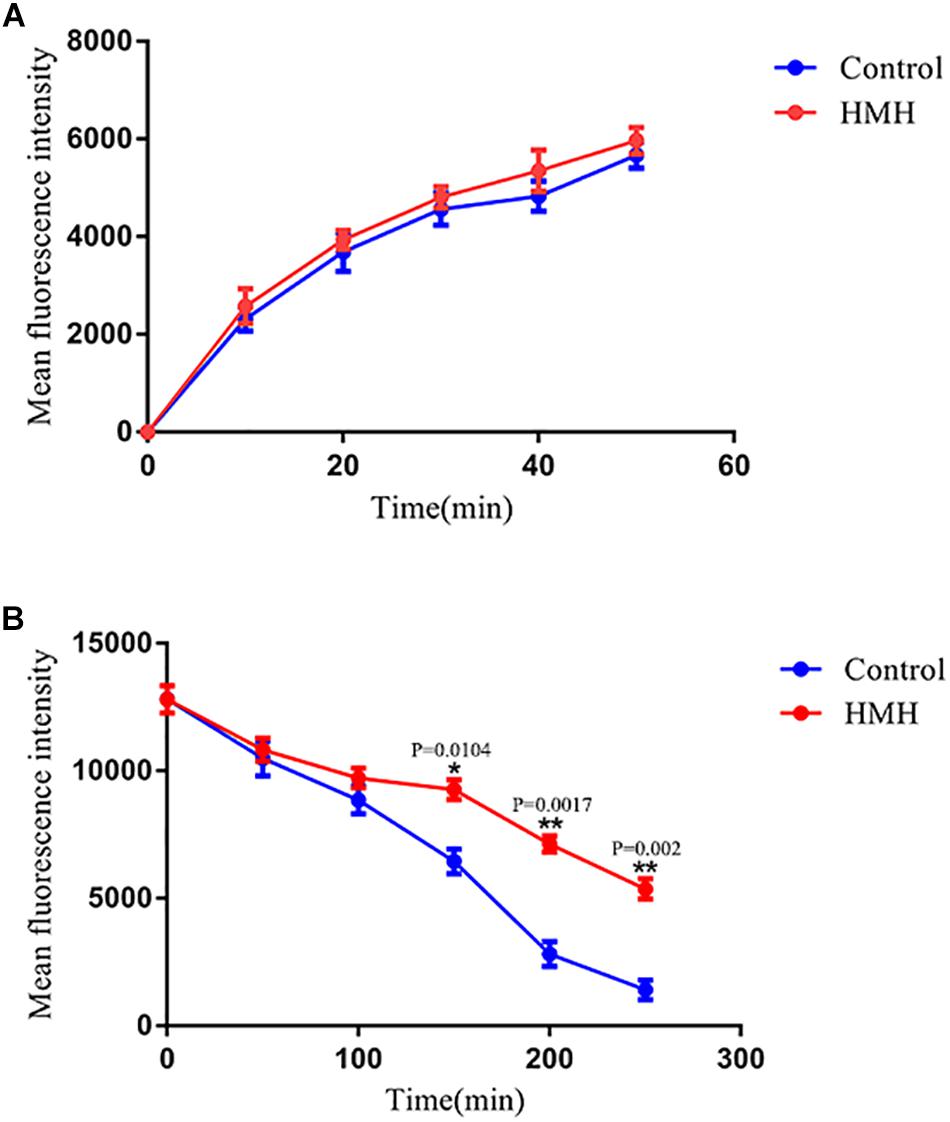
Figure 3. Effects of HMH on the (A) uptake and (B) efflux of R6G. The uptake and efflux of Rh6G in the absence and presence of HMH (128 μg/mL) were determined by a flow cytometer. Ten thousand events were counted for each sample at specific time intervals. MFIs represent the intracellular Rh6G in C. albicans. GraphPad Prism 7 software was used to analyze the data.
Hyphal Growth Observation
Microscopic images were visualized to determine the effects of HMH and FLC against resistant C. albicans (CA10) hyphal growth. As illustrated in Figure 4, the control group had many biofilms with abundant filamentous growth and yeast forms. The same morphology was observed in the FLC group and HMH alone group while little hyphae were observed in the drug combination group. Based on the microscopic images illustrated in Figure 4, this drug combination was found to exert an inhibitory effect on hyphal growth of resistant C. albicans.
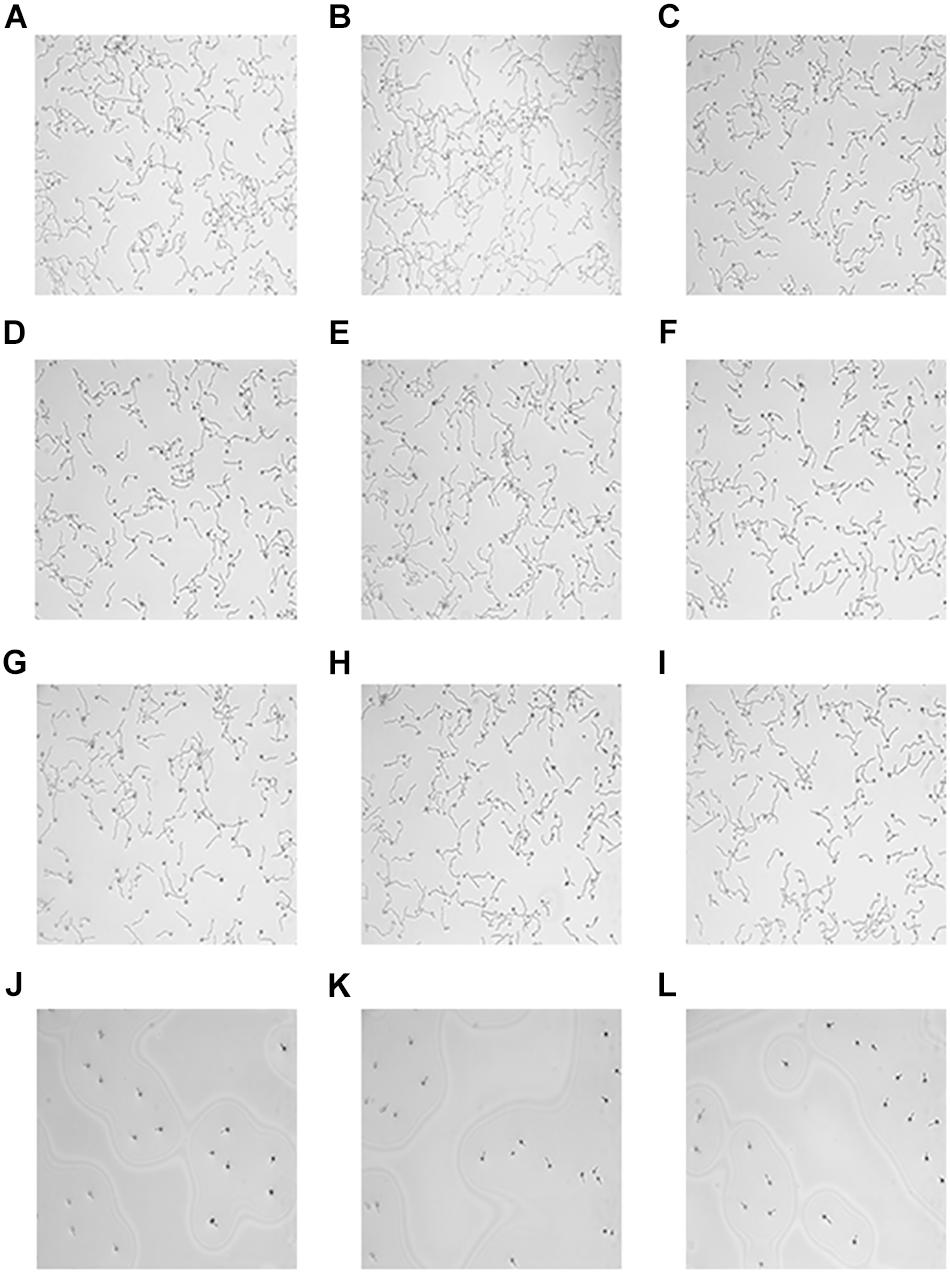
Figure 4. Microscope images hyphae. The concentration of CA10 cells was 1.0 × 105 cells/mL. Drug treatments consisted of PBS (A–C), FLC (1 μg/mL) alone (D–F), HMH (32 μg/mL) alone (G–I), and a combination (J–L) of FLC (1 μg/mL) with HMH (32 μg/mL).
[Ca2+]i Measurement
[Ca2+]i was calculated using the aforementioned formula and the changes in [Ca2+]i for each sample at different time points are shown in Figure 5. The results demonstrated that the drug combination group showed a significant increase in [Ca2+]i at 10 and 20 min, indicating that the disorder of [Ca2+]i may be one of the exact antifungal mechanisms for this drug combination against resistant C. albicans (CA10).
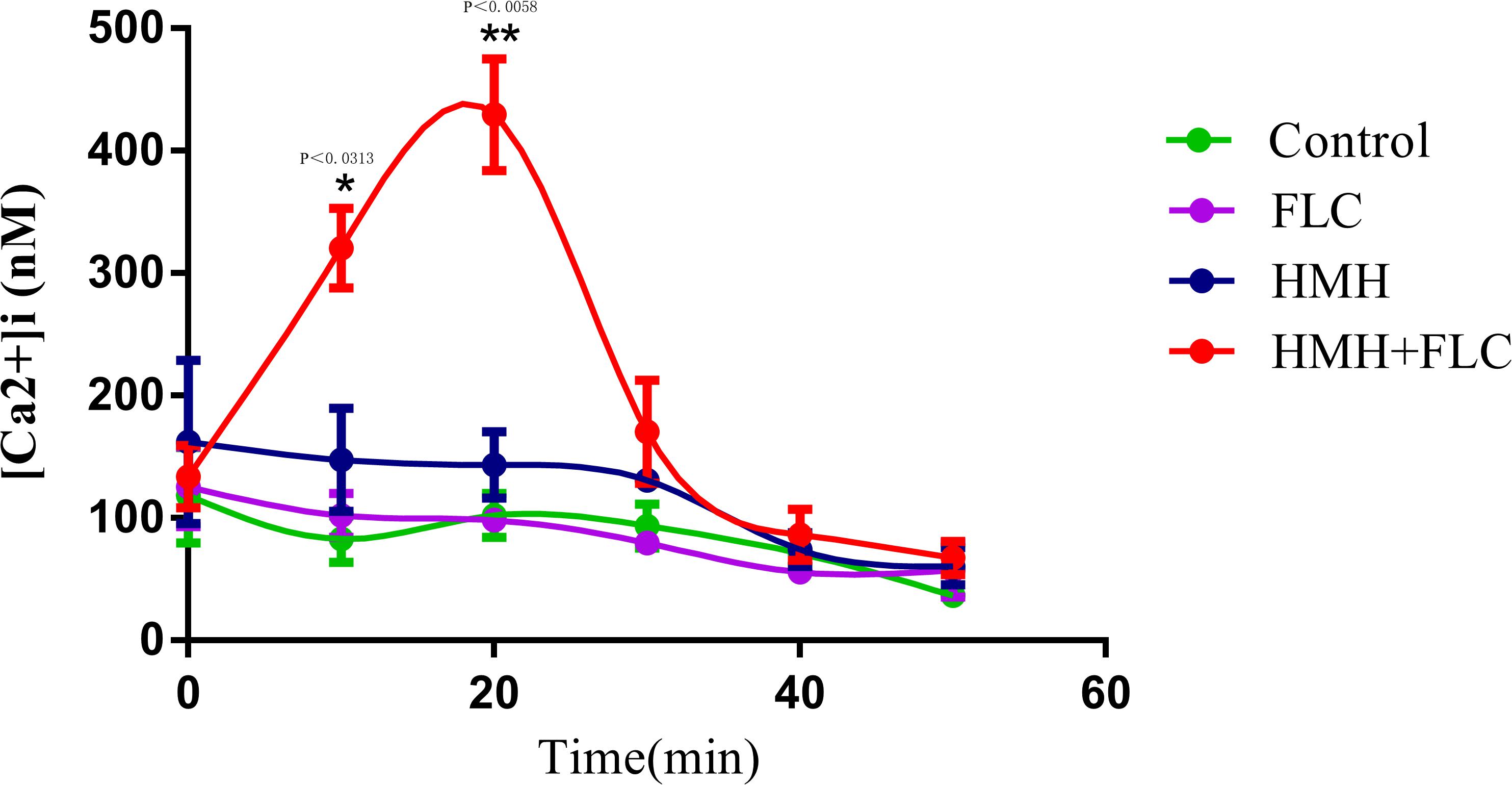
Figure 5. Changes of [Ca2+]i in resistant C. albicans. CA10 cells with Fluo-3/AM staining were analyzed using the flow cytometry. Drug treatments consisted of PBS, FLC (1 μg/mL), HMH (32 μg/mL), and a combination of FLC (1 μg/mL) with HMH (32 μg/mL). GraphPad Prism 7 software was used to analyze the data.
ROS Production Assay
To determine whether ROS participates in the synergistic mechanisms of HMH and FLC, the fluorescent probe DCFH-DA was used to monitor the production of intracellular ROS of resistant C. albicans (CA10) cells. Expectedly, cellular ROS level in the drug combination group was significantly higher than that in the other three groups (P < 0.05, Figure 6). This finding suggests that the remarkable accumulation of intracellular ROS partially results in synergistic antifungal effects of HMH and FLC against resistant C. albicans.
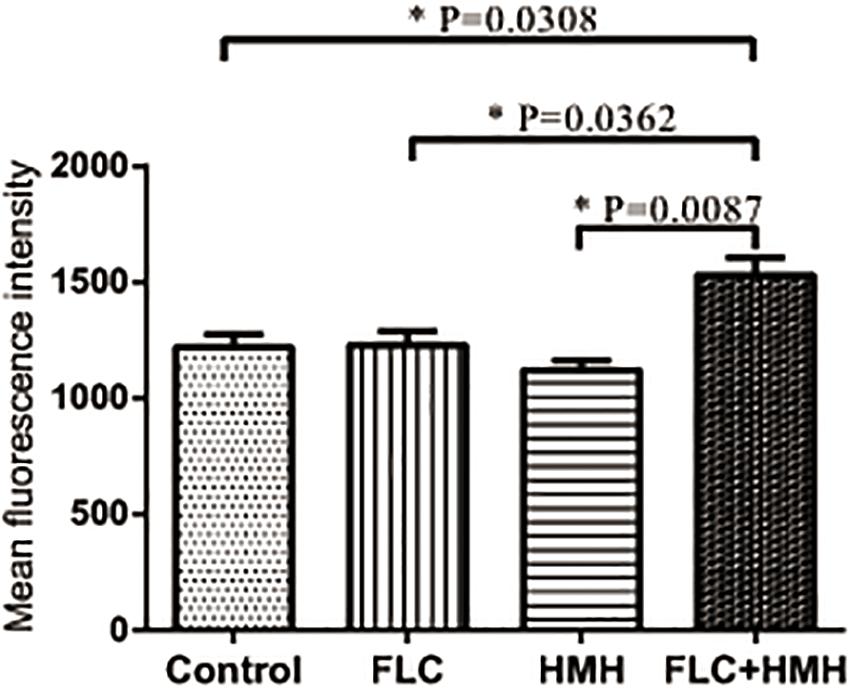
Figure 6. Effect of HMH alone or in combination with FLC on the production of ROS. CA10 cells with DCFH-DA staining were analyzed using the flow cytometry. Drug treatments consisted of PBS, FLC (1 μg/mL), HMH (32 μg/mL), and a combination of FLC (1 μg/mL) with HMH (32 μg/mL). GraphPad Prism 7 software was used to analyze the data.
Metacaspase Activity Detection
CaspACE FITC-VAD-FMK is a fluorescent dye that binds specifically to the active site of caspases. Although caspases are not present in fungi, the orthologs of caspases in animals, called metacaspases, have been identified in fungi and plants, and their activity can be assessed using the same fluorescent dye. In this study, resistant C. albicans (CA10) cells were stained with CaspACE FITC-VAD-FMK to monitor the metacaspase response. As depicted in Figure 7, cells that were simultaneously exposed to HMH and FLC significant green fluorescence, indicating metacaspases activation. In the other three groups, only few fluorescent cells were observed.
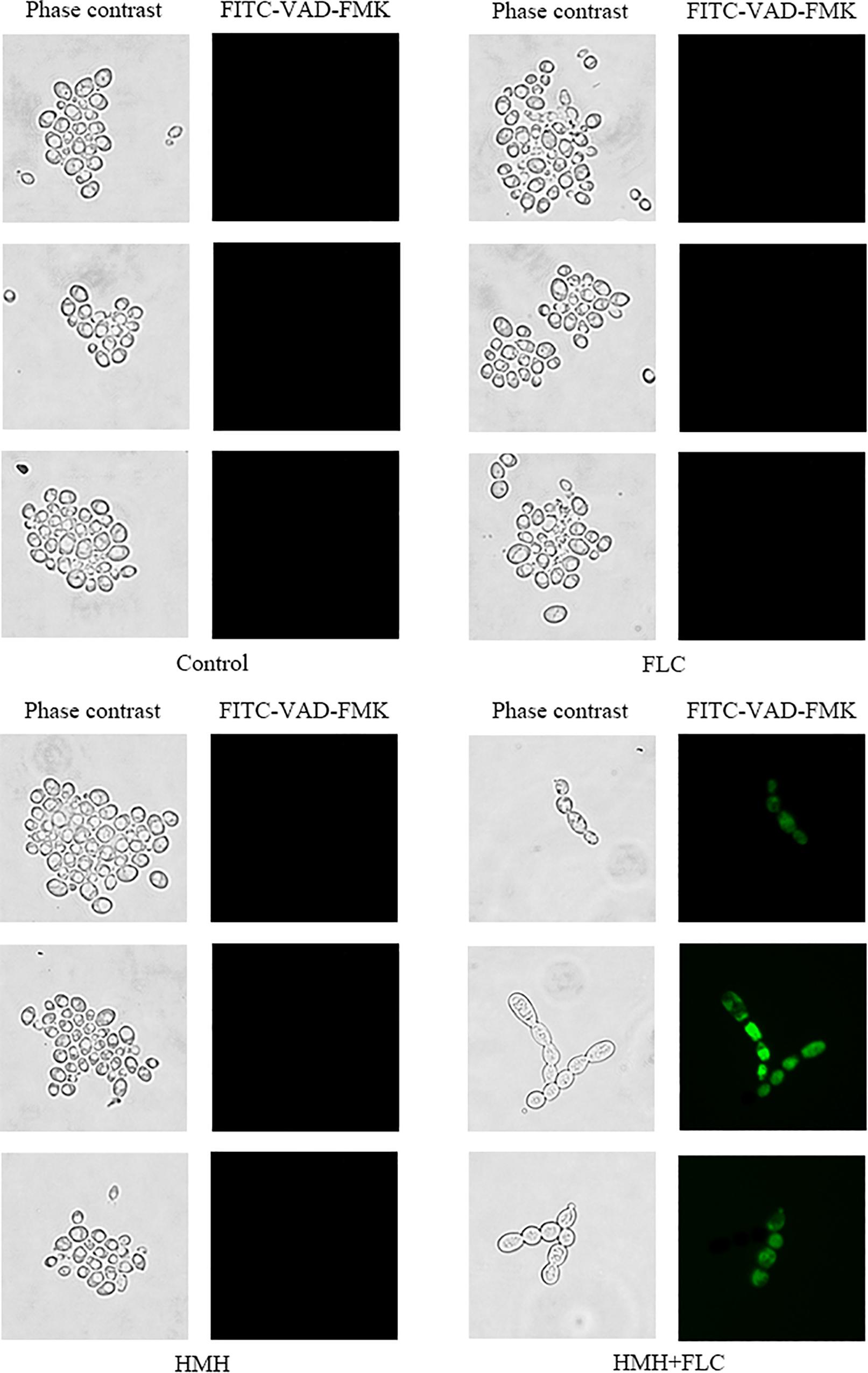
Figure 7. Effect of HMH alone or in combination with FLC on metacaspase activity. CA10 cells were collected, stained with FITC-VAD-FMK, and observed under a fluorescent microscope. Drug treatments consisted of PBS, FLC (1 μg/mL), HMH (32 μg/mL), and a combination of FLC (1 μg/mL) with HMH (32 μg/mL).
Discussion
Candida albicans is the most common human opportunistic fungal pathogen for immunocompromised patients. With the wide use of medical inbuilt catheters and the extensively abuse of conventional antifungal agents, fungal infections caused by C. albicans, especially resistant C. albicans, have become a severe clinical problem, warranting the need for newly improved antifungal strategies and therapies. As a research focus, combination therapies of antifungal drugs with non-antifungal agents have been proposed to treat drug-resistant C. albicans (Liu et al., 2014). To date, there has been no research on the combined antifungal effects of HMH with antifungal drugs. Nonetheless, HMH alone has been shown to exert antifungal effect on C. albicans (Nenaah, 2010). In this study, we evaluated the effects of azoles (FLC, ITR, and VRC) combined with HMH against Candia species in vitro. G. mellonella model was chosen to evaluate the effects of the drug combination in vivo. Furthermore, we sought to determine the possible synergistic mechanisms.
In the present study, we reported the MICs of drugs across all tested strains. The synergistic antifungal effects of HMH with FLC, ITR or VRC on resistant C. albicans strains were found. However, no synergy was seen with azole sensitive C. albicans strains nor with other Candida species. The results of MICs indicated that HMH and azoles can be combined as potential therapeutic protocols against resistant C. albicans infections.
The pathogenesis of C. albicans infection is mediated by a variety of virulence factors. Biofilm formation is a determinant of the cause of C. albicans infections (Uppuluri et al., 2010). Here, we found that biofilm pre-formed for 90 min was synergistically inhibited by the combination of HMH and azoles, thereby indicating the potential use of these combinations for the prevention or early treatment of biofilm-related C. albicans infections.
In recent years, a great similarity between results obtained from invertebrate models and mammalian models has been proven for drug efficacy evaluation (Cotter et al., 2000; Browne et al., 2013). To avoid the ethical issues associated with mammalian models and to reduce the cost of experiments, researchers have sought to develop invertebrate models to study microbial virulence (Cotter et al., 2000; Browne et al., 2013). Compared to mammalian models, G. mellonella model can be easily accessed, is large enough to be easily operated, and can survive at temperatures similar to mammalian hosts. Such advantages ultimately contribute to its wide acceptance as an invertebrate model for efficacy evaluation of antimicrobial agents (Brennan et al., 2002; Slater et al., 2011). In this study, the survival rate of infected larvae in the drug combination group was significantly improved compared to control group and drug monotherapy groups. Furthermore, the decreased fungal cells of tissues in the drug combination group depicted that the efficacy of FLC could be enhanced by HMH in vivo. The results showed that drug combination treatment was more efficacious than drug monotherapy for C. albicans growth inhibition. This is because the melanized nodules in larvae were less than that found in the drug monotherapy group. Therefore, the in vivo data further confirmed the in vitro antifungal effect of this drug combination and indicated the potential efficacy of this drug combination against resistant C. albicans in vivo.
Azole-resistant isolates commonly display reduced intracellular accumulation of azoles due to their overexpression of drug transport pumps. Functional activities of drug transport pumps have traditionally been assayed with R6G, a known fluorescent substrate of several drug transport pumps responsible for multidrug resistance in yeasts. Both R6G and azoles are the substrate of drug transport pumps. R6G was employed as the fluorescent alternate of azoles in this experiment (Holmes et al., 2012; De Cremer et al., 2015). For further explorations of mechanisms, we performed the R6G uptake and efflux assay to determine whether the synergism of HMH and azoles is relevant to the drug transporters. As a result, we detected no difference in the uptake of R6G between the HMH group and control group. However, HMH was found to significantly decrease the efflux of R6G, demonstrating that it reverses the resistance of resistant C. albicans to azoles by inhibiting the activity of drug transporters.
Besides biofilm, hyphal morphogenesis is considered to be one of the crucial virulence factors associated with the pathogenesis of C. albicans (Naglik et al., 2003; Dalle et al., 2010; Gow et al., 2011). The results showed that HMH combined with FLC has potential synergistic antifungal effects against the hyphae formation of resistant C. albicans. We suspected that hyphae formation, as one of the virulence factors, may be closely associated with drug resistance in C. albicans, and serve as a potent target for this drug combination against resistant C. albicans.
Calcium, as the second messenger in eukaryotic cells, plays a key role in overcoming drug resistance by fungus (Li and Sun, 2016). Hence, clarifying the calcium-related mechanisms of pathogenicity in C. albicans may contribute to the discovery of new targets for the treatment of resistant C. albicans infection. Our study found that HMH combined with FLC can increase [Ca2+]i level of resistant C. albicans, revealing the important role of [Ca2+]i in drug resistance of C. albicans.
ROS play an important physiological role in the survival of C. albicans and ROS generation is a momentous hallmark in fungal apoptosis (Madeo et al., 1999). As a primary cell death regulator, ROS can impact many crucial physiological pathways in fungus (Carmona-Gutierrez et al., 2010). In addition, studies have suggested that ROS production is involved in the antifungal mechanisms utilized by several antifungal agents on C. albicans (Sharma and Srivastava, 2014; Peralta et al., 2015; Lee and Lee, 2018). In the present study, resistant C. albicans exposed to the HMH and FLC drug combination produced a higher ROS level. This result suggested that ROS production could be involved in the antifungal mechanisms of this drug combination on resistant C. albicans. Metacaspases also play a central role in the apoptotic-signaling network of fungi. As metacaspase activity was significantly promoted by the drug combination in resistant C. albicans, the apoptotic process induced by this drug combination in resistant C. albicans was found to be mediated by a metacaspase-dependent apoptotic pathway.
A model for the antifungal mechanisms of HMH combined with FLC is provided in Figure 8. Such combination may exert synergistic antifungal effects on resistant C. albicans via three pathways (Figure 8). When resistant C. albicans cell is exposed to this drug combination, the activity of drug transporters and hyphae formation are both inhibited. Moreover, with stimulation by this combination, [Ca2+]i level is disturbed, ROS increases, and finally, cell apoptosis occurs. Further future studies are needed or the inter-relations of the three pathways in Figure 8.
Conclusion
Our results demonstrate the synergistic effects of HMH and azoles against resistant C. albicans in vitro (planktonic cells and biofilm in the early stage) and in vivo. To add, it suggests that the synergistic antifungal mechanisms may be involved in the inhibition of the efflux of azoles and hyphal growth, and the induction of apoptosis in resistant C. albicans.
Future studies are required on the mechanisms where by the synergistic antifungal effects of HMH and azoles occur.
Data Availability Statement
The datasets analyzed in this manuscript are not publicly available. Requests to access the datasets should be directed to XL, lixiuyun666666@163.com.
Author Contributions
XL and LH designed the experiments and conducted the in vitro and in vivo experiments. XL wrote the manuscript. LH corrected the manuscript. XW and YG conducted the experiments of synergistic mechanisms.
Funding
This study was supported by the Health and Family Planning Commission of Jinan Municipality (2017-2-20), Department of Science and Technology of Shandong Province (2017G006038), and Shandong Health and Family Planning Commission (2018WS490).
Conflict of Interest
The authors declare that the research was conducted in the absence of any commercial or financial relationships that could be construed as a potential conflict of interest.
Acknowledgments
We would like to thank Ke Li, the daughter of XL. This research was conducted during the duration of pregnancy of XL. Ke Li has brought little trouble to her mother and let her mother have enough energy to finish our research. Thank you, our angel baby!
Supplementary Material
The Supplementary Material for this article can be found online at: https://www.frontiersin.org/articles/10.3389/fmicb.2019.02295/full#supplementary-material
References
Brennan, M., Thomas, D. Y., Whiteway, M., and Kavanagh, K. (2002). Correlation between virulence of Candida albicans mutants in mice and Galleria mellonella larvae. FEMS Immunol. Med. Microbiol. 34, 153–157. doi: 10.1016/s0928-8244(02)00374-7
Browne, N., Heelan, M., and Kavanagh, K. (2013). An analysis of the structural and functional similarities of insect hemocytes and mammalian phagocytes. Virulence 4, 597–603. doi: 10.4161/viru.25906
Carmona-Gutierrez, D., Eisenberg, T., Buttner, S., Meisinger, C., Kroemer, G., and Madeo, F. (2010). Apoptosis in yeast: triggers, pathways, subroutines. Cell Death. Differ. 17, 763–773. doi: 10.1038/cdd.2009.219
Chen, D., Su, A., Fu, Y., Wang, X., Lv, X., Xu, W., et al. (2015). Harmine blocks herpes simplex virus infection through downregulating cellular NF-kappaB and MAPK pathways induced by oxidative stress. Antiviral Res. 123, 27–38. doi: 10.1016/j.antiviral.2015.09.003
Cotter, G., Doyle, S., and Kavanagh, K. (2000). Development of an insect model for the in vivo pathogenicity testing of yeasts. FEMS Immunol. Med. Microbiol. 27, 163–169. doi: 10.1016/s0928-8244(99)00185-6
Dalle, F., Wächtler, B., L’Ollivier, C., Holland, G., Bannert, N., Wilson, D., et al. (2010). Cellular interactions ofCandida albicanswith human oral epithelial cells and enterocytes. Cell Microbiol. 12, 248–271. doi: 10.1111/j.1462-5822.2009.01394.x
De Cremer, K., Staes, I., Delattin, N., Cammue, B. P., Thevissen, K., and De Brucker, K. (2015). Combinatorial drug approaches to tackle Candida albicans biofilms. Expert Rev. Anti Infect. Ther. 13, 973–984. doi: 10.1586/14787210.2015.1056162
Egusa, H., Doi, M., Saeki, M., Fukuyasu, S., Akashi, Y., Yokota, Y., et al. (2011). The small molecule harmine regulates NFATc1 and Id2 expression in osteoclast progenitor cells. Bone 49, 264–274. doi: 10.1016/j.bone.2011.04.003
Frost, D., Meechoovet, B., Wang, T., Gately, S., Giorgetti, M., Shcherbakova, I., et al. (2011). beta-carboline compounds, including harmine, inhibit DYRK1A and tau phosphorylation at multiple Alzheimer’s disease-related sites. PLoS One 6:e19264. doi: 10.1371/journal.pone.0019264
Gow, N. A., van de Veerdonk, F. L., Brown, A. J., and Netea, M. G. (2011). Candida albicans morphogenesis and host defence: discriminating invasion from colonization. Nat. Rev. Microbiol. 10, 112–122. doi: 10.1038/nrmicro2711
Hamsa, T. P., and Kuttan, G. (2011). Harmine activates intrinsic and extrinsic pathways of apoptosis in B16F-10 melanoma. Chin. Med. 6:11. doi: 10.1186/1749-8546-6-11
Holmes, A. R., Keniya, M. V., Ivnitski-Steele, I., Monk, B. C., Lamping, E., Sklar, L. A., et al. (2012). The monoamine oxidase a inhibitor clorgyline is a broad-spectrum inhibitor of fungal ABC and MFS transporter efflux pump activities which reverses the azole resistance of Candida albicans and Candida glabrata clinical isolates. Antimicrob. Agents Chemother. 56, 1508–1515. doi: 10.1128/AAC.05706-11
Lee, W., and Lee, D. G. (2018). Reactive oxygen species modulate itraconazole-induced apoptosis via mitochondrial disruption in Candida albicans. Free Radic. Res. 52, 39–50. doi: 10.1080/10715762.2017.1407412
Li, X., and Sun, S. (2016). Targeting the fungal calcium-calcineurin signaling network in overcoming drug resistance. Future Med. Chem. 8, 1379–1381. doi: 10.4155/fmc-2016-0094
Li, X., Zhao, Y., Huang, X., Yu, C., Yang, Y., and Sun, S. (2017). Ambroxol hydrochloride combined with fluconazole reverses the resistance of candida albicans to fluconazole. Front. Cell. Infect. Microbiol. 7:124. doi: 10.3389/fcimb.2017.00124
Liu, S., Hou, Y., Chen, X., Gao, Y., Li, H., and Sun, S. (2014). Combination of fluconazole with non-antifungal agents: a promising approach to cope with resistant Candida albicans infections and insight into new antifungal agent discovery. Int. J. Antimicrob. Agents 43, 395–402. doi: 10.1016/j.ijantimicag.2013.12.009
Madeo, F., Frohlich, E., Ligr, M., Grey, M., Sigrist, S. J., Wolf, D. H., et al. (1999). Oxygen stress: a regulator of apoptosis in yeast. J. Cell Biol. 145, 757–767. doi: 10.1083/jcb.145.4.757
Marr, K. (2004). Combination antifungal therapy: where are we now, and where are we going? Oncology 18, 24–29.
Mylonakis, E., Moreno, R., El Khoury, J. B., Idnurm, A., Heitman, J., Calderwood, S. B., et al. (2005). Galleria mellonella as a model system to study Cryptococcus neoformans pathogenesis. Infect. Immun. 73, 3842–3850. doi: 10.1128/iai.73.7.3842-3850.2005
Naglik, J. R., Challacombe, S. J., and Hube, B. (2003). Candida albicans secreted aspartyl proteinases in virulence and pathogenesis. Microbiol. Mol. Biol. Rev. 67, 400–428. doi: 10.1128/mmbr.67.3.400-428.2003
Nenaah, G. (2010). Antibacterial and antifungal activities of (beta)-carboline alkaloids of Peganum harmala (L) seeds and their combination effects. Fitoterapia 81, 779–782. doi: 10.1016/j.fitote.2010.04.004
Odds, F. C. (2003). Synergy, antagonism, and what the chequerboard puts between them. J. Antimicrob. Chemother. 52:1. doi: 10.1093/jac/dkg301
Onishi, Y., Oishi, K., Kawano, Y., and Yamazaki, Y. (2012). The harmala alkaloid harmine is a modulator of circadian bmal1 transcription. Biosci. Rep. 32, 45–52. doi: 10.1042/BSR20110002
Patel, K., Gadewar, M., Tripathi, R., Prasad, S. K., and Patel, D. K. (2012). A review on medicinal importance, pharmacological activity and bioanalytical aspects of beta-carboline alkaloid “Harmine”. Asian Pac. J. Trop. Biomed. 2, 660–664. doi: 10.1016/S2221-1691(12)60116-6
Peralta, M. A., da Silva, M. A., Ortega, M. G., Cabrera, J. L., and Paraje, M. G. (2015). Antifungal activity of a prenylated flavonoid from Dalea elegans against Candida albicans biofilms. Phytomedicine 22, 975–980. doi: 10.1016/j.phymed.2015.07.003
Pfaller, M. A., Diekema, D. J., Ostrosky-Zeichner, L., Rex, J. H., Alexander, B. D., Andes, D., et al. (2008). Correlation of MIC with outcome for Candida species tested against caspofungin, anidulafungin, and micafungin: analysis and proposal for interpretive MIC breakpoints. J. Clin. Microbiol. 46, 2620–2629. doi: 10.1128/JCM.00566-08
Pu, S., Niu, S., Zhang, C., Xu, X., Qin, M., Huang, S., et al. (2017). Epidemiology, antifungal susceptibilities, and risk factors for invasive candidiasis from 2011 to 2013 in a teaching hospital in southwest China. J. Microbiol. Immunol. Infect. 50, 97–103. doi: 10.1016/j.jmii.2015.01.005
Reus, G. Z., Stringari, R. B., Goncalves, C. L., Scaini, G., Carvalho-Silva, M., Jeremias, G. C., et al. (2012). Administration of harmine and imipramine alters creatine kinase and mitochondrial respiratory chain activities in the rat brain. Depress. Res. Treat. 2012:987397. doi: 10.1155/2012/987397
Scorzoni, L., de Lucas, M. P., Mesa-Arango, A. C., Fusco-Almeida, A. M., Lozano, E., Cuenca-Estrella, M., et al. (2013). Antifungal efficacy during Candida krusei infection in non-conventional models correlates with the yeast in vitro susceptibility profile. PLoS One 8:e60047. doi: 10.1371/journal.pone.0060047
Sharma, A., and Srivastava, S. (2014). Anti-Candida activity of two-peptide bacteriocins, plantaricins (Pln E/F and J/K) and their mode of action. Fungal Biol. 118, 264–275. doi: 10.1016/j.funbio.2013.12.006
Slater, J. L., Gregson, L., Denning, D. W., and Warn, P. A. (2011). Pathogenicity of Aspergillus fumigatus mutants assessed in Galleria mellonella matches that in mice. Med. Mycol. 49(Suppl. 1), S107–S113. doi: 10.3109/13693786.2010.523852
Uppuluri, P., Chaturvedi, A. K., Srinivasan, A., Banerjee, M., Ramasubramaniam, A. K., Kohler, J. R., et al. (2010). Dispersion as an important step in the Candida albicans biofilm developmental cycle. PLoS Pathog. 6:e1000828. doi: 10.1371/journal.ppat.1000828
Vila, T. V., Ishida, K., de Souza, W., Prousis, K., Calogeropoulou, T., and Rozental, S. (2013). Effect of alkylphospholipids on Candida albicans biofilm formation and maturation. J. Antimicrob. Chemother. 68, 113–125. doi: 10.1093/jac/dks353
Wang, T., Shi, G., Shao, J., Wu, D., Yan, Y., Zhang, M., et al. (2015). in vitro antifungal activity of baicalin against Candida albicans biofilms via apoptotic induction. Microb. Pathog. 87, 21–29. doi: 10.1016/j.micpath.2015.07.006
Wisplinghoff, H., Bischoff, T., Tallent, S. M., Seifert, H., Wenzel, R. P., and Edmond, M. B. (2004). Nosocomial bloodstream infections in US hospitals: analysis of 24,179 cases from a prospective nationwide surveillance study. Clin. Infect. Dis. 39, 309–317. doi: 10.1086/421946
Yapar, N. (2014). Epidemiology and risk factors for invasive candidiasis. Therapeut. Clin. Risk Manag. 10, 95–105. doi: 10.2147/TCRM.S40160
Yonezawa, T., Hasegawa, S., Asai, M., Ninomiya, T., Sasaki, T., Cha, B. Y., et al. (2011). Harmine, a beta-carboline alkaloid, inhibits osteoclast differentiation and bone resorption in vitro and in vivo. Eur. J. Pharmacol. 650, 511–518. doi: 10.1016/j.ejphar.2010.10.048
Keywords: harmine hydrochloride, azoles, resistant Candida albicans, Galleria mellonella, synergistic mechanism
Citation: Li X, Wu X, Gao Y and Hao L (2019) Synergistic Effects and Mechanisms of Combined Treatment With Harmine Hydrochloride and Azoles for Resistant Candida albicans. Front. Microbiol. 10:2295. doi: 10.3389/fmicb.2019.02295
Received: 29 July 2019; Accepted: 20 September 2019;
Published: 15 October 2019.
Edited by:
Tamás Papp, University of Szeged, HungaryReviewed by:
Ashutosh Singh, University of Lucknow, IndiaJon Y. Takemoto, Utah State University, United States
Copyright © 2019 Li, Wu, Gao and Hao. This is an open-access article distributed under the terms of the Creative Commons Attribution License (CC BY). The use, distribution or reproduction in other forums is permitted, provided the original author(s) and the copyright owner(s) are credited and that the original publication in this journal is cited, in accordance with accepted academic practice. No use, distribution or reproduction is permitted which does not comply with these terms.
*Correspondence: Lina Hao, aGFvbGluYTE5ODRAMTYzLmNvbQ==