- 1State Key Laboratory for Diagnosis and Treatment of Infectious Diseases, National Clinical Research Center for Infectious Diseases, The First Affiliated Hospital, College of Medicine, Zhejiang University, Hangzhou, China
- 2Collaborative Innovation Center for Diagnosis and Treatment of Infectious Diseases, Zhejiang University, Hangzhou, China
Inflammatory bowel diseases (IBDs) develop as a result of complex interactions among genes, innate immunity and environmental factors, which are related to the gut microbiota. Multiple clinical and animal data have shown that Akkermansia muciniphila is associated with a healthy mucosa. However, its precise role in colitis is currently unknown. Our study aimed to determine its protective effects and underlying mechanisms in a dextran sulfate sodium (DSS)-induced colitis mouse model. Twenty-four C57BL/6 male mice were administered A. muciniphila MucT or phosphate-buffered saline (PBS) once daily by oral gavage for 14 days. Colitis was induced by drinking 2% DSS from days 0 to 6, followed by 2 days of drinking normal water. Mice were weighed daily and then sacrificed on day 8. We found that A. muciniphila improved DSS-induced colitis, which was evidenced by reduced weight loss, colon length shortening and histopathology scores and enhanced barrier function. Serum and tissue levels of inflammatory cytokines and chemokines (TNF-α, IL1α, IL6, IL12A, MIP-1A, G-CSF, and KC) decreased as a result of A. muciniphila administration. Analysis of 16S rDNA sequences showed that A. muciniphila induced significant gut microbiota alterations. Furthermore, correlation analysis indicated that pro-inflammatory cytokines and other injury factors were negatively associated with Verrucomicrobia, Akkermansia, Ruminococcaceae, and Rikenellaceae, which were prominently abundant in A. muciniphila-treated mice. We confirmed that A. muciniphila treatment could ameliorate mucosal inflammation either via microbe-host interactions, which protect the gut barrier function and reduce the levels of inflammatory cytokines, or by improving the microbial community. Our findings suggest that A. muciniphila may be a potential probiotic agent for ameliorating colitis.
Introduction
Inflammatory bowel diseases (IBDs), including ulcerative colitis (UC) and Crohn’s disease (CD), have developed into a global scourge with an increasing incidence and prevalence worldwide in the last 50 years (Kaplan, 2015; Parada Venegas et al., 2019). IBDs were convincingly shown to develop as a result of complex interactions among genes, innate immunity and environmental factors (Parada Venegas et al., 2019). Intriguingly, gut microbiota, relevant to the mucosal immunity and host metabolism, is likely the most crucial environmental factors in IBD pathogenesis. Multiple studies have confirmed that gut microbiota plays an key role in the severity and progression of colitis in IBD patients (Marion-Letellier et al., 2016). Although there is a strong linkage between the gut microbiota and IBDs, the exact mechanisms of the interactions are still unclear (Von Schillde et al., 2012).
Dextran sulfate sodium (DSS) administration is a chemical method to induce colitis, which results in similar key clinical and pathological features of IBDs to those in humans (Okayasu et al., 1990). DSS administration is believed to impair intestinal epithelial cells due to its toxicity. Consequently, the destroyed barrier function allows the immune cells to be exposed to antigens (e.g., microbes), resulting in a subsequent immune response (Wirtz et al., 2017). Based on the properties of colitis, this method is suitable to investigate the impacts of the intestinal microbiota on colitis progression (Hudcovic et al., 2001; Hernandez-Chirlaque et al., 2016). For instance, a recent study revealed that some specific bacterial taxa were present in the crypts due to the functions of NOD-like receptor protein 6 (NLRP6), which are associated with the severity of colitis (Elinav et al., 2011). Moreover, DSS-induced colitis has been broadly applied as a model for its simplicity and reproducibility.
Akkermansia muciniphila has been identified as the representative of the phylum Verrucomicrobia and represents 1–5% of the human intestinal microbial community (Collado et al., 2007; Derrien et al., 2008). A. muciniphila, as a gram-negative and strictly anaerobic bacterium, tends to widely colonize the nutrient-rich intestinal mucus layer (Derrien et al., 2004). Studies have demonstrated that A. muciniphila is able to degrade host mucin into various products (e.g., short chain fatty acids) to regulate host biological functions, such as glucose and lipid metabolism, and to modulate the expression of mucus-related genes, which participate in host immune responses (Derrien et al., 2004, 2011).
A. muciniphila was confirmed to exert a major role in maintaining gut barrier function, host metabolism and other biological functions through interactions between intestinal microbes and host in metabolic disorders (Everard et al., 2013). Moreover, Png et al. (2010) observed that the abundance of A. muciniphila was markedly reduced in patients with IBD compared with the abundance in controls, indicating that A. muciniphila may be related to the health of the intestinal mucosa. These findings indicate that A. muciniphila might play a crucial role in protecting against intestinal injury, which occurs in IBD. However, the precise protective roles A. muciniphila plays and the underlying mechanisms during the progression of the disease are not completely understood. Therefore, our study aimed to investigate the protective effects of A. muciniphila against colitis and the potential underlying mechanisms in a DSS-induced colitis mouse model.
Materials and Methods
Bacterial Strains and Growth Conditions
A. muciniphila MucT (ATTC BAA835) was cultured in a basal mucin-based brain heart Infusion medium (OXOID, Thermo Fisher Biochemicals Ltd., Baesingstoke, United Kingdom) at 37°C for 48 h under an anaerobic environment, as previously reported (Derrien et al., 2004). The cultures were centrifuged (8000 × g for 10 min at 4°C), washed with sterile phosphate buffer saline (PBS, pH 7.2) twice, and then concentrated to the final concentration of 1.5∗1010 colony-forming units (CFU)/ml by measuring absorbance at 630 nm (O.D. range from 0.6 to 0.8) for further use. The experiments described above were performed under strict anaerobic conditions.
Animals
All animal experiments were reviewed and approved by the Local Committee of Animal Use and Protection. Specific pathogen-free (SPF) male C57BL/6 mice aged 5 weeks were initially obtained from Shanghai Laboratory Animal, Co., Ltd. (SLAC). All mice were conventionally raised under SPF conditions, and the experiments were performed when the mice were 6–7 weeks old.
DSS-Induced Colitis and Experimental Design
Twenty-four mice were randomly assigned to three groups (n = 8 each): normal control group (CP group), experimental model group (DP group) and A. muciniphila group (AKK group). Mice in the AKK group (DSS + A. muciniphila) were administered 0.2 ml A. muciniphila (3∗109 CFU) once daily by oral gavage for 14 days (day -7 to day 7, Figure 1A), while mice in the DP group (DSS + PBS) and the CP group (Water + PBS) were given equal amounts of anaerobic sterile PBS. On day 0, the mice in the AKK group and the DP group were administered 2% (wt/vol) DSS (molecular weight: 36,000–50,000; MP Biomedicals, cat. no. 0216011080) dissolved in drinking water for 7 days (days 0–6) followed by 2 days of normal water. New fresh DSS solutions were prepared every 2nd day. Meanwhile, the mice in the CP group were given normal drinking water for 9 days (days 0–8). Mouse weights were recorded throughout the experiment and fecal samples were collected on days 0 and 8. At the end of the experiment (day 8), all mice were humanely euthanized after serum and colon segment collection (Figure 1A). Feces and serum were stored at −80°C until analysis. Colon segments were either kept in 10% neutral-buffered formalin or stored at −80°C for further procedures.
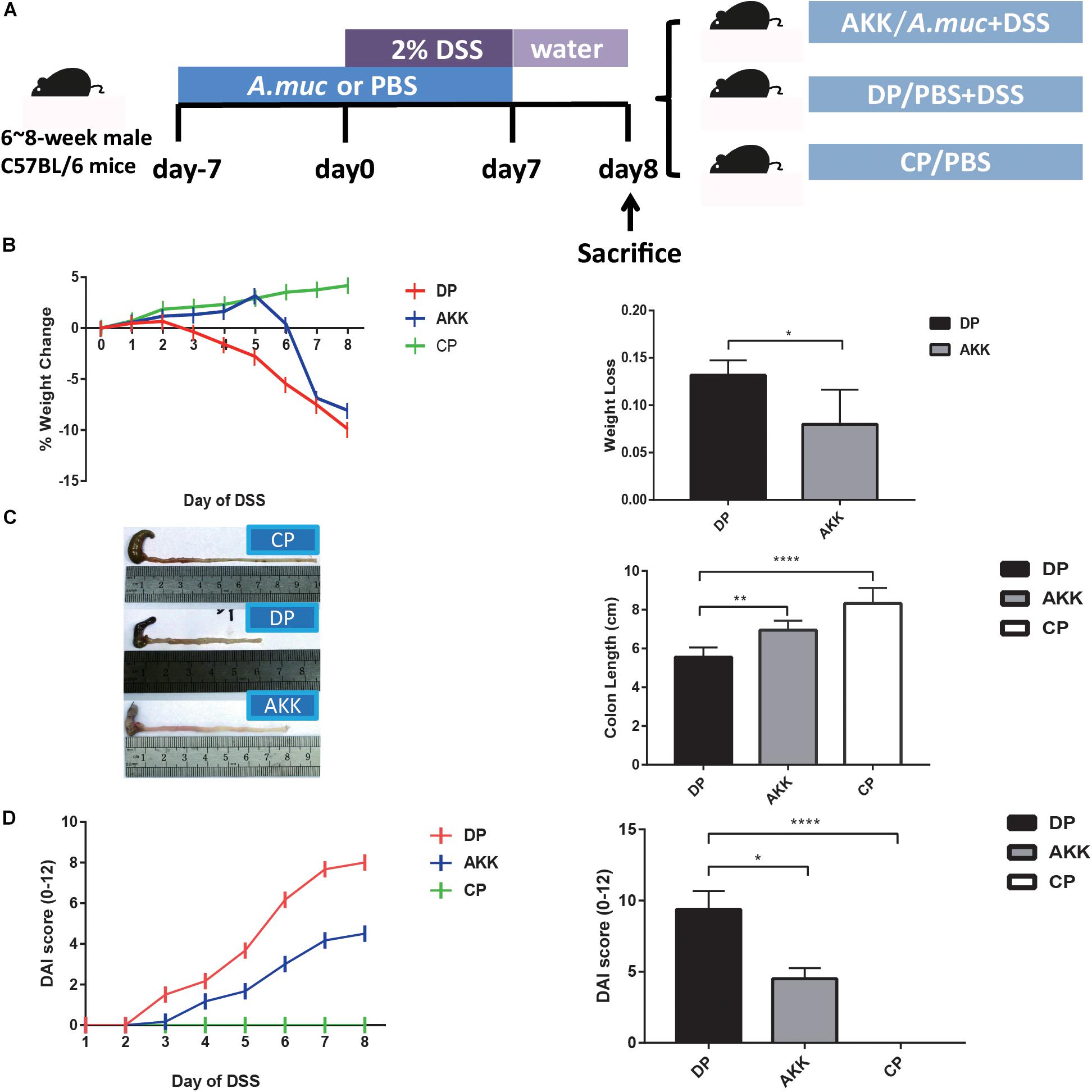
Figure 1. Akkermansia muciniphila alleviated DSS-induced colon injury (A) Scheme of the animal experimental design. Mice were assigned to three groups randomly (Group CP, n = 8; Group DP, n = 8; Group AKK, n = 8). Mice were continuously administered A. muciniphila or PBS for 14 days (day -7 to day 7). Colitis was induced by drinking 2% DSS from days 0 to 6, followed by 2 days (days 7–8) of normal drinking water. On day 8, mice were sacrificed. (B) The percentage of mouse weight change from days 0 to 8 (left) and the total weight loss relative to the baseline (day 0) (right). (C) Representative colons of three groups (left) and the colon length after DSS administration (right). (D) The disease activity index (DAI) scores calculated with time (left) and the bar chart represents the DAI scores among the groups at day 8 (right). Data are shown as the mean ± SEM. ∗P < 0.05; ∗∗P < 0.01; ****P < 0.0001 by unpaired t-tests, Kruskal–Wallis tests and post hoc one-way ANOVA.
Disease Activity Index (DAI) Assessment and Severity of Colitis
The severity of colitis was measured using DAI assessment, a scoring system which includes three parts: body weight loss (0–4), degree of intestinal bleeding (0–4) and stool consistency (0–4) (Wirtz et al., 2017). The colon length, another indicator of colitis severity, was also measured when the mice were sacrificed.
Histopathological and Immunofluorescence Analysis
Distal colon segments in the same defined region were collected carefully and were transferred immediately to 10% neutral-buffered formalin. After paraffin embedding, colon fragments were generated into 5 μm cross-sections. The sections were stained with hematoxylin and eosin (H&E) and then scanned by a NanoZoomer Digital Pathology system (Hamamatsu Photonics, KK, Japan) for further histopathological analysis. At least three slides were randomly selected and observed by a blinded pathologist. The histological activity index (HAI) is an established scoring system based on inflammatory cell infiltration (1–3), both in terms of severity and extent, and intestinal architecture (1–3), which includes epithelial changes and mucosal architecture, as previously described (Erben et al., 2014).
The colon sections were stained with the intestinal barrier markers ZO-1 and Occludin (both Proteintech, Rosemont, IL, United States) with a previously described standard immunofluorescence analysis process (Chung et al., 2014). Images were captured and visualized using a Zeiss LSM T-PMT confocal microscope (Zeiss, Jena, Germany).
Intestinal Permeability
Intestinal permeability was determined by the fluorescein isothiocyanate conjugated dextran (FITC-dextran) assay. Mice were fasted overnight and were administered FITC-dextran (3–5 kDA, Sigma-Aldrich, 60 mg/100 g body weight) by oral gavage 4 h before blood collection. The concentration of FITC was determined by spectrophoto fluorometry (490/525 nm).
Serum Parameter Analysis
Lipopolysaccharide (LPS)-binding protein (LBP) concentrations, an indicator of endotoxin levels, were measured using an appropriate ELISA kit (Guduo, Shanghai, China) as previously reported (Ye et al., 2018).
A Bio-Plex Pro mouse cytokine 23-plex assay kit (Bio-Rad, Hercules, CA, United States) were performed to analyze the serum cytokine levels according to the manufacturer’s instructions. The 23-plex contains the following antibodies specific for cytokines and chemokines: interleukin (IL)-1α,IL-1β, IL-2, IL-3, IL-4, IL-5, IL-6, IL-9, IL-10, IL-12 (p40), IL-12 (p70), IL-13, IL-17, Interferon (IFN)-γ, tumor necrosis factor alpha (TNF-α), eotaxin, granulocyte colony-stimulating factor (G-CSF), granulocyte-macrophage-colony-stimulating factor (GM-CSF), keratinocyte-derived chemokine (KC), macrophage chemoattractant protein 1 (MCP-1), macrophage inflammatory protein (MIP)-1α, MIP-1β and regulated upon activation, normal T-cell expressed and secreted (RANTES).
Determination of Colonic mRNA Expression
An RNeasy Plus Mini Kit (Qiagen, Valencia, CA, United States) was used to extract total colon tissue RNA according to the manufacturer’s procedures. The reverse transcription for two-step reverse transcription polymerase chain reaction (RT-PCR) was performed using a PrimeScript RT Master Mix reverse transcription reagent kit (Takara Biomedicals, Kusatsu, Japan). The relative mRNA expression was measured and analyzed by a ViiA7 real-time PCR system (Applied Biosystems) using a SYBR Premix Ex Taq II (Tli RNase H Plus) reagent (Takara Biomedicals, Kusatsu, Japan). All samples were tested in duplicate and assessed using the 2–ΔΔCT method before the results were normalized to the expression of β-actin. The final results of the AKK and DP groups were calculated relative to the CP group. Primer pairs used are presented in Supplementary Table S1.
Fecal Microbial Community Analysis
Fecal samples were immediately frozen and stored at −80°C freezer after collection. Total bacterial DNA was extracted from fecal samples (approximately 200 mg) using a QIAamp Fast DNA Stool Mini Kit (Qiagen, Hilden, Germany) following the manufacturer’s procedures. The V3–V4 regions of the bacterial 16S rRNA were amplified by Phusion High-Fidelity PCR Master Mix (New England Biolabs) using the specific primer (e.g., 16S V4:515F-806R) with a barcode. After PCR product purification, sequencing libraries, were generated using Ion Plus Fragment Library Kit 48 rxns (Thermo Scientific) following the manufacturer’s instructions, The library quality was assessed on the Qubit@2.0 Fluorometer (Thermo Scientific). At last, the library was sequenced was on an Ion S5TM XL platform (Thermo Scientific) and 400 bp/600 bp single-end reads were generated. Clean reads were finally obtained after single-end read quality controlling procedures. Sequences analyses were performed by Uparse software (Uparse v7.0.1001) (Edgar, 2013). Sequences with ≥97% similarity were assigned to the same OTUs. Representative sequence for each OUT was screened for further annotation.
Taxonomic information was annotated based on the Silva Database1 for each representative sequence. Alpha diversity indices, including Chao1, ACE, Shannon and Simpson, were calculated with QIIME (Version 1.7.0). Principal coordinate analysis (PCoA) was performed by R software (version 2.15.3) based on weighted UniFrac distances. Linear discriminant analysis (Yatsunenko et al., 2012) effect size measurement (LefSe) analysis was conducted online (Bajaj et al., 2014). Spearman’s rank correlation test was performed to analyze the relationships between the microbiota and colitis-related indices in AKK group and DP group using SPSS version 20.0 (SPSS, Inc., Chicago, IL, United States), and Cytoscape was used to visualize the results graphically. Prediction of metabolic functions of the microbiota was performed by using Phylogenetic Investigation of Communities by Reconstruction of Unobserved States (PICRUSt V1.0.0).
Short Chain Fatty Acid Quantification
Short chain fatty acids (SCFAs) were extracted by mixing 20 mg of feces with 500 μl internal standard (hexanoic acid-d3, 10 μg/ml). After homogenization and centrifugation (15,000 rpm, 5 min, 4°C), the supernatant was transferred into an Eppendorf tube with 5% concentrated sulfuric acid (supernatant vs. 5% concentrated sulfuric acid, volume 10:1). Then, an equivalent volume of ethyl acetate was added to the mixture. After centrifugation and incubation at 4°C for 30 min, the supernatant was transferred to chromatographic vials equipped with 150 μl inserts before the gas chromatography mass spectrometry (GC-MS) analysis. The analysis was performed on an Agilent 7890A GC oven coupled to an Agilent 5975C inert mass selective (MS) detector (Agilent Technologies, Santa Clara, CA, United States). SCFAs were identified by the times of retention in accordance with the standard solutions. Standard mixes of 0.01, 0.1, 1, 10, 100, 1000 μl/ml and blank were also prepared in the same way as the samples.
Statistical Analysis
The Kolmogorov-Smirnov test was performed to assess the normality of the data. Unpaired t tests with Welch’s correction were used to determine weight loss difference between the DP and AKK groups. Colon length, serum cytokines, colonic cytokine expression, LBP, Shannon index, relative abundance, FITC-dextran and SCFAs were analyzed by post hoc one-way ANOVA. Kruskal–Wallis tests were performed to analyze the DAI and histopathology score among groups. A multi response permutation procedure (MRPP) test was used to analyze the β diversity in fecal analysis. Correlations analysis was conducted using Spearman’s rank correlation. P < 0.05 were considered significant. All data analyses were performed using SPSS version 20.0 (SPSS, Inc., Chicago, IL, United States) and GraphPad Prism 7.0 (GraphPad Software, Inc.), with a two-tailed P < 0.05 considered significant, and the results are presented as the mean ± SEM.
Results
A. muciniphila Alleviated DSS-Induced Colitis
As reported previously, body weight loss during DSS model establishment can be used as a metric of colitis severity because it is easily measured and is consistent with the inflammatory markers (Llewellyn et al., 2018). The percentage of weight change was measured daily for 9 days relative to their baseline body weight that was recorded on day 0. As Figure 1B shows, the body weights of the mice in the DP group were consistently reduced since day 2, while the mice in the AKK group mice showed a significant improvement in weight loss, although there was an apparent decrease on day 6. At the end of the experiment, reduced body weight loss was observed in the mice in the AKK group compared to the mice in the DP group (8.00 ± 1.49% vs. 13.17 ± 0.64%, P < 0.05). In the colitis model, disease severity is typically associated with colon length shortening due to intestinal inflammation (Wirtz et al., 2017). Following the 7 days of DSS exposure, the colons of the mice were shorter in the DP group than in the CP group (p < 0.0001) and the AKK group (p < 0.01) (Figure 1C). Importantly, administration of DSS induced weight loss and bloody diarrhea in the mice, and these factors are inflammation-associated parameters resembling some clinical features of human UC and can be used to calculate the DAI score. In our research, the score increased with the time of DSS administration and was much higher in the mice in the DP group than in those in the CP group (P < 0.0001) and the AKK group at the end of the experiment (P < 0.05) (Figure 1D).
As far as we know, all chemically induced colitis models, including the oral administration of DSS, the method we used in our experiment, begin with epithelial barrier disruption. H&E colon staining (Figure 2A) showed that DSS-induced colitis resulted in extended ulcerations, destroyed crypts and transmural inflammatory infiltration, with a barely complete mucosal structure. However, in mice treated with A. muciniphila, histological damage was ameliorated, as evidenced by a preserved mucosal architecture showing focal erosions and mild/moderate mucosa inflammatory infiltration. To measurably evaluate the intestinal mucosal architecture (0–3) and inflammatory infiltration (0–3), we used the histopathology score (0–6) system (Erben et al., 2014), and the mice treated with A. muciniphila had a significantly lower score than the mice treated with DSS only (P < 0.05) (Figure 2B).
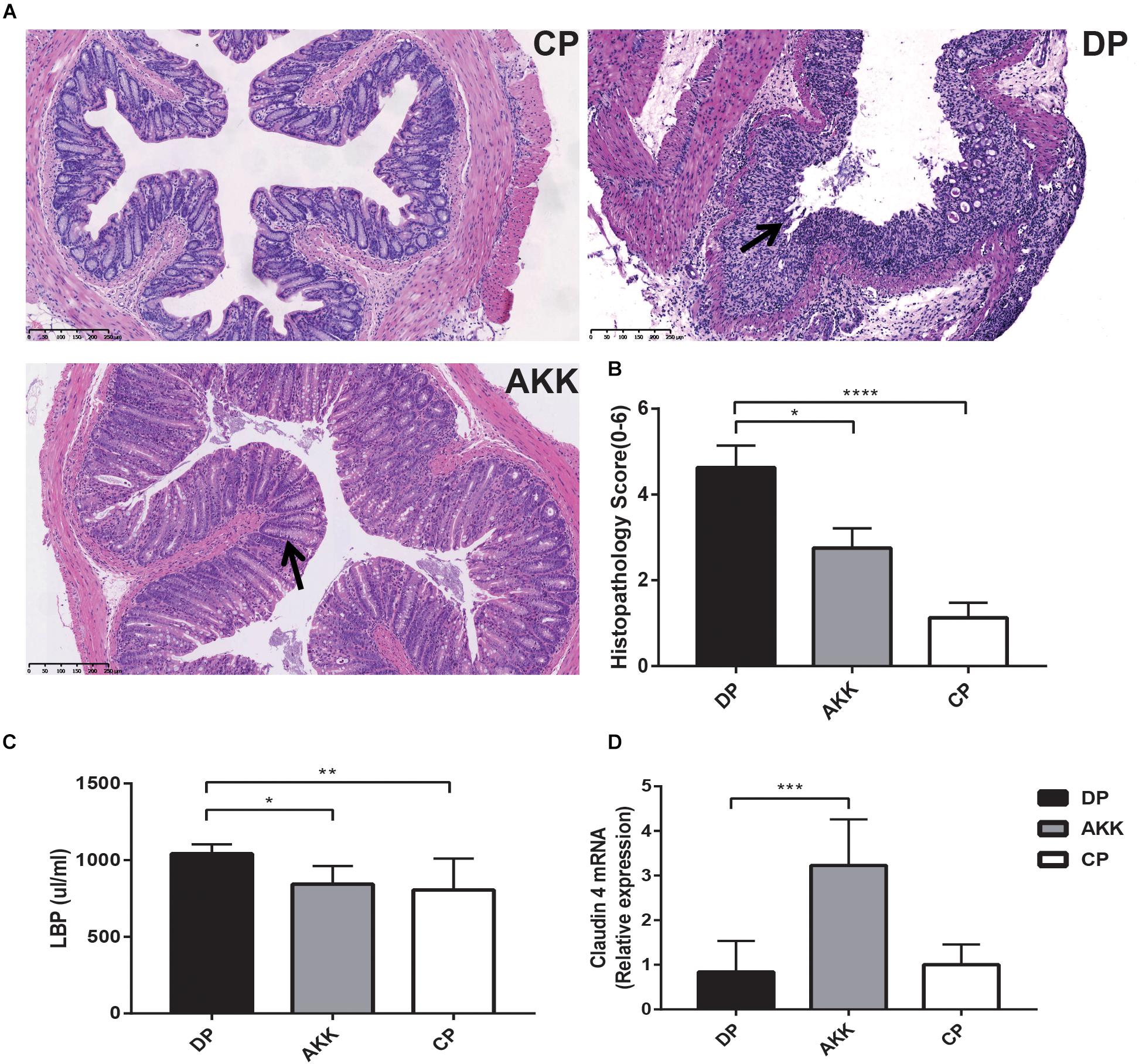
Figure 2. Akkermansia muciniphila ameliorated colon mucosal barrier damage in mice (A) Representative H&E staining of colon tissues of three groups. The black arrows show epithelial damage (scale bar: 100 μm). (B) Histopathology scores (0–6) of colon tissues and colon expression genes of (C) LBP and (D) claudin 4. Data are shown as the mean ± SEM. ∗P < 0.05; ∗∗P < 0.01; ∗∗∗P < 0.001; ****P < 0.0001 by Kruskal–Wallis tests and post hoc one-way ANOVA.
A. muciniphila Ameliorated Colon Mucosal Barrier Damage in Mice
Colon epithelial destruction and inflammatory activity increase the risk of intestinal bacterial translocation, which in turn aggravates colitis severity (Lakatos et al., 2011). Therefore, serum LBP was measured by ELISAs to assess bacterial translocation and colitis activity. The results (Figure 2C) revealed that serum LBP levels in the DP group were higher than the levels in the CP and AKK groups (P < 0.01, P < 0.05, respectively).
Intestinal mucosal barrier destruction was associated with colitis progression; additionally, junction protein expression (occludin, ZO-1, and claudin-4) in the colon was determined by quantitative PCR and immunofluorescence. Claudin-4 mRNA expression was decreased in the colon tissue of the DP group; in contrast, the AKK group had an upregulated level (P < 0.001, Figure 2D). Notably, occludin mRNA expression in the colon was downregulated in the DP group compared with that in the CP group (P < 0.001). However, no significant difference in occludin and ZO-1 mRNA expression was found between the AKK and DP groups (data not shown). As Figure 3A shows, the colon tissue in the DP group presented increased TJ structure defects with destroyed crypts and a disrupted apical region, while A. muciniphila administration stabilized mucosal integrity, resulting in smooth and continuous localization of occludin and ZO-1.
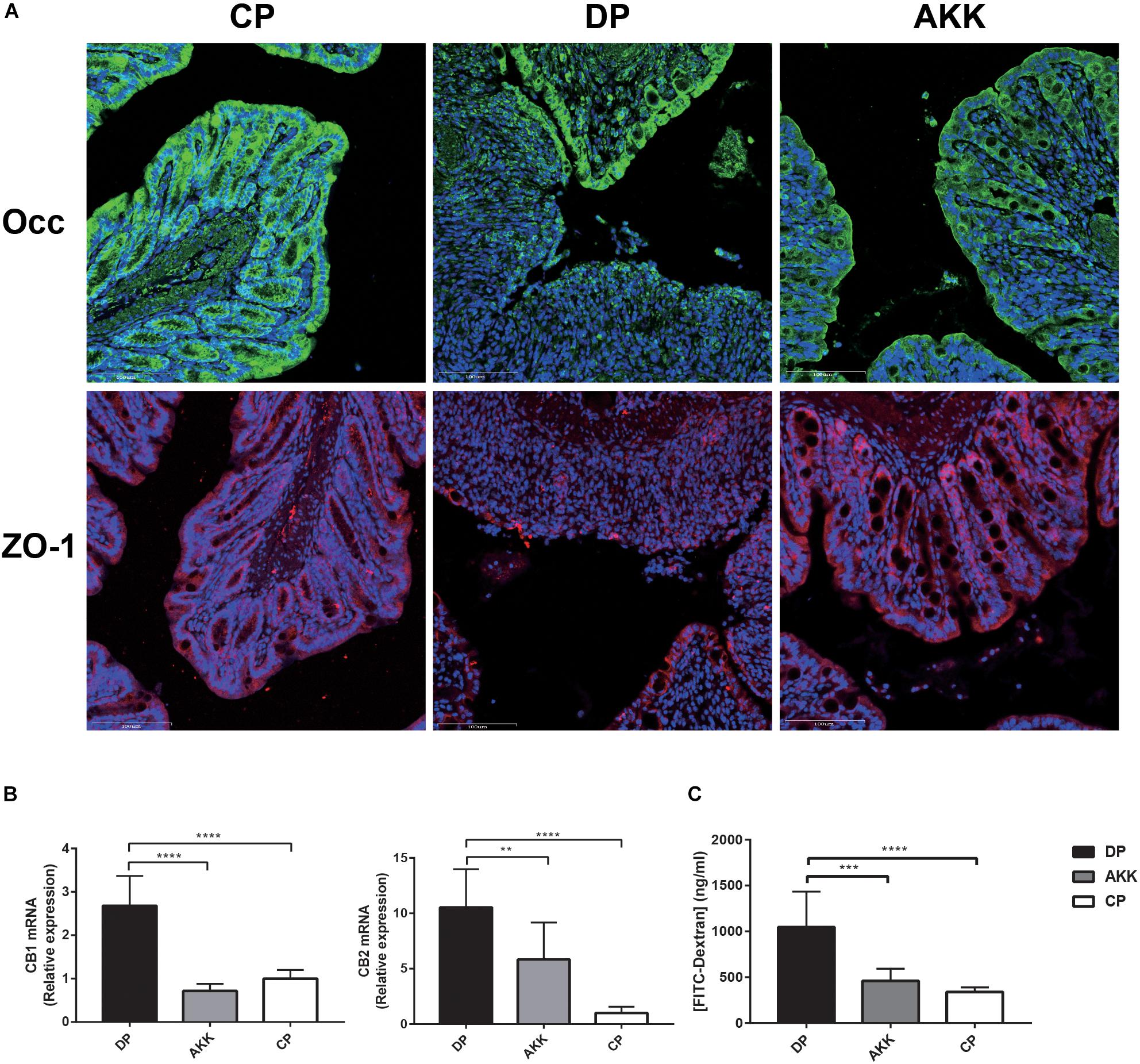
Figure 3. Akkermansia muciniphila enhanced gut barrier function (A) Occludin and ZO-1 immunofluorescence staining of representative colon tissue (scale bar: 100 μm). (B) Colon expression of genes related to gut barrier function, including CB1 and CB2. (C) Intestinal permeability measured by determining the plasma concentration of FITC-dextran. Data are shown as the mean ± SEM. ∗∗P < 0.01; ∗∗∗P < 0.001; ****P < 0.0001 by post hoc one-way ANOVA.
Furthermore, cannabinoid receptor (CB) 1 and CB2 were positively associated with maintaining gut permeability and plasma LPS levels (Muccioli et al., 2010). Consistent with the serum LBP level, the expression of these two markers (CB1 and CB2) was significantly increased after DSS administration (P < 0.0001, p < 0.0001), while the expression was downregulated in mice treated with A. muciniphila (P < 0.0001, P < 0.01, Figure 3B). Notably, FITC-dextran analysis showed a decreased intestinal permeability in mice of the AKK group compared to those of the DP group, suggesting an increased barrier function following A. muciniphila treatment (P < 0.001, Figure 3C).
A. muciniphila Treatment Resulted in Systemic and Intestinal Anti-inflammation
Colon inflammatory cell infiltration increases mucosal production of cytokines. Pro-inflammatory cytokines play an crucial role in the progression of DSS-induced colitis (Andlujar et al., 2011). To explore whether A. muciniphila treatment could alleviate colitis by regulating inflammation, we detected the serum cytokines and cytokine expression in distal colon tissue. An increase in serum cytokines, including the pro-inflammatory cytokines TNF-α, IL1α, IL6, and IL12A, as well as the chemokines MIP-1A, G-CSF, and KC, was observed in mice administered DSS only (DP vs. CP, Figure 4A). In the AKK group, these cytokines were significantly downregulated (P < 0.05, P < 0.001, P < 0.05, P < 0.0001, P < 0.0001, P < 0.0001, P < 0.05, respectively), and their levels were similar to those in the CP group. In addition, similar results were found in colon tissue inflammatory expression (Figure 4B). The expression levels of TNF-α (P < 0.01), IL12A (P < 0.01), and INFγ (P < 0.05) were significantly upregulated in the DP group compared to those observed in the CP group. In contrast, TNF-α (P < 0.05), IL12A (P < 0.05), and INFγ (P < 0.05) were downregulated compared with the levels in the DP group and were almost reduced to the normal level after administration of A. muciniphila. IL1α, IL6, and MIP-1A showed similar decreasing trends (data not shown). Furthermore, in mice treated with A. muciniphila, the immunomodulatory cytokine IL10 expression level was increased both in the serum (P < 0.05, Figure 3A) and colon tissue (P < 0.05, Figure 3B). The remaining serum cytokines tested are shown in the Supplementary Figure S1.
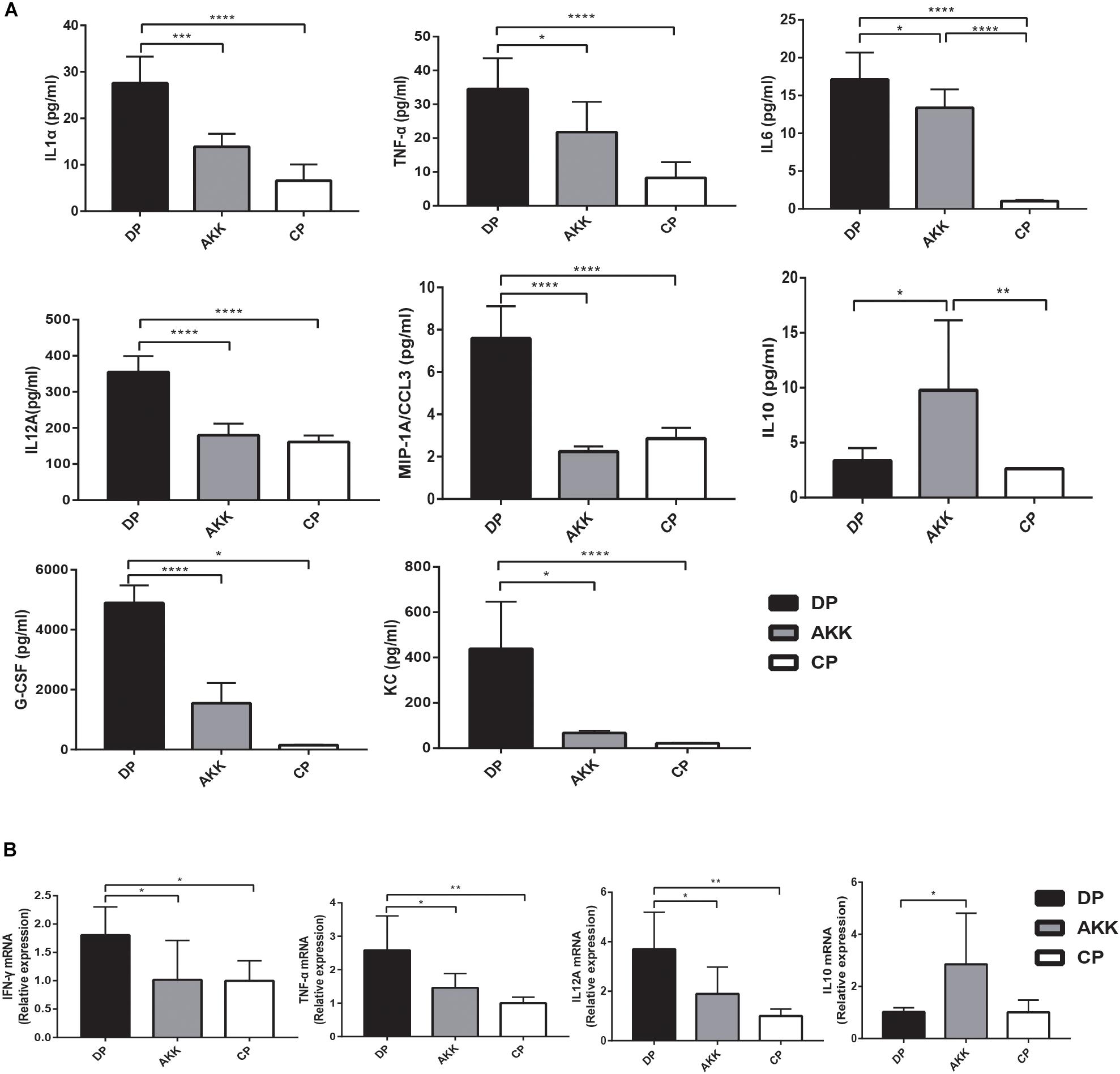
Figure 4. Akkermansia muciniphila exhibited systemic and intestinal anti-inflammatory effects. (A) Bar charts represent the serum cytokine levels of IL-1α, TNF-α, IL6, IL12A, MIP-1A, and IL10 among the groups. (B) Colonic cytokine expression of TNF-α, IL12A, IFNγ, and IL10. Data are shown as the mean ± SEM. ∗P < 0.05; ∗∗P < 0.01; ∗∗∗P < 0.001 by post hoc one-way ANOVA.
A. muciniphila Alleviated DSS-Induced Microbiome Dysbiosis and Reshaped the Gut Microbiota Community
Gut microbes are an important factor in intestinal inflammation (Tamboli et al., 2004; Sartor and Wu, 2017). To further explore the protective impact of A. muciniphila supplementation on mice with DSS-induced colitis, 16S rRNA sequencing analysis was conducted to assess alterations in the gut microbiota. The sequencing data have been deposited in the sequence read archive (SRA) database with the accession PRJNA 54980. Fecal samples were collected from three groups (CP group = 6, DP group = 6, AKK group = 6) after (day 8) DSS administration. Rarefaction curves of three groups reflected the sequencing depth of the fecal analysis (Supplementary Figure S2). A total of 1,285,692 clean reads (AKK = 431,984, CP = 427,244, DP = 426,464) were obtained for further analysis and 11,982 identified OTUs (AKK = 3,853, CP = 4,530, DP = 3,599) were clustered with a 97% similarity cutoff. In contrast to the CP group, the fecal microbiome of the mice administered DSS (DP group) exhibited reduced α-diversity, which was reflected in the decrease in observed species (P < 0.01) and the Ace (P < 0.001), Chao1 (P < 0.01), and Shannon (P < 0.01) indices (data not shown). The Shannon index, an estimator used to identify community diversity, was observed to be significantly higher in mice treated with A. muciniphila than those not treated with A. muciniphila (P < 0.05, AKK vs. DP, Figure 5B). Next, we performed weighted UniFrac PCoA analysis to investigate the differences in species complexity and structural alterations in gut microbial communities (β diversity). The results revealed significant different β diversity among three groups (Figure 5A and Supplementary Table S2). The DP group samples were separated from the CP group samples (P = 0.002, Supplementary Table S2). Likewise, the microbiota of the AKK and DP group samples were clearly separated into two clusters (P = 0.002, Supplementary Table S2), demonstrating that continuous administration of A. muciniphila alleviated DSS-induced dysbiosis of the gut microbiome.
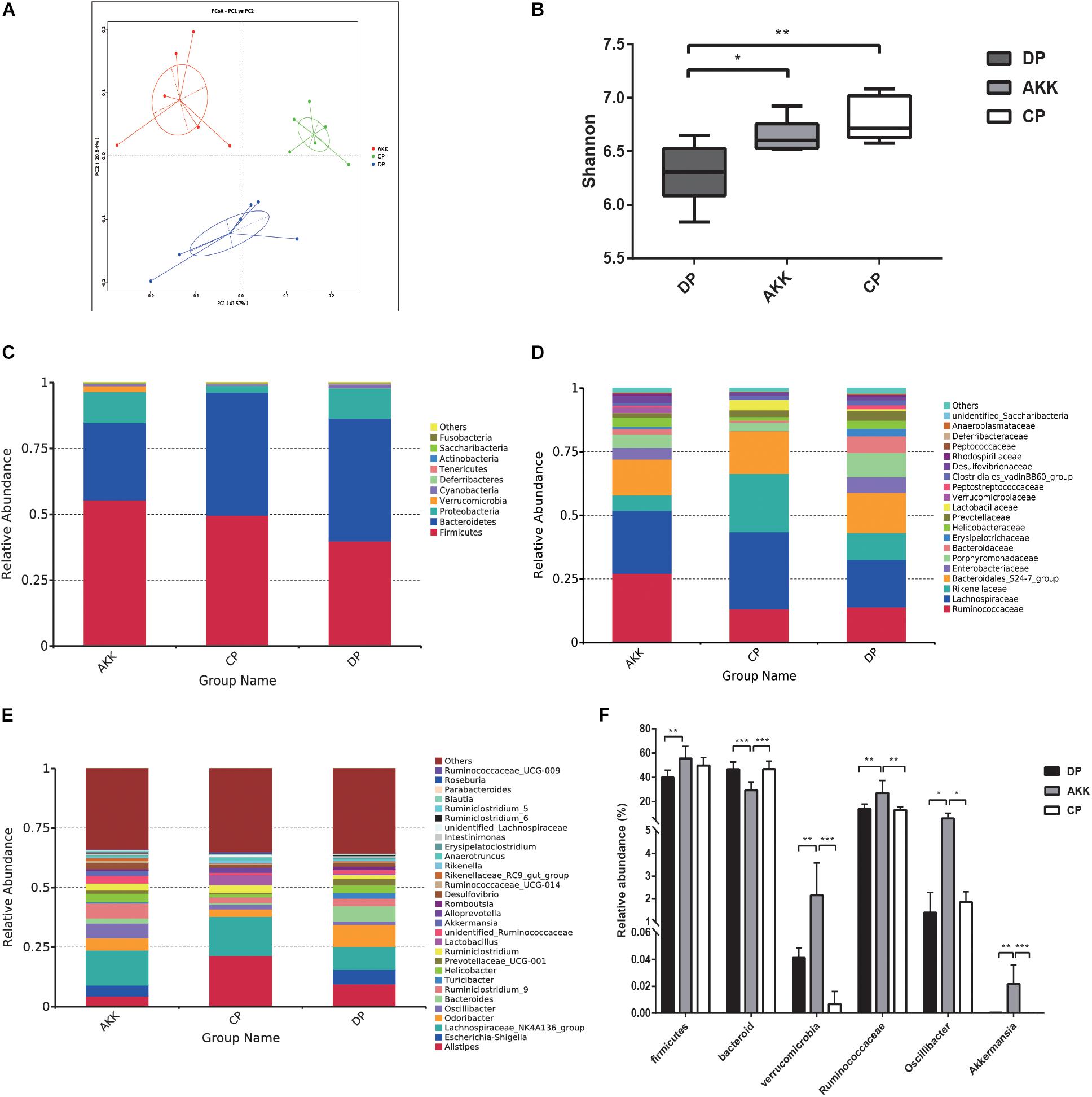
Figure 5. A. muciniphila alleviated DSS-induced microbiome dysbiosis. (A) PCoA analysis among three groups based on weighted UniFrac distances. Each plot represents one sample. (B) The Shannon index among three groups. (C–E) Relative abundance of taxa at the phylum (C), family (D) and genus (E) levels. (F) Relative abundance of taxa in the DP group and AKK group. Data are shown as the mean ± SEM. ∗P < 0.05; ∗∗P < 0.01; ∗∗∗P < 0.001; ****P < 0.0001 by post hoc one-way ANOVA.
The relative abundance of bacteria taxa was altered in individuals with IBD (Zuo and Ng, 2018). Therefore, we used Wilcoxon rank sum tests to compare the relative taxa abundance between the three groups. The top most abundant taxa at the phylum, family and genus levels are shown in Figures 5C–E. Previous studies have clarified that Firmicutes and Bacteroidetes are two dominant phyla in mouse fecal samples (Wu et al., 2017). At the phylum level (Figure 5C), compared to the CP group, the abundance of Firmicutes was decreased and that of Bacteroidetes was increased as a result of DSS oral administration (F/B < 1). In contrast, there was a marked increase in the proportion of Firmicutes (P < 0.01, vs. DP) and a decrease in Bacteroidetes (P < 0.001 vs. DP, P < 0.001 vs. CP) after continuous administration of A. muciniphila. Additionally, the abundance of Verrucomicrobia was also increased due to the treatment (P < 0.001 vs. DP, P < 0.001 vs. CP). At the family level, the relative abundance of Ruminococcaceae (P < 0.01) in the AKK group was increased compared with that in the DP group (Figure 5D), while the relative abundances of Rikenellaceae and Enterobacteriaceae were decreased, although there was no significant difference. At the genus level, the fecal microbiota of the AKK group had a significantly higher abundance of Oscillibacter than that of the DP group (P < 0.05) and CP group (P < 0.05, Figure 5F).
Studies have shown that microbial markers might be instruments that can be used for personalized therapy (Zuo and Ng, 2018). Therefore, we performed LEfSe analysis to explore the prognostic biomarkers from the microbial abundance profiling between the three groups. We found that treatment with A. muciniphila increased the abundance of Clostridia, Firmicutes, Ruminococcaceae, Oscillibacter, Verrucomicrobia, and Akkermansia [LDA score (−log10) > 4.8] and decreased the abundance of Bacteroidetes [LDA score (log10) > 4.8] (Figures 6A,B). Interestingly, due to DSS administration, the fecal flora Enterobacteriales, Escherichia Shigella, and Erysipelotrichales were enriched, and there was a depletion of Lactobacillales, Rikenellaceae, and Lachnospiraceae (Figures 6C,D). Regarding the Kyoto Encyclopedia of Genes and Genomes (KEGG) metabolic pathways, several predicted functional differences were observed between the DP and AKK groups, such as the NOD-like receptor signaling pathway, the glycosaminoglycan degradation pathway, and the lipid metabolism pathway (Supplementary Figure S3).
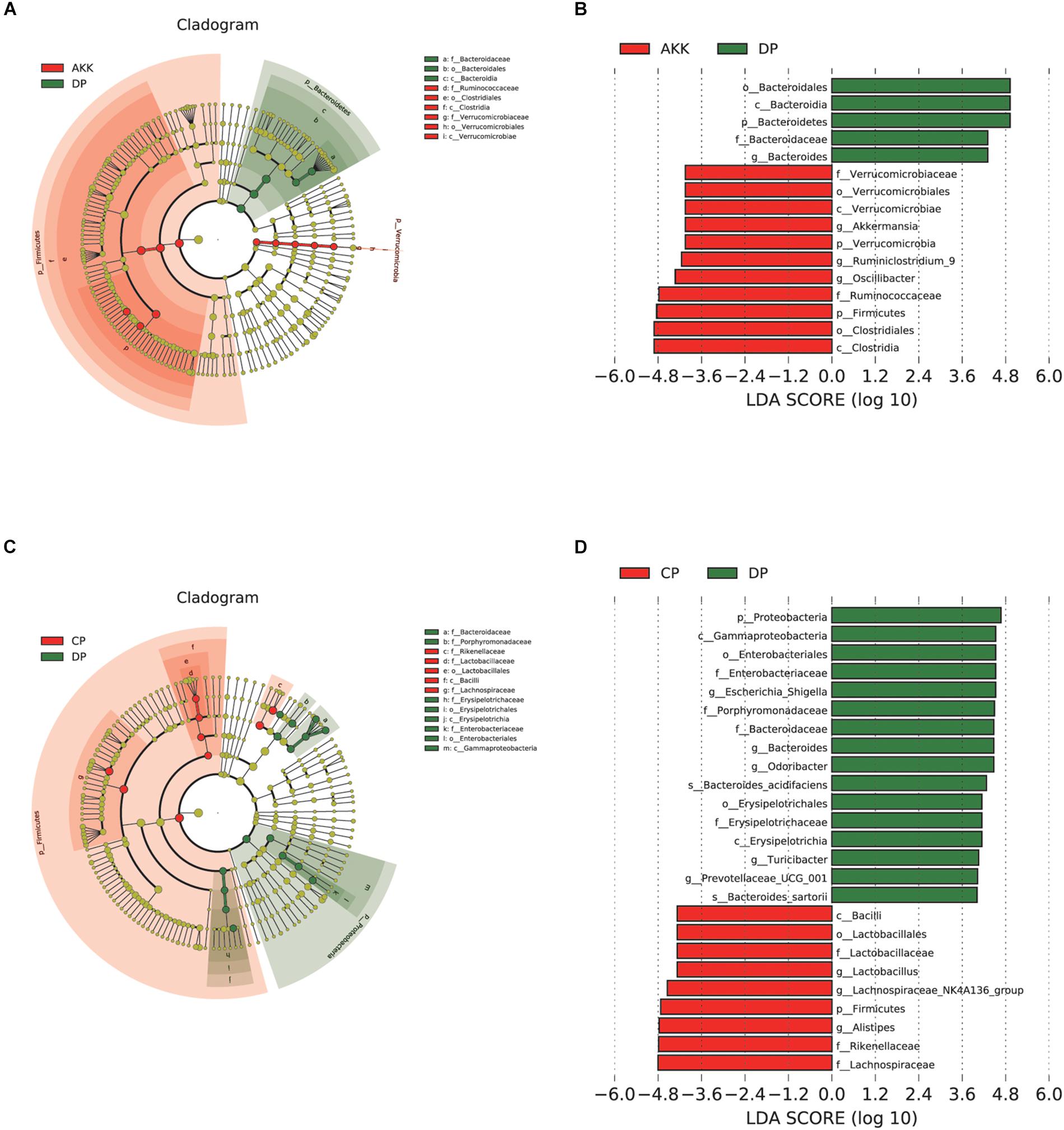
Figure 6. Akkermansia muciniphila reshaped the gut microbiota community. (A) LEfSe cladogram represents the taxa enriched in the AKK group (Red) and DP group (Green). (B) Discriminative biomarkers with an LDA score >4.8 between the AKK group (Red) and DP group (Green). (C) LEfSe cladogram represents taxa enriched in the CP group and DP group. (D) Discriminative biomarkers with an LDA score >4.8 between the CP group (Red) and DP group (Green).
A total of six SCFAs, including acetate acid, propionate acid, isobutyric acid, butyrate acid, 2-metylbutic acid and valeric acid were identified in our study from 24 fecal samples in all three groups. The acetate acid, propionate acid, isobutyric acid and butyrate acid levels were significantly increased in the AKK group (vs. DP group, P < 0.001, P < 0.05, P < 0.0001, and P < 0.05, respectively, Figures 7A–D). However, there was no change observed in 2-metylbutic acid and valeric acid levels with A. muciniphila treatment (Figure 7).
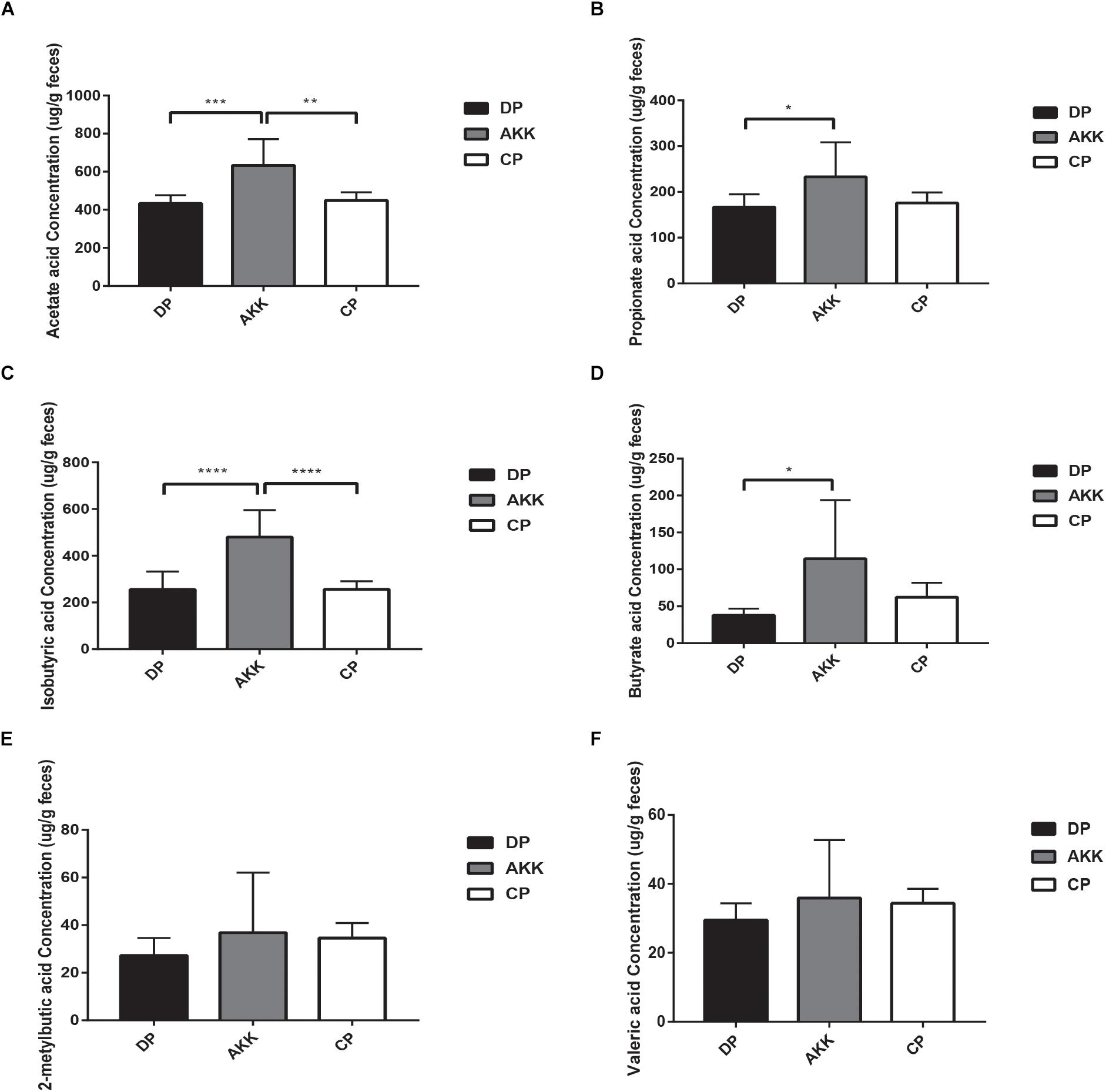
Figure 7. Short-chain fatty acids (SCFAs) distribution observed in three groups. The fecal concentrations of acetate acid (A), propionate acid (B), isobutyric acid (C), butyrate acid (D), 2-metylbutic acid (E), and valeric acid (F) were determined by GC–MS. Data are shown as the mean ± SEM. ∗P < 0.05; ∗∗P < 0.01; ∗∗∗P < 0.001; ****P < 0.0001 by post hoc one-way ANOVA.
Correlation Analysis Indicated That the A. muciniphila-Modified Gut Microbiota Played an Essential Role in the Progression of DSS-Induced Colitis
To verify the relevance of the altered microbial community and the severity of colitis, a correlation analysis between gut bacteria abundance and colitis parameters was performed. In our study, the relative abundances of A. muciniphila-modified bacteria were associated with gut barrier markers and related inflammatory cytokines (Figure 8). As one of the representative colitis severity markers, TNF-α was negatively related to Verrucomicrobia, Ruminococcaceae_UCG-014, and Akkermansia. Additionally, the primary pro-inflammatory cytokine IL12 (p40) was negatively associated with the population densities of Ruminococcaceae_UCG-014, Verrucomicrobia, Akkermansia, and Rikenellaceae_RC9_gut_group. Notably, we found that Verrucomicrobia, was prominently abundant in the AKK group and that it was significantly negatively correlated with all tested gut barrier markers (claudin-4, LBP, and CB1) and inflammatory cytokines, including TNF-α,IFN-γ, IL12 (p40), IL1α, and IL6. In contrast, Bacteroides, which were enriched in the DP group, were positively associated with MIP-1α, IL1α, and LBP. The anti-inflammatory cytokine IL10, upregulated due to A. muciniphila administration as mentioned above, was markedly positively correlated with Verrucomicrobia, Ruminococcaceae_UCG-014, and Akkermansia.
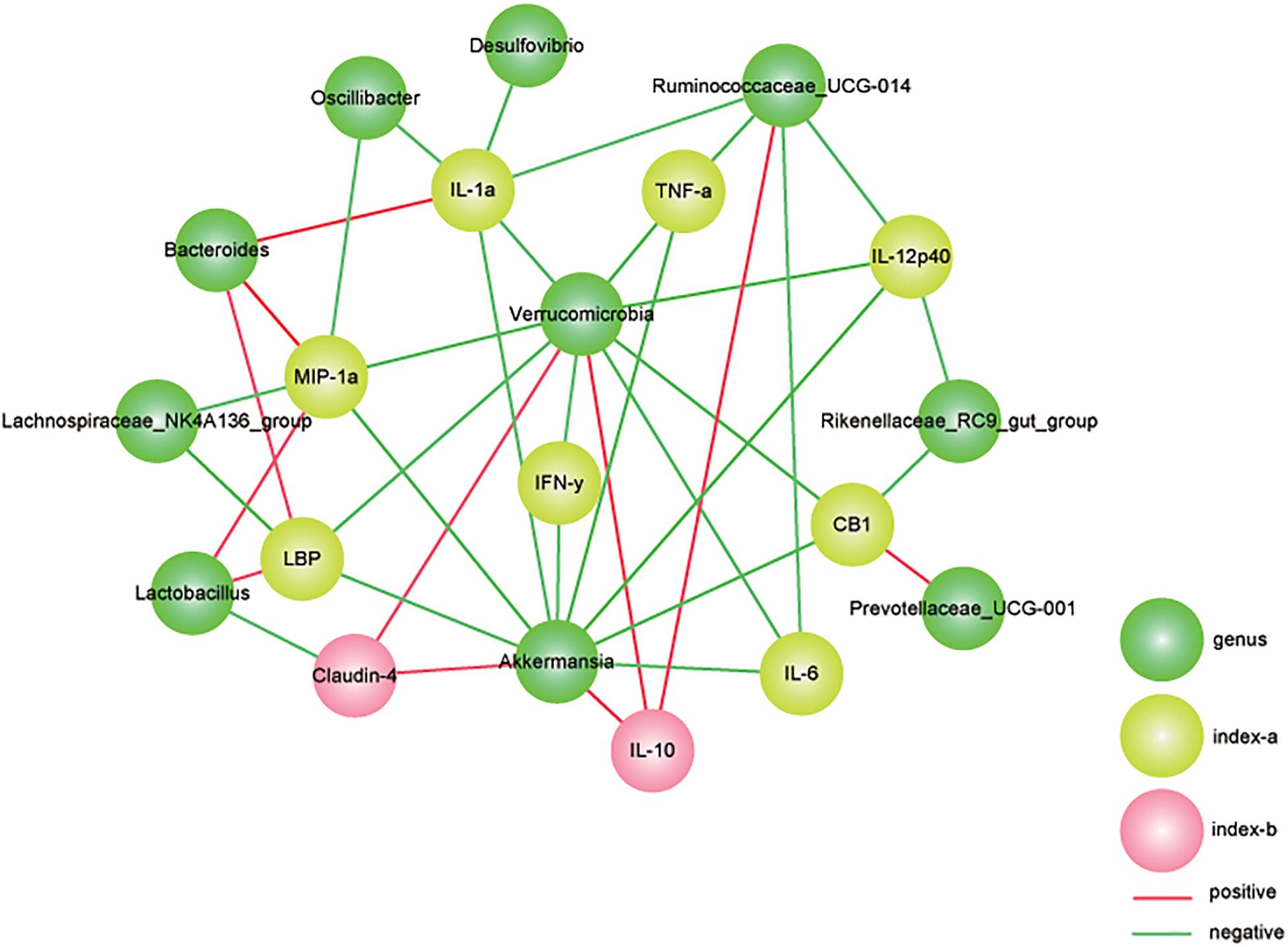
Figure 8. Correlation analysis between gut microbiota and colitis parameters. We used Spearman’s rank correlation and significant relationships with P < 0.05 and r > 0.5 are displayed. Yellow nodes represent the colitis parameters. Green nodes represent the differentially distributed genus. Green lines represent negative relationships, and the red lines represent positive relationships. The thickness of the line indicates the value of r.
Discussion
Recently, several studies have demonstrated that the newly isolated bacterium A. muciniphila MucT is highly associated with healthy mucosa in the context of multiple disorders (Derrien et al., 2011; Shin et al., 2014; Plovier et al., 2017). A similar finding was observed in IBD patients, suggesting that A. muciniphila may exert a beneficial effect during the progression of colitis (Png et al., 2010). However, the protective effects of A. muciniphila have not been widely demonstrated and its microbial and immunity moderation properties in colitis. Therefore, we determined the impacts of A. muciniphila using a DSS-induced colitis mouse model. In our study, we found that A. muciniphila could substantially ameliorate the colitis, as indicated by lower body weight loss, colon length shortening, histological scores and DAI scores. Additionally, some of the potential mechanisms were presented, including (i) amelioration of colon mucosal barrier damage; (ii) modulation of the inflammatory response; (iii) reshaping the gut microbiota; and (iv) regulation of metabolic functions.
As previously reported, thinner colonic mucus was found in patients with UC, as well as in mouse and rat models of colitis, compared with controls (Petersson et al., 2011; Dicksved et al., 2012; Johansson et al., 2014). Furthermore, multiple studies have revealed that intestinal permeability and LPS levels were increased in mice with colitis (Souza et al., 2015; Llewellyn et al., 2018), these findings suggested intestinal barrier damage and sequentially aggravated intestinal colitis. Importantly, A. muciniphila, identified as a mucin-degrading bacteria (Derrien et al., 2004), could continuously reshape and refresh the mucus layer, thereby creating a healthy environment for epithelial cells and maintaining the integrity of the intestinal barrier (Everard et al., 2013). In vitro, Reunanen et al. (2015) found that A. muciniphila could strengthen Intestinal epithelial integrity and fortify a destroyed gut barrier. Our findings of decreased serum LBP levels, strengthen tight junctions, and reduced CB1 and CB2 expression due to A. muciniphila administration were in agreement with the results of previous studies (Lin et al., 2012).
Accumulating evidence has revealed that the gut microbiota regulates the gut endocannabinoid (eCB) system, which in turn controls intestinal barrier function and inflammation by modulating intestinal permeability and LPS levels, but the molecular link between the colonic eCB system and IBD remains unknown (Maccarrone et al., 2001; Di Marzo et al., 2009; Hoareau et al., 2009). Muccioli et al. (2010) also found that there was a close relationship between the intestinal eCB system and LPS and that LPS levels were reduced by CB1 blockage through a mechanism related to the integrity of the intestinal barrier. Crucially, A. muciniphila treatment was observed to increase the colonic levels of endocannabinoids (Everard et al., 2013). In the current study, the expression of CB1 was upregulated after DSS administration, and A. muciniphila supplementation markedly downregulated the CB1 level, indicating an important role of A. muciniphila in improving gastrointestinal function through eCB function.
Importantly, the serum and colon tissue inflammatory cytokine levels in the AKK group showed significant inflammation relief, suggesting that A. muciniphila may have an important impact on systemic and intestinal anti-inflammation in mice with DSS-induced colitis.
Epithelium damage and profound intestinal inflammation were observed in IBD patients (Souza et al., 2005; Coskun, 2014). Likewise, during DSS administration, the colonic immune cells were exposed to luminal antigens as a result of the injured intestinal epithelium, subsequently inducing a strong inflammatory immune response (Wirtz et al., 2017). Our results demonstrated that A. muciniphila treatment exerted a protective effect against mouse colitis by inhibiting some critical components associated with the inflammatory response, including pro-inflammatory cytokines (TNF-α, IL6, IL12A, INF-γ, and IL1α), anti-inflammatory cytokines (IL-10), and chemokines (MIP-1α, G-CSF, and KC). A previous study also observed that the expression of genes associated with the immune response was increased by A. muciniphila colonization (Derrien et al., 2011). Cytokines such as TNF-α and IL6 were highly expressed in humans with IBD (Reinecker et al., 1993) and experimental models of IBD (Yoshida et al., 2011; Senchenkova et al., 2013; Yan et al., 2014), and interventions to block their accumulation could protect against colitis (Souza et al., 2015). Moreover, these cytokines have been found to be involved in the disruption of epithelial tight junctions through the NF-κB signaling pathway, resulting in mucosal dysfunction (Fink, 2003; Ahl et al., 2016; Woodhouse et al., 2018; Li et al., 2019). In experimental colitis (Souza et al., 2015) and in IBD patients (Vainer et al., 1998; Banks et al., 2003), chemokines (e.g., MIP-1α) were produced at an accelerated rate and were involved in monocytes/macrophages activations and inflammatory immune modulation (Carr et al., 1994; Kostova et al., 2015). Our study consistently found that DSS administration markedly upregulated MIP-1α expression both in serum and colon tissue, and treatment with A. muciniphila could reverse the alteration.
IL-10, secreted by dendritic cells (DCs), monocytes, B cells, CD4+ T cells and CD8+ T cells, plays an important role in anti-inflammatory responses by binding the IL10R1 and IL10R2 receptors (Del Prete et al., 1993). Therefore, an IL10R intervention was commonly a way to study the correlation between the immune response and gut microbiota composition (Loren et al., 2017). Pre-treatment with Lactobacillus GG could prevent rat colitis associated with increased IL10 expression (Dieleman et al., 2003), and IL10 gene therapy could prevent TNBS-induced colitis in mice (Lindsay et al., 2002). In vitro, the anti-inflammatory function of IL10 has been demonstrated in a range of cell types (Ribbons et al., 1997), and increased IL10 subsequently reduces the underlying production of TNF-α and IL6 (Malefyt et al., 1991). Lammers et al. (2003) found that IL10 production was increased in human peripheral blood mononuclear cells (PBMCs) stimulated by components from certain VSL#3 bacteria. Importantly, IL10 exerts its preventative function before the development of inflammation, and it is ineffective once inflammation has occurred (Barbara et al., 2000; Lindsay et al., 2002; Van Montfrans et al., 2002). Consistent with the findings of these studies (Dieleman et al., 2003; Di Giacinto et al., 2005), our experiment indicated that the beneficial impact of A. muciniphila in experimental colitis was involved in the induction of IL10.
Compelling studies have revealed that broad intestinal microbial dysbiosis, including a reduction in bacterial abundance and a decrease in diversity, occurs in IBD (Manichanh et al., 2006; Frank et al., 2007; Dicksved et al., 2012; Putignani et al., 2016). Our results have consistently shown that there is an intestinal microbiota alteration pattern in mice administered DSS, revealing a decrease in α diversity and an altered relative abundance of specific bacteria taxa. In the group supplemented with A. muciniphila, we observed a restored α diversity and changes in fecal bacteria, including increased Firmicutes and decreased Bacteroidetes. However, restored β diversity in the group supplemented with A. muciniphila was not observed in our study, indicating that the A. muciniphila administration cannot fully reverse the gut microbiota construction in a state of colitis.
Specific bacteria in the Enterobacteriacae family were enriched both in mice and IBD patients (Lupp et al., 2007). In human studies, Escherichia was found in UC patients (Sokol et al., 2006) and ileac biopsies of individuals with CD (Darfeuille-Michaud et al., 2004). Similarly, the augmented Escherichia/Shigella levels may worsen disease severity by increasing intestinal permeability (Quigley et al., 2013). These data provide support for the conjecture that Enterobacteriaceae may have growth advantages in the inflammatory gut environment of IBD. Similar results were observed in our study in which Enterobacteriacae was clustered in mice treated with DSS, and overgrowth of this bacterial clade destroyed the intestinal permeability, resulting in worsened colitis.
A range of specific groups of microbes have the ability to protect the host from colitis. Some members of the gut microbiota were capable of modulating mucosal inflammation by stimulating IL10 secretion (Sokol et al., 2006). Clostridium species could protect the host from mucosal inflammation by recruiting regulatory T cells (Atarashi et al., 2013), and other gut bacterial groups can mitigate intestinal colitis via the NF-κB pathway (Kelly et al., 2004). The results obtained from our study consistently revealed that Verrucomicrobiales was correlated with a majority of tested factors involved in immune regulation. Akkermansia and Ruminococcaceae were positively associated with IL10 and negatively associated with IL6 and TNF-α. These microbes were significantly clustered in the group that was treated with A. muciniphila, indicating that their protective role was possibly modulated by the mucosal immune response.
Recent studies have revealed that A. muciniphila is able to degrade host mucin into various metabolites (e.g., SCFAs) to regulate host biological functions, such as glucose and lipid metabolism, and to modulate the expression of mucus-related genes associated with cell death receptor signaling, which participate in host immune responses (Derrien et al., 2004; Derrien et al., 2011). SCFAs could exert beneficial impacts on host energy metabolism (Zuo and Ng, 2018). SCFAs are fermented from dietary fiber by some gut microbes and are considered the primary energy source of gut epithelial cells (Ahmad et al., 2000). Several studies found that SCFAs have important immunomodulatory functions in innate immune cells (Fusunyan et al., 1999; Ciarlo et al., 2016). SCFAs, such as butyrate, can inhibit NF-κB activation and stimulate mucin production, resulting in an induced inflammatory response and enhanced barrier function (Mchardy et al., 2013). In addition, SCFAs are an important fuel for some gut commensals, which might increase the abundance of SCFA-dependent bacteria and modulate the interactions of gut commensals, resulting in the alteration of the microbiota composition and functions (Belzer and De Vos, 2012). Notably, the increased relative abundance of Verrucomicrobia, Ruminococcaceae and Oscillibacter in mice with A. muciniphila, which has not been observed in the CP and DP groups, might be due to A. muciniphila and its metabolites. Firmicutes, including the families Lachnospiraceae and Ruminococcaceae, is considered an anti-inflammatory factor due to their production of SCFAs, especially butyric acid. In humans, members of the Lachnospiraceae family may exert a protective effect against colon cancer (Meehan and Beiko, 2014), mainly due to the production of butyric acid, a substance that is associated with the gut microbiome, host epithelial cells and human physiology. Within these two families, some genera were positively correlated with anti-inflammatory cytokines and gut barrier markers and negatively correlated with pro-inflammatory cytokines, indicating protective impacts of these microbes on host health. These data implied that the favorable role of A. muciniphila in immune response was mediated not only by the strengthened gut barrier function, but also by the productions of beneficial bacteria.
To understand the functional interactions between microbes and hosts, we investigated the predicted functional differences in KEGG pathways. We observed that several predicted pathways, such as the NOD-like receptor signaling pathway, glycosaminoglycan degradation pathway, and the lipid metabolism pathway, differed between the DP group and the AKK group. Consistent with our results, Dunn et al. (2016) found that there was a significantly different NOD-like receptor signaling pathway between patients with CD and controls. The NOD-like receptor signaling pathway may contribute to host immune regulation (Shade and Handelsman, 2012; Tremaroli and Backhed, 2012; Guinane and Cotter, 2013; Larsen and Dai, 2015; Moore-Connors et al., 2016). Glycosaminoglycans (GAGs), including keratan sulfate and dermatan sulfate, are essential for the gut mucosa because they regulate gut permeability (Sharpton et al., 2017). Sharpton et al. (2017) also reported that the glycosaminoglycan degradation pathway increased as colitis progressed in patients with IBD. In addition, these GAGs were depleted in patients’ intestines (Murch et al., 1993), and GAG metabolism was considered relevant to intestinal colonization (Benjdia et al., 2011; Ulmer et al., 2014). Our identification of pathways may contribute to novel hypotheses determining the cause-and-effect between the gut microbiota and IBD.
Several studies have shown contradictory results. They found that A. muciniphila was strongly associated with higher rates of tumorigenesis in colorectal cancer, and some mucin-degradation genes were positively correlated with cancer incidence (Baxter et al., 2014). Colonic anaerobic bacteria could ferment ingested carbohydrates and proteins into SCFAs (Cummings, 1981). SCFAs are known as the main energy source for colonocytes, and a deficiency of this energy source may cause the onset of colitis (Roediger, 1980). The strong correlation between A. muciniphila and colorectal cancer might be due to the energy dependence. In addition, several studies have found that excessive mucin degradation may aggravate colitis by allowing increased microbial access to the mucus layer (Ganesh et al., 2013; Seregin et al., 2017). However, a previous study has demonstrated that the mucin degradation properties of A. muciniphila could provide nutrition for other gut commensals and produce SCFAs (Wu et al., 2017), which are beneficial to the host as we discussed above. In our study, we found that A. muciniphila could exert a beneficial role through a combination of different factors.
More importantly, fecal microbiota transplant (FMT) and probiotics are being increasingly used to treat UC, but their use is controversial because of their uncertain efficacy. FMT is recognized as a novel therapy of UC, as it may alter the gut microbial dysbiosis (Sood et al., 2019). Four randomized controlled trials (RCTs) along with several cases have showed the efficacy of FMT to induce remission in active UC patients (Angelberger et al., 2013; Kump et al., 2013; Kunde et al., 2013; Cui et al., 2015; Moayyedi et al., 2015; Ren et al., 2015; Rossen et al., 2015; Suskind et al., 2015; Wei et al., 2015; Paramsothy et al., 2017; Costello et al., 2019). A meta-analysis including 1491 patients with UC (from 18 placebo-controlled and active treatment-controlled studies) found that probiotics were beneficial for the induction of remission in patients with UC (Asto et al., 2019). Recently, a proof-of-concept prospective study unequivocally showed that long-term (3 months) administration of A. muciniphila (1010 cells per day) was safe and verified the feasibility and tolerance of A. muciniphila supplementation in humans (Depommier et al., 2019). A. muciniphila is emerging as a novel protective agent, but the efficacy, reliability and safety of A. muciniphila in patients with IBD still need to be determined.
Nevertheless, there are certain limitations to our study. The animal model we used mimics some typical histopathological and certain immunological characteristics of patients with IBD, but the model is not fully representative of the complex features of the disease. Additionally, our study demonstrated a correlation between alleviated colitis and A. muciniphila-modified gut microbiota. Other potential mechanisms are needed, and further clinical studies in humans with IBD need to be investigated.
Conclusion
Overall, we confirmed that A. muciniphila administration could alleviate mucosal inflammation either by microbe-host interactions that protect the gut epithelial barrier function and downregulate the levels of related inflammatory cytokines or by improving the microbial community. Our findings show that A. muciniphila exerted a protective effect on DSS-induced colitis in mice, indicating that A. muciniphila may be a potential probiotic agent for ameliorating colitis.
Data Availability Statement
The datasets generated for this study can be found in NCBI https://www.ncbi.nlm.nih.gov/sra/?term=PRJNA554980.
Ethics Statement
The animal study was reviewed and approved by the Local Committee of Animal Use and was performed in accordance with the criteria of “the Animal Care Committee of Zhejiang University School of Medicine”, “Guide for the Care and Use of Laboratory Animals” (NIH publication 86–23 revised 1985). Written informed consent was obtained from the owners for the participation of their animals in this study.
Author Contributions
XB, WW, and YL designed the experiments. XB and LY analyzed the data. XB wrote the manuscript. All authors reviewed the manuscript.
Funding
This work was funded by the Key Program of the National Natural Science Foundation of China (No. 81330011) and the Science Fund for Creative Research Groups of the National Natural Science Foundation of China (No. 81721091).
Conflict of Interest
The authors declare that the research was conducted in the absence of any commercial or financial relationships that could be construed as a potential conflict of interest.
Supplementary Material
The Supplementary Material for this article can be found online at: https://www.frontiersin.org/articles/10.3389/fmicb.2019.02259/full#supplementary-material
FIGURE S1 | Bar charts represents the serum cytokine levels of IL-1α, TNF-α, IL6, IL12A, IL12B, lL17, eotaxin, G-CSF, KC, MIP-1A, RANTES, and IL10 among the groups. ∗P < 0.05; ∗∗P < 0.01; ∗∗∗P < 0.001; ****P < 0.0001 by post hoc one-way ANOVA.
FIGURE S2 | Akkermansia muciniphila reshaped the gut microbiota community. The rarefaction curves of three groups.
FIGURE S3 | PISRUST results of predicted metabolic pathways in the AKK group (Green) and DP group (Orange).
TABLE S1 | Specific primers used for the RT-PCR analyses.
TABLE S2 | MRPP test used to analyze the β diversity in fecal analysis.
Footnotes
References
Ahl, D., Liu, H., Schreiber, O., Roos, S., Phillipson, M., and Holm, L. (2016). Lactobacillus reuteri increases mucus thickness and ameliorates dextran sulphate sodium-induced colitis in mice. Acta Physiol. 217, 300–310. doi: 10.1111/apha.12695
Ahmad, M. S., Krishnan, S., Ramakrishna, B. S., Mathan, M., Pulimood, A. B., and Murthy, S. N. (2000). Butyrate and glucose metabolism by colonocytes in experimental colitis in mice. Gut 46, 493–499. doi: 10.1136/gut.46.4.493
Andlujar, I., Carmen Recio, M., Giner, R. M., Cienfuegos-Jovellanos, E., Laghi, S., Muguerza, B., et al. (2011). Inhibition of ulcerative colitis in mice after oral administration of a polyphenol-enriched cocoa extract is mediated by the inhibition of STAT1 and STAT3 phosphorylation in colon cells. J. Agric. Food Chem. 59, 6474–6483. doi: 10.1021/jf2008925
Angelberger, S., Reinisch, W., Makristathis, A., Lichtenberger, C., Dejaco, C., Papay, P., et al. (2013). Temporal bacterial community dynamics vary among ulcerative colitis patients after fecal microbiota transplantation. Am. J. Gastroenterol. 108, 1620–1630. doi: 10.1038/ajg.2013.257
Asto, E., Mendez, I., Audivert, S., and Farran-Codina, A. (2019). The efficacy of probiotics, prebiotic inulin-type fructans, and synbiotics in human ulcerative colitis: a systematic review and meta-analysis. Nutrients 11:E293. doi: 10.3390/nu11020293
Atarashi, K., Tanoue, T., Oshima, K., Suda, W., Nagano, Y., Nishikawa, H., et al. (2013). T-reg induction by a rationally selected mixture of clostridia strains from the human microbiota. Nature 500, 232–236. doi: 10.1038/nature12331
Bajaj, J. S., Heuman, D. M., Hylemon, P. B., Sanyal, A. J., White, M. B., Monteith, P., et al. (2014). Altered profile of human gut microbiome is associated with cirrhosis and its complications. J. Hepatol. 60, 940–947. doi: 10.1016/j.jhep.2013.12.019
Banks, C., Bateman, A., Payne, R., Johnson, P., and Sheron, N. (2003). Chemokine expression in IBD. Mucosal chemokine expression is unselectively increased in both ulcerative colitis and Crohn’s disease. J. Pathol. 199, 28–35. doi: 10.1002/path.1245
Barbara, G., Xing, Z., Hogaboam, C. M., Gauldie, J., and Collins, S. M. (2000). Interleukin 10 gene transfer prevents experimental colitis in rats. Gut 46, 344–349. doi: 10.1136/gut.46.3.344
Baxter, N. T., Zackular, J. P., Chen, G. Y., and Schloss, P. D. (2014). Structure of the gut microbiome following colonization with human feces determines colonic tumor burden. Microbiome 2:20. doi: 10.1186/2049-2618-2-20
Belzer, C., and De Vos, W. M. (2012). Microbes inside–from diversity to function: the case of Akkermansia. Isme J. 6, 1449–1458. doi: 10.1038/ismej.2012.6
Benjdia, A., Martens, E. C., Gordon, J. I., and Berteau, O. (2011). Sulfatases and a radical S-Adenosyl-L-methionine (AdoMet) enzyme are key for mucosal foraging and fitness of the prominent human gut symbiont, Bacteroides thetaiotaomicron. J. Biol. Chem. 286, 25973–25982. doi: 10.1074/jbc.M111.228841
Carr, M. W., Roth, S. J., Luther, E., Rose, S. S., and Springer, T. A. (1994). Monocyte chemoattractant protein 1 acts as a T-lymphocyte chemoattractant. Proc. Natl. Acad. Sci. U.S.A. 91, 3652–3656. doi: 10.1073/pnas.91.9.3652
Chung, C. Y., Alden, S. L., Funderburg, N. T., Fu, P., and Levine, A. D. (2014). Progressive proximal-to-distal reduction in expression of the tight junction complex in colonic epithelium of virally-suppressed HIV plus Individuals. Plos Pathog. 10:e1004198. doi: 10.1371/journal.ppat.1004198
Ciarlo, E., Heinonen, T., Herderschee, J., Fenwick, C., Mombelli, M., Le Roy, D., et al. (2016). Impact of the microbial derived short chain fatty acid propionate on host susceptibility to bacterial and fungal infections in vivo. Sci. Rep. 6:37944. doi: 10.1038/srep37944
Collado, M. C., Derrien, M., and Isolauri, E. (2007). Intestinal integrity and Akkermansia muciniphila, a mucin-degrading member of the intestinal microbiota present in infants, adults, and the elderly. Appl. Environ. Microbiol. 73, 7767–7770. doi: 10.1128/aem.01477-07
Costello, S. P., Hughes, P. A., Waters, O., Bryant, R. V., Vincent, A. D., Blatchford, P., et al. (2019). Effect of fecal microbiota transplantation on 8-week remission in patients with ulcerative colitis: a randomized clinical trial. Jama 321, 156–164. doi: 10.1001/jama.2018.20046
Cui, B., Li, P., Xu, L., Zhao, Y., Wang, H., Peng, Z., et al. (2015). Step-up fecal microbiota transplantation strategy: a pilot study for steroid-dependent ulcerative colitis. J. Transl. Med. 13:298. doi: 10.1186/s12967-015-0646-2
Cummings, J. H. (1981). Short chain fatty acids in the human colon. Gut 22, 763–779. doi: 10.1136/gut.22.9.763
Darfeuille-Michaud, A., Boudeau, J., Bulois, P., Neut, C., Glasser, A. L., Barnich, N., et al. (2004). High prevalence of adherent-invasive Escherichia coli associated with ileal mucosa in Crohn’s disease. Gastroenterology 127, 412–421. doi: 10.1053/j.gastro.2004.04.061
Del Prete, G., De Carli, M., Almerigogna, F., Giudizi, M. G., Biagiotti, R., and Romagnani, S. (1993). Human IL-10 is produced by both type 1 helper (Th1) and type 2 helper (Th2) T cell clones and inhibits their antigen-specific proliferation and cytokine production. J. Immunol. 150, 353–360.
Depommier, C., Everard, A., Druart, C., Plovier, H., Van Hul, M., Vieira-Silva, S., et al. (2019). Supplementation with Akkermansia muciniphila in overweight and obese human volunteers: a proof-of-concept exploratory study. Nat. Med. 25, 1096–1103. doi: 10.1038/s41591-019-0495-2
Derrien, M., Collado, M. C., Ben-Amor, K., Salminen, S., and De Vos, W. M. (2008). The mucin degrader Akkermansia muciniphila is an abundant resident of the human intestinal tract. Appl. Environ. Microbiol. 74, 1646–1648. doi: 10.1128/aem.01226-07
Derrien, M., Van Baarlen, P., Hooiveld, G., Norin, E., Muller, M., and De Vos, W. M. (2011). Modulation of mucosal immune response, tolerance, and proliferation in mice colonized by the mucin-degrader Akkermansia muciniphila. Front. Microbiol. 2:166. doi: 10.3389/fmicb.2011.00166
Derrien, M., Vaughan, E. E., Plugge, C. M., and De Vos, W. M. (2004). Akkermansia muciniphila gen. nov., sp nov., a human intestinal mucin-degrading bacterium. Int. J. Syst. and Evol. Microbiol. 54, 1469–1476. doi: 10.1099/ijs.0.02873-0
Di Giacinto, C., Marinaro, M., Sanchez, M., Strober, W., and Boirivant, M. (2005). Probiotics ameliorate recurrent Th1-mediated murine colitis by inducing IL-10 and IL-10-dependent TGF-beta-bearing regulatory cells. J. Immunol. 174, 3237–3246. doi: 10.4049/jimmunol.174.6.3237
Di Marzo, V., Cote, M., Matias, I., Lemieux, I., Arsenault, B. J., Cartier, A., et al. (2009). Changes in plasma endocannabinoid levels in viscerally obese men following a 1 year lifestyle modification programme and waist circumference reduction: associations with changes in metabolic risk factors. Diabetologia 52, 213–217. doi: 10.1007/s00125-008-1178-6
Dicksved, J., Schreiber, O., Willing, B., Petersson, J., Rang, S., Phillipson, M., et al. (2012). Lactobacillus reuteri maintains a functional mucosal barrier during DSS treatment despite mucus layer dysfunction. Plos One 7:e46399. doi: 10.1371/journal.pone.0046399
Dieleman, L. A., Goerres, M. S., Arends, A., Sprengers, D., Torrice, C., Hoentjen, F., et al. (2003). Lactobacillus GG prevents recurrence of colitis in HLA-B27 transgenic rats after antibiotic treatment. Gut 52, 370–376. doi: 10.1136/gut.52.3.370
Dunn, K. A., Moore-Connors, J., Macintyre, B., Stadnyk, A., Thomas, N. A., Noble, A., et al. (2016). The gut microbiome of pediatric crohn’s disease patients differs from healthy controls in genes that can influence the balance between a healthy and dysregulated immune response. Inflamm. Bowel Dis. 22, 2607–2618. doi: 10.1097/mib.0000000000000949
Edgar, R. C. (2013). UPARSE: highly accurate OTU sequences from microbial amplicon reads. Nat. Methods 10, 996–998. doi: 10.1038/nmeth.2604
Elinav, E., Strowig, T., Kau, A. L., Henao-Mejia, J., Thaiss, C. A., Booth, C. J., et al. (2011). NLRP6 inflammasome regulates colonic microbial ecology and risk for colitis. Cell 145, 745–757. doi: 10.1016/j.cell.2011.04.022
Erben, U., Loddenkemper, C., Doerfel, K., Spieckermann, S., Haller, D., Heimesaat, M. M., et al. (2014). A guide to histomorphological evaluation of intestinal inflammation in mouse models. Int. J. Clin. Exp. Pathol. 7, 4557–U4527.
Everard, A., Belzer, C., Geurts, L., Ouwerkerk, J. P., Druart, C., Bindels, L. B., et al. (2013). Cross-talk between Akkermansia muciniphila and intestinal epithelium controls diet-induced obesity. Proc. Natl. Acad. Sci. U. S. A. 110, 9066–9071. doi: 10.1073/pnas.1219451110
Fink, M. P. (2003). Intestinal epithelial hyperpermeability: update on the pathogenesis of gut mucosal barrier dysfunction in critical illness. Curr. Opin. Crit. Care 9, 143–151. doi: 10.1097/00075198-200304000-00011
Frank, D. N., Amand, A. L. S., Feldman, R. A., Boedeker, E. C., Harpaz, N., and Pace, N. R. (2007). Molecular-phylogenetic characterization of microbial community imbalances in human inflammatory bowel diseases. Proc. Natl. Acad. Sci. U.S.A. 104, 13780–13785. doi: 10.1073/pnas.0706625104
Fusunyan, R. D., Quinn, J. J., Fujimoto, M., Macdermott, R. P., and Sanderson, I. R. (1999). Butyrate switches the pattern of chemokine secretion by intestinal epithelial cells through histone acetylation. Mol. Med. 5, 631–640. doi: 10.1007/bf03402075
Ganesh, B. P., Klopfleisch, R., Loh, G., and Blaut, M. (2013). Commensal Akkermansia muciniphila exacerbates gut inflammation in Salmonella Typhimurium-infected gnotobiotic mice. PLoS One 8:e74963. doi: 10.1371/journal.pone.0074963
Guinane, C. M., and Cotter, P. D. (2013). Role of the gut microbiota in health and chronic gastrointestinal disease: understanding a hidden metabolic organ. Ther. Adv. Gastroenterol. 6, 295–308. doi: 10.1177/1756283X13482996
Hernandez-Chirlaque, C., Aranda, C. J., Ocon, B., Capitan-Canadas, F., Ortega-Gonzalez, M., Carrero, J. J., et al. (2016). Germ-free and antibiotic-treated mice are highly susceptible to epithelial injury in DSS colitis. J. Crohns. Colitis. 10, 1324–1335. doi: 10.1093/ecco-jcc/jjw096
Hoareau, L., Buyse, M., Festy, F., Ravanan, P., Gonthier, M.-P., Matias, I., et al. (2009). Anti-inflammatory effect of palmitoylethanolamide on human adipocytes. Obesity 17, 431–438. doi: 10.1038/oby.2008.591
Hudcovic, T., Stepankova, R., Cebra, J., and Tlaskalova-Hogenova, H. (2001). The role of microflora in the development of intestinal inflammation: acute and chronic colitis induced by dextran sulfate in germ-free and conventionally reared immunocompetent and immunodeficient mice. Folia Microbio. 46, 565–572. doi: 10.1007/bf02818004
Johansson, M. E. V., Gustafsson, J. K., Holmen-Larsson, J., Jabbar, K. S., Xia, L., Xu, H., et al. (2014). Bacteria penetrate the normally impenetrable inner colon mucus layer in both murine colitis models and patients with ulcerative colitis. Gut 63, 281–291. doi: 10.1136/gutjnl-2012-303207
Kaplan, G. G. (2015). The global burden of IBD: from 2015 to 2025. Nat. Rev. Gastroenterol. Amp Hepatol. 12:720. doi: 10.1038/nrgastro.2015.150
Kelly, D., Campbell, J. I., King, T. P., Grant, G., Jansson, E. A., Coutts, A. G. P., et al. (2004). Commensal anaerobic gut bacteria attenuate inflammation by regulating nuclear-cytoplasmic shuttling of PPAR-gamma and RelA. Nat. Immunol. 5, 104–112. doi: 10.1038/ni1018
Kostova, Z., Batsalova, T., Moten, D., Teneva, I., and Dzhambazov, B. (2015). Ragweed-allergic subjects have decreased serum levels of chemokines CCL2, CCL3, CCL4 and CCL5 out of the pollen season. Cent. Eur. J. Immunol. 40, 442–446. doi: 10.5114/ceji.2015.56965
Kump, P. K., Grochenig, H. P., Lackner, S., Trajanoski, S., Reicht, G., Hoffmann, K. M., et al. (2013). Alteration of intestinal dysbiosis by fecal microbiota transplantation does not induce remission in patients with chronic active ulcerative colitis. Inflamm. Bowel Dis. 19, 2155–2165. doi: 10.1097/mib.0b013e31829ea325
Kunde, S., Pham, A., Bonczyk, S., Crumb, T., Duba, M., Conrad, H., et al. (2013). Safety, tolerability, and clinical response after fecal transplantation in children and young adults with ulcerative colitis. J. Pediatr. Gastroenterol. Nutr. 56, 597–601. doi: 10.1097/MPG.0b013e318292fa0d
Lakatos, P. L., Kiss, L. S., Palatka, K., Altorjay, I., Antal-Szalmas, P., Palyu, E., et al. (2011). Serum lipopolysaccharide-binding protein and soluble CD14 are markers of disease activity in patients with Crohn’s disease. Inflamm. Bowel Dis. 17, 767–777. doi: 10.1002/ibd.21402
Lammers, K. M., Brigidi, P., Vitali, B., Gionchetti, P., Rizzello, F., Caramelli, E., et al. (2003). Immunomodulatory effects of probiotic bacteria DNA: IL-1 and IL-10 response in human peripheral blood mononuclear cells. FEMS Immunol. Med. Microbiol. 38, 165–172. doi: 10.1016/s0928-8244(03)00144-5
Larsen, P. E., and Dai, Y. (2015). Metabolome of human gut microbiome is predictive of host dysbiosis. Gigascience 4:42. doi: 10.1186/s13742-015-0084-3
Li, Y., Lv, L., Ye, J., Fang, D., Shi, D., Wu, W., et al. (2019). Bifidobacterium adolescentis CGMCC 15058 alleviates liver injury, enhances the intestinal barrier and modifies the gut microbiota in d-galactosamine-treated rats. Appl. Microbiol. Biotechnol. 103, 375–393. doi: 10.1007/s00253-018-9454-y
Lin, Y., Yu, L.-X., Yan, H.-X., Yang, W., Tang, L., Zhang, H.-L., et al. (2012). Gut-derived lipopolysaccharide promotes T-Cell-Mediated hepatitis in mice through toll-like receptor 4. Cancer Prev. Res. 5, 1090–1102. doi: 10.1158/1940-6207.CAPR-11-0364
Lindsay, J., Van Montfrans, C., Brennan, F., Van Deventer, S., Drillenburg, P., Hodgson, H., et al. (2002). IL-10 gene therapy prevents TNBS-induced colitis. Gene Therapy 9, 1715–1721. doi: 10.1038/sj.gt.3301841
Llewellyn, S. R., Britton, G. J., Contijoch, E. J., Vennaro, O. H., Mortha, A., Colombel, J.-F., et al. (2018). Interactions between diet and the intestinal microbiota alter intestinal permeability and colitis severity in mice. Gastroenterology 154, 1037.e2–1046.e2. doi: 10.1053/j.gastro.2017.11.030
Loren, V., Manye, J., Fuentes, M. C., Cabre, E., Ojanguren, I., and Espadaler, J. (2017). Comparative effect of the I3.1 probiotic formula in two animal models of colitis. Probiotics Antimicrob. Proteins 9, 71–80. doi: 10.1007/s12602-016-9239-5
Lupp, C., Robertson, M. L., Wickham, M. E., Sekirov, I., Champion, O. L., Gaynor, E. C., et al. (2007). Host-mediated inflammation disrupts the intestinal microbiota and promotes the overgrowth of Enterobacteriaceae (Vol 2, pg 119, 2007). Cell Host Microbe 2, 204–204.
Maccarrone, M., De Petrocellis, L., Bari, M., Fezza, F., Salvati, S., Di Marzo, V., et al. (2001). Lipopolysaccharide downregulates fatty acid amide hydrolase expression and increases anandamide levels in human peripheral lymphocytes. Arch. Biochem. Biophys. 393, 321–328. doi: 10.1006/abbi.2001.2500
Malefyt, R. D., Abrams, J., Bennett, B., Figdor, C. G., and Devries, J. E. (1991). Interleukin-10(Il-10) inhibits cytokine synthesis by human monocytes - an autoregulatory role Of Il-10 produced by monocytes. J. Exp. Med. 174, 1209–1220. doi: 10.1084/jem.174.5.1209
Manichanh, C., Rigottier-Gois, L., Bonnaud, E., Gloux, K., Pelletier, E., Frangeul, L., et al. (2006). Reduced diversity of faecal microbiota in Crohn’s disease revealed by a metagenomic approach. Gut 55, 205–211. doi: 10.1136/gut.2005.073817
Marion-Letellier, R., Savoye, G., and Ghosh, S. (2016). IBD: in food we trust. J. Croh’s Colitis 10, 1351–1361. doi: 10.1093/ecco-jcc/jjw106
Mchardy, I. H., Goudarzi, M., Tong, M., Ruegger, P. M., Schwager, E., Weger, J. R., et al. (2013). Integrative analysis of the microbiome and metabolome of the human intestinal mucosal surface reveals exquisite inter-relationships. Microbiome 1:17. doi: 10.1186/2049-2618-1-17
Meehan, C. J., and Beiko, R. G. (2014). A phylogenomic view of ecological specialization in the Lachnospiraceae, a family of digestive tract-associated bacteria. Genome Biol. Evol. 6, 703–713. doi: 10.1093/gbe/evu050
Moayyedi, P., Surette, M. G., Kim, P. T., Libertucci, J., Wolfe, M., Onischi, C., et al. (2015). Fecal microbiota transplantation induces remission in patients with active ulcerative colitis in a randomized controlled trial. Gastroenterology 149, 102.e6–109.e6. doi: 10.1053/j.gastro.2015.04.001
Moore-Connors, J. M., Dunn, K. A., Bielawski, J. P., and Van Limbergen, J. (2016). Novel strategies for applied metagenomics. Inflamm. Bowel Dis. 22, 709–718. doi: 10.1097/MIB.0000000000000717
Muccioli, G. G., Naslain, D., Backhed, F., Reigstad, C. S., Lambert, D. M., Delzenne, N. M., et al. (2010). The endocannabinoid system links gut microbiota to adipogenesis. Mol. Syst. Biol. 6:392. doi: 10.1038/msb.2010.46
Murch, S. H., Macdonald, T. T., Walkersmith, J. A., Levin, M., Lionetti, P., and Klein, N. J. (1993). Disruption of sulfated glycosaminoglycans in intestinal inflammation. Lancet 341, 711–714. doi: 10.1016/0140-6736(93)90485-y
Okayasu, I., Hatakeyama, S., Yamada, M., Ohkusa, T., Inagaki, Y., and Nakaya, R. (1990). A novel method in the induction of reliable experimental acute and chronic ulcerative colitis in mice. Gastroenterology 98, 694–702. doi: 10.1016/0016-5085(90)90290-h
Parada Venegas, D., De La Fuente, M. K., Landskron, G., Gonzalez, M. J., Quera, R., Dijkstra, G., et al. (2019). Short Chain Fatty Acids (SCFAs)-mediated gut epithelial and immune regulation and its relevance for inflammatory bowel diseases. Front. Immunol. 10:277. doi: 10.3389/fimmu.2019.00277
Paramsothy, S., Kamm, M. A., Kaakoush, N. O., Walsh, A. J., Van Den Bogaerde, J., Samuel, D., et al. (2017). Multidonor intensive faecal microbiota transplantation for active ulcerative colitis: a randomised placebo-controlled trial. Lancet 389, 1218–1228. doi: 10.1016/S0140-6736(17)30182-4
Petersson, J., Schreiber, O., Hansson, G. C., Gendler, S. J., Velcich, A., Lundberg, J. O., et al. (2011). Importance and regulation of the colonic mucus barrier in a mouse model of colitis. Am. J. Physiol. Gastrointest. Liver Physiol. 300, G327–G333. doi: 10.1152/ajpgi.00422.2010
Plovier, H., Everard, A., Druart, C., Depommier, C., Van Hul, M., Geurts, L., et al. (2017). A purified membrane protein from Akkermansia muciniphila or the pasteurized bacterium improves metabolism in obese and diabetic mice. Nat. Med. 23, 107–113. doi: 10.1038/nm.4236
Png, C. W., Linden, S. K., Gilshenan, K. S., Zoetendal, E. G., Mcsweeney, C. S., Sly, L. I., et al. (2010). Mucolytic bacteria with increased prevalence in IBD mucosa augment in vitro utilization of mucin by other bacteria. Am. J. Gastroenterol. 105, 2420–2428. doi: 10.1038/ajg.2010.281
Putignani, L., Del Chierico, F., Vernocchi, P., Cicala, M., Cucchiara, S., Dallapiccola, B., et al. (2016). Gut microbiota dysbiosis as risk and premorbid factors of IBD and IBS along the childhood-adulthood transition. Inflamm. Bowel Dis. 22, 487–504. doi: 10.1097/MIB.0000000000000602
Quigley, E. M. M., Stanton, C., and Murphy, E. F. (2013). The gut microbiota and the liver. Pathophysiol. clin. Implic. J. Hepatol. 58, 1020–1027.
Reinecker, H. C., Steffen, M., Witthoeft, T., Pflueger, I., Schreiber, S., Macdermott, R. P., et al. (1993). Enhanced secretion of Tumor-Necrosis-Factor-Alpha, Il-6, And Il-1-beta by isolated lamina propria mononuclear-cells from patients with ulcerative-colitis and crohns-disease. Clin. Exp. Immunol. 94, 174–181. doi: 10.1111/j.1365-2249.1993.tb05997.x
Ren, R., Sun, G., Yang, Y., Peng, L., Zhang, X., Wang, S., et al. (2015). A pilot study of treating ulcerative colitis with fecal microbiota transplantation. Zhonghua Nei Ke Za Zhi 54, 411–415.
Reunanen, J., Kainulainen, V., Huuskonen, L., Ottman, N., Belzer, C., Huhtinen, H., et al. (2015). Akkermansia muciniphila adheres to enterocytes and strengthens the integrity of the epithelial cell layer. Appl. Environ. Microbiol. 81, 3655–3662. doi: 10.1128/AEM.04050-14
Ribbons, K. A., Thompson, J. H., Liu, X. P., Pennline, K., Clark, D. A., and Miller, M. J. S. (1997). Anti-inflammatory properties of interleukin-10 administration in hapten-induced colitis. Eur. J. Pharmacol. 323, 245–254. doi: 10.1016/s0014-2999(97)00017-4
Roediger, W. E. (1980). The colonic epithelium in ulcerative colitis: an energy-deficiency disease? Lancet 2, 712–715. doi: 10.1016/s0140-6736(80)91934-0
Rossen, N. G., Fuentes, S., Van Der Spek, M. J., Tijssen, J. G., Hartman, J. H., Duflou, A., et al. (2015). Findings from a randomized controlled trial of fecal transplantation for patients with ulcerative colitis. Gastroenterology 149, 110.e4–118.e4. doi: 10.1053/j.gastro.2015.03.045
Sartor, R. B., and Wu, G. D. (2017). Roles for intestinal bacteria, viruses, and fungi in pathogenesis of inflammatory bowel diseases and therapeutic approaches. Gastroenterology 152, 327.e4–339.e4.
Senchenkova, E. Y., Komoto, S., Russell, J., Almeida-Paula, L. D., Yan, L.-S., Zhang, S., et al. (2013). Interleukin-6 mediates the platelet abnormalities and thrombogenesis associated with experimental colitis. Am. J. Pathol. 183, 173–181. doi: 10.1016/j.ajpath.2013.03.014
Seregin, S. S., Golovchenko, N., Schaf, B., Chen, J., Pudlo, N. A., Mitchell, J., et al. (2017). NLRP6 Protects Il10(-/-) mice from colitis by limiting colonization of akkermansia muciniphila. Cell Rep. 19, 2174. doi: 10.1016/j.celrep.2017.05.074
Shade, A., and Handelsman, J. (2012). Beyond the venn diagram: the hunt for a core microbiome. Environ. Microbiol. 14, 4–12. doi: 10.1111/j.1462-2920.2011.02585.x
Sharpton, T., Lyalina, S., Luong, J., Pham, J., Deal, E. M., Armour, C., et al. (2017). Development of inflammatory bowel disease is linked to a longitudinal restructuring of the gut metagenome in mice. Msystems 2:e00036-17. doi: 10.1128/mSystems.00036-17
Shin, N.-R., Lee, J.-C., Lee, H.-Y., Kim, M.-S., Whon, T. W., Lee, M.-S., et al. (2014). An increase in the Akkermansia spp. population induced by metformin treatment improves glucose homeostasis in diet-induced obese mice. Gut 63, 727–735. doi: 10.1136/gutjnl-2012-303839
Sokol, H., Lepage, P., Seksik, P., Dore, J., and Marteau, P. (2006). Temperature gradient gel electrophoresis of fecal 16S rRNA reveals active Escherichia coli in the microbiota of patients with ulcerative colitis. J. Clin. Microbiol. 44, 3172–3177. doi: 10.1128/jcm.02600-05
Sood, A., Mahajan, R., Singh, A., Midha, V., Mehta, V., Narang, V., et al. (2019). Role of fecal microbiota transplantation for maintenance of remission in patients with ulcerative colitis: a pilot Study. J. Crohns. Colitis. doi: 10.1093/ecco-jcc/jjz060 [Epub ahead of print].
Souza, D. G., Senchenkova, E. Y., Russell, J., and Granger, D. N. (2015). MyD88 mediates the protective effects of probiotics against the arteriolar thrombosis and leukocyte recruitment associated with experimental colitis. Inflamm. Bowel Dis. 21, 888–900. doi: 10.1097/MIB.0000000000000331
Souza, H. S., Tortori, C. J., Castelo-Branco, M. T., Carvalho, A. T., Margallo, V. S., Delgado, C. F., et al. (2005). Apoptosis in the intestinal mucosa of patients with inflammatory bowel disease: evidence of altered expression of FasL and perforin cytotoxic pathways. Int. J. Colorectal. Dis. 20, 277–286. doi: 10.1007/s00384-004-0639-8
Suskind, D. L., Singh, N., Nielson, H., and Wahbeh, G. (2015). Fecal microbial transplant via nasogastric tube for active pediatric ulcerative colitis. J. Pediatr. Gastroenterol. Nutr. 60, 27–29. doi: 10.1097/MPG.0000000000000544
Tamboli, C. P., Neut, C., Desreumaux, P., and Colombel, J. F. (2004). Dysbiosis as a prerequisite for IBD. Gut 53, 1057–1057.
Tremaroli, V., and Backhed, F. (2012). Functional interactions between the gut microbiota and host metabolism. Nature 489, 242–249. doi: 10.1038/nature11552
Ulmer, J. E., Vilen, E. M., Namburi, R. B., Benjdia, A., Beneteau, J., Malleron, A., et al. (2014). Characterization of glycosaminoglycan (GAG) sulfatases from the human gut symbiont Bacteroides thetaiotaomicron reveals the first GAG-specific bacterial endosulfatase. J. Biol. Chemistry 289, 24289–24303. doi: 10.1074/jbc.M114.573303
Vainer, B., Nielsen, O. H., and Horn, T. (1998). Expression of E-selectin, sialyl Lewis X, and macrophage inflammatory protein-1alpha by colonic epithelial cells in ulcerative colitis. Dig. Dis. Sci. 43, 596–608.
Van Montfrans, C., Pena, M. S. R., Pronk, I., Ten Kate, F. J. W., Te Velde, A. A., and Van Deventer, S. J. H. (2002). Prevention of colitis by interleukin 10-transduced T lymphocytes in the SCID mice transfer model. Gastroenterology 123, 1865–1876. doi: 10.1053/gast.2002.37067
Von Schillde, M. A., Hormannsperger, G., Weiher, M., Alpert, C. A., Hahne, H., Bauerl, C., et al. (2012). Lactocepin secreted by lactobacillus exerts anti-inflammatory effects by selectively degrading proinflammatory chemokines. Cell Host Microbe. 11, 387–396. doi: 10.1016/j.chom.2012.02.006
Wei, Y., Zhu, W., Gong, J., Guo, D., Gu, L., Li, N., et al. (2015). Fecal microbiota transplantation improves the quality of life in patients with inflammatory bowel disease. Gastroenterol. Res. Pract. 2015:517597. doi: 10.1155/2015/517597
Wirtz, S., Popp, V., Kindermann, M., Gerlach, K., Weigmann, B., Fichtner-Feigl, S., et al. (2017). Chemically induced mouse models of acute and chronic intestinal inflammation. Nat. Protoc. 12, 1295–1309. doi: 10.1038/nprot.2017.044
Woodhouse, C. A., Patel, V. C., Singanayagam, A., and Shawcross, D. L. (2018). Review article: the gut microbiome as a therapeutic target in the pathogenesis and treatment of chronic liver disease. Aliment. Pharmacol. Ther. 47, 192–202. doi: 10.1111/apt.14397
Wu, W., Lv, L., Shi, D., Ye, J., Fang, D., Guo, F., et al. (2017). Protective effect of akkermansia muciniphila against immune-mediated liver injury in a mouse model. Front. Microbiol. 8:1804. doi: 10.3389/fmicb.2017.01804
Yan, S. L. S., Russell, J., and Granger, D. N. (2014). Platelet activation and platelet-leukocyte aggregation elicited in experimental colitis are mediated by interleukin-6. Inflamm. Bowel Dis. 20, 353–362. doi: 10.1097/01.MIB.0000440614.83703.84
Yatsunenko, T., Rey, F. E., Manary, M. J., Trehan, I., Dominguez-Bello, M. G., Contreras, M., et al. (2012). Human gut microbiome viewed across age and geography. Nature 486, 222–227. doi: 10.1038/nature11053
Ye, J., Lv, L., Wu, W., Li, Y., Shi, D., Fang, D., et al. (2018). Butyrate protects mice against Methionine-Choline-Deficient Diet-Induced Non-alcoholic steatohepatitis by improving gut barrier function, attenuating inflammation and reducing endotoxin levels. Front. Microbiol. 9:1967. doi: 10.3389/fmicb.2018.01967
Yoshida, H., Yilmaz, C. E., and Granger, D. N. (2011). Role of tumor necrosis factor-alpha in the extraintestinal thrombosis associated with colonic inflammation. Inflamm. Bowel Dis. 17, 2217–2223. doi: 10.1002/ibd.21593
Keywords: Akkermansia muciniphila, microbiota, IBD, DSS-induced colitis, metabolism
Citation: Bian X, Wu W, Yang L, Lv L, Wang Q, Li Y, Ye J, Fang D, Wu J, Jiang X, Shi D and Li L (2019) Administration of Akkermansia muciniphila Ameliorates Dextran Sulfate Sodium-Induced Ulcerative Colitis in Mice. Front. Microbiol. 10:2259. doi: 10.3389/fmicb.2019.02259
Received: 21 May 2019; Accepted: 17 September 2019;
Published: 01 October 2019.
Edited by:
Andrea Masotti, Bambino Gesù Children Hospital (IRCCS), ItalyReviewed by:
Farzam Vaziri, Pasteur Institute of Iran (PII), IranFederica Del Chierico, Ospedale Bambino Gesu’ Roma, Italy
Julio Galvez, University of Granada, Spain
Copyright © 2019 Bian, Wu, Yang, Lv, Wang, Li, Ye, Fang, Wu, Jiang, Shi and Li. This is an open-access article distributed under the terms of the Creative Commons Attribution License (CC BY). The use, distribution or reproduction in other forums is permitted, provided the original author(s) and the copyright owner(s) are credited and that the original publication in this journal is cited, in accordance with accepted academic practice. No use, distribution or reproduction is permitted which does not comply with these terms.
*Correspondence: Lanjuan Li, bGpsaUB6anUuZWR1LmNu