- 1Academic Ophthalmology, Division of Clinical Neuroscience, School of Medicine, University of Nottingham, Nottingham, United Kingdom
- 2Ophthalmology Clinic, University “G. d’Annunzio” of Chieti-Pescara, Chieti, Italy
Pseudomonas aeruginosa (PA) is the leading cause of corneal blindness worldwide. A constant increase in multi-drug resistant PA strains have heightened the challenge of effectively managing corneal infections with conventional antibiotics. Antimicrobial peptides are promising antibiotic analogs with a unique mode of action. Cathelicidin-derived shorter peptides (FK13 and FK16) have previously been shown to kill a range of pathogens in both in vitro and in vivo systems. Here, our aim was to exploit the potential of FK13 or FK16 to enhance the anti-Pseudomonas activity of vancomycin, which normally has low clinical efficacy against PA. Our results have demonstrated that FK16 is more potent than FK13 against different PA strains including a clinical isolate from a patient’s ocular surface. FK16 was shown to enhance the membrane permeability of PAO1 at sub-inhibitory concentrations. Moreover, FK16 at lower concentrations was shown to increase the antibacterial susceptibility of vancomycin against PA strains up to eightfold. The bactericidal synergism between FK16 and vancomycin was shown to be stable in the presence of physiological tear salt concentration and did not cause toxic effects on the human corneal epithelial cells and human red blood cells. Our results have revealed that sub-inhibitory concentration of FK16 could augment the antimicrobial effects of vancomycin against PA. It is anticipated that the future exploitation of the peptide design approach may enhance the effectiveness of FK16 and its application as an adjuvant to antibiotic therapy for the treatment of multi-drug resistant infections.
Introduction
Pseudomonas aeruginosa is a ubiquitous Gram-negative bacterium, which causes opportunistic fatal infections in patients with compromised immunity (e.g., HIV, burn, cancer, and cystic fibrosis) (Poole, 2011). It is also the commonest cause of bacterial keratitis in extended-use contact lens wearers. Pseudomonas keratitis (PsK) is a rapidly progressing disease which frequently leads to irreversible corneal scar or melt (Hazlett, 2007). Due to its nature of the emergency, patients are mostly hospitalized requiring aggressive treatment with mono or fortified topical drops formulations (Otri et al., 2013). However, fortified drops have been shown to cause toxicity, tissue damage and reduce wound-healing (Dua et al., 2012; Austin et al., 2017). Severe PsK cases that require surgical transplantation of donor corneal tissue are often at potential risk of graft tissue rejection (Rush and Rush, 2016). Moreover, constant paucity of transplant tissue and recent emergence of multi-drug resistant (MDR) P. aeruginosa (MDRP) has further added to the challenge to manage PsK (Willcox, 2011; Morita et al., 2014; Vazirani et al., 2015; Tuli and Gray, 2016; Chojnacki et al., 2019).
In developing countries such as India and Brazil, ocular P. aeruginosa isolates have shown increased resistance to ciprofloxacin (25 to 54%), gentamicin (10 to 46%), and cefazolin/ceftazidime (80 to 97%) (Willcox, 2011). Moreover, 23% of cases of Pseudomonas keratitis at a single center in India were shown to be multi-drug resistant with very poor prognosis (Vazirani et al., 2015). In the last two decades, developed countries in North America and Europe have also reported an increased resistance of P. aeruginosa ocular isolates to first-generation cephalosporins, tobramycin, polymyxin B, and fluoroquinolones (Alexandrakis et al., 2000; Willcox, 2011; Asbell et al., 2018). Antimicrobial resistance poses a significant impact on public health and health services globally (Laxminarayan et al., 2013). One of the strategic guidelines of the World Health Organization (WHO) to combat antimicrobial resistance has been to promote judicial use of available antibiotics both in veterinary and hospital settings (Simonsen et al., 2004; Manyi-Loh et al., 2018). Another strategy has been to preserve the effectiveness of existing antimicrobials and the development of newer alternative therapies (O’Neill, 2016; Bloom et al., 2018).
Vancomycin, a tricyclic glycopeptide discovered in the 1950s, has greater specificity toward Gram-positive bacteria and widely used as a last resort treatment for methicillin-resistant Staphylococcus aureus (MRSA) infection (Alvarez et al., 2016; Yarlagadda et al., 2016b). Glycopeptide antibiotics are less preferred as monotherapy for the management of Gram-negative bacterial infections because of poor permeability through the outer membrane (Yarlagadda et al., 2016a). However, once this barrier is breached, for example with polymyxin antibiotic (colistin), vancomycin has shown efficacy against Gram-negative organisms. Clinical effectiveness of colistin-vancomycin combination against MDR Gram-negative infections has been previously demonstrated (Schina et al., 2006; Ceccarelli et al., 2015; O’Driscoll et al., 2018). Recent reports of colistin-resistant P. aeruginosa and potential nephrotoxicity of colistin have discouraged their further use (Poole, 2011; Poirel et al., 2017). This further highlights the clinical need for development and testing of alternative approaches for effective management of P. aeruginosa infections.
Antimicrobial peptides (AMPs) are naturally occurring host-defense molecules with unique microbicidal properties (Mohammed et al., 2017). These are considered to be a promising alternative to antibiotics for the treatment of MDR bacterial infections (Gordon et al., 2005). LL-37 is a lone member of the cathelicidin class of AMPs that is found in humans. It has been shown to display a broad-spectrum microbicidal activity against a range of pathogens (Mookherjee and Hancock, 2007). In addition, it also exhibits intracellular bactericidal and anti-biofilm activities against a variety of Gram-negative and Gram-positive bacteria (Wang et al., 2014; Luo et al., 2017). LL-37 is produced by a variety of immune and non-immune mammalian cells in response to different stimuli which subsequently aid in immunomodulation (Rosenfeld et al., 2006). However, despite its strong properties, the clinical use of LL-37 was limited due to its toxicity and cost of manufacturing. Recent studies have demonstrated that synthetic short peptides derived from LL-37 sequence such as KR12, FK13, and FK16 are capable of killing a variety of pathogens and do not elicit caustic immunologic responses and host tissue toxicity (Wang et al., 2012; Rajasekaran et al., 2017). Based on previous studies, we aimed to test whether these peptides are capable of enhancing the antimicrobial activity of vancomycin against different virulent strains of P. aeruginosa and assess the safety of peptide/antibiotic combination toward host cells.
First, we investigated the antibacterial efficacy of cathelicidin-derived shorter peptides and antibiotics in presence of the physiological salt concentration of tears, against a collection of P. aeruginosa strains including a clinical isolate from a patient with Pseudomonas keratitis. Next, we examined whether sub-inhibitory concentrations of FK13 or FK16 would increase the efficacy of vancomycin against P. aeruginosa. Finally, we assessed the cytotoxic effects of peptides and the combination of peptides and vancomycin on human corneal epithelial cells and human red blood cells (RBCs).
Materials and Methods
As a first step, we tested commercially synthesized peptides [LL-37, FK13, and FK16 (200 to 0.78 μg/mL)] and antibiotics [gentamicin (64 to 0.5 μg/mL); amikacin (16 to 0.125 μg/mL), and vancomycin (512 to 4 μg/mL)] in broth-microdilution and growth-inhibition assays, in presence or absence of physiological tear salt concentration for elucidation of minimum inhibitory concentration (MIC) and IC50 against three virulent strains of P. aeruginosa. Bactericidal activity of FK16 was further tested by the SYTOX-green dye uptake assay, using a known membrane-disruptor, melittin as control. Next, the optimum concentration of FK16 and vancomycin was tested for synergism against all PA strains by determining fractional-inhibitory concentration (FIC) index in the presence or absence of physiological tear salt concentration. Gentamicin and amikacin were used as the positive controls. Lastly, we determined the toxicity of FK16 and vancomycin alone or in combination against human corneal epithelial cells (HCE-2) using a cell viability dye assay. The individual methodologies are detailed below.
Bacterial Strains, Peptides, and Antibiotics
Three P. aeruginosa strains were used in this study. PAO1-L (Lausanne sub-line), an invasive strain, was procured from Dr. Stephan Heeb, School of Life Sciences, University of Nottingham, United Kingdom. P. aeruginosa ATCC 19660, which is cytotoxic, was obtained commercially from ATCC-LGC Standards, United Kingdom. A clinical isolate of P. aeruginosa (PA-OS) from scrapes of human corneal surface with severe corneal melt was obtained from the Department of Clinical Microbiology, Nottingham University Hospitals, United Kingdom. All the work in this study was conducted as per the Health and Safety laboratory guidelines under the Biological safety standards of the University of Nottingham. Cation-adjusted Mueller-Hinton broth (MHB-II), Mueller-Hinton agar (MHA), and Tryptic soy agar (TSA) were purchased from Sigma-Aldrich, United Kingdom. P. aeruginosa selective agar F was procured from Merck Millipore, United Kingdom. LL-37 (LLGDFFRKSKEKIGKEFKRIVQRIKDFLRNLVPRTES), FK13 (FKRIVQRIKDFLR) and FK16 (FKRIVQRIKDFLRNLV) were purchased from Anaspec, Fremont, CA, United States. Gentamicin and Amikacin were commercially obtained from Sigma-Aldrich, United Kingdom. Vancomycin Hydrochloride was procured from MP Biomedicals, France.
Broth Microdilution Assay
Minimum inhibitory concentration was determined for peptides and antibiotics in duplicate using broth microdilution assay according to CLSI guidelines (Wiegand et al., 2008). Twofold serial dilution of peptides and antibiotics were made in duplicate across the 96-well polypropylene microtiter plate using acidified water (0.01% Acetic acid and 0.1% Bovine serum albumin). This resulted in 10 μL of 10x concentration of peptides or antibiotics (gentamicin, amikacin, and vancomycin) in each well. Three to five colonies of PA strain from MHA culture plate were incubated in 5 mL MHB-II in an orbital shaker at 35°C for 16 h. The overnight cultures were sub-inoculated in fresh 5 mL MHB-II at 1 in 50 dilutions and incubated for 3–4 h in the shaking incubator at 35°C until OD600 readings gave approximately 2 × 108 CFU/mL. A suspension of 1 × 106 CFU/mL in fresh MHB-II was prepared by further dilution and 90 uL of this suspension was then transferred to each well-containing 10 uL of 10x peptide or antibiotics which resulted in final inoculum of 5 × 105 CFU/mL with desired final concentration of peptide (range 200 to 0.78 μg/mL) or antibiotics [gentamicin (64 to 0.5 μg/mL); amikacin (16 to 0.125 μg/mL), and vancomycin (512 to 4 μg/mL)]. Growth control (only bacteria) and sterility control (no bacteria) were also included in each plate. The plate was incubated at 37°C for 18–21 h and the MIC was determined as the lowest concentration of peptide or antibiotic that inhibited the visible growth of PA strain as observed with the unaided eye.
Growth Inhibition Assay
Three to five colonies of a PA strain were incubated overnight in 5 mL MHB-II at 35°C in an orbital shaker. The overnight bacterial culture was then diluted to 1 in 50 in fresh MHB-II (5 mL) and further incubated for 3–4 h at 35°C in an orbital shaker to achieve 0.3 OD600 (approximately 2 × 108 CFU/mL). Twofold serial dilution of each peptide or antibiotic (gentamicin, amikacin, and vancomycin) in duplicate at 2x concentration in 100 μL was prepared in acidified water containing 0.002% polysorbate-80 (to prevent binding of cationic peptides to the anionic surface of the plate) in a 96-well microtiter polystyrene plate. To each well, 100 μL of bacterial suspension containing 1 × 106 bacteria/mL in MHB-II/0.002% polysorbate-80 was added, which resulted in the inoculum of 5 × 105CFU/mL per well in 200 μL final volume. In addition, individual wells containing vehicle control (1% DMSO) and positive control (50 μg/mL melittin) was also prepared. The plate was incubated at 37°C for 21 h in BMG Clariostar microplate reader and OD600 measurements were recorded at 30 min intervals. Two independent experiments were performed for each peptide and antibiotic. Percent growth of bacteria at 13 h for each concentration of peptide and antibiotic was estimated. The IC50 value (concentration at which growth reduced to 50%) was then derived from non-linear regression curves using the GraphPad Prism (version 8.0). Growth inhibition assay was also performed for different concentrations of vancomycin (512 to 0 μg/mL, twofold decreasing serial dilutions) against all the three PA strains alone or in combination with FK16 peptide at 0.5x MIC (50 μg/mL) and 0.25x MIC (25 μg/mL), respectively.
Time-Kill Assay
An overnight culture, each of PAO1-L and PA19660 (approximately 16–18 h) was diluted 50-fold (1 in 50) in fresh MHB-II and further incubated at 35°C in an orbital shaker for 3–4 h until 0.3 OD600 (approximately 2 × 108 CFU/mL) was achieved. PAO1-L suspension (2 × 106 CFU/mL) was incubated with FK16 peptide (2 or 0.5x MIC) with or without vancomycin (128 or 256 μg/mL) at 35°C in an orbital shaker. At 0 (starting), 0.5, 3, and 24 h time-points, 10 μL of the mixture was aliquoted and serially diluted in sterile 1x phosphate-buffered saline (PBS), pH 7.2. The diluted bacteria were spread on MHA plates in duplicate and incubated at 37°C for 24 h. Colony count (CFU/mL) was performed and the concentration of FK16 or vancomycin (alone or in combination) was considered bactericidal if there was a ≥ 3 log10 reduction in CFU/mL.
SYTOX-Green Dye Uptake Assay
SYTOX-green does not permeate viable bacteria, but once inside the cytoplasm it binds the nucleic acids and emit fluorescence (λEX = 488 nm; λEM = 520 nm). PAO1 and PA19660 were grown overnight in MHB-II medium and further sub-cultured to a mid-logarithmic growth phase (up to 3 h) at 35°C in an orbital shaker. The suspension was spun at 3000 rpm for 10 min, washed with PBS and diluted to 0.05 OD600 in 10% MHB2. To the diluted bacteria, SYTOX-green dye at a final concentration of 2 μM was added and incubated for 15 min. A 96-well black propylene plate containing a mix of 100 μL of bacteria-SYTOX green suspension and 100 μL of FK16 (at 2x concentrations) or melittin (20 μg/mL; positive control) or PBS (negative control) in each well was placed in a fluorescence plate reader (BMG Clariostar) for 30 min. The relative fluorescence unit (RFU) was analyzed by deducting fluorescence from PBS treated bacteria. Two independent experiments were performed in duplicates for each strain.
Checkerboard Assay
The combined activity and interactions (synergism, sum of activities, or antagonism) between peptides and vancomycin against PA strains in presence or absence of 150 mM NaCl (physiological salt concentration) was determined using checkerboard assay method (7 × 7 matrix format). 25 μL of test peptide at 4x concentration was serially diluted (twofold) in decreasing concentration (final 160 to 0 μg/mL) from column 2 to 8 of a 96-well microtiter polypropylene plate (labeled as ‘Main plate’). In another 96-well plate (labeled as ‘Helper plate’), 25 μL of vancomycin at 4x concentration was prepared in twofold step dilutions (final 512 to 0 μg/mL) from row B to H. The contents from each well of the helper plate was then transferred to the corresponding wells of the main plate to set up an equal 1:1 mix of peptide and vancomycin. The bacterial suspension was prepared (as described in above sections) and diluted to 1 × 106 CFU/mL in fresh MHB-II. 50 μL of diluted suspension (containing 0 or 300 mM NaCl) was then added to each well, resulting in final inoculum of 5 × 105 CFU/mL in 100 μL total volume (final 0 or 150 mM NaCl). Positive control (melittin 50 μg/mL), vehicle control (1% DMSO), and sterility control (no bacteria) was also included in all assay plates. After 21 h of incubation at 37°C, the plates were visually examined for growth. The FIC index for combination of each peptide and vancomycin (in MHB-II ± 150 mM NaCl) against each PA strain was calculated as [(MIC of peptide in combination with vancomycin)/(MIC of peptide alone)] + [(MIC of vancomycin in combination with peptide)/(MIC of vancomycin alone)]. The results were interpreted as FIC ≤ 0.5, synergistic; 0.5 < FIC ≤ 1, additive; 1 < FIC ≤ 4, indifferent; FIC > 4, antagonistic (Ng et al., 2018).
Cytotoxicity Assay
Human corneal epithelial cells (HCE-2, ATCC) were cultured in keratinocyte serum-free media (KSFM) supplemented with human recombinant epidermal growth factor and bovine pituitary extract. HCE-2 cells were seeded into a 96-well plate at a density of 7.5 × 103 cells per well and allowed to attach overnight. After 24 h of treatment with different concentration of peptides and vancomycin (alone or in combination) in duplicate, the viability of cells was measured using CCK-8 assay kit (Sigma, United Kingdom) as per manufacturer’s guidelines. The WST-8 reagent was bio-reduced by the viable cells into a colored formazan product, which was measured at OD450 using BMG Clariostar microplate reader. Percent viable cells was calculated as [(OD450 of cells treated with peptide or antibiotic)/(OD450 of untreated cells)] x 100.
Hemolysis Assay
Human blood for hemolysis assay was collected from the healthy subjects with prior consent under the approved ethics (Reference No. 176-1812) from the local Research Ethics Committee of the Faculty of Medicine and Health Sciences, University of Nottingham. Blood was collected in EDTA-coated tube and centrifuged for 10 min at 1300 × g for separation of plasma. RBCs were rinsed three times in Ca2+/Mg2+ free PBS by centrifugation for 5 min at 1300 g. RBCs were diluted to 4% vol/vol in PBS and incubated with 100 μL of LL-37 and FK16 (200 to 0.78 μg/mL), vancomycin alone (512 to 2 μg/mL) and in combination with FK16 (50 μg/mL), 1% Triton X-100 (positive control) and PBS (negative control) for 1 h at 37°C in a U-bottom polypropylene 96-well plate. The plate was then centrifuged at 1300 × g for 10 min and 100 μL of supernatant was transferred into 96-well polystyrene plate. The absorbance was measured at 540 nm for estimation of percentage hemolysis compared to the positive control. Percent lysis was calculated as [(OD540 of suspension from RBC treated with peptide and antibiotic alone or in combination – OD540 of suspension from negative control-treated RBCs)/(OD540 of suspension treated with positive control – OD540 of suspension from negative control-treated RBCs)] x 100.
Results
Anti-Pseudomonas Activity of Peptides and Antibiotics
LL-37 and its two derivatives, FK13 and FK16, and a range of antibiotics that are commonly used for the treatment of bacterial keratitis were tested against three different strains of Pseudomonas aeruginosa (PAO1, PA19660, and PA-OS). The biochemical and structural details of peptides and antibiotics was depicted in the Supplementary Table 1. As shown in Table 1, LL-37 demonstrated higher bactericidal activity against all PA strains when compared to FK16 (50 vs. 100 μg/mL) and FK13 (50 vs. 200 μg/mL), respectively. Of the two shorter peptides, FK16 showed stable bactericidal activity against PAO1, PA19660, and PA-OS. All antibiotics, except vancomycin (>256 μg/mL), showed potent anti-Pseudomonas activity. Amikacin, in particular, demonstrated a fourfold higher bactericidal activity compared to gentamicin. However, in the presence of physiological salt concentration (150 mM NaCl), the MIC of gentamicin, amikacin, LL-37, and FK16 was reduced twofold against all tested strains. FK13 was shown to be ineffective at the highest test concentration (200 μg/mL) in the presence of salt against PA19660 and PA-OS indicating that there was no difference in the behavior of FK13 and FK16 with respect to reduction of activity in the presence of salt.
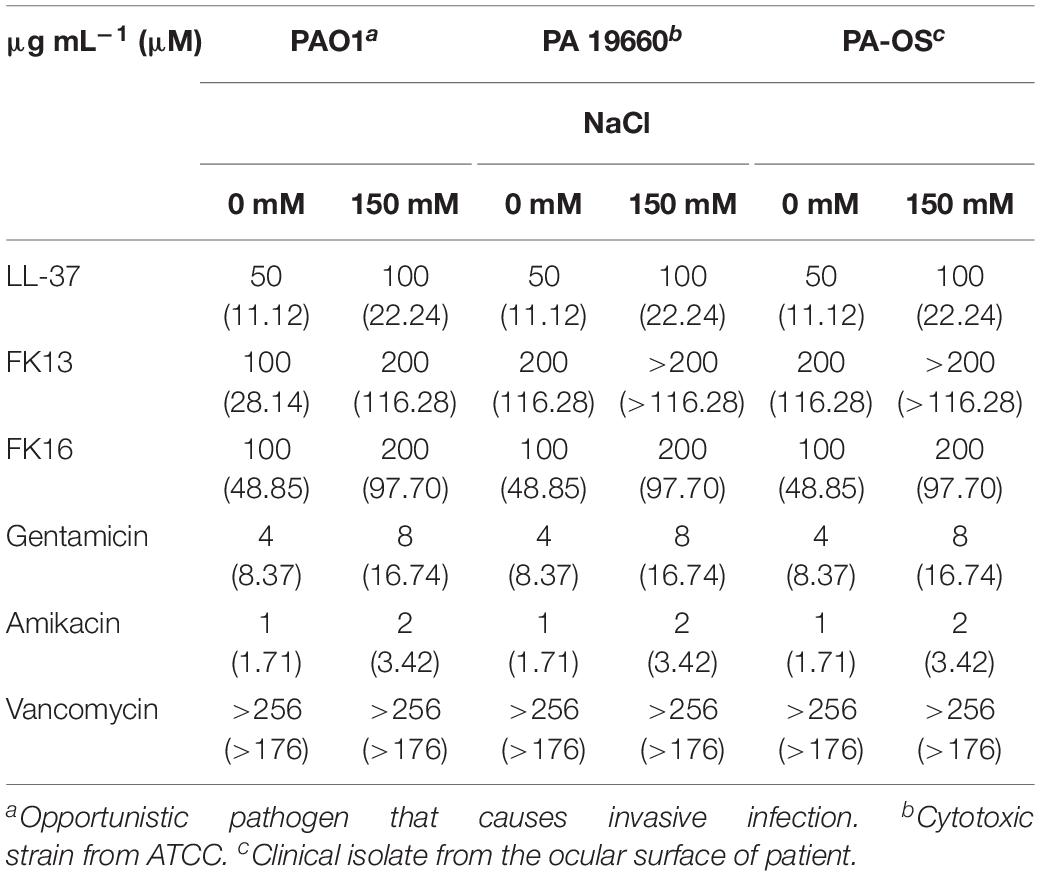
Table 1. Minimum inhibition concentration (MIC) of the antimicrobial peptides and antibiotics against three different Pseudomonas aeruginosa strains.
As an alternative dynamic methodology to static MIC (Ng et al., 2018), the efficacy of FK16 and FK13 against all PA strains was tested using kinetic growth-inhibition assay. The sigmoidal dose-response curves for FK16 (Figure 1A) showed sharp decrease in percentage growth of PAO1 (IC50 = 21.3 ± 3.5 μg/mL), PA19660 (IC50 = 29 ± 4.2 μg/mL) and PA-OS (IC50 = 27 ± 3.2 μg/mL). In contrast, FK13 (Figure 1B) showed > 2-fold reduced activity compared to FK16 in inhibiting PAO1 (IC50 = 49.3 ± 4.2 μg/mL), PA19660 (IC50 = 89.5 ± 6 μg/mL), and PA-OS (IC50 = 91.5 ± 8 μg/mL). Against PAO1, LL-37 (IC50 = 16.3 ± 6.2 μg/mL), gentamicin (IC50 = 3 ± 1.5 μg/mL), and amikacin (IC50 = 0.5 ± 0.1 μg/mL) all showed higher killing activity (Supplementary Figure 1 and Supplementary Table 2). However, vancomycin required a higher concentration to inhibit PAO1 growth (IC50 > 291 μg/mL).
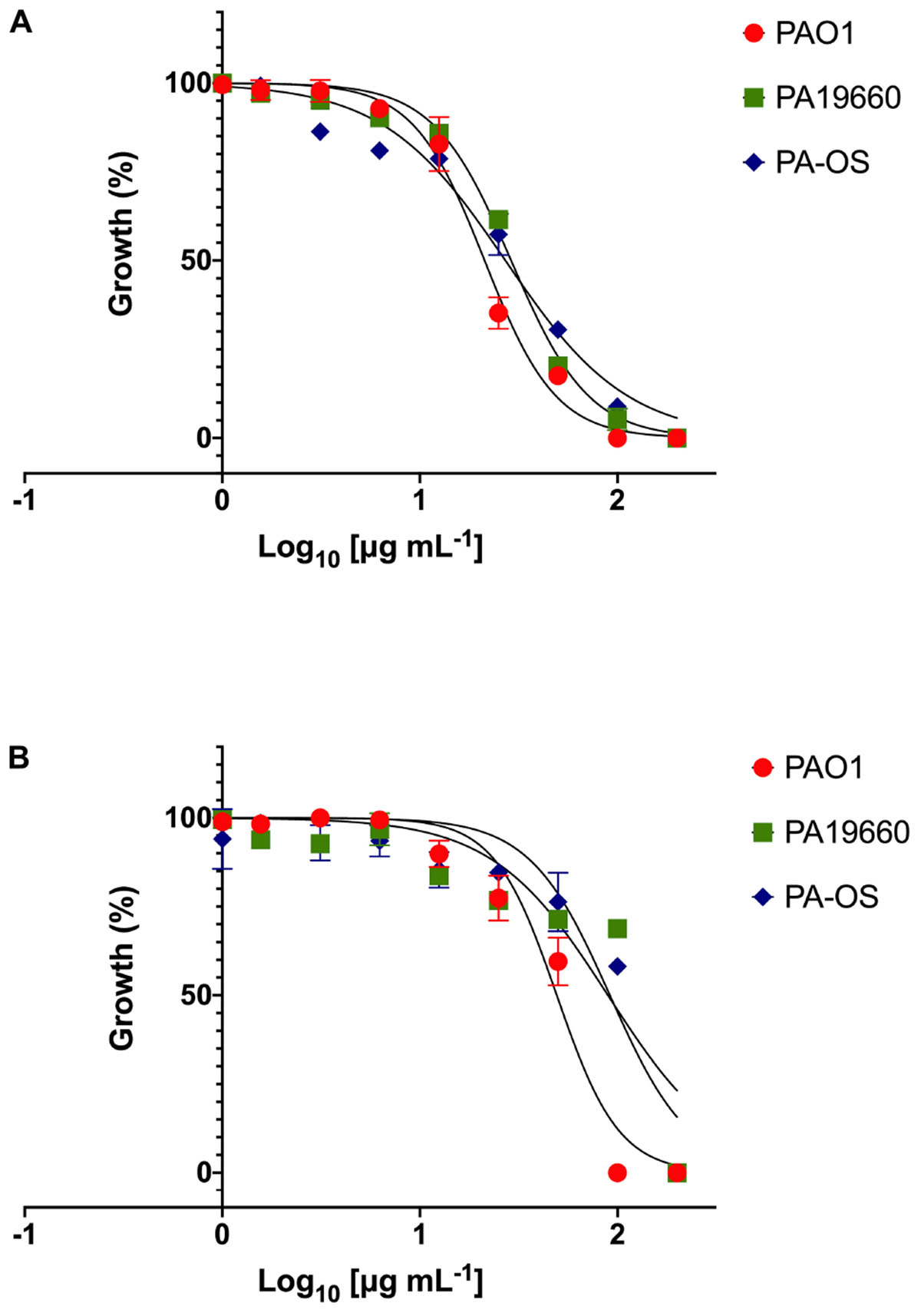
Figure 1. Dose response curves of FK16 (A) and FK13 (B) against PAO1, PA19660, and PA-OS. Percentage growth is presented as mean ± standard deviation (SD) of two independent experiments performed in triplicate (n = 6 data sets). The IC50 values were depicted in the Table 2. Some of the error bars are missing due to smaller SD.
GF-17 (also known as N-glycinated FK16) has been previously shown to kill Escherichia coli via membrane-disruption (Wang et al., 2017). To validate whether the FK16 also kills P. aeruginosa utilizing a similar mechanism, we performed the SYTOX-green dye uptake assay. The increased intensity of fluorescence corresponds to the membrane disruption and binding of the dye to the nucleic acids of the bacterium. As shown in Figure 2A, the influx of SYTOX-green dye in PAO1 was directly proportional to the concentration of FK16 and the maximum relative fluorescence was noted at 1x MIC. Melittin, a known membrane disruptor from honey-bee venom, was used as a positive control. We further assessed the killing efficacy of FK16 (1, 0.5, and 0.25x MIC) against PAO1 at 5-min interval up to 30 min and then at 60 min and 24 h, respectively. As shown in Figure 2B, FK16 at 1x MIC was shown to kill PAO1 at 60 min with no growth noted up to 24 h. Whilst LL-37 at 1x MIC was shown to kill PAO1 at 30 min.
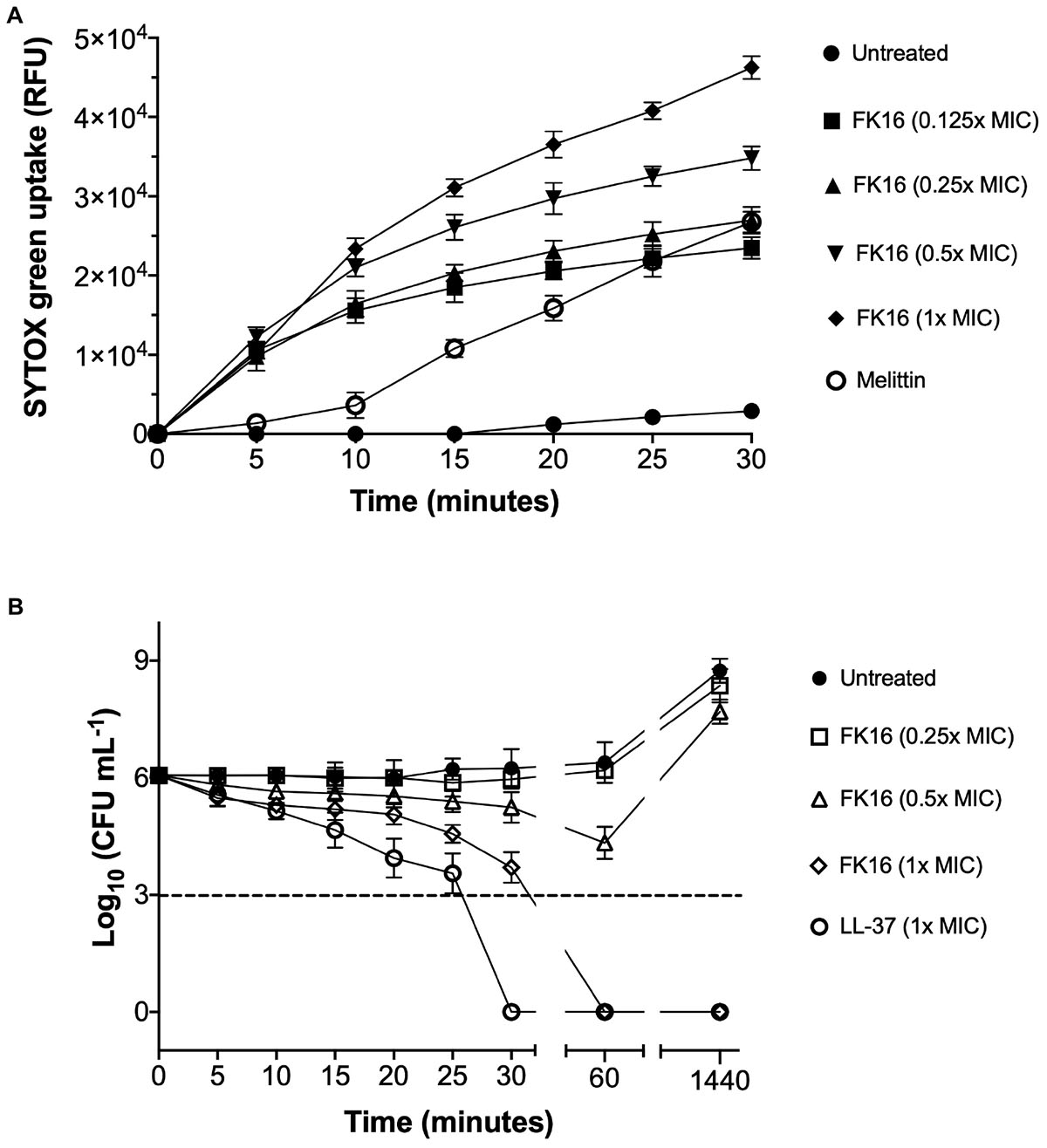
Figure 2. Killing mechanism of FK16 against PAO1. (A) Fluorometric analysis of SYTOX-green dye uptake in PAO1. Fluorescence emission was recorded at λ = 520 nm at 5 min interval up to 30 min. Relative fluorescence unit (RFU) vs. time plots for different concentrations of FK16 (in μg/mL), Melittin (20 μg/mL), or PBS control (untreated) were constructed. Data representing three independent experiments performed in duplicate. (B) Killing kinetics of PAO1. Bacteria were treated with vehicle (closed circle), FK16 at 1x MIC (open diamond), 0.5x MIC (open triangle) and 0.25x MIC (open square) and LL-37 at 1x MIC (open circle) for 5, 10, 15, 20, 25, 30, 60, and 1440 min, respectively. ‘0 h’ represents the starting inoculum. Colonies were counted (CFU/mL) 24 h post-treatment from serial dilutions in duplicate and presented in a logarithmic scale. Data is presented as mean ± standard deviation (SD) of three independent experiments. Some of the error bars are missing due to smaller SD.
Antimicrobial Effect of Peptides and Vancomycin Combination Against P. aeruginosa
As demonstrated above, vancomycin failed to exhibit significant bactericidal activity against P. aeruginosa (MIC > 256 and IC50 > 291 μg/mL). This is due to poor permeation of vancomycin through the outer membrane of P. aeruginosa. Based on the ability of FK16 to modulate membrane permeability, we hypothesized that in combination with FK16 the antimicrobial susceptibility of vancomycin against P. aeruginosa could be augmented. To test this hypothesis, we performed the growth-inhibition assay for the combination of FK16 (at sub-MIC levels; 25 and 50 μg/mL) and vancomycin (512 to 0 μg/mL in twofold serial dilution) against all three PA strains. Kinetic kill curves (Supplementary Figure 2) were plotted using OD600 readings that were recorded at 30 min intervals up to 21 h. The normalized percentage growth of each strain at 13- and 21-h time point was then calculated for analysis of FK16 + vancomycin combination against P. aeruginosa.
As shown in Figure 3 (orange bars), at 21-h time point, FK16 (25 μg/mL) enhanced the killing efficacy of vancomycin by eightfold (512 vs. 64 μg/mL) against PAO1 (Figure 3D), PA-19660 (Figure 3E), and PA-OS (Figure 3F). Moreover, at 25 μg/mL FK16 + 64 μg/mL vancomycin combination, >99.5% normalized growth of PAO1 (Figure 3D) and >95% of both PA19660 (Figure 3E) and PA-OS (Figure 3F) were shown to be inhibited. To assess whether the bactericidal activity of vancomycin is dependent on FK16 concentration, we increased the concentration of FK16 to 50 μg/mL (0.5x MIC) in the growth inhibition assay. We have noted an 16-fold improvement in bactericidal activity against PAO1 [512 vs. 32 μg/mL; (Figure 3D, cyan bars)] and eightfold improvement against PA19660 and PA-OS (512 vs. 64 μg/mL; Figures 3E,F, cyan bars), respectively.
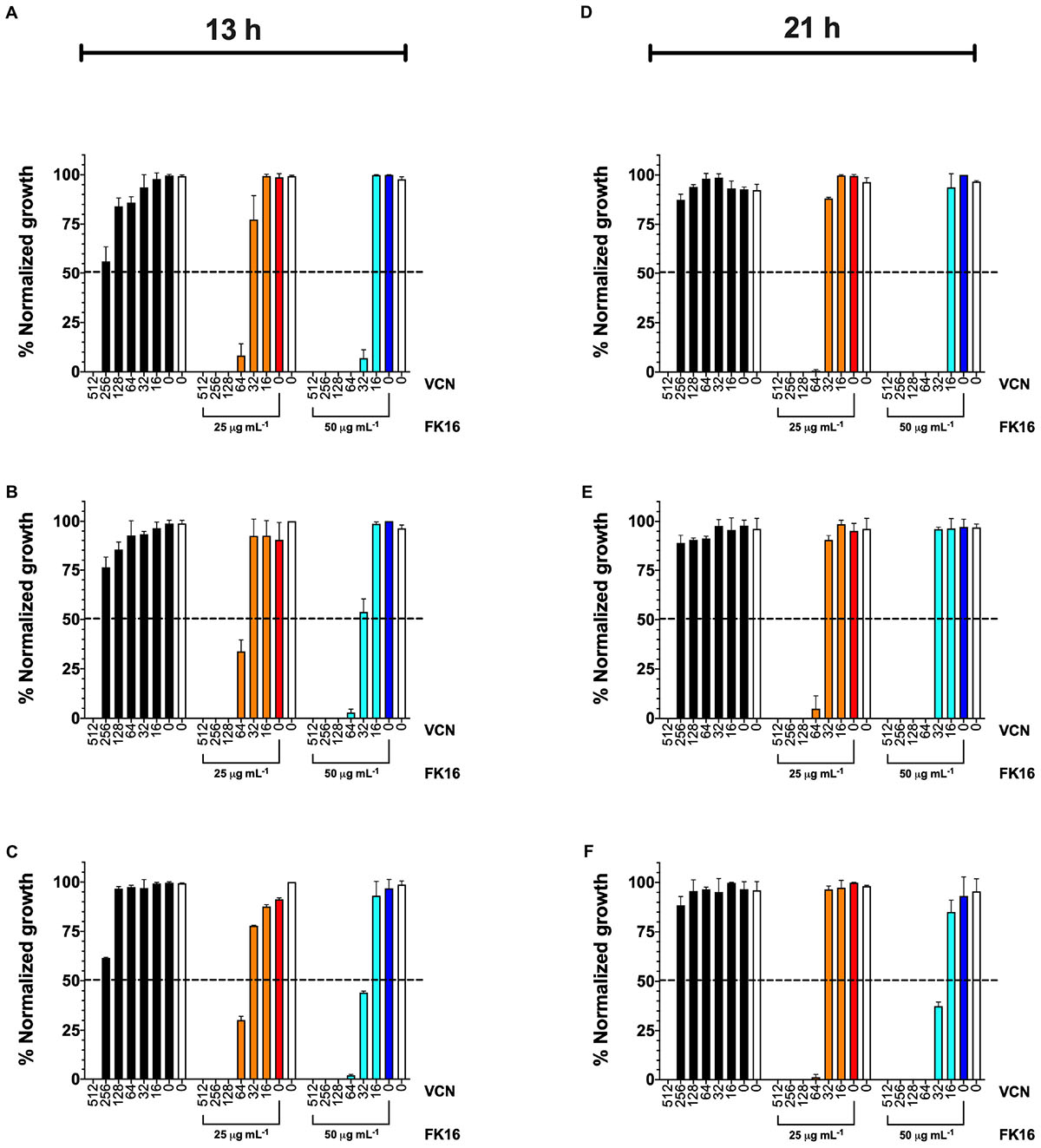
Figure 3. Normalized percentage growth of PAO1 (A,D), PA-19660 (B,E), and PA-OS (C,F) from OD600 recordings at 13-h (early stationary phase) and 21-h (late stationary phase) time point following treatment with FK16 and vancomycin combination. Vancomycin (VCN) alone treatment (512 to 0 μg/mL) is represented with black bars. VCN (512 to 0 μg/mL) + FK16 at 25 μg/mL (orange bars) and VCN (512 to 0 μg/mL) + FK16 at 50 μg/mL (cyan bars). Vehicle treatment (clear bar) and FK16 alone at 25 μg/mL (red bar) and 50 μg/mL (blue bar). Data is presented as mean ± standard deviation (SD) of two independent experiments performed in triplicate (n = 6 data sets). Some of the error bars are missing due to smaller SD. Inhibition is seen up to the 21-h time point (D–F).
We further validated the killing kinetics of FK16-vancomycin combination against PAO1 and PA19660 in a time-kill assay. Gentamicin, which was used as a positive control (5x MIC; 20 μg/mL) and FK16 at twofold above the MIC (200 μg/mL) have been shown to completely kill PAO1 (Figure 4A) and PA19660 (Figure 4B) at 45 min. Notably, FK16 (50 μg/mL; sub-MIC) in combination with vancomycin (128 μg/mL) have been shown to completely inhibit the growth of both strains at 2 h and demonstrated sustained activity up to 24 h. With combination of FK16 (50 μg/mL; sub-MIC) and vancomycin (256 μg/mL; twofold higher concentration), PAO1 (Figure 4A) and PA19660 (Figure 4B) were shown to be reduced > 3 log10(CFU/mL) within 60 min and complete kill achieved by 2 h with no growth noted up to 24 h.
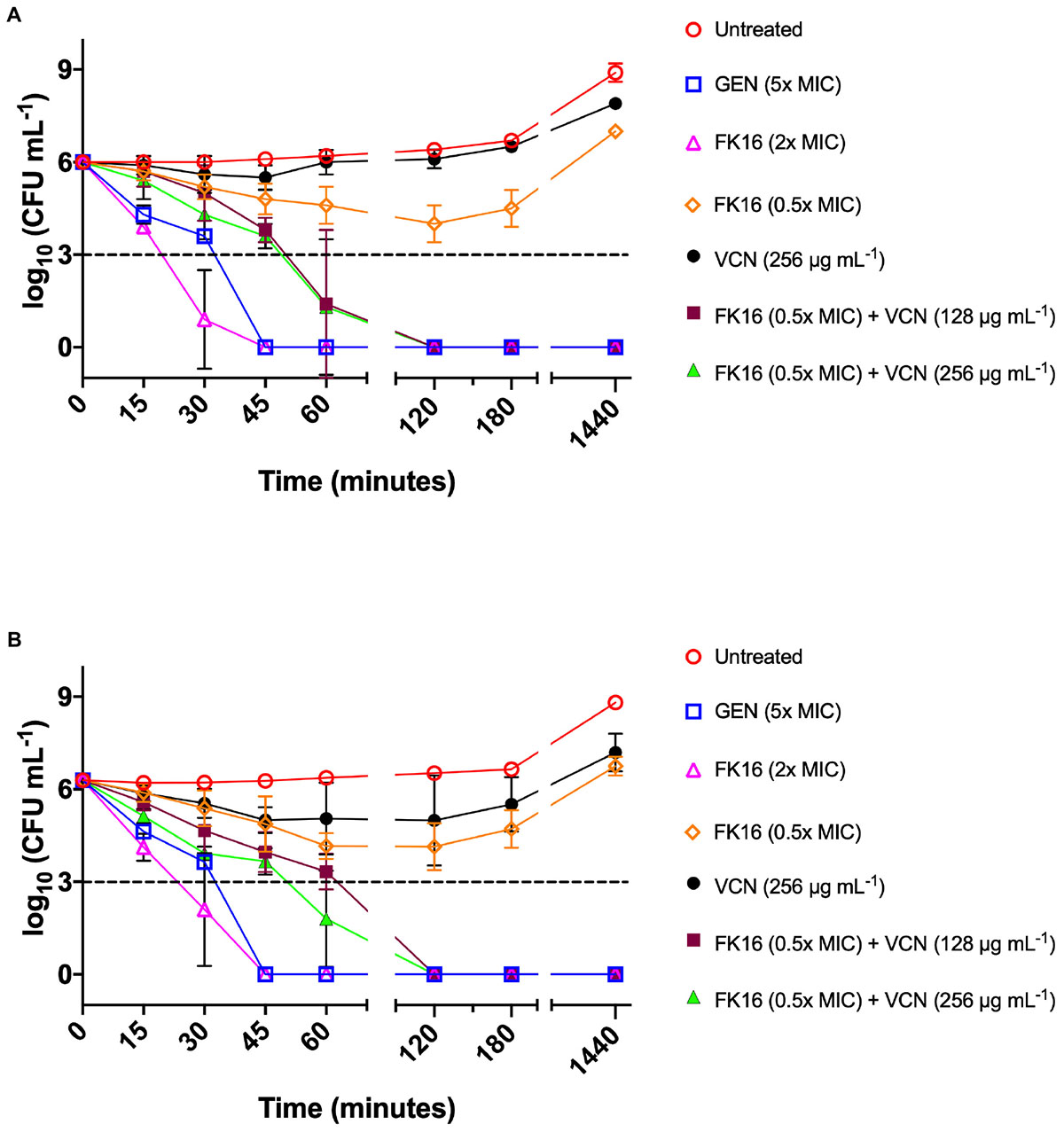
Figure 4. Time-kill curves of FK16 and vancomycin alone or in combination against PAO1 (A) and PA19660 (B). Bacteria were treated with FK16 alone (2x MIC; open magenta triangle) and (0.5x MIC; open orange diamond), VCN alone (256 μg/mL; closed black circle), FK16 (0.5x MIC) + vancomycin (VCN; 128 μg/mL closed brown square), FK16 (0.5x MIC) + VCN (256 μg/mL; closed green triangle), Gentamicin (5x MIC; open blue square) and vehicle (open red circle) for 15, 30, 45, 60, 120, 180 and 1440 min, respectively. ‘0 minutes’ represents the starting inoculum. Colonies were counted (CFU/mL) 24 h post-treatment from serial dilutions in duplicate and presented in a logarithmic scale. Data is presented as mean ± standard deviation (SD) of three independent experiments. Some of the error bars are missing due to smaller SD.
To establish a synergistic or additive effect of the FK16 and vancomycin combination, a MIC-based checkerboard assay against all three PA strains was performed. In addition, we have also examined the combination effect in the presence of 150 mM NaCl. As depicted in Table 3, FK16-vancomycin combination has demonstrated synergistic bactericidal activity against PAO1 (FICI = 0.25), PA19660 (FICI = 0.375), and PA-OS (FICI = 0.375), respectively. In the presence of physiological salt, combination of FK16 and vancomycin remained synergistic against PAO1 (FICI = 0.375). Although the combination effect against PA19660 (FICI = 0.50) and PA-OS (FICI = 0.50) have been slightly reduced in assay buffer containing 150 mM NaCl, the FIC indices against both strains were still at the borderline between synergism and additive level.
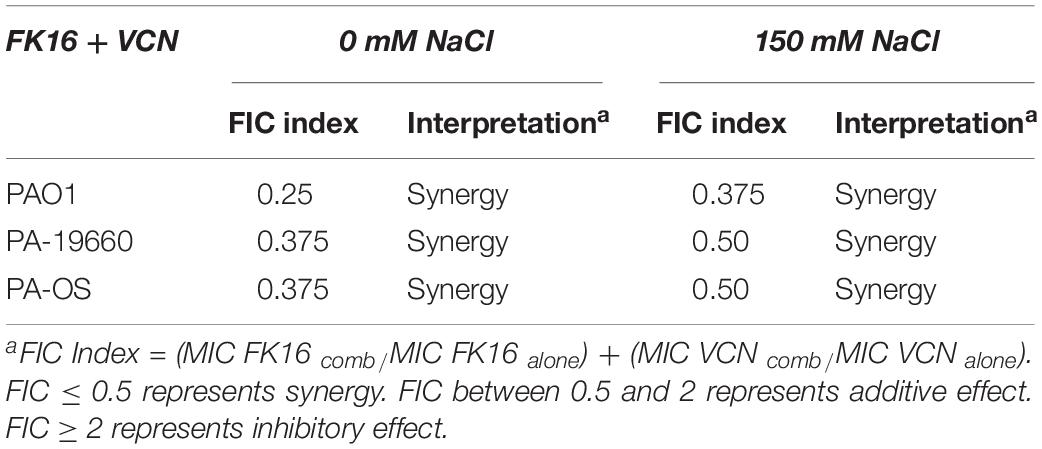
Table 3. Effect of FK16 and Vancomycin combination in presence and absence of physiological salt concentration.
Potential Toxicity of Peptides and Vancomycin Toward Human Corneal Epithelial Cells and Human Red Blood Cells
Antimicrobial peptides at higher concentrations have been shown to elicit toxic responses on host tissue. This non-selective effect of AMPs has therefore limited their clinical application (Haney et al., 2019). Here, we evaluated the potential cytotoxic effects of cationic peptides (LL-37, FK13, and FK16), vancomycin and different concentrations of FK16 in combination with vancomycin on human corneal epithelial cells (HCE-2, ATCC) until 24 h treatment duration. As shown in Figure 5A, LL-37 has demonstrated significant cytotoxic effects on HCE2 (EC50 = 43.20 ± 4.08 μg/mL). Notably, EC50 value of LL37 was matching to its 1x MIC levels against P. aeruginosa (Table 1). FK13 and FK16 were shown to be non-toxic to HCE2 (EC50 > 200 μg/mL; Table 4). Similarly, treatment of HCE2 for 24 h with different concentrations of vancomycin either alone (EC50 > 512 μg/mL) or in combination with FK16 at 25 μg/mL (EC50 > 512 μg/mL) or 50 μg/mL (EC50 > 512 μg/mL) have not elicited toxic effects (Figure 5B).
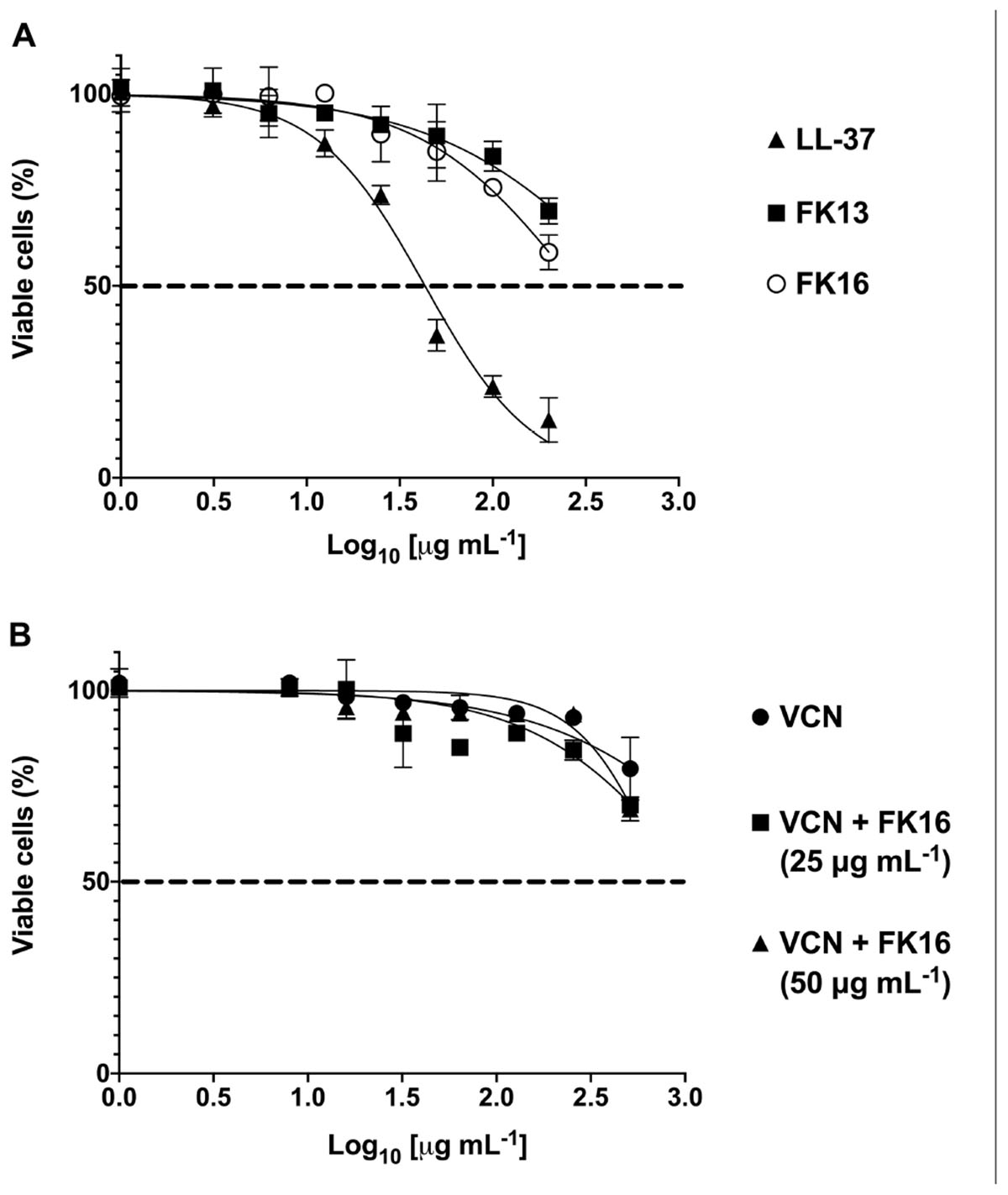
Figure 5. Toxic effects of peptides (A) and vancomycin (VCN) + FK16 combination (B) against human corneal epithelial cells (HCE-2). After 24 h of treatment with different concentration of peptides alone or in combination with different concentration of VCN, HCE2 viability was assessed with CCK8 reagent. EC50 and Vmax was derived (Table 4) from the percentage viable cell curves using GraphPad Prism (ver. 8.0). Data is presented as mean ± standard deviation (SD) of three independent experiments performed in duplicate. Some of the error bars are missing due to smaller SD.
We also assessed the hemolytic effect of peptides and vancomycin alone or in combination on human red blood cells (hRBC). LL-37 displayed 25.03 ± 1.97 percent lysis of hRBC at 256 μg/mL (Figure 6). Whereas FK16 exhibited 13.61 ± 3.29% lysis at 256 μg/mL. Vancomycin alone (512 to 2 μg/mL) or in combination with FK16 (50 μg/mL) did not induce significant hemolysis.
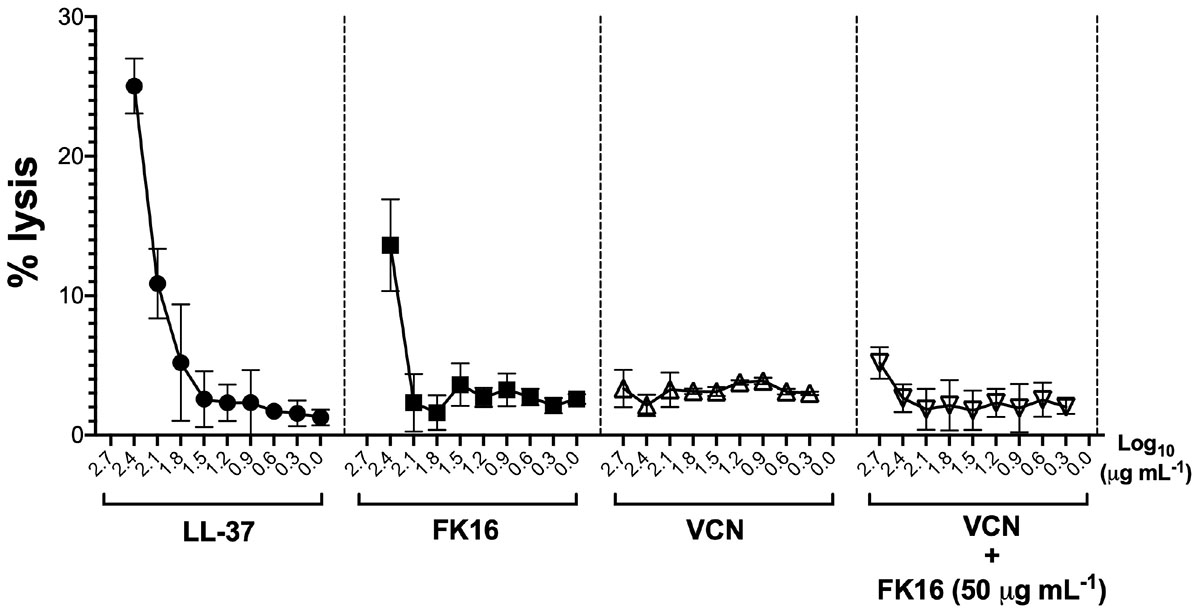
Figure 6. Lytic effect against human red blood cells (RBCs). After 1 h of treatment with different concentration of LL-37 (closed circle; 200 to 0 μg/mL), FK16 alone (closed square; 200 to 0 μg/mL), VCN alone (upright open triangle; 512 to 8 μg/mL) and in combination with FK16 at 50 μg/mL (inverted open triangle), hemolysis was assessed by measuring optical absorbance of supernatant at 540 nm. Percentage lysis curves were plotted using GraphPad Prism (ver. 8.0). Data is presented as mean ± standard deviation (SD) of three independent experiments performed in duplicate. Some of the error bars are missing due to smaller SD.
Discussion
The growing threat of MDR P. aeruginosa has profoundly affected a diverse patient cohort and health services worldwide. A key strategy to counter the antimicrobial resistance would be to exploit the potential of AMPs to improve the effectiveness of conventional antibiotics (Hollmann et al., 2018). This approach was deemed unique because of the differences in the mechanisms of action of AMPs compared to antibiotics.
In the present work, we have selected LL-37 and its shorter peptides on the basis of previous findings from our and another laboratories (McIntosh et al., 2005; Huang et al., 2006, 2007; Wang et al., 2012, 2014; Rajasekaran et al., 2017). LL-37 was shown to be abundantly expressed on the human ocular surface in response to P. aeruginosa (McIntosh et al., 2005; Huang et al., 2006). Moreover, its genetic deletion in mouse has been shown to increase the susceptibility to Pseudomonas keratitis (Huang et al., 2007). Thus, there is sufficient evidence in the literature to justify a study to explore the efficacy and safety of AMPs or AMP-derived peptides in isolation and in combination with antibiotics.
Published results from the Steroids for Corneal Ulcers Trial (SCUT) have shown that the corneal ulcers with genotypically invasive P. aeruginosa subgroup have marked differences in the clinical presentation and responses to treatment when compared to genotypically cytotoxic P. aeruginosa subgroup (Borkar et al., 2013). A subsequent report from the SCUT study has further demonstrated that exoU(+) encoding cytotoxic P. aeruginosa isolates were significantly resistant to ciprofloxacin, gatifloxacin, and ofloxacin compared to exoU(−) P. aeruginosa strain (Borkar et al., 2014). This illustrates that the specific virulence determinants of a single species of a pathogen respond differently to treatment. Although LL-37 was found to be twofold more potent than its shorter peptides against P. aeruginosa, its detrimental effect on corneal epithelial cells made it undesirable for further synergism experiments in the context of ocular surface infections. Our results agree with earlier studies which also demonstrated the toxicity of LL-37 against RBCs and a variety of human cell lines (Jaskiewicz et al., 2018; Haney et al., 2019).
Structure-activity relationship (SAR) studies have enabled the development of short fragments of LL-37 with improved cell selectivity (Mookherjee and Hancock, 2007; Mishra et al., 2013; Wang et al., 2014). It was shown that the region between 2 and 31 residues of LL-37 is important for antibacterial activity (Li et al., 2006). A recent study utilizing nuclear magnetic resonance (NMR) has confirmed that the amino acid residues between 17 and 32 (i.e., FK16) have strong binding affinity toward anionic (bacterial) but not zwitterionic (host) model membranes (Wang et al., 2012). Moreover, FK16 has been shown to exhibit strong bactericidal activity against ESKAPE organisms (Enterococcus faecium, Staphylococcus aureus, Klebsiella pneumoniae, Acinetobacter baumanii, Pseudomonas aeruginosa, and Enterobacter spp.) (Mishra and Wang, 2017). GF17, a N-glycinated variant of FK16, has also been shown to exhibit anti-cancer (Ren et al., 2013), anti-bacterial (Wang et al., 2012; Kiattiburut et al., 2018) and anti-viral activities (He et al., 2018). It was further confirmed that GF17 and its structurally modified variants were able to kill a variety of Gram-negative and Gram-positive bacteria via membrane disruption (Wang et al., 2017). In addition, GF17 and 17BIPHE2 (second generation peptide based on GF17 structure) precoated biomaterials were shown to prevent bacterial biofilm formation both in in vitro and in vivo model systems (Mishra and Wang, 2017). Consistent with the above reports, we have also demonstrated that FK16 is more effective than FK13 against all P. aeruginosa strains and specifically damages the cell membranes as confirmed with the SYTOX-green uptake assay. However, the activity of FK16 was found to be slightly reduced in the presence of physiological salt conditions. A similar observation has been reported with GF17 activity against E. coli (Wang et al., 2017), which suggested that the rate of interaction between the peptide and bacterial membrane may reduce under physiological salt conditions.
Although antibiotics have been shown in this study to kill P. aeruginosa at lower concentrations than LL-37 and its smaller peptides, they are clinically used at very high concentration, i.e., between 0.3 and 1.5% as topical formulations (3 mg/mL; used at > 1500x MIC) for the treatment of Pseudomonas keratitis (Dua et al., 2012; Otri et al., 2013). AMPs, on the other hand, have been shown to exhibit in vivo bactericidal efficacy at low micromolar concentrations (Gordon et al., 2005; Beaumont et al., 2014; Kolar et al., 2015; Pletzer et al., 2017; Wuerth et al., 2019). Given the low antimicrobial efficacy of the small peptides, which is compounded by the tear salt concentration and presence of other proteases, a 4x MIC concentration (800 μM) would be needed for therapeutic effect. This would be too high but in combination with antibiotics, the former can be used in sub-inhibitory concentrations thus minimizing their cytotoxic effects whilst potentiating the effect of the antibiotics to effectively treat ocular surface infections.
The standard MIC assay is useful for ascertaining the antibacterial efficacy of a test compound at the end of a fixed time-point (18 or 21 h). However, it does not provide detailed information on the growth-rate inhibition of bacteria in response to different concentrations of an antimicrobial agent (Abdelbaqi et al., 2016; Ng et al., 2018). Here, we further validated the antimicrobial activity of our peptides and antibiotics utilizing a dynamic pharmacological approach for the generation of dose-response curves. The resultant growth inhibition curves from OD600 measurements have allowed us to report the IC50 readings (concentration at which 50% of the bacterial growth was inhibited) in addition to MIC values for all our test compounds. In our experiments ‘growth inhibition’ could be a reflection of the initial kill of the bacteria by the agents used rather than retardation of growth (of the surviving bacteria) as would be seen by alteration of pH for example. However, the use of term ‘inhibition’ here would be in the same context as used in MIC assay where the ‘growth inhibition’ is a reflection of the killing of bacteria.
We have noted that the activity of FK13 but not FK16 was greatly reduced against cytotoxic PA19660 and ocular PA isolate. Although not tested here, it is likely that FK13 may require higher concentration (>200 μg/mL) to achieve similar efficacy as FK16 against P. aeruginosa in the presence of salt. Moreover, the enhanced activity of FK16 could be attributed to the additional hydrophobic residues on the C-terminus (Leu31 and Val32 as per LL-37 sequence). Interestingly, a previous structural study has provided evidence that C-terminus residues of FK16 were shown to form a 310 helix (Li et al., 2006). However, implication of these hydrophobic residues to the functional activity of FK16 against Gram-negative strains of different virulence was not well-understood. Our comparison of FK13 and FK16 activity have revealed that the hydrophobic C-terminus of FK16 is key for stable bactericidal activity against cytotoxic and ocular P. aeruginosa strains. It is anticipated that further SAR studies would validate our findings and may lead to the development of rationally designed analogs of FK16.
The results from our study are in agreement with earlier reports that vancomycin exhibits much weaker activity against P. aeruginosa (Alvarez et al., 2016; Ng et al., 2018; Pletzer et al., 2018). Earlier studies have demonstrated that polymyxins and ciprofloxacin are capable of enhancing the activity of vancomycin against P. aeruginosa (Day et al., 1993; Ng et al., 2018). However, the wider use of antibiotic combinations has been greatly discouraged due to the increased risk of toxicity (Poole, 2011; Dua et al., 2012; Vazirani et al., 2015).
Synergism between antibiotics and AMPs has been demonstrated both in in vitro and in vivo infection model systems (Blazewicz et al., 2018; Pletzer et al., 2018). Synthetic AMPs such as 1008 and DJK-5 were shown to kill P. aeruginosa via disruption of stringent-stress response pathway (Pletzer et al., 2017). It was further demonstrated that the cutaneous abscesses caused by ESKAPE pathogens can be successfully treated with the combination of peptides and conventional antibiotics (Pletzer et al., 2018). A modified FK13 peptide, FK13-a1, was also shown to enhance the activity of chloramphenicol against MDR bacteria both in the presence and absence of salt (Rajasekaran et al., 2017).
Our results have demonstrated that FK16 at sub-MIC levels was capable of enhancing the susceptibility of P. aeruginosa against vancomycin (up to eightfold). Moreover, further analysis in checkerboard assays has shown that FK16 activity in combination with vancomycin is stable in the physiological tear salt conditions. Although MIC-based checkerboard assay has its own limitation, our results from plate-count experiments implicated a possible synergism between FK16 and vancomycin against both invasive and cytotoxic strain of PA. Thus, while further assessment of FK16-vancomycin in in vivo bacterial keratitis model is required, it may be reasonable to infer that the observed synergism/additive effect would likely be effective in the treatment of P. aeruginosa that have already demonstrated multi-drug resistance. The exact mechanism of the enhanced effect of the two agents together is unclear. Though increased permeabilization of the membrane(s) is observed with FK16, it does not directly suggest the mechanism by which the enhanced effect is achieved. Despite meticulous attention to details regarding time of incubation, volume and concentration of inoculum, subtle errors can inadvertently creep in and affect the outcome and consequent FIC index. Thus, though suggestive of synergism, it is not conclusive. To bring this one step closer to the therapeutic realization, we have also shown that FK16 + vancomycin combination is non-toxic to the human corneal epithelial cells and human RBCs. Therefore, our in vitro results have the potential to form the basis for early preclinical studies particularly for the assessment of toxicity and pharmacokinetic properties of combination therapy including compatibility of formulation, stability, and effective administration route.
In summary, FK16 alone or in combination with vancomycin has shown enhanced ability to kill P. aeruginosa of different virulence without eliciting host cell toxicity. These results provide further credence to the overarching concept of developing the next generation of AMP-antibiotic combination therapies as a viable option to counter antibiotic resistance. Future studies to test the preclinical efficacy of FK16-vancomycin synergism against PA in an in vivo model of bacterial keratitis are planned to take this concept further toward clinical realization.
Data Availability Statement
All datasets generated for this study are included in the manuscript/ Supplementary Files.
Ethics Statement
Human blood for hemolysis assay was collected from the healthy subjects with prior consent under the approved ethics (Reference No. 176-1812) from the local Research Ethics Committee of the Faculty of Medicine and Health Sciences, University of Nottingham.
Author Contributions
IM and HD conceived and designed the experiments. IM performed the experiments and prepared the figures. DS, MN, and LM contributed reagents, materials, analysis tools, and proofread and approved the final draft. IM and HD analyzed the data and prepared the draft of manuscript.
Funding
Financial support from the Royal Blind Asylum and the Royal College of Surgeons of Edinburgh (to HD and IM).
Conflict of Interest
HD is consultant to Dompe, Santen, Thea, and Visufarma and holds shares in Glaxosmithkline and NuVision biotherapeutics.
The remaining authors declare that the research was conducted in the absence of any commercial or financial relationships that could be construed as a potential conflict of interest.
Acknowledgments
We would like to acknowledge Dr. Stephen Heeb, School of Life Sciences, University of Nottingham for providing the slant of PAO1-L strain. We would like to mention the support of the Department of Clinical Microbiology especially for providing the ocular P. aeruginosa isolate (PA-OS). Finally, we would also like to thank Mr. Nagi Marsit, Ph.D. student, Academic Ophthalmology for preparing the microbiological culture stocks at the start of this study.
Supplementary Material
The Supplementary Material for this article can be found online at: https://www.frontiersin.org/articles/10.3389/fmicb.2019.02190/full#supplementary-material
References
Abdelbaqi, S., Deslouches, B., Steckbeck, J., Montelaro, R., and Reed, D. S. (2016). Novel engineered cationic antimicrobial peptides display broad-spectrum activity against Francisella tularensis, Yersinia pestis and Burkholderia pseudomallei. J. Med. Microbiol. 65, 188–194. doi: 10.1099/jmm.0.000209
Alexandrakis, G., Alfonso, E. C., and Miller, D. (2000). Shifting trends in bacterial keratitis in south Florida and emerging resistance to fluoroquinolones. Ophthalmology 107, 1497–1502. doi: 10.1016/s0161-6420(00)00179-2
Alvarez, R., Lopez Cortes, L. E., Molina, J., Cisneros, J. M., and Pachon, J. (2016). Optimizing the clinical use of vancomycin. Antimicrob. Agents Chemother. 60, 2601–2609. doi: 10.1128/AAC.03147-14
Asbell, P. A., Pandit, R. T., and Sanfilippo, C. M. (2018). Antibiotic resistance rates by geographic region among ocular pathogens collected during the ARMOR surveillance study. Ophthalmol. Ther. 7, 417–429. doi: 10.1007/s40123-018-0141-y
Austin, A., Lietman, T., and Rose-Nussbaumer, J. (2017). Update on the management of infectious keratitis. Ophthalmology 124, 1678–1689. doi: 10.1016/j.ophtha.2017.05.012
Beaumont, P. E., McHugh, B., Gwyer Findlay, E., Mackellar, A., Mackenzie, K. J., Gallo, R. L., et al. (2014). Cathelicidin host defence peptide augments clearance of pulmonary Pseudomonas aeruginosa infection by its influence on neutrophil function in vivo. PLoS One 9:e99029. doi: 10.1371/journal.pone.0099029
Blazewicz, I., Jaskiewicz, M., Piechowicz, L., Neubauer, D., Nowicki, R. J., Kamysz, W., et al. (2018). Activity of antimicrobial peptides and conventional antibiotics against superantigen positive Staphylococcus aureus isolated from patients with atopic dermatitis. Postepy Dermatol. Alergol. 35, 74–82. doi: 10.5114/ada.2018.62141
Bloom, D. E., Black, S., Salisbury, D., and Rappuoli, R. (2018). Antimicrobial resistance and the role of vaccines. Proc. Natl. Acad. Sci. U.S.A. 115, 12868–12871. doi: 10.1073/pnas.1717157115
Borkar, D. S., Acharya, N. R., Leong, C., Lalitha, P., Srinivasan, M., Oldenburg, C. E., et al. (2014). Cytotoxic clinical isolates of Pseudomonas aeruginosa identified during the steroids for corneal ulcers trial show elevated resistance to fluoroquinolones. BMC Ophthalmol. 14:54. doi: 10.1186/1471-2415-14-54
Borkar, D. S., Fleiszig, S. M., Leong, C., Lalitha, P., Srinivasan, M., Ghanekar, A. A., et al. (2013). Association between cytotoxic and invasive Pseudomonas aeruginosa and clinical outcomes in bacterial keratitis. JAMA Ophthalmol. 131, 147–153. doi: 10.1001/jamaophthalmol.2013.778
Ceccarelli, G., Oliva, A., d’Ettorre, G., D’Abramo, A., Caresta, E., Barbara, C. S., et al. (2015). The role of vancomycin in addition with colistin and meropenem against colistin-sensitive multidrug resistant Acinetobacter baumannii causing severe infections in a paediatric intensive care unit. BMC Infect. Dis. 15:393. doi: 10.1186/s12879-015-1133-3
Chojnacki, M., Philbrick, A., Wucher, B., Reed, J. N., Tomaras, A., Dunman, P. M., et al. (2019). Development of a broad-spectrum antimicrobial combination for the treatment of Staphylococcus aureus and Pseudomonas aeruginosa corneal infections. Antimicrob. Agents Chemother. 63:e1929-18. doi: 10.1128/AAC.01929-18
Day, C. A., Marceau-Day, M. L., and Day, D. F. (1993). Increased susceptibility of Pseudomonas aeruginosa to ciprofloxacin in the presence of vancomycin. Antimicrob. Agents Chemother. 37, 2506–2508. doi: 10.1128/aac.37.11.2506
Dua, H. S., Otri, A. M., Said, D. G., and Faraj, L. A. (2012). The ’up-down’ sign of acute ocular surface drug toxicity. Br. J. Ophthalmol. 96, 1439–1440. doi: 10.1136/bjophthalmol-2012-301978
Gordon, Y. J., Romanowski, E. G., and McDermott, A. M. (2005). A review of antimicrobial peptides and their therapeutic potential as anti-infective drugs. Curr. Eye Res. 30, 505–515. doi: 10.1080/02713680590968637
Haney, E. F., Straus, S. K., and Hancock, R. E. W. (2019). Reassessing the host defense peptide landscape. Front. Chem. 7:43. doi: 10.3389/fchem.2019.00043
Hazlett, L. D. (2007). Bacterial infections of the cornea (Pseudomonas aeruginosa). Chem. Immunol. Allergy 92, 185–194. doi: 10.1159/000099269
He, M., Zhang, H., Li, Y., Wang, G., Tang, B., Zhao, J., et al. (2018). Cathelicidin-derived antimicrobial peptides inhibit zika virus through direct inactivation and interferon pathway. Front. Immunol. 9:722. doi: 10.3389/fimmu.2018.00722
Hollmann, A., Martinez, M., Maturana, P., Semorile, L. C., and Maffia, P. C. (2018). Antimicrobial peptides: interaction with model and biological membranes and synergism with chemical antibiotics. Front. Chem. 6:204. doi: 10.3389/fchem.2018.00204
Huang, L. C., Petkova, T. D., Reins, R. Y., Proske, R. J., and McDermott, A. M. (2006). Multifunctional roles of human cathelicidin (LL-37) at the ocular surface. Invest Ophthalmol. Vis. Sci. 47, 2369–2380. doi: 10.1167/iovs.05-1649
Huang, L. C., Reins, R. Y., Gallo, R. L., and McDermott, A. M. (2007). Cathelicidin-deficient (Cnlp -/-) mice show increased susceptibility to Pseudomonas aeruginosa keratitis. Invest. Ophthalmol. Vis. Sci. 48, 4498–4508. doi: 10.1167/iovs.07-0274
Jaskiewicz, M., Neubauer, D., Kazor, K., Bartoszewska, S., and Kamysz, W. (2018). Antimicrobial activity of selected antimicrobial peptides against planktonic culture and biofilm of Acinetobacter baumannii. Probiotics Antimicrob. Proteins 11, 317–324. doi: 10.1007/s12602-018-9444-5
Kiattiburut, W., Zhi, R., Lee, S. G., Foo, A. C., Hickling, D. R., Keillor, J. W., et al. (2018). Antimicrobial peptide LL-37 and its truncated forms, GI-20 and GF-17, exert spermicidal effects and microbicidal activity against Neisseria gonorrhoeae. Hum. Reprod. 33, 2175–2183. doi: 10.1093/humrep/dey315
Kolar, S. S. N., Luca, V., Baidouri, H., Mannino, G., McDermott, A. M., and Mangoni, M. L. (2015). Esculentin-1a(1-21)NH2: a frog skin-derived peptide for microbial keratitis. Cell Mol. Life Sci. 72, 617–627. doi: 10.1007/s00018-014-1694-0
Laxminarayan, R., Duse, A., Wattal, C., Zaidi, A. K., Wertheim, H. F., Sumpradit, N., et al. (2013). Antibiotic resistance-the need for global solutions. Lancet Infect. Dis. 13, 1057–1098. doi: 10.1016/S1473-3099(13)70318-9
Li, X., Li, Y., Han, H., Miller, D. W., and Wang, G. (2006). Solution structures of human LL-37 fragments and NMR-based identification of a minimal membrane-targeting antimicrobial and anticancer region. J. Am. Chem. Soc. 128, 5776–5785. doi: 10.1021/ja0584875
Luo, Y., McLean, D. T., Linden, G. J., McAuley, D. F., McMullan, R., and Lundy, F. T. (2017). The naturally occurring host defense peptide, LL-37, and its truncated mimetics KE-18 and KR-12 have selected biocidal and antibiofilm activities against Candida albicans, Staphylococcus aureus, and Escherichia coli in vitro. Front. Microbiol. 8:544. doi: 10.3389/fmicb.2017.00544
Manyi-Loh, C., Mamphweli, S., Meyer, E., and Okoh, A. (2018). Antibiotic use in agriculture and its consequential resistance in environmental sources: potential public health implications. Molecules 23:E795. doi: 10.3390/molecules23040795
McIntosh, R. S., Cade, J. E., Al-Abed, M., Shanmuganathan, V., Gupta, R., Bhan, A., et al. (2005). The spectrum of antimicrobial peptide expression at the ocular surface. Invest. Ophthalmol. Vis. Sci. 46, 1379–1385. doi: 10.1167/iovs.04-0607
Mishra, B., Epand, R. F., Epand, R. M., and Wang, G. (2013). Structural location determines functional roles of the basic amino acids of KR-12, the smallest antimicrobial peptide from human cathelicidin LL-37. RSC Adv∗ 3, doi: 10.1039/C3RA42599A
Mishra, B., and Wang, G. (2017). Titanium surfaces immobilized with the major antimicrobial fragment FK-16 of human cathelicidin LL-37 are potent against multiple antibiotic-resistant bacteria. Biofouling 33, 544–555. doi: 10.1080/08927014.2017.1332186
Mohammed, I., Said, D. G., and Dua, H. S. (2017). Human antimicrobial peptides in ocular surface defense. Prog. Retin. Eye Res. 61, 1–22. doi: 10.1016/j.preteyeres.2017.03.004
Mookherjee, N., and Hancock, R. E. (2007). Cationic host defence peptides: innate immune regulatory peptides as a novel approach for treating infections. Cell Mol. Life Sci. 64, 922–933. doi: 10.1007/s00018-007-6475-6
Morita, Y., Tomida, J., and Kawamura, Y. (2014). Responses of Pseudomonas aeruginosa to antimicrobials. Front. Microbiol. 4:422. doi: 10.3389/fmicb.2013.00422
Ng, V., Kuehne, S. A., and Chan, W. C. (2018). Rational design and synthesis of modified teixobactin analogues: in vitro antibacterial activity against Staphylococcus aureus, Propionibacterium acnes and Pseudomonas aeruginosa. Chemistry 24, 9136–9147. doi: 10.1002/chem.201801423
O’Driscoll, N. H., Cushnie, T. P. T., Matthews, K. H., and Lamb, A. J. (2018). Colistin causes profound morphological alteration but minimal cytoplasmic membrane perforation in populations of Escherichia coli and Pseudomonas aeruginosa. Arch. Microbiol. 200, 793–802. doi: 10.1007/s00203-018-1485-3
O’Neill, J. (2016). Tackling Drug-Resistant Infections Globally: Final Report and Recommendations. The Review on Antimicrobial Resistance. Available at: https://amr-review.org/sites/default/files/160518_Final%20paper_with%20cover.pdf (accessed January 24, 2017).
Otri, A. M., Fares, U., Al-Aqaba, M. A., Miri, A., Faraj, L. A., Said, D. G., et al. (2013). Profile of sight-threatening infectious keratitis: a prospective study. Acta Ophthalmol. 91, 643–651. doi: 10.1111/j.1755-3768.2012.02489.x
Pletzer, D., Mansour, S. C., and Hancock, R. E. W. (2018). Synergy between conventional antibiotics and anti-biofilm peptides in a murine, sub-cutaneous abscess model caused by recalcitrant ESKAPE pathogens. PLoS Pathog. 14:e1007084. doi: 10.1371/journal.ppat.1007084
Pletzer, D., Wolfmeier, H., Bains, M., and Hancock, R. E. W. (2017). Synthetic peptides to target stringent response-controlled virulence in a Pseudomonas aeruginosa murine cutaneous infection model. Front. Microbiol. 8:1867. doi: 10.3389/fmicb.2017.01867
Poirel, L., Jayol, A., and Nordmann, P. (2017). Polymyxins: antibacterial activity, susceptibility testing, and resistance mechanisms encoded by plasmids or chromosomes. Clin. Microbiol. Rev. 30, 557–596. doi: 10.1128/CMR.00064-16
Poole, K. (2011). Pseudomonas aeruginosa: resistance to the max. Front. Microbiol. 2:65. doi: 10.3389/fmicb.2011.00065
Rajasekaran, G., Kim, E. Y., and Shin, S. Y. (2017). LL-37-derived membrane-active FK-13 analogs possessing cell selectivity, anti-biofilm activity and synergy with chloramphenicol and anti-inflammatory activity. Biochim. Biophys. Acta Biomembr. 1859, 722–733. doi: 10.1016/j.bbamem.2017.01.037
Ren, S. X., Shen, J., Cheng, A. S., Lu, L., Chan, R. L., Li, Z. J., et al. (2013). FK-16 derived from the anticancer peptide LL-37 induces caspase-independent apoptosis and autophagic cell death in colon cancer cells. PLoS One 8:e63641. doi: 10.1371/journal.pone.0063641
Rosenfeld, Y., Papo, N., and Shai, Y. (2006). Endotoxin (lipopolysaccharide) neutralization by innate immunity host-defense peptides. Peptide properties and plausible modes of action. J. Biol. Chem. 281, 1636–1643. doi: 10.1074/jbc.M504327200
Rush, S. W., and Rush, R. B. (2016). Outcomes of infectious versus sterile perforated corneal ulcers after therapeutic penetrating keratoplasty in the United States. J. Ophthalmol. 2016:6284595. doi: 10.1155/2016/6284595
Schina, M., Spyridi, E., Daoudakis, M., Mertzanos, E., and Korfias, S. (2006). Successful treatment of multidrug-resistant Pseudomonas aeruginosa meningitis with intravenous and intrathecal colistin. Int. J. Infect. Dis. 10, 178–179. doi: 10.1016/j.ijid.2005.03.005
Simonsen, G. S., Tapsall, J. W., Allegranzi, B., Talbot, E. A., and Lazzari, S. (2004). The antimicrobial resistance containment and surveillance approach–a public health tool. Bull. World Health Organ. 82, 928–934.
Tuli, S., and Gray, M. (2016). Surgical management of corneal infections. Curr. Opin. Ophthalmol. 27, 340–347. doi: 10.1097/ICU.0000000000000274
Vazirani, J., Wurity, S., and Ali, M. H. (2015). Multidrug-resistant Pseudomonas aeruginosa keratitis: risk factors, clinical characteristics, and outcomes. Ophthalmology 122, 2110–2114. doi: 10.1016/j.ophtha.2015.06.007
Wang, G., Epand, R. F., Mishra, B., Lushnikova, T., Thomas, V. C., Bayles, K. W., et al. (2012). Decoding the functional roles of cationic side chains of the major antimicrobial region of human cathelicidin LL-37. Antimicrob. Agents Chemother. 56, 845–856. doi: 10.1128/AAC.05637-11
Wang, G., Mishra, B., Epand, R. F., and Epand, R. M. (2014). High-quality 3D structures shine light on antibacterial, anti-biofilm and antiviral activities of human cathelicidin LL-37 and its fragments. Biochim. Biophys. Acta 1838, 2160–2172. doi: 10.1016/j.bbamem.2014.01.016
Wang, X., Junior, J. C. B., Mishra, B., Lushnikova, T., Epand, R. M., and Wang, G. (2017). Arginine-lysine positional swap of the LL-37 peptides reveals evolutional advantages of the native sequence and leads to bacterial probes. Biochim. Biophys. Acta Biomembr. 1859, 1350–1361. doi: 10.1016/j.bbamem.2017.04.018
Wiegand, I., Hilpert, K., and Hancock, R. E. (2008). Agar and broth dilution methods to determine the minimal inhibitory concentration (MIC) of antimicrobial substances. Nat. Protoc. 3, 163–175. doi: 10.1038/nprot.2007.521
Willcox, M. D. (2011). Review of resistance of ocular isolates of Pseudomonas aeruginosa and staphylococci from keratitis to ciprofloxacin, gentamicin and cephalosporins. Clin. Exp. Optom. 94, 161–168. doi: 10.1111/j.1444-0938.2010.00536.x
Wuerth, K., Lee, A. H. Y., Falsafi, R., Gill, E. E., and Hancock, R. E. W. (2019). Characterization of host responses during Pseudomonas aeruginosa acute infection in the lungs and blood and after treatment with the synthetic immunomodulatory peptide IDR-1002. Infect. Immun. 87:e661-18. doi: 10.1128/IAI.00661-18
Yarlagadda, V., Manjunath, G. B., Sarkar, P., Akkapeddi, P., Paramanandham, K., Shome, B. R., et al. (2016a). Glycopeptide antibiotic to overcome the intrinsic resistance of gram-negative bacteria. ACS Infect. Dis. 2, 132–139. doi: 10.1021/acsinfecdis.5b00114
Keywords: Pseudomonas aeruginosa, antimicrobial peptides, vancomycin, LL-37, FK13, FK16, antibiotic resistance, bacterial keratitis
Citation: Mohammed I, Said DG, Nubile M, Mastropasqua L and Dua HS (2019) Cathelicidin-Derived Synthetic Peptide Improves Therapeutic Potential of Vancomycin Against Pseudomonas aeruginosa. Front. Microbiol. 10:2190. doi: 10.3389/fmicb.2019.02190
Received: 31 March 2019; Accepted: 06 September 2019;
Published: 19 September 2019.
Edited by:
Marina Rautenbach, Stellenbosch University, South AfricaReviewed by:
Robert Bucki, Medical University of Bialystok, PolandMaria Luisa Mangoni, Sapienza University of Rome, Italy
Copyright © 2019 Mohammed, Said, Nubile, Mastropasqua and Dua. This is an open-access article distributed under the terms of the Creative Commons Attribution License (CC BY). The use, distribution or reproduction in other forums is permitted, provided the original author(s) and the copyright owner(s) are credited and that the original publication in this journal is cited, in accordance with accepted academic practice. No use, distribution or reproduction is permitted which does not comply with these terms.
*Correspondence: Harminder S. Dua, Harminder.Dua@nottingham.ac.uk; profdua@gmail.com