- 1Department of Pharmacology, Shanghai Tenth People’s Hospital, Tongji University School of Medicine, Shanghai, China
- 2Department of Pharmacology and Chemical Biology, Institute of Medical Sciences, Shanghai Jiao Tong University School of Medicine, Shanghai, China
- 3New Drug Research and Development Center, School of Pharmacy, Second Military Medical University, Shanghai, China
- 4Department of Neurosurgery, Shanghai Tenth People’s Hospital, Tongji University School of Medicine, Shanghai, China
Candida albicans is the most common opportunistic fungal pathogen which can cause life-threatening bloodstream infections known as candidaemia. It is very important to discover new drugs and targets for the treatment of candidaemia. In this study, we first investigated the combination antifungal effects of the small molecule ENOblock and fluconazole (FLC) against FLC-resistant C. albicans. A checkerboard microdilution assay showed that ENOblock has a significant synergistic effect in combination with FLC against FLC-resistant C. albicans. The time-kill curve further confirmed the synergism of this compound with FLC against FLC-resistant C. albicans. Moreover, we demonstrated the significant inhibitory effects of ENOblock alone and in combination with FLC against C. albicans hypha and biofilm formation. Furthermore, the XTT assay showed that ENOblock has relatively low toxicity to human umbilical vein endothelial cells. The in vivo antifungal efficacy of ENOblock was further assessed in a murine model of systemic C. albicans infection. Although ENOblock alone was not sufficient to treat C. albicans infection, the combination of FLC and ENOblock showed significant in vivo activity against FLC-resistant C. albicans. Finally, using surface plasmon resonance analysis as well as an inhibition assay, we determined that ENOblock directly interacted with CaEno1 and significantly inhibited the transglutaminase activity of this enzyme, which is involved in the growth and morphogenesis of C. albicans. In summary, these results demonstrate the synergistic effects of FLC and ENOblock against FLC-resistant C. albicans, and indicate that inhibition of the transglutaminase activity of CaEno1 by ENOblock might confer an advantage for the synergism of FLC and ENOblock, suggesting the potential of ENOblock as a new antifungal candidate.
Introduction
The treatment of invasive fungal infections with high mortality rates in immunocompromised hosts is notoriously difficult due to drug resistance and the limited number of antifungal agents available (Brown et al., 2012; Calderone et al., 2014; Ng et al., 2015). Candida albicans, one of the opportunistic fungal pathogens, which include non-albicans Candida species, Cryptococcus neoformans and Aspergillus fumigatus, is the most common cause of disseminated systemic candidiasis, especially in immunocompromised individuals, with mortality rates more than 40% (Pfaller and Diekema, 2007; Gow and Yadav, 2017; Robbins et al., 2017). Currently, the major classes of antifungals used in clinic are polyenes, azoles and echinocandins. However, the broad and irrational utilization of azoles, especially fluconazole (FLC), has led to the emergence azoles-resistant clinical isolates (White et al., 1998, 2002; Kohli et al., 2002). The treatment of C. albicans infection has been challenging due to the substantial host toxicities from current antifungal agents and the emergence of drug resistance to standard therapies (Perfect, 2016; Robbins et al., 2017). Moreover, C. albicans biofilms readily forming on medical devices show high levels of resistance to most of the antifungal agents and play a contributing role in the process of C. albicans infections (Ramage et al., 2009; Tobudic et al., 2010; Sarkar et al., 2014; Rajendran et al., 2015; Gow and Yadav, 2017). Antifungal resistance is a serious threat to human health, and there is an urgent need for the development of novel antifungals.
As a homolog of human enolase, enolase 1 (Eno1) encoded by the only one ENO1 gene in C. albicans is a multifunctional protein that is essential for the growth and virulence of C. albicans (Yang et al., 2006; Ko et al., 2013). In particular, the heterozygosity or homozygosity of the ENO1 gene may affect the growth of C. albicans under some conditions, decrease virulence in the host and increase the susceptibility to various drugs, such as FLC (Yang et al., 2006; Ko et al., 2013; Vyas et al., 2018).
CaEno1 is likely to be a good antifungal target. Transglutaminases (TGases) are widely distributed in plants, fungi and animals and are known to catalyze the formation of N-ε-(γ-glutamyl)-lysine amide bonds, resulting in covalent cross-links between proteins (Ruiz-Herrera et al., 1995). As previously reported in cell-free extracts of Saccharomyces cerevisiae and C. albicans, TGase activity is closely associated with cell wall assembly, and inhibition of the activity of this enzyme decreased the incorporation of proteins into the cell wall (Ruiz-Herrera et al., 1995; Iranzo et al., 2002). Recently, the multifunctional protein CaEno1 in the cell wall was identified as a TGase that is involved in cell growth, cell division, and morphogenesis (Reyna-Beltran et al., 2018). Moreover, as one virulence determinant of C. albicans, Hwp1 has previously been identified as a substrate for mammalian TGases (Staab et al., 1999), indicating the virtual interaction between Hwp1 and CaEno1. All of the above findings indicate that inhibition of multiple functions of CaEno1 may emerge as a promising strategy for enhancement of the efficacy of antifungals.
As the first reported enolase inhibitor, ENOblock is a non-substrate analog that directly binds human enolase to affect the non-glycolytic functions of this enzyme and has been revealed to possess some bioactivities, such as anti-tumor and anti-diabetic activities (Jung et al., 2013; Cho et al., 2017). However, the antimicrobial activity of ENOblock, such as antifungal activity, has not been reported to date. Thus, it would be interesting to speculate that ENOblock could exhibit significant activity against common human fungal pathogens such as Candida species and C. neoformans, and to further assess the interaction of ENOblock with potential fungal targets and the potential antifungal efficacy of ENOblock.
In this study, we first explored the in vitro and in vivo antifungal efficacy of small molecule ENOblock, and measured the cytotoxicity of this compound to evaluate its potential as an antifungal agent. We further assessed the interaction of ENOblock with CaEno1 and the ability to inhibit CaEno1 enzymatic activity, which might contribute to the synergism of FLC and ENOblock. To test the antifungal activity, we mainly investigated the synergistic effects of ENOblock and FLC against clinical C. albicans isolates, and used a murine model of systemic C. albicans infection to test the potential therapeutic efficacy of ENOblock in combination with FLC.
Materials and Methods
Strains and Agents
Thirty clinical isolates of FLC-resistant C. albicans including C. albicans strain 0304103, C. albicans reference strain SC5314, C. neoformans 56992, C. tropicalis ATCC20026, C. parapsilosis ATCC 22010, C. krusei ATCC2340, and C. glabrata ATCC1182, were used in this study. All of the clinical isolates including C. albicans strain 0304103 were provided by Changhai Hospital of Shanghai, the People’s Republic of China, and were widely used in our lab (Quan et al., 2006; Li et al., 2015a, b, 2017). C. albicans reference strain SC5314 can form normal biofilms and is susceptible to FLC (Ramage et al., 2001; Paulone et al., 2017). All of the various strains were grown in YPD (1% yeast extract, 2% peptone, and 2% dextrose) liquid medium overnight in a shaking incubator at 30°C. Human umbilical vein endothelial cells (HUVECs) were purchased from ATCC (Manassas, VA, United States) and incubated in Dulbecco modified Eagle medium (DMEM) containing 10% fetal bovine serum (FBS), and then were used to evaluate the toxicity of ENOblock (Beedie et al., 2016; Ma et al., 2016; Zhong et al., 2017).
Fluconazole (Sigma), and ENOblock hydrochloride (Selleck) were dissolved in dimethyl sulfoxide (DMSO), and the initial stock concentration was 6.4 mg/ml for susceptibility testing. For all experiments, DMSO comprised less than 1% of the total test volume to avoid affecting outcomes of experiments.
Antifungal Susceptibility Testing
Antifungal susceptibility testing was performed according to the broth microdilution protocol of the Clinical and Laboratory Standards Institute (M27-A3), with a few modifications (Quan et al., 2006; Li et al., 2017; Zhong et al., 2017). Briefly, after 24 h or 72 h of incubation at 35°C, the growth inhibition was measured by the optical densities at 600 nm (OD600), from which the background optical densities were already subtracted. The final concentration of ENOblock and FLC ranged from 0.125 to 64 μg/ml, and then MICs were determined. Synergsim and antagonism were defined by FICIs of ≤ 0.5 and > 4, respectively. An FICI value of > 0.5 but ≤ 4 was considered indifferent (Odds, 2003).
Time-Kill Test
Candida albicans 0304103 was grown in RPMI 1640 medium with a starting inoculum of 105 CFU/ml. Different concentrations of ENOblock (8 μg/ml or 16 μg/ml) and FLC (8 μg/ml) were added. DMSO comprised < 1% of the total test volume. At predetermined time points (0, 4, 8, 12, 16, 24, 36, and 48 h) after incubation with agitation at 30°C, a 100-μl aliquot was removed from every solution and serially diluted 10-fold in PBS. A 100-μl aliquot from each dilution was spread on a SDA plate. Colony counts were determined after incubation at 30°C for 48 h. Synergism and antagonism were defined as described previously (Canton et al., 2005; Quan et al., 2006; Pankey et al., 2014). Briefly, Synergism was defined as a ≥ 2-log10 decrease in CFU per milliliter for the combination compared to that by the most active agent alone after 24 h, antagonism was defined as a ≥ 2-log10 increase in CFU per milliliter for the combination compared to that by the most active agent alone after 24 h, while a change of < 2 log10 CFU/ml was considered indifferent.
Biofilm Formation and XTT Reduction Assay
The in vitro biofilm formation assay was carried out according to methods described previously (Ramage et al., 2002; Li et al., 2017) with some modifications. In brief, 100-μl aliquots of C. albicans 0304103 cells or C. albicans SC5314 (1.0 × 106 CFU/ml) in RPMI 1640 medium were introduced into wells of 96-well tissue culture plates and incubated statically at 37°C. After 1 h of adhesion, the medium and non-adherent cells were removed, and the fresh RPMI 1640 medium was added. The plates were further incubated statically for 48 h at 37°C. Biofilm formation was semiquantitatively measured using a 2,3-bis(2-methoxy-4-nitro-5-sulfophenyl)-2H-terazolium-5-carboxanilide (XTT) reduction assay (Ramage et al., 2001). To investigate the effect of FLC in the absence or presence of ENOblock (2 to 16 μg/ml) on the formation of C. albicans biofilms, different concentrations of the agents were added to the fresh medium after 1 h of adhesion. Following incubation at 37°C for 48 h, biofilm cells were washed with PBS and incubated with 0.5 mg/ml XTT and 1 mM menadione in PBS at 37°C for 90 min. The optical density (OD) was measured at 490 nm using a microtiter plate reader. To detect the synergistic effect on mature biofilms, FLC and ENOblock (8 to 64 μg/ml) were added after 24 h of incubation with the mature biofilms at 37°C, and the plates were incubated at 37°C for an additional 24 h.
Toxicity Evaluation of ENOblock
Human umbilical vein endothelial cells (HUVECs) were purchased from ATCC (Manassas, VA, United States) and incubated in DMEM containing 10% FBS (Beedie et al., 2016; Ma et al., 2016; Zhong et al., 2017). The cytotoxic effect of ENOblock on HUVECs viability was assessed by the XTT assay (Scudiero et al., 1988). HUVECs (5 × 103 cells/well) were seeded in 96-well microtiter plates and cultured in DMEM medium (HyClone) supplemented with 10% FBS (HyClone) at 37°C for 24 h in the presence of 5% CO2. After incubation, the cell supernatant was removed and different concentrations of ENOblock (2 to 64 μg/ml) dissolved in fresh media without FBS were added. The plates were incubated for another 24 h. At the end of incubation, 50 μl of PMS-XTT solution (final concentration, 50 μg of XTT, and 0.38 μg of PMS per well) was added to each well and incubated at 37°C for 4 h. The absorbance at 450 nm was measured using an ELISA Plate Reader. Cells incubated in DMEM just with DMSO (<1% of the total test volume) were set as the standard for 100% viability.
The hemolytic activity of ENOblock was assessed using a previously reported method (Radwan et al., 2017; Yang et al., 2019) with some modifications. Briefly, 100 μl of a healthy rabbit red cell suspension in 96-well microtiter plates was mixed with 100 μl of a solution containing different concentrations of ENOblock, and final ENOblock concentrations of 2 to 64 μg/ml were applied. After 1 h of incubation at 37°C, the supernatants were collected, and the absorbance (Abs) at 405 nm was measured using a spectrophotometer. Additionally, red cells incubated with PBS containing DMSO (<1% of the total test volume) served as a negative-control group (to estimate natural hemolysis of PBS with DMSO), and those incubated with distilled water served as a positive control (serving as 100% hemolysis). The percentage of hemolysis induced by ENOblock was then calculated using the equation% hemolysis = (AbsS – Abs0)/(Abs100 – Abs0) × 100%, where AbsS is the average absorbance of the sample, Abs0 is the average absorbance of the negative control, and Abs100 is the average absorbance of the positive control. AMB and FLC were used as controls.
Recombinant Protein Expression and Purification
The full-length C. albicans enolase 1 gene (ENO1, C1_08500C_A/orf19.395, Candida Genome Database) cloned into the pET-21a(+) plasmid with a C-terminal His6 tag was expressed in the Escherichia coli BL21(DE3)pLysS strain. For protein expression, cultures were grown in LB medium containing 0.1 mg/ml ampicillin in a rotary shaker at 37°C to an OD600 nm of 0.6–0.8 before induced using 0.5 mM IPTG at 37°C for 5 h. After harvesting by centrifugation, cells were lysed by sonication in lysis buffer, and then centrifuged at more than 12,000 rpm for 20 min to collect the supernatant containing recombinant protein.
To obtain highly purified protein, the recombinant C. albicans enolase 1 (rCaEno1) protein was purified by Ni-chelate affinity chromatography (Qiagen), followed by size exclusion chromatography using a HiLoad 16/60 Superdex 200 prep grade column (GE Healthcare). Following purity determination by SDS-PAGE, aliquots (less than 100 μl) of purified recombinant proteins were flash-frozen with liquid nitrogen and stored at −80°C.
Protein Activity Determination
The enolase activity of rCaEno1 was determined using the Enolase Activity Assay Kit (Sigma-Aldrich) according to the manufacturer’s instructions with slight modification. Briefly, the mixture with a final volume of 100 μl (50 μl of sample dilutions + 50 μl of the appropriate reaction mix) was mixed well by pipetting and incubated at 25°C. To test the inhibition of enolase activity by ENOblock, rCaEno1 was preincubated with ENOblock or sodium fluoride (NaF) in a total volume of 50 μl at room temperature, and then the experiment was continued as described above. After 30 min, the absorbance at 570 nm was measured as the OD570 nm value. NaF used as a control is a well-known enolase inhibitor.
The transglutaminase activity of rCaEno1 was determined using Transglutaminase Assay Kit (Sigma) according to the manufacturer’s instructions. The kit can also be used to assay the inhibitory activity of ENOblock. Briefly, rCaEno1 and ENOblock were added into the appropriate wells, and the volume was adjusted to 50 μl with ultrapure water. rCaEno1 was preincubated with ENOblock or cystamine at room temperature, and then the experiment was continued according to the manufacturer’s instructions. The absorbance at 450 nm was measured as the OD450 nm value. Cystamine was used as a control is a specific inhibitor of TGase.
Surface Plasmon Resonance (SPR) Analysis
Surface plasmon resonance analysis was performed with a Biacore T200 instrument (GE Healthcare) with CM5 sensor chips (GE Healthcare). Activation, deactivation, preparation of the coupled flow cell and the ligand-binding assay were performed essentially as described previously (Song et al., 2016). Briefly, the rCaEno1 protein was immobilized in parallel-flow channels of on a BIAcoreTM CM5 sensor chip using an amine coupling kit (GE Healthcare). To test the binding of rCaEno1 protein by ENOblock, serial dilutions of ENOblock were injected into the flow system. Experiments were conducted with PBS as the running buffer, and the analyte was injected at a flow rate of 30 μl/min. The association time was 90 s and the dissociation time was 60 s. The affinity constants of binding were obtained using a 1:1 Langmuir binding model via BIAevaluation software. To avoid the non-specific protein binding, bovine serum albumin (BSA) was used as the negative control, and amphotericin B (AMB) which is big and greasy like ENOblock was used as the other control.
Murine Model of Disseminated Candidiasis
After growth in YPD broth for 14 h at 30°C and 200 rpm, C. albicans 0304103 cells or C. albicans SC5314 cells were washed with PBS three times and diluted to the desired concentration (2.5 × 106 cells/ml) determined by counting with a hemacytometer. Female C57BL/6 mice aged 8–10 weeks were infected via the tail vein with 200 μl of a 2.5 × 106 CFU/ml PBS suspension, and then randomly placed into four groups (five mice per group): an untreated control group, FLC alone group, ENOblock alone group and FLC + ENOblock group. At 2 h after infection, 0.25 mg/kg FLC and 12 mg/kg ENOblock were administered intraperitoneally (i.p.). At 48 h following infection, the mice were euthanized and the kidneys were removed aseptically, placed in PBS, and then homogenized via bead beating. Serial dilutions of the homogenized kidneys were inoculated on YPD agar plates to measure the renal fungal burden. CFU values in kidneys were expressed as CFU/g of tissue, then transformed into log10 units and the differences between groups were analyzed by analysis of variance (ANOVA). For the survival assay (10 mice per group), FLC (0.25 mg/kg) and ENOblock (12 mg/kg) were administered i.p. at 2, 24, and 48 h post infection. Mice were given free access to standard laboratory diet and water, and checked daily until day 30. The survival curves were statistically analyzed by the Kaplan-Meier method (log-rank test, GraphPad Prism).
Statistical Criteria
Statistics were calculated in GraphPad Prism 6.0 (GraphPad Software, San Diego, CA, United States), in which P-value of < 0.05 (∗), < 0.01 (∗∗), or < 0.001 (∗∗∗) was considered statistically significant.
Results
In vitro Synergistic Activity of FLC and ENOblock Against FLC-Resistant C. albicans
ENOblock is the first reported non-substrate inhibitor of enolase. Here, we first evaluated the antifungal activity of ENOblock alone or in combination with FLC against two C. albicans isolates (FLC-sensitive C. albicans SC5314 and FLC-resistant C. albicans 0304103) and various other yeast strains, including C. neoformans, Candida krusei, Candida tropicalis, Candida glabratas, and Candida parapsilosis (Table 1). The MICs of ENOblock alone against these tested strains ranged from 8 to 64 μg/ml. When ENOblock was used in combination with FLC, the MICs ranged from 1 to 16 μg/ml. In terms of MICs, the synergistic activity was observed in FLC-resistant C. albicans 0304103, C. krusei, C. glabratas, and C. parapsilosis. However, the combination of FLC and ENOblock did not show synergism against the FLC-susceptible C. albicans SC5314, exhibiting a fractional inhibitory concentration index (FICI) of 1.03 (Table 1).
To further assess the antifungal activity of ENOblock against C. albicans, we tested the effect of ENOblock in combination with FLC against 30 clinical FLC-resistant and 10 FLC-sensitive C. albicans isolates by a checkerboard microdilution assay. The results are summarized in Table 2. The range of MICs of FLC alone against all the FLC-resistant strains was > 64 μg/ml, and the MICs of ENOblock alone ranged from 16 to 32 μg/ml. Compared to FLC alone, the combination of FLC and ENOblock markedly reduced the MICs and showed a significant synergistic effect against all the FLC-resistant strains tested, as the FICI ranged from 0.13 to 0.38 (Table 2). Unlike the results of FLC-resistant isolates, the combination of FLC and ENOblock did not exhibit synergism on FLC-susceptible strains tested, since the FICI was 0.56 to 1.06 (Table 2).
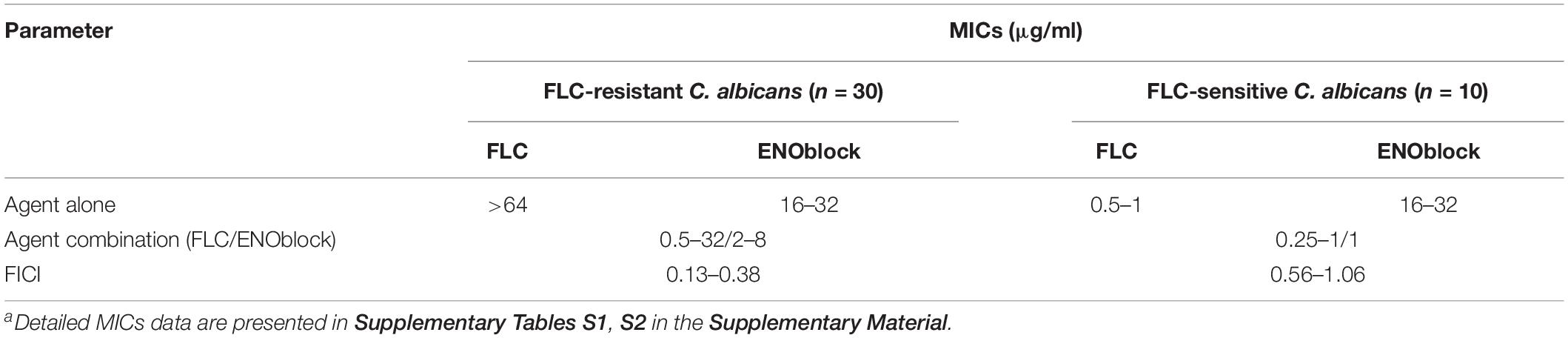
Table 2. Interaction of fluconazole (FLC) and ENOblock against C. albicans as determined by microdilution assaya.
In addition, the time-kill assay also confirmed the synergism of ENOblock and FLC against FLC-resistant C. albicans 0304103 (Figure 1). As shown in Figure 1, compared with the control with a starting inoculum of 105 CFU/ml, ENOblock alone, even at 16 μg/ml, had no impact on the viability of C. albicans 0304103 after 24 h, while FLC (8 μg/ml) alone had a weak effect on its growth. However, the combination of ENOblock (8 μg/ml) and FLC (8 μg/ml) produced a 2.1-log10-CFU/ml decrease compared with the number of CFU produced by FLC (8 μg/ml) alone at 24 h, suggesting the combination of ENOblock and FLC significantly enhanced the antifungal effect compared with FLC (8 μg/ml) alone. Meanwhile, the antifungal effect was further improved as the dose (16 μg/ml) of ENOblock increased when the dose (8 μg/ml) of FLC remained unchanged (Figure 1), indicating that the synergistic activity of ENOblock and FLC was dependent on the dose of ENOblock.
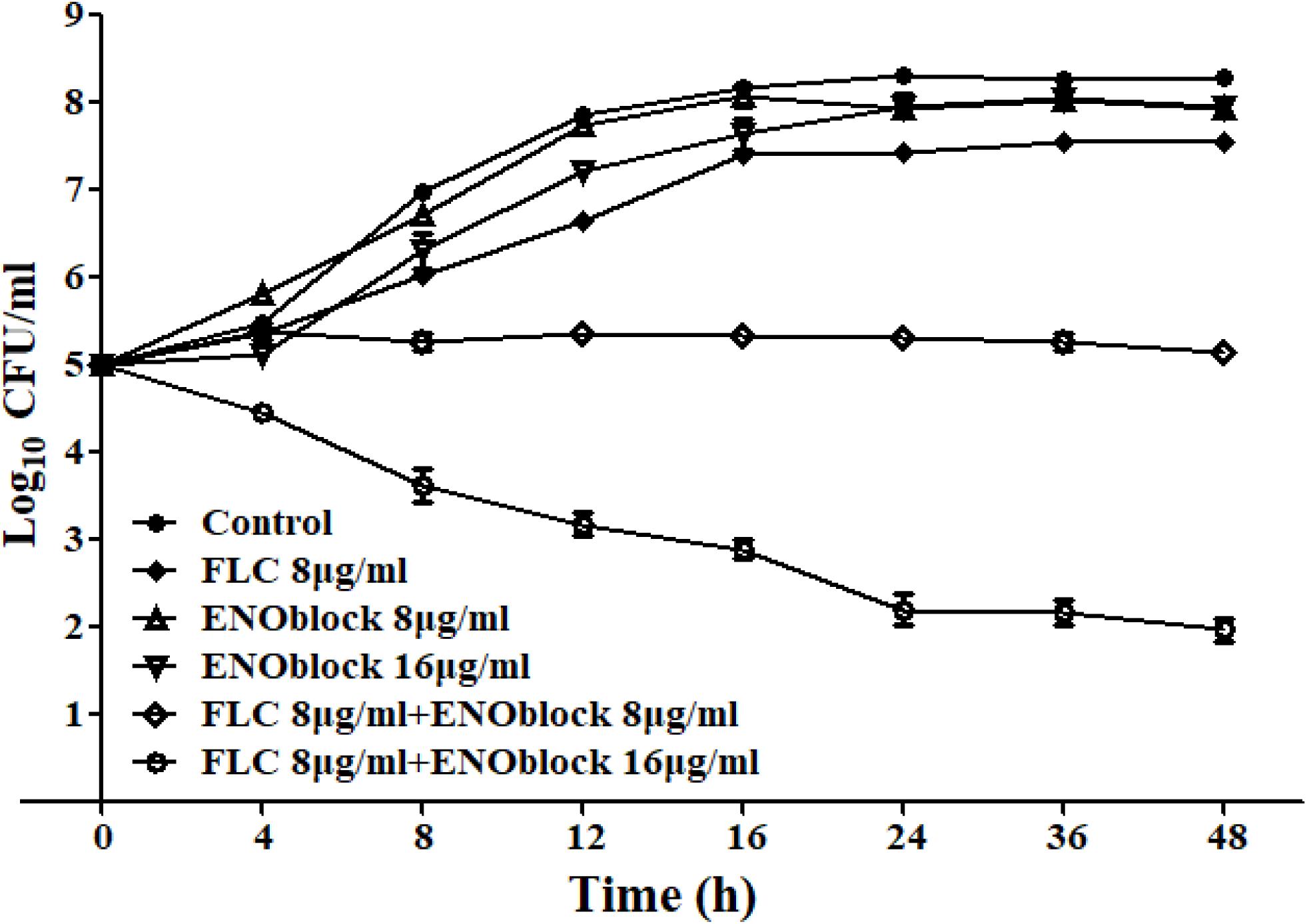
Figure 1. Time-kill curves of Candida albicans 0304103 treated with different concentrations of ENOblock/FLC using an initial inoculum of 105 CFU/ml. Aliquots were obtained at the indicated time points and serially dilutions were spread on agar plates. Colony counts were determined after 48 h of incubation. The data represent the means ± standard deviations for three independent experiments.
Effects of ENOblock Against C. albicans Hypha and Biofilm Formation
To determine the effect of ENOblock alone or in combination with FLC on the yeast-to-hypha morphological transition of C. albicans, C. albicans 0304103 cells were grown in several different media that are known to induce morphological transition, including liquid Lee medium, liquid Spider medium and liquid RPMI 1640 medium. In all the ENOblock-free media with or without FLC (2 to 8 μg/ml), C. albicans 0304103 cells formed true hyphae (Figure 2 and Supplementary Figure S1). Interestingly, 4 μg/ml ENOblock in combination with FLC (2 to 8 μg/ml) inhibited the yeast-to-hypha morphological transition to some extent in liquid Lee or Spider medium, and the inhibitory effect was dependent on the dose of ENOblock. The addition of 8 μg/ml ENOblock obviously disrupted the formation of true hyphae: pseudohyphae and yeast-form cells were observed in liquid Lee or Spider medium without FLC, and hypha formation was completely inhibited in liquid Lee and Spider media by 8 μg/ml ENOblock in combination with FLC (2 to 8 μg/ml) (Figure 2 and Supplementary Figure S1). However, in liquid RPMI 1640 medium with ENOblock (8 μg/ml) plus FLC (2 to 8 μg/ml), the morphological transition began to be inhibited (Supplementary Figure S2). When in combination with FLC (2 to 8 μg/ml), ENOblock at 16 μg/ml substantially damaged the formation of true hyphae, and only yeast-form cells were observed in liquid RPMI 1640 medium when the ENOblock concentration approached 32 μg/ml (Supplementary Figure S2).
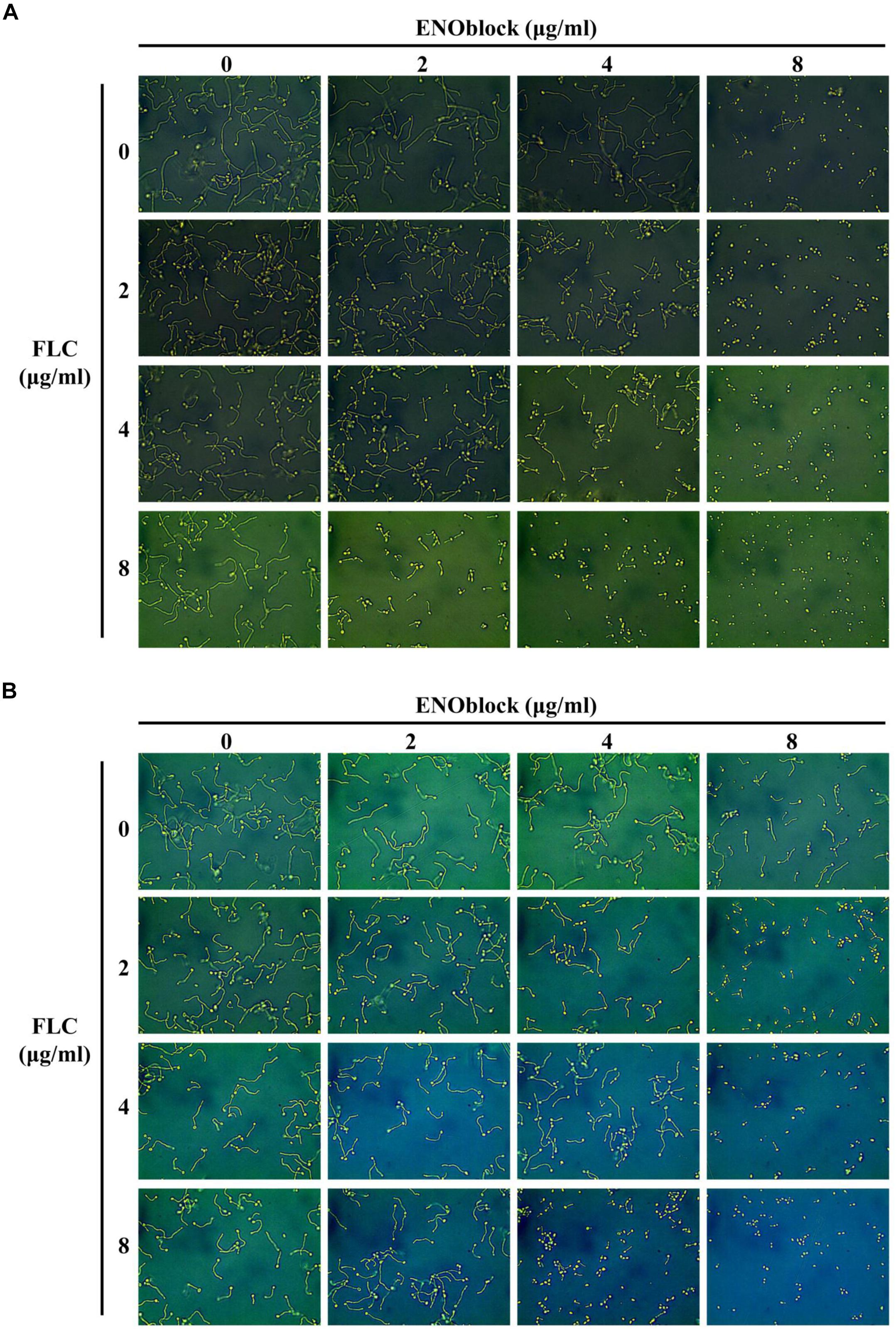
Figure 2. Effects of different concentrations of ENOblock in combination with FLC on hypha formation. Representative photomicrographs of indicated C. albicans 0304103 growing in different media, including liquid Lee medium (A) and liquid Spider medium (B), for 3.5 h at 37°C, as observed with an inverted phase contrast microscope with a 40 × objective.
Furthermore, the in vitro inhibitory activity of ENOblock alone or in combination with FLC against C. albicans biofilm formation was assessed by the XTT reduction assay. As shown in Figure 3A, 8 μg/ml and 16 μg/ml ENOblock inhibited biofilm formation by approximately 45 and 80%, respectively (P < 0.001), while FLC used alone at both 8 and 64 μg/ml did not exhibit antibiofilm activity. Notably, at 4 μg/ml, ENOblock alone had no effect on biofilm formation, but in combination with FLC (8 μg/ml), ENOblock exhibited a significant inhibitory effect with an of approximately 12.5% reduction of biofilm formation (P < 0.05). The inhibitory activity increased when the ENOblock concentration increased. The combination of ENOblock (8 μg/ml or 16 μg/ml) and FLC (8 μg/ml) substantially reduced biofilm formation by approximately 75% or 95%, respectively (P < 0.001). Specifically, the combination of ENOblock and FLC exhibited inhibitory activity against mature biofilms. Neither ENOblock nor FLC alone had a significant inhibitory effect on mature biofilms (Figure 3B), but ENOblock (64 μg/ml) in combination with FLC (8 μg/ml) destroyed mature biofilms by 29% (P < 0.05). Consistently, as shown in Supplementary Figure S3, the significant inhibitory activity of ENOblock against biofilm formation of C. albicans SC5314 was also observed and even more obvious.
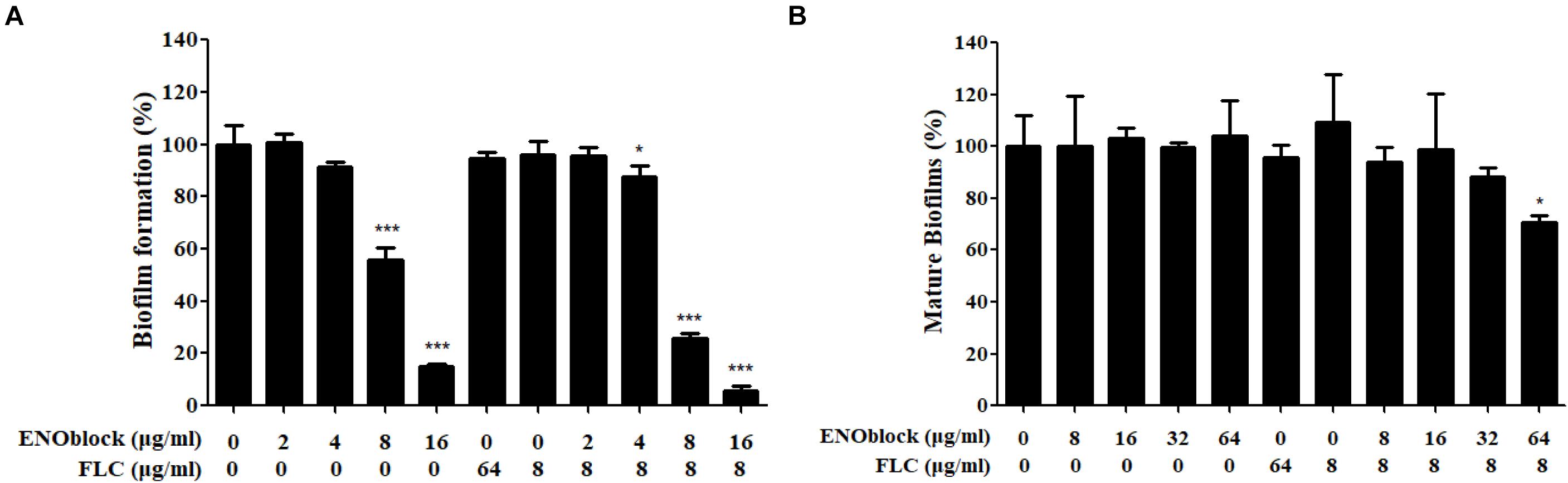
Figure 3. Effects of the combination of ENOblock and FLC on biofilm formation of C. albicans 0304103 in vitro. (A) Effects of different concentrations of ENOblock alone and in combination with 8 μg/ml FLC on C. albicans biofilm formation. (B) Effects of different concentrations of ENOblock alone and in combination with 8 μg/ml FLC on the maintenance of mature biofilms. Biofilm formation was evaluated by the XTT reduction assay by calculating the percentage of viable C. albicans cells relative to the control cells without drug treatment. Data are shown as the means ± standard deviations for three independent experiments. ∗∗P < 0.01; ∗∗∗P < 0.001 compared with the value of the control biofilms.
The in vivo Activity of ENOblock-FLC Combination in a Murine Model of Systemic Candidiasis
To evaluate the efficacy of combination therapy with FLC and ENOblock, we compared the renal fungal burden and survival rate of C. albicans-infected mice using the well-established murine model of systemic candidiasis. Firstly, we checked the in vitro toxicity of ENOblock including cell viability and hemolysis, and found that ENOblock had neither significant toxic effect on HUVECs nor in vitro hemolytic activity at concentrations that show significant antifungal activity (Figure 4). In a murine model of systemic candidiasis with C. albicans 0304103, treatment of mice with ENOblock (12 mg/kg) alone did not show significant reduction in fungal burden when compared to the untreated counterparts. However, the combination of ENOblock and FLC significantly reduced the renal fungal burden and improved the survival relative to FLC alone (∗P < 0.05) (Figure 5). Consistent with this finding, the combination treatment also significantly reduced the renal fungal burden and extended the survival of C. albicans SC5314-infected mice, while neither FLC nor ENOblock was sufficient to treat the C. albicans infection (Supplementary Figure S4), confirming the synergistic in vivo antifungal efficacy of these two agents.
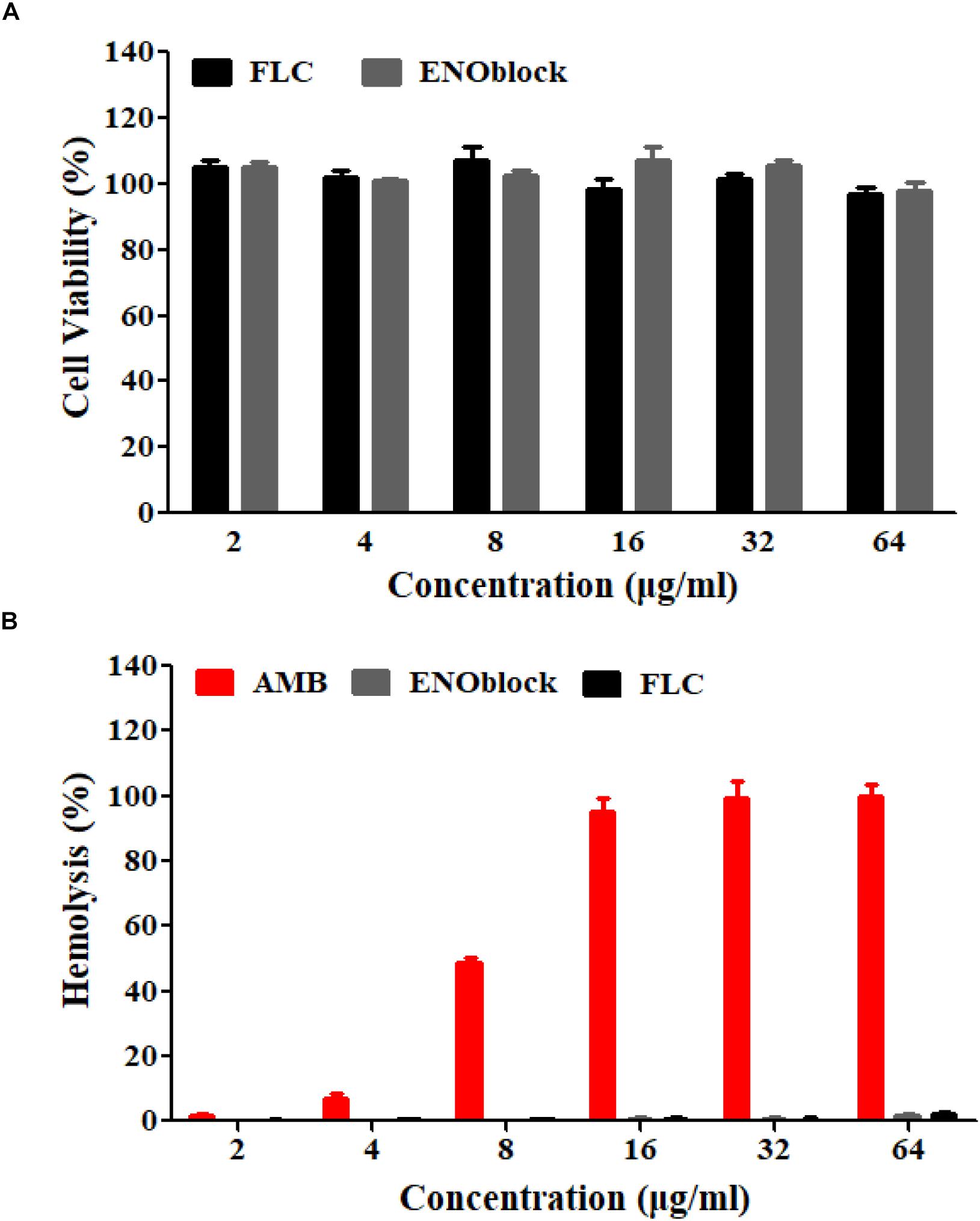
Figure 4. The in vitro toxicity evaluation of ENOblock. (A) The cytotoxic effect of ENOblock, compared to that of FLC, on HUVEC viability was assessed by the XTT test following a 4-h treatment. (B) Hemolysis of rabbit red blood cells following incubation with ENOblock. AMB and FLC were used as controls. The results represent the means ± standard deviations from three independent experiments.
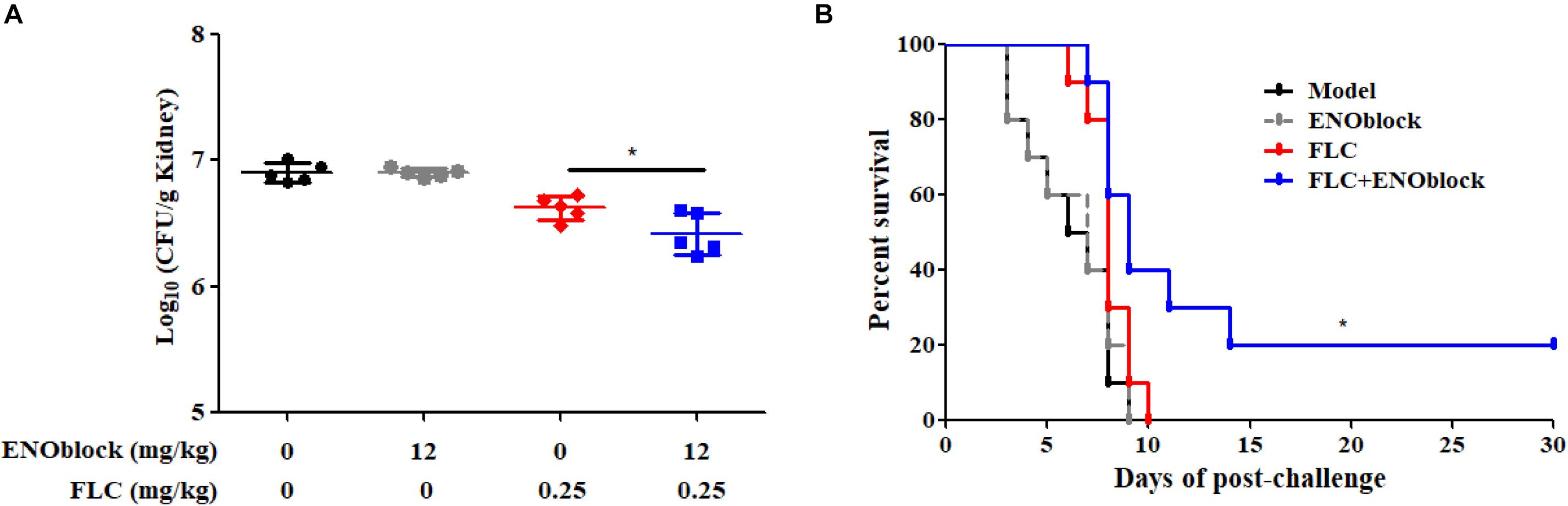
Figure 5. ENOblock enhances the activity of FLC in a murine model of systemic candidiasis. (A) Kidney CFU assay in mice with systemic candidiasis after 2 days. Female C57BL/6 mice were infected with 5 × 105 CFU of C. albicans 0304103. ENOblock and FLC were administered 2 h post infection. ∗P < 0.05 (P-values are from ANOVA). (B) Survival curves of C57BL/6 mice infected with 5 × 105 CFU of C. albicans 0304103. ENOblock and FLC were administered at 2, 24, and 48 h post infection. The log-rank test was used for statistical analysis.
Effects of ENOblock on the Transglutaminase Activity of C. albicans Enolase1
It has been reported that ENOblock binds to purified human enolase and inhibits the activity of the enzyme (Jung et al., 2013). In this study, we cloned the full-length C. albicans enolase 1 gene (ENO1, C1_08500C_A/orf19.395, Candida Genome Database) into the pET-21a(+) vector with a C-terminal His6 tag and purified recombinant C. albicans enolase 1 (rCaEno1) produced in E. coli BL21(DE3)pLysS using a Ni2+-NTA-agarose column. The rCaEno1 protein was identified by Western blotting with anti-His-tag antibodies and mouse anti-rCaEno1 antibodies produced with purified rCaEno1 (Supplementary Figure S5).
Furthermore, we demonstrated the enolase activity and transglutaminase (TGase) activity of rCaEno1 using enzyme assay kits (Figures 6A,B), and investigated the binding of ENOblock to rCaEno1 using surface plasmon resonance (SPR), showing the potent interaction of them with a KD of approximately 0.3 μM (Figure 7). To avoid the problem that this is just not non-specific protein binding, we also performed the SPR analysis using BSA and AMB as controls. The results indicated that while ENOblock showed strong interaction with rCaEno1p, it had no obvious binding with BSA. However, AMB showed weak binding with both rCaEno1p and BSA, suggesting the non-specific protein binding between AMB and rCaEno1p (Supplementary Figure S6).
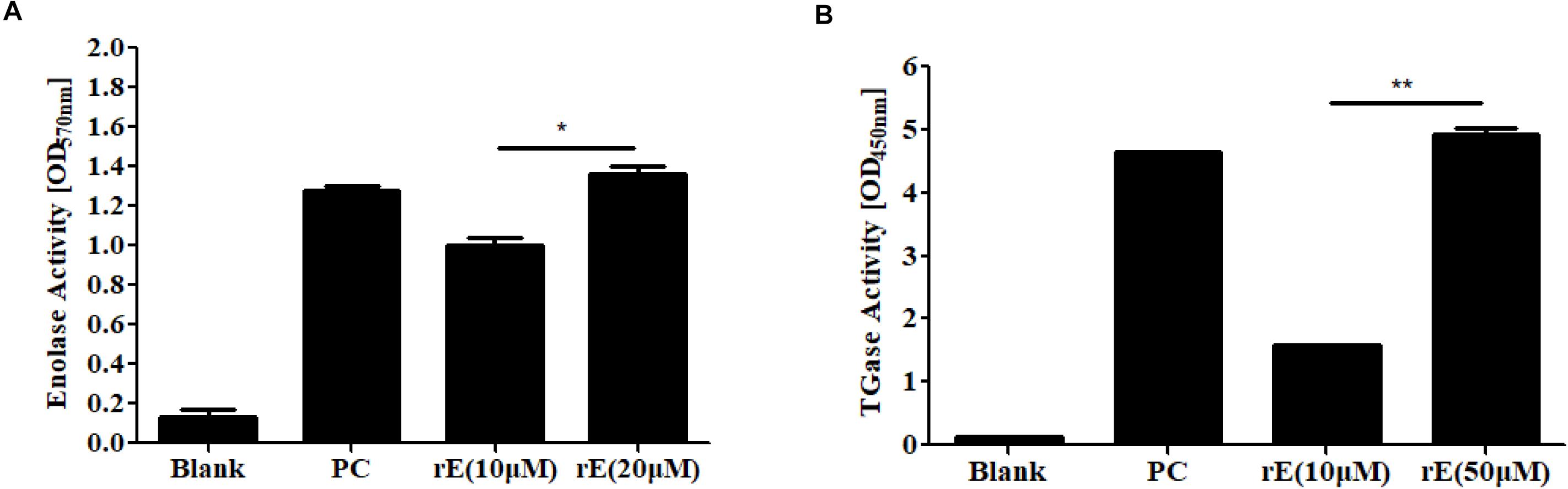
Figure 6. Enzyme activity of recombinant C. albicans enolase 1 (rCaEno1). (A) Enolase activity was determined with purified rCaEno1 protein. rE, rCaEno1; PC, enolase-positive control (catalog number MAK178F). (B) TGase activity was determined with purified rCaEno1 protein. rE, rCaEno1; PC, positive control (transglutaminase from guinea pig liver, catalog number T5398). ∗P < 0.05; ∗∗P < 0.01 (statistical two-tailed unpaired t-test). Data are shown as the means ± standard deviations for three independent experiments.
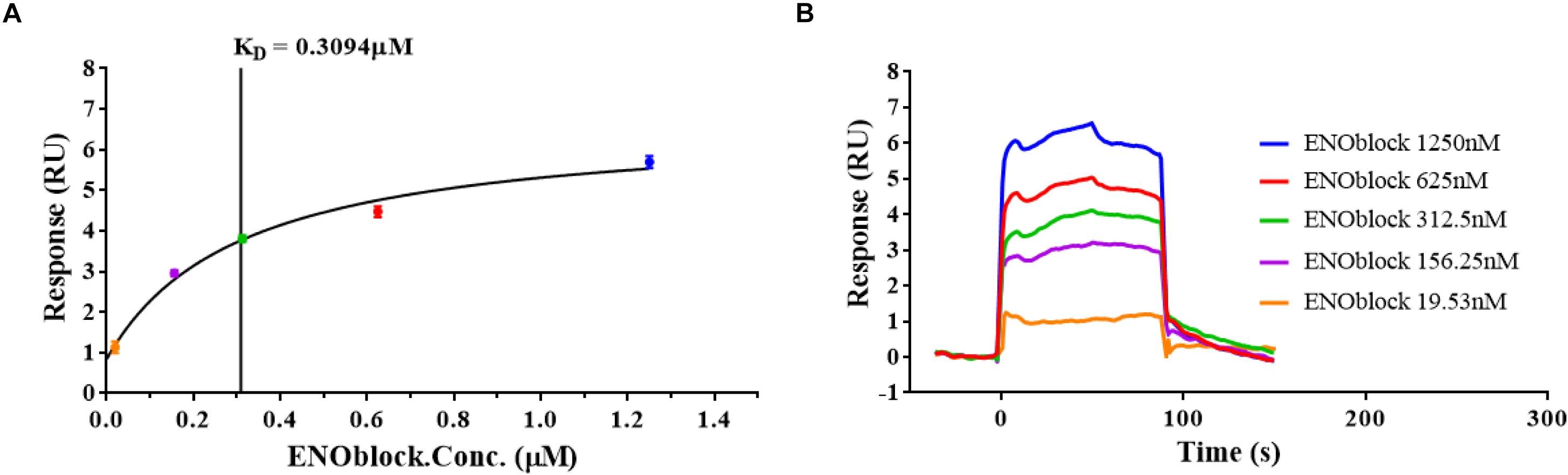
Figure 7. ENOblock binds recombinant C. albicans enolase 1 (rCaEno1). Interactions of rCaEno1 with ENOblock measured by surface plasmon resonance. The rCaEno1 protein was coated on the CM5 sensor chip and serial dilutions of ENOblock (1250, 625, 312.5, 156.25, and 19.53 nM) were used as analytes. Changes in plasmon resonance are shown as response units. (A) The results of the steady-state affinity analysis (KD, equilibrium). Plotted in the left panel (colored circles) is the binding response (RU) versus concentration of ENOblock offered, and the vertical line indicates the binding affinity. (B) Binding curves (colored lines) obtained by passing different concentrations of ENOblock (1250, 625, 312.5, 156.25, and 19.53 nM) over rCaEno1 immobilized on a biosensor surface. The data are representative of two independent experiments.
We then examined the inhibitory effect of ENOblock on the activities of rCaEno1 using the kits. As shown in Figure 8A, the well-known enolase inhibitor sodium fluoride (NaF) dose-dependently inhibited the enolase activity of rCaEno1, while ENOblock did not have a significant effect on it. Intriguingly, the TGase activity of rCaEno1 was significantly inhibited by ENOblock (Figure 8B), and this inhibition was dose dependent, as reflected by a progressive reduction in TGase activity with increasing concentrations of ENOblock (Figure 8C). The half maximal inhibitory concentration (IC50) of TGase inhibition by ENOblock is approximately 12.6 μM (7.95 μg/ml). Therefore, it indicated that the inhibition of TGase activity of CaEno1 by ENOblock resulted in the disruption of hypha and biofilm formation, and affected the cell wall construction of C. albicans, increasing the susceptibility of C. albicans to FLC and contributing to the synergism of FLC and ENOblock.
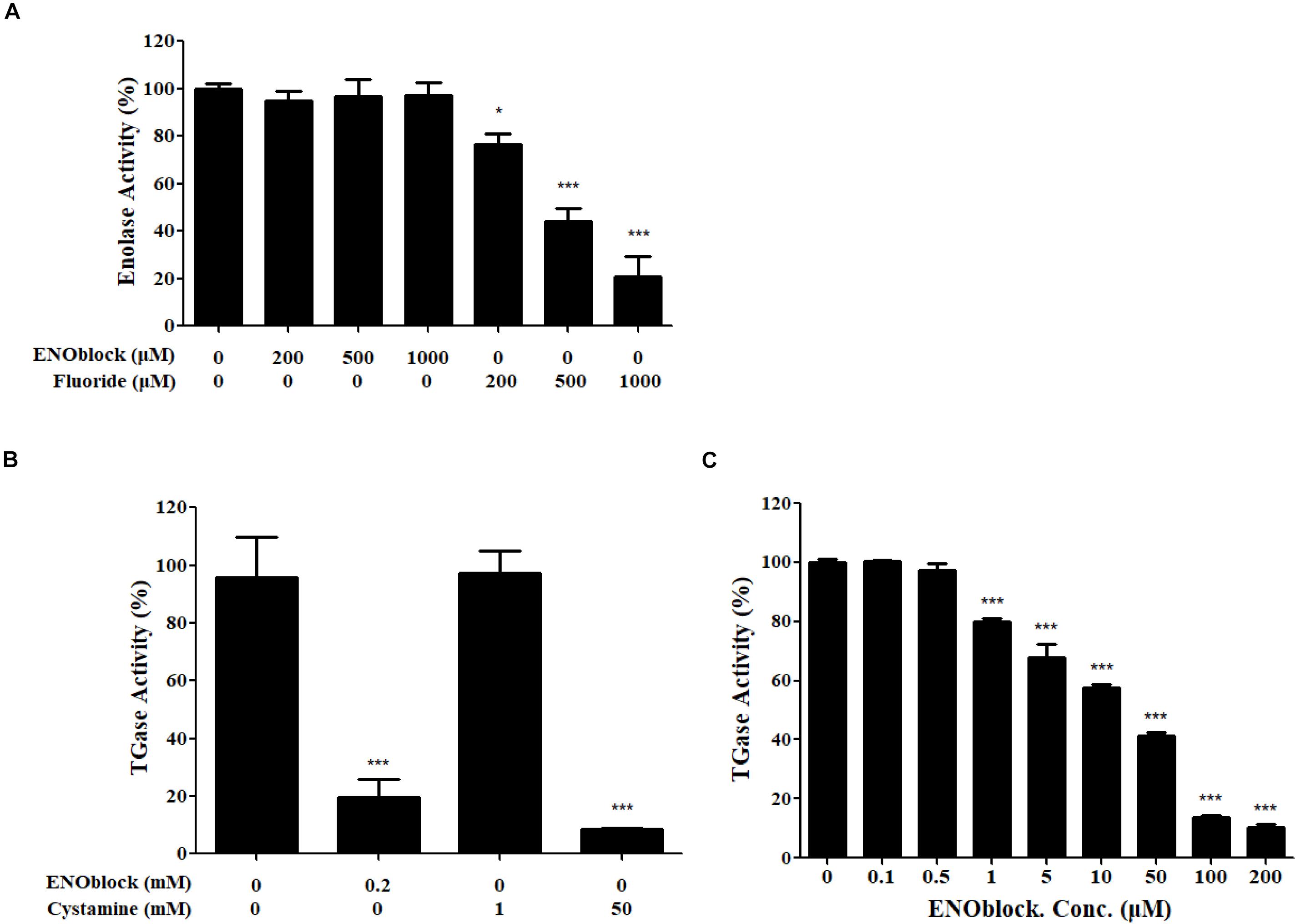
Figure 8. ENOblock inhibits the TGase activity of recombinant C. albicans enolase 1 (rCaEno1). (A) Inhibition of enolase activity was determined with purified rCaEno1 protein with or without different concentrations of ENOblock or sodium fluoride (NaF). The well-known enolase inhibitor NaF was used as a control. (B) Inhibition of TGase activity was determined with purified rCaEno1 protein with ENOblock or the specific inhibitor cystamine used as a control. (C) Inhibition of TGase activity was determined with purified rCaEno1 protein with or without different concentrations of ENOblock. ∗P < 0.05; ∗∗∗P < 0.001 compared to the untreated group (statistical two-tailed unpaired t-test). Data are shown as the means ± standard deviations for three independent experiments.
Discussion
In this study, we focused on evaluating the potential of ENOblock as an antifungal drug by examining its antifungal activities against C. albicans, C. neoformans, and non-albicans Candidaspecies, including C. krusei, C. tropicalis, C. glabratas and C. parapsilosis (Tables 1, 2). ENOblock in combination with FLC showed significant synergistic efficacy against FLC-resistant C. albicans after screening all 30 selected clinical FLC-resistant C. albicans strains. Whereas, FLC and ENOblock did not exhibit synergistic activity against ten FLC-sensitive C. albicans strains tested, clinical C. neoformans 56992, and C. tropicalis ATCC20026 (Tables 1, 2). This finding suggests that their synergistic antifungal activity might be relevant to FLC resistance, or that FLC alone was sufficiently effective against drug-sensitive strains at low concentrations, so the synergism could not be observed. In contrast, FLC alone was not enough for drug-resistant strains, so the synergism was discovered, which is consistent with the hypothesis proposed by Li et al. (2017).
Although, we focused on the antifungal activity of ENOblock alone or in combination with FLC against FLC-resistant C. albicans in this study, biofilm formation assay and antifungal therapy in the murine model were also examined on FLC-sensitive C. albicans SC5314 that is a reference strain which can form normal biofilms. ENOblock alone or in combination with FLC showed significant inhibitory activity against biofilm formation of C. albicans SC5314 (Supplementary Figure S3), and the combination of ENOblock and FLC also showed in vivo activity against C. albicans SC5314 although in vitro synergy measured by antifungal susceptibility test was not observed (Supplementary Figure S4). According to previous reports (Minn et al., 1997; Zhanel et al., 2001), it is known that in vitro testing of some compounds can be used to predict in vivo efficacy, but also may not correlate with in vivo efficacy, due to the influence of serum, formulation of compounds, and some other factors. It is possible to observe the in vivo synergism against C. albicans SC5314 in the animal study.
Enolase 1 was identified as a multifunctional protein showing non-glycolytic functions and is highly conserved across species, including plants, bacteria, and mammals (such as humans). CaEno1 interacts with some proteins of the glycolytic pathway (STRING database) and is also reported to be genetically associated with C. albicans Cbk1 (cell wall biosynthesis kinase), which is involved in regulating cell wall biosynthesis, hyphal morphogenesis and biofilm formation (McNemar and Fonzi, 2002; Song et al., 2008; Gutierrez-Escribano et al., 2012). Some virulence determinants such as Hwp1 in C. albicans cell wall have been shown to serve as acceptors of TGases (Sundstrom, 1999), suggesting that further exploration for targets of CaEno1 as a TGase should be an interesting area.
In addition, CaEno1 is described as an immunodominant antigen that is abundant predominantly in patients with invasive candidiasis and may be used as a biomarker or to produce candidate vaccines for the diagnosis and treatment of invasive candidiasis (Pitarch et al., 2014; Marin et al., 2015). As an integral protein of the cell wall, CaEno1 is responsible for TGase activity, but was also found in the secretome and extracellular vesicles (EVs) of C. albicans via different proteomic approaches, while its function in the secretome and EVs is still unclear (Gil-Bona et al., 2017).
As the first reported non-substrate small-molecule inhibitor of enolase, ENOblock is well tolerated in mice and has been revealed to inhibit cancer cell metastasis in vivo, and have potential anti-diabetic effects in wild-type zebrafish larvae and mammals via the inhibition of non-glycolytic functions of enolase, suggesting ENOblock is a suitable drug candidate for cancer and diabetes (Jung et al., 2013; Cho et al., 2017). In this study, our data confirmed that ENOblock in combination with FLC changed the fungistatic azoles into fungicidal (Figure 1), reversing antifungal drug resistance and broadening the therapeutic implications of combination therapy with azoles. In addition, ENOblock binds rCaEno1 with strong enzymatic activities as described above, and significantly inhibits its TGase activity, possibly contributing to the synergism of ENOblock and FLC. Furthermore, the XTT reduction assay showed the inhibitory effect of ENOblock alone or in combination with FLC against C. albicans biofilm formation, indicating that the binding of ENOblock to CaEno1 might impact interactions between CaEno1 and several important proteins associated with biofilm formation, such as Cbk1 and Hwp1. Although ENOblock has previously been shown to bind human enolase and inhibit its enolase activity, we did not observe any obvious difference in the enolase activity of rCaEno1 in the presence or absence of ENOblock at different concentrations, possibly because ENOblock acts via a mechanism other than direct inhibition of the enolase activity in different organisms (Satani et al., 2016). This finding could also suggest that the conformational difference between rCaEno1 and human enolase may result in no-detectable inhibitory effect of ENOblock on the enolase activity of rCaEno1. The further underlying mechanism for the antifungal effect of ENOblock binding CaEno1, using techniques such as fluorescent probe and the X-ray crystallography, should be worth carrying out.
Conclusion
In conclusion, our present results demonstrate for the first time that ENOblock could enhance the antifungal activity of FLC against FLC-resistant C. albicans in vitro, and significantly improve the activity of FLC in a murine model of systemic candidiasis, while it alone has no therapeutic activity. Furthermore, our data suggest that the synergism of FLC and ENOblock was associated with the disruption of hypha and biofilm formation due to the inhibition of TGase activity of CaEno1 bound by ENOblock, and the potential of ENOblock as a new antifungal candidate. Next, we will carry out the modification and reconstruction of ENOblock as a pro-compound to produce several promising synergists in combination with FLC.
Data Availability
The raw data supporting the conclusions of this manuscript will be made available by the authors, without undue reservation, to any qualified researcher.
Ethics Statement
All animal experiments were performed under the standardized procedures of the “Regulations on the Administration of Laboratory Animals” approved by the State Council of the People’s Republic of China. The animal experimental protocol has been verified and approved by the Animal Care and Use Committee of Tongji University. All of the clinical isolates were provided by the Changhai Hospital of Shanghai, China.
Author Contributions
MA and YJ conceived and designed the experiments. LL, TZ, JX, JW, YW, XQ, YZ, WH, and LY performed the experiments. LL and MA analyzed the data. LL wrote the manuscript.
Funding
This study was supported by the National Natural Science Foundation of China (81330083, 81601745, and 81671989).
Conflict of Interest Statement
The authors declare that the research was conducted in the absence of any commercial or financial relationships that could be construed as a potential conflict of interest.
Acknowledgments
We thank Professor Changzhong Wang (School of Integrated Traditional and Western Medicine, Anhui University of Traditional Chinese Medicine, Hefei, China) for providing C. krusei ATCC2340 and C. glabrata ATCC1182. We also thank Changhai Hospital of Shanghai (China) for providing the clinical isolates.
Supplementary Material
The Supplementary Material for this article can be found online at: https://www.frontiersin.org/articles/10.3389/fmicb.2019.02071/full#supplementary-material
FIGURE S1 | Effects of different concentrations of ENOblock in combination with FLC on hypha formation in liquid Lee medium and liquid Spider medium. Representative photomicrographs of indicated C. albicans 0304103 growing in different media including liquid Lee medium (A) and liquid Spider medium (B) with different concentrations of ENOblock (0, 8, 16, and 32 μg/ml) and FLC (0, 2, 4, and 8 μg/ml), for 3.5 h at 37°C, as observed with an inverted phase contrast microscope with a 40 × objective.
FIGURE S2 | Effects of different concentrations of ENOblock in combination with FLC on hypha formation in liquid RPMI 1640 medium. Representative photomicrographs of indicated C. albicans 0304103 grown in liquid RPMI 1640 medium for 3.5 h at 37°C, as observed with an inverted phase contrast microscope with a 40 × objective.
FIGURE S3 | Effects of the combination of ENOblock and FLC on biofilm formation of C. albicans SC5314 in vitro. (A) Effects of different concentrations of ENOblock alone and in combination with 8 μg/ml FLC on C. albicans biofilm formation. (B) Effects of different concentrations of ENOblock alone and in combination with 8 μg/ml FLC on the maintenance of mature biofilms. Biofilm formation was evaluated by the XTT reduction assay by calculating the percentage of viable C. albicans cells relative to the control cells without drug treatment. Data are shown as the means ± standard deviations for three independent experiments. ∗∗P < 0.01; ∗∗∗P < 0.001 compared with the value of the control biofilms.
FIGURE S4 | ENOblock enhances the activity of FLC in a murine model of systemic candidiasis. (A) Kidney CFU assay in mice with systemic candidiasis after 2 days. Female C57BL/6 mice were infected with 5 × 105 CFU of C. albicans SC5314. ENOblock and FLC were administered 2 h post infection. ∗P < 0.05 (P-values are from ANOVA). (B) Survival curves of C57BL/6 mice infected with 5 × 105 CFU of C. albicans SC5314. ENOblock and FLC were administered at 2, 24, and 48 h post infection. The log-rank test was used for statistical analysis.
FIGURE S5 | Expression and purification of recombinant C. albicans enolase 1 (rCaEno1). (A) The rCaEno1 protein was purified by Ni2+-NTA-agarose column chromatography as described, and elution fractions were evaluated by 10% SDS-PAGE. Lane 1, protein marker; lane 2, negative control (empty vector); lane 3, whole-cell protein; lane 4, supernatant fractions; lane 5, precipitation fractions; lanes 6–8, washed fractions; lane 9, purified rCaEno1 fusion protein. (B) Western blot of total proteins from C. albicans SC5314 (lane 2) and purified rCaEno1 protein (lane 3) using mouse anti-rCaEno1 protein antibodies and anti-His-tag polyclonal antibodies (lane 4).
FIGURE S6 | The interactions of rCaEno1 with ENOblock and amphotericin B measured by surface plasmon resonance. The rCaEno1 protein (BSA as the negative control) was coated on the CM5 sensor chip and serial dilutions (1562.5, 781.25, 390.6, 195.3, and 97.6 nM) of ENOblock (AMB as the control) were used as analytes. Changes in plasmon resonance are shown as response units. The panels (A,B) show binding curves (colored lines) obtained by passing different concentrations of ENOblock (1562.5, 781.25, 390.6, 195.3, and 97.6 nM) over rCaEno1 or BSA immobilized on a biosensor surface. The panels (C,D) show binding curves (colored lines) obtained by passing different concentrations of AMB (1562.5, 781.25, 390.6, 195.3, and 97.6 nM) over rCaEno1 or BSA immobilized on a biosensor surface. The data are representative of two independent experiments. AMB, amphotericin B.
TABLE S1 | Interaction of fluconazole (FLC) and ENOblock against 30 FLC-resistant C. albicans.
TABLE S2 | Interaction of fluconazole (FLC) and ENOblock against 10 FLC-sensitive C. albicans.
References
Beedie, S. L., Mahony, C., Walker, H. M., Chau, C. H., Figg, W. D., and Vargesson, N. (2016). Shared mechanism of teratogenicity of anti-angiogenic drugs identified in the chicken embryo model. Sci. Rep. 6:30038. doi: 10.1038/srep30038
Brown, G. D., Denning, D. W., Gow, N. A., Levitz, S. M., Netea, M. G., and White, T. C. (2012). Hidden killers: human fungal infections. Sci. Transl. Med. 4:165rv113. doi: 10.1126/scitranslmed.3004404
Calderone, R., Sun, N., Gay-Andrieu, F., Groutas, W., Weerawarna, P., Prasad, S., et al. (2014). Antifungal drug discovery: the process and outcomes. Future Microbiol. 9, 791–805. doi: 10.2217/fmb.14.32
Canton, E., Peman, J., Gobernado, M., Viudes, A., and Espinel-Ingroff, A. (2005). Synergistic activities of fluconazole and voriconazole with terbinafine against four Candida species determined by checkerboard, time-kill, and Etest methods. Antimicrob. Agents Chemother. 49, 1593–1596. doi: 10.1128/AAC.49.4.1593-1596.2005
Cho, H., Um, J., Lee, J. H., Kim, W. H., Kang, W. S., Kim, S. H., et al. (2017). ENOblock, a unique small molecule inhibitor of the non-glycolytic functions of enolase, alleviates the symptoms of type 2 diabetes. Sci. Rep. 7:44186. doi: 10.1038/srep44186
Gil-Bona, A., Amador-Garcia, A., Gil, C., and Monteoliva, L. (2017). The external face of Candida albicans: a proteomic view of the cell surface and the extracellular environment. J. Proteomics 180, 70–79. doi: 10.1016/j.jprot.2017.12.002
Gow, N. A. R., and Yadav, B. (2017). Microbe profile: Candida albicans: a shape-changing, opportunistic pathogenic fungus of humans. Microbiology 163, 1145–1147. doi: 10.1099/mic.0.000499
Gutierrez-Escribano, P., Zeidler, U., Suarez, M. B., Bachellier-Bassi, S., Clemente-Blanco, A., Bonhomme, J., et al. (2012). The NDR/LATS kinase Cbk1 controls the activity of the transcriptional regulator Bcr1 during biofilm formation in Candida albicans. PLoS Pathog. 8:e1002683. doi: 10.1371/journal.ppat.1002683
Iranzo, M., Aguado, C., Pallotti, C., Canizares, J. V., and Mormeneo, S. (2002). Transglutaminase activity is involved in Saccharomyces cerevisiae wall construction. Microbiology 148, 1329–1334. doi: 10.1099/00221287-148-5-1329
Jung, D. W., Kim, W. H., Park, S. H., Lee, J., Kim, J., Su, D., et al. (2013). A unique small molecule inhibitor of enolase clarifies its role in fundamental biological processes. ACS Chem. Biol. 8, 1271–1282. doi: 10.1021/cb300687k
Ko, H. C., Hsiao, T. Y., Chen, C. T., and Yang, Y. L. (2013). Candida albicans ENO1 null mutants exhibit altered drug susceptibility, hyphal formation, and virulence. J. Microbiol. 51, 345–351. doi: 10.1007/s12275-013-2577-z
Kohli, A., Smriti, Mukhopadhyay, K., Rattan, A., and Prasad, R. (2002). In vitro low-level resistance to azoles in Candida albicans is associated with changes in membrane lipid fluidity and asymmetry. Antimicrob. Agents Chemother. 46, 1046–1052. doi: 10.1128/aac.46.4.1046-1052.2002
Li, D. D., Chai, D., Huang, X. W., Guan, S. X., Du, J., Zhang, H. Y., et al. (2017). Potent in vitro synergism of fluconazole and osthole against fluconazole-resistant Candida albicans. Antimicrob. Agents Chemother. 61:e00436-17. doi: 10.1128/AAC.00436-17
Li, L., An, M., Shen, H., Huang, X., Yao, X., Liu, J., et al. (2015a). The non-geldanamycin Hsp90 inhibitors enhanced the antifungal activity of fluconazole. Am. J. Transl. Res. 7, 2589–2602.
Li, L. P., Liu, W., Liu, H., Zhu, F., Zhang Da, Z., Shen, H., et al. (2015b). Synergistic antifungal activity of berberine derivative B-7b and fluconazole. PLoS One 10:e0126393. doi: 10.1371/journal.pone.0126393
Ma, B., Niu, C., Zhou, Y., Xue, X., Meng, J., Luo, X., et al. (2016). The disulfide bond of the peptide thanatin is dispensible for its antimicrobial activity in vivoandin vitro. Antimicrob. Agents Chemother. 60, 4283–4289. doi: 10.1128/aac.00041-16
Marin, E., Parra-Giraldo, C. M., Hernandez-Haro, C., Hernaez, M. L., Nombela, C., Monteoliva, L., et al. (2015). Candida albicans shaving to profile human serum proteins on hyphal surface. Front. Microbiol. 6:1343. doi: 10.3389/fmicb.2015.01343
McNemar, M. D., and Fonzi, W. A. (2002). Conserved serine/threonine kinase encoded by CBK1 regulates expression of several hypha-associated transcripts and genes encoding cell wall proteins in Candida albicans. J. Bacteriol. 184, 2058–2061. doi: 10.1128/jb.184.7.2058-2061.2002
Minn, Y., Brummer, E., and Stevens, D. A. (1997). Effect of iron on fluconazole activity against Candida albicans in presence of human serum or monocyte-derived macrophages. Mycopathologia 138, 29–35.
Ng, K. P., Kuan, C. S., Kaur, H., Na, S. L., Atiya, N., and Velayuthan, R. D. (2015). Candida species epidemiology 2000-2013: a laboratory-based report. Trop. Med. Int. Health 20, 1447–1453. doi: 10.1111/tmi.12577
Odds, F. C. (2003). Synergy, antagonism, and what the chequerboard puts between them. J. Antimicrob. Chemother. 52:1. doi: 10.1093/jac/dkg301
Pankey, G., Ashcraft, D., Kahn, H., and Ismail, A. (2014). Time-kill assay and etest evaluation for synergy with polymyxin B and fluconazole against Candida glabrata. Antimicrob. Agents Chemother. 58, 5795–5800. doi: 10.1128/aac.03035-14
Paulone, S., Malavasi, G., Ardizzoni, A., Orsi, C. F., Peppoloni, S., Neglia, R. G., et al. (2017). Candida albicans survival, growth and biofilm formation are differently affected by mouthwashes: an in vitro study. New Microbiol. 40, 45–52.
Perfect, J. R. (2016). Is there an emerging need for new antifungals? Expert Opin. Emerg. Drugs 21, 129–131. doi: 10.1517/14728214.2016.1155554
Pfaller, M. A., and Diekema, D. J. (2007). Epidemiology of invasive candidiasis: a persistent public health problem. Clin. Microbiol. Rev. 20, 133–163. doi: 10.1128/CMR.00029-06
Pitarch, A., Nombela, C., and Gil, C. (2014). Serum antibody signature directed against Candida albicans Hsp90 and enolase detects invasive candidiasis in non-neutropenic patients. J. Proteome Res. 13, 5165–5184. doi: 10.1021/pr500681x
Quan, H., Cao, Y. Y., Xu, Z., Zhao, J. X., Gao, P. H., Qin, X. F., et al. (2006). Potent in vitro synergism of fluconazole and berberine chloride against clinical isolates of Candida albicans resistant to fluconazole. Antimicrob. Agents Chemother. 50, 1096–1099. doi: 10.1128/AAC.50.3.1096-1099.2006
Radwan, M. A., Alquadeib, B. T., Siller, L., Wright, M. C., and Horrocks, B. (2017). Oral administration of amphotericin B nanoparticles: antifungal activity, bioavailability and toxicity in rats. Drug Deliv. 24, 40–50. doi: 10.1080/10717544.2016.1228715
Rajendran, R., Sherry, L., Nile, C. J., Sherriff, A., Johnson, E., Hanson, M., et al. (2015). Biofilm formation is a risk factor for mortality in patients with Candida albicans bloodstream infection - Scotland, 2012-2013. Clin. Microbiol. Infect. 22, 87–93. doi: 10.1016/j.cmi.2015.09.018
Ramage, G., Mowat, E., Jones, B., Williams, C., and Lopez-Ribot, J. (2009). Our current understanding of fungal biofilms. Crit. Rev. Microbiol. 35, 340–355. doi: 10.3109/10408410903241436
Ramage, G., Saville, S. P., Wickes, B. L., and Lopez-Ribot, J. L. (2002). Inhibition of Candida albicans biofilm formation by farnesol, a quorum-sensing molecule. Appl. Environ. Microbiol. 68, 5459–5463. doi: 10.1128/aem.68.11.5459-5463.2002
Ramage, G., Vande Walle, K., Wickes, B. L., and Lopez-Ribot, J. L. (2001). Standardized method for in vitro antifungal susceptibility testing of Candida albicans biofilms. Antimicrob. Agents Chemother. 45, 2475–2479. doi: 10.1128/aac.45.9.2475-2479.2001
Reyna-Beltran, E., Iranzo, M., Calderon-Gonzalez, K. G., Mondragon-Flores, R., Labra-Barrios, M. L., Mormeneo, S., et al. (2018). The Candida albicans ENO1 gene encodes a transglutaminase involved in growth, cell division, morphogenesis, and osmotic protection. J. Biol. Chem. 293, 4304–4323. doi: 10.1074/jbc.M117.810440
Robbins, N., Caplan, T., and Cowen, L. E. (2017). Molecular evolution of antifungal drug resistance. Annu. Rev. Microbiol. 71, 753–775. doi: 10.1146/annurev-micro-030117-020345
Ruiz-Herrera, J., Iranzo, M., Elorza, M. V., Sentandreu, R., and Mormeneo, S. (1995). Involvement of transglutaminase in the formation of covalent cross-links in the cell wall of Candida albicans. Arch. Microbiol. 164, 186–193. doi: 10.1007/s002030050253
Sarkar, S., Uppuluri, P., Pierce, C. G., and Lopez-Ribot, J. L. (2014). In vitro study of sequential fluconazole and caspofungin treatment against Candida albicans biofilms. Antimicrob. Agents Chemother. 58, 1183–1186. doi: 10.1128/AAC.01745-13
Satani, N., Lin, Y. H., Hammoudi, N., Raghavan, S., Georgiou, D. K., and Muller, F. L. (2016). ENOblock does not inhibit the activity of the glycolytic enzyme enolase. PLoS One 11:e0168739. doi: 10.1371/journal.pone.0168739
Scudiero, D. A., Shoemaker, R. H., Paull, K. D., Monks, A., Tierney, S., Nofziger, T. H., et al. (1988). Evaluation of a soluble tetrazolium/formazan assay for cell growth and drug sensitivity in culture using human and other tumor cell lines. Cancer Res. 48, 4827–4833.
Song, Q., Song, H., Xu, J., Huang, J., Hu, M., Gu, X., et al. (2016). Biomimetic ApoE-reconstituted high density lipoprotein nanocarrier for blood-brain barrier penetration and amyloid beta-targeting drug delivery. Mol. Pharm. 13, 3976–3987. doi: 10.1021/acs.molpharmaceut.6b00781
Song, Y., Cheon, S. A., Lee, K. E., Lee, S. Y., Lee, B. K., Oh, D. B., et al. (2008). Role of the RAM network in cell polarity and hyphal morphogenesis in Candida albicans. Mol. Biol. Cell 19, 5456–5477. doi: 10.1091/mbc.E08-03-0272
Staab, J. F., Bradway, S. D., Fidel, P. L., and Sundstrom, P. (1999). Adhesive and mammalian transglutaminase substrate properties of Candida albicans Hwp1. Science 283, 1535–1538. doi: 10.1126/science.283.5407.1535
Sundstrom, P. (1999). Adhesins in Candida albicans. Curr. Opin. Microbiol. 2, 353–357. doi: 10.1016/S1369-5274(99)80062-9
Tobudic, S., Lassnigg, A., Kratzer, C., Graninger, W., and Presterl, E. (2010). Antifungal activity of amphotericin B, caspofungin and posaconazole on Candida albicans biofilms in intermediate and mature development phases. Mycoses 53, 208–214. doi: 10.1111/j.1439-0507.2009.01690.x
Vyas, V. K., Bushkin, G. G., Bernstein, D. A., Getz, M. A., Sewastianik, M., Barrasa, M. I., et al. (2018). New CRISPR mutagenesis strategies reveal variation in repair mechanisms among fungi. mSphere 3:e00154-18. doi: 10.1128/mSphere.00154-18
White, T. C., Holleman, S., Dy, F., Mirels, L. F., and Stevens, D. A. (2002). Resistance mechanisms in clinical isolates of Candida albicans. Antimicrob. Agents Chemother. 46, 1704–1713. doi: 10.1128/aac.46.6.1704-1713.2002
White, T. C., Marr, K. A., and Bowden, R. A. (1998). Clinical, cellular, and molecular factors that contribute to antifungal drug resistance. Clin. Microbiol. Rev 11, 382–402. doi: 10.1128/cmr.11.2.382
Yang, M., Du, K., Hou, Y., Xie, S., Dong, Y., Li, D., et al. (2019). Synergistic antifungal effect of amphotericin B-loaded poly(Lactic-Co-Glycolic acid) nanoparticles and ultrasound against Candida albicans biofilms. Antimicrob. Agents Chemother. 63:e02022-18. doi: 10.1128/AAC.02022-18
Yang, Y. L., Chen, H. F., Kuo, T. J., and Lin, C. Y. (2006). Mutations on CaENO1 in Candida albicans inhibit cell growth in the presence of glucose. J. Biomed. Sci. 13, 313–321. doi: 10.1007/s11373-005-9054-6
Zhanel, G. G., Saunders, D. G., Hoban, D. J., and Karlowsky, J. A. (2001). Influence of human serum on antifungal pharmacodynamics with Candida albicans. Antimicrob. Agents Chemother. 45, 2018–2022. doi: 10.1128/AAC.45.7.2018-2022.2001
Keywords: Candida albicans, ENOblock, fluconazole, synergism, enolase1, transglutaminase activity
Citation: Li L, Zhang T, Xu J, Wu J, Wang Y, Qiu X, Zhang Y, Hou W, Yan L, An M and Jiang Y (2019) The Synergism of the Small Molecule ENOblock and Fluconazole Against Fluconazole-Resistant Candida albicans. Front. Microbiol. 10:2071. doi: 10.3389/fmicb.2019.02071
Received: 29 May 2019; Accepted: 22 August 2019;
Published: 06 September 2019.
Edited by:
Rustam Aminov, University of Aberdeen, United KingdomReviewed by:
Ashutosh Singh, University of Lucknow, IndiaMasoomeh Shams-Ghahfarokhi, Tarbiat Modares University, Iran
Copyright © 2019 Li, Zhang, Xu, Wu, Wang, Qiu, Zhang, Hou, Yan, An and Jiang. This is an open-access article distributed under the terms of the Creative Commons Attribution License (CC BY). The use, distribution or reproduction in other forums is permitted, provided the original author(s) and the copyright owner(s) are credited and that the original publication in this journal is cited, in accordance with accepted academic practice. No use, distribution or reproduction is permitted which does not comply with these terms.
*Correspondence: Maomao An, YW5tYW9tYW9AdG9uZ2ppLmVkdS5jbg==; Yuanying Jiang, MTM3NjE1NzE1NzhAMTYzLmNvbQ==