- Faculty of Science, Engineering and Built Environment, School of Life and Environmental Sciences, Centre for Integrative Ecology, Deakin University, Burwood, VIC, Australia
Viruses are non-living, acellular entities, and the most abundant biological agents on earth. They are widely acknowledged as having the capacity to influence global biogeochemical cycles by infecting the bacterial and archaeal populations that regulate carbon and nutrient turnover. Evidence suggests that the majority of viruses in wetlands are bacteriophages, but despite their importance, studies on how viruses control the prokaryotic community and the concomitant impacts on ecosystem function (such as carbon cycling and greenhouse gas flux) in wetlands are rare. Here we investigate virus-prokaryote interactions in freshwater wetland ecosystems in the context of their potential influence on biogeochemical cycling. Specifically, we (1) synthesize existing literature to establish current understanding of virus-prokaryote interactions, focusing on the implications for wetland greenhouse gas dynamics and (2) identify future research priorities. Viral dynamics in freshwater wetlands have received much less attention compared to those in marine ecosystems. However, based on our literature review, within the last 10 years, viral ecology studies on freshwater wetlands have increased twofold. Despite this increase in literature, the potential implication of viral infections on greenhouse gas emission dynamics is still a knowledge gap. We hypothesize that the rate of greenhouse gas emissions and the pool of sequestered carbon could be strongly linked to the type and rate of viral infection. Viral replication mechanism choice will consequently influence the microbial efficiency of organic matter assimilation and thus the ultimate fate of carbon as a greenhouse gas or stored in soils.
Introduction
The most widely used definition of wetlands under the Ramsar convention is “areas of marsh, fen, peatland or water, whether natural or artificial, permanent or temporary, with water that is static or flowing, fresh, brackish or salt, including areas of marine water the depth of which at low tide does not exceed six metres.” According to this definition, what is formally known as “wetland” spans a huge and diverse group of ecosystems that are all characterized by the presence of water (temporary or permanently) and aquatic macrophytes (Mitsch and Gosselink, 2007). Although many classification systems have been identified over the past years (Shaw and Fredine, 1956; Cowardin et al., 1979), here we use the classification system adopted by Mitsch and Gosselink (2007), whereby wetlands are classified into seven broad types (see Supplementary Table S1), with a particular focus on freshwater ecosystems. Wetlands are broadly considered one of earth’s most efficient ecosystems for sequestering and storing carbon (Delwart, 2007; Limpens et al., 2008; Dise, 2009; Kirwan and Mudd, 2012), thereby reducing the concentration of atmospheric carbon dioxide (Mitsch and Gosselink, 2007). As governments across the globe try to reduce their greenhouse gas emissions, wetlands have become an important area for both reducing emissions and increasing carbon sequestration. The accurate measurement of the pool of sequestered carbon and gas emissions is crucial to inform overall greenhouse gas budgets, as well as quantifying opportunities for achieving emission reductions through nature-based approaches [“biosequestration”; (Carnell et al., 2018)]. Microbes are often considered the “gatekeepers” of the carbon cycle in marine ecosystems (Arnosti, 2011). In freshwater and coastal wetland ecosystems, the prokaryotic communities are responsible for aerobic (i.e., respiration) and anaerobic (i.e., methanogenesis, denitrification, and sulfate reduction) metabolic pathways that produce greenhouse gases from wetland soils. However, a part of this carbon bypasses microbial remineralization becoming more likely to be permanently sequestered into the soil (Bodelier and Dedysh, 2013; Boon et al., 2014).
In marine and aquatic ecosystems, the “microbial loop” is the pathway that regulates organic matter transfer from prokaryotes to higher trophic levels, but the exact function of prokaryotes in this process is unclear. Different models concerning the existence of the microbial loop in pelagic ecosystems with no benthic influence nor empirical measurements (Azam et al., 1983) and suggesting the role of heterotrophic prokaryotes as carbon sink (Ducklow et al., 1986) or carbon link (Sherr et al., 1987, 1988) have been proposed over the last 40 years. In 1990s, the role viruses were suggested to be key in diverting carbon flow from the higher trophic levels, thereby short-circuiting the microbial loop (Fuhrman, 1999). Viral abundance in soil and aquatic (freshwater and marine) sediments worldwide is estimated to range from 107 to 109 viruses g−1 dry mass of sediment (Danovaro et al., 2008a; Williamson et al., 2017). Since viruses need a host to complete their replication cycle, this high abundance of viruses in nature suggests that large numbers of soil-based organisms experience viral infections. In freshwater ecosystems, emerging evidence suggests that bacteriophages likely represent the dominant category of viruses; however, viruses infecting algae, protozoa, and plants might also be presented (Wommack and Colwell, 2000; Jackson and Jackson, 2008).
In this perspective, we focus on the functional role of viruses in freshwater wetlands, discussing the current literature on wetland microbiology and biogeochemical cycling and virus-prokaryote interactions in marine, aquatic, and terrestrial ecosystems. We conclude with three specific questions, followed by our hypotheses, we consider as key points to progress environmental virology in wetlands, focusing on viral infection strategies within the context of carbon cycling and greenhouse gas emission.
Virus-Prokaryote Interactions
Jackson and Jackson reported in their 2008 review that less than 50 studies have published on topics concerning the keywords “wetland, swamp, pond, bog and virus, phage, temperate, lytic, lysogenic.” The majority of the resulting studies had been conducted in constructed wetlands, rather than natural wetlands. We performed a similar bibliographic research by using the same keywords and search tools (Science Direct, WoK, PubMed, Google Scholar, and Scopus). Only studies with the combination of wetland-related keywords and virus-related keywords in the title, abstract, or keywords were included. Unpublished studies, studies published in languages other than English, and studies on pathogens and pathogen removal were excluded. We found that in the last 10 years since Jackson and Jackson (2008), <30 studies had been published and the research focus on freshwater virology has increased twofold ranging from quantitative and qualitative aspects of the viral ecology to virus-prokaryote interactions, metagenomics, and method development (Supplementary Figures S1A,B, Supplementary Table S1). Most of the studies performed in riparian wetlands (Rooks et al., 2010; Fancello et al., 2013; Kyle and Grant, 2013; Basemer, 2015; Alexyuk et al., 2016; Saxton et al., 2016) and freshwater marshes (Leroy et al., 2008; Thomas et al., 2011; Kyle and Grant, 2013; Zheng et al., 2013; Motlagh et al., 2017; Qin et al., 2017; Dalcin Martins et al., 2018). Few studies were also performed in wetland microcosms (Pradeep Ram and Sime-Ngando, 2008, 2010; Esteban et al., 2015), and few others were in the form of reviews (Middelboe et al., 2008; Wilhelm and Matteson, 2008; Danovaro et al., 2008a; Neori Amir, 2017). The lack of studies in tidal salt marsh and mangrove swamps might be explained with the use of “freshwater” as key word (Supplementary Table S1).
Viruses are widely acknowledged as being the most numerous entity on earth, exceeding prokaryotic abundance by at least one order of magnitude (Danovaro et al., 2008b), yet what is known about the roles of viruses in pelagic ocean ecosystems vastly outweighs our knowledge of viral ecology in wetlands. This limitation is also related to the current challenge of extracting, concentrating, and purifying viral particles from soil material, as well as the complexity of developing and testing several protocols for different soil habitats (Pratama and van Elsas, 2018; Emerson, 2019). The microscale variation within the soil affects the prokaryotic diversity and should then modulate the abundance and diversity of the relative bacteriophages (Fierer et al., 2012; Pratama and van Elsas, 2018). In addition, experiments conducted in thawing permafrost ecosystems suggested that viral infection dynamics might be driven by host lineages (Emerson, 2019). The dynamics underpinning a “top-down” (predation on prokaryotes) or “bottom-up” (resource availability) ecosystem regulation in wetlands remain unclear despite the recognized potential importance of virus-prokaryote interactions in regulating the ocean’s biogeochemical cycles (Pradeep Ram and Sime-Ngando, 2008). Within the microbial loop, protists are bacterivores that have been acknowledged as the major control over the prokaryotes by impacting abundance, production, and community composition. However, the discovery in the late 1980s of the high number of viruses in aquatic ecosystems (108–1011) suggested that viruses are also a significant top-down control on the prokaryotic populations (Weinbauer, 2004; Fischer et al., 2006; Suttle, 2007) and viral-mediated mortality of prokaryotes makes up ca. 10–50% of the prokaryotic daily production (Mostajir et al., 2015). Since the prokaryotes are an important source of enzymes, which are known to be involved in the carbon cycle (Karner et al., 1994; Bochdansky et al., 1995; Arnosti, 2011), viral-mediated mortality of prokaryotes can stop the carbon from flowing to the upper trophic levels (Pradeep Ram and Sime-Ngando, 2008).
The main focus of viral-relative research to-date has been on their roles as pathogens across a range of ecosystems, rather than their ecological function (Farnell-Jackson and Ward, 2003). As a result of our review, what we do know about viral ecology in marine, aquatic, and terrestrial ecosystems are their role as a major controller of bacterial mortality that results in the diversion of nutrient flows, in preventing algal blooms and promoting genetic exchange across distantly related species as well as their roles as components of sinking particles (Brum et al., 2015; Stroynov and Philippov, 2017; Williamson et al., 2017; Dalcin Martins et al., 2018). Temperate viruses are one of the major causes of mortality for a wide range of organisms, and in the wetlands, it seems that the majority of these viruses are bacteriophages that infect prokaryotes (Wommack and Colwell, 2000; Jackson and Jackson, 2008). The viral infection occurs through a lytic (destruction of the host cell after viral replication) or lysogenic (host cell division with part of the viral genome integrated in the host cell) cycle, and the inclination to one of these replication mechanisms is driven by biotic and/or abiotic factors, such as host availability or the presence of unfavorable conditions for the viral communities (Danovaro et al., 2008a).
The survival of viruses relies upon the number and diversity of their prokaryotic hosts, and in turn, prokaryotic growth and abundance rely on the availability of organic and inorganic matter (Pradeep Ram and Sime-Ngando, 2008). In freshwater environments, the relatively high carbon, nitrogen, and phosphorus availability seems to encourage the choice of a lytic cycle, while the resource limitation seems to yield a lysogenic infection mechanism (Wilson and Mann, 1997; Pradeep Ram and Sime-Ngando, 2010). There is evidence that when phosphorus is limited, the lytic pathway can be hindered due to the resulting negative effect on viral DNA structure (i.e., phosphate backbone; Pradeep Ram and Sime-Ngando, 2010; Thomas et al., 2011).
Acting as top-down control, viruses play a key role in regulating the prokaryotic component of the microbial loop. According to the “Kill-the-Winner” model (KtW), by infecting the most abundant population, viruses are predicted to control the food competition among prokaryotic species or functional groups, which is particularly important under resource-limited conditions (Pradeep Ram and Sime-Ngando, 2008). In this top-down control scenario, viral lysis releases the prokaryotic dissolved organic carbon (hereafter referred to as “DOC”), which is then recycled back into the system and made available for the local prokaryotic population. In this way, viruses presumably contribute to organic carbon bioavailability and can simultaneously be considered as bottom-up controls on the prokaryotic communities that remineralize the “viral-produced” DOC again (Azam et al., 1983; del Giorgio and Williams, 2005; Robinson, 2008). The amount of organic carbon that prokaryotes uptake into their biomass (or synthesis) versus what is respired as CO2, CH4 or N2O depends on the microbial growth efficiency (MGE), also referred to as carbon-use efficiency (Sterner and Elser, 2002; Manzoni et al., 2012; Wieder et al., 2013).
Knowles and colleagues recently proposed a new model that contrasts the KtW called the “Piggyback the Winner” (PtW) model. The PtW model outlines that during prokaryotic bloom events lysogeny is promoted (low energy cost of superinfection immunity vs. mutation to combat phage infection) resulting in viruses integrating themselves into the host instead of killing it and explaining the low abundance of viruses detected (Knowles et al., 2016; Pratama and van Elsas, 2018). Although the PtW model has been debated taking also into account the evidence of decreasing lysogenic cells with increasing host density observed in aquatic ecosystems (Brum et al., 2016; Weitz et al., 2016), both KtW and PtW models are considered key paradigms to explain virus-host dynamics in soil ecosystems (Pratama and van Elsas, 2018).
From the literature, we know that for prokaryotes in terrestrial soil there is a positive correlation between the MGE and the CO2 production, but we lack the mechanistic drivers behind this respiration and carbon assimilation/resynthesis (Schimel, 2013). Based on the literature to-date, we hypothesize that there is an important correlation between the viral infection mechanisms and the MGE that consequently affects on the carbon cycling in wetland soils.
Wetland Viral Ecology: Questions, Hypotheses, and Future Directions
Based on our literature review and synthesis in the following section, we propose three key questions and our respective hypotheses on virus-prokaryote interactions in freshwater wetlands. These key questions represent the future research directions needed to improve our understanding of the entire microbial loop in freshwater wetlands, and thus, a more complete understanding on how greenhouse gas and carbon sequestration can be managed in these ecosystems.
How Does Viral Replication Mechanism Influence the Microbial Efficiency, the Carbon Sequestration Rate, and the Greenhouse Gas Emissions in Freshwater Wetlands?
Viruses are acellular and inert entities that require infection of host cells to replicate. Temperate viruses are bacteriophages that accomplish their reproductive cycle via a lysogenic or lytic strategy (Ackermann and DuBow, 1987; Weinbauer, 2004). The viral infection through the lytic cycle is a key process in the biogeochemical cycling, especially nutrient turnover, whereby the organic material assimilated by the prokaryotes is released back into the environment once the cell lysis is completed. Additionally, the viral component of the microbial loop is thought to prevent the dominance of few species and niche competition, thus promoting biodiversity (Mostajir et al., 2015). During lytic infection, viruses may also acquire specific functional genes from the host, frequently referred to as auxiliary metabolic genes (AMGs). Recent studies conducted on pelagic marine ecosystems, thawing permafrost, hypolith organisms, and soil viruses have discovered a rich diversity of AMGs capable of altering prokaryotic metabolism and/or improving viral fitness and infection under unfavorable conditions (Hurwitz et al., 2013; Brum and Sullivan, 2015; Pratama and van Elsas, 2018; Sieradzki et al., 2019). These AMGs include genes that encode enzymes involved in functions such as photosynthesis (Mann et al., 2003), metabolism (carbon, nitrogen, sulfur, and lipid; Roux et al., 2016), phosphate regulation (marine; Sullivan et al., 2005), polysaccharide binding, polymer hydrolysis (thawing permafrost; Emerson et al., 2018; Trubl et al., 2018), ribonucleotide reductase (hypolith; Adriaenssens et al., 2015), and atrazine catabolism (soil; Ghosh et al., 2008). Specifically, the virus-encoded polymer hydrolysis might actively contribute to the degradation of complex polymers into smaller forms. The smaller compounds could then be easily accessible to microbes, thus boosting the CO2 and CH4 production (Emerson et al., 2018; Trubl et al., 2018).
Viral infections also strongly modulate prokaryotic fitness through infection by the lysogenic cycle, whereby the viral genetic material can be transferred from a lysed to a lysogen host (Horizontal Gene Transfer, HGT). The result would consist of a change in host morphology (lysogenic conversion) and/or the acquisition of immunity from potential phage infection (superinfection immunity; Weinbauer, 2004; Williamson et al., 2017). During lysogeny the viral genome expression may lead to (1) a reduction in the host’s metabolism and (2) a decrease in organic and inorganic matter utilization and growth rate compared to uninfected hosts, as demonstrated with culturable bacteria (Chen et al., 2005; Paul, 2008). Given this, we argue that a strong lysogenic infection might decrease the MGE and the associated substrate usage, resulting in the increase of the carbon not consumed (i.e., remineralized) by the prokaryotic members of the microbial loop. On the other hand, a strong lytic infection and viral shunt might increase the rates of microbial-mediated recycling of the sedimentary organic matter and resulting CO2 or CH4 production, and we hypothesize that this pathway will have significant negative consequences on carbon cycling and nutrient regeneration in freshwater wetlands, as already demonstrated in marine ecosystems (Corinaldesi et al., 2012). Therefore, we hypothesize that both greenhouse gas emissions and sequestered soil organic carbon are strongly linked to the lytic and lysogenic infection rates (Figure 1), and thus, conditions that trigger one viral strategy over the other may be important levers for manipulation and management of wetland ecosystems.
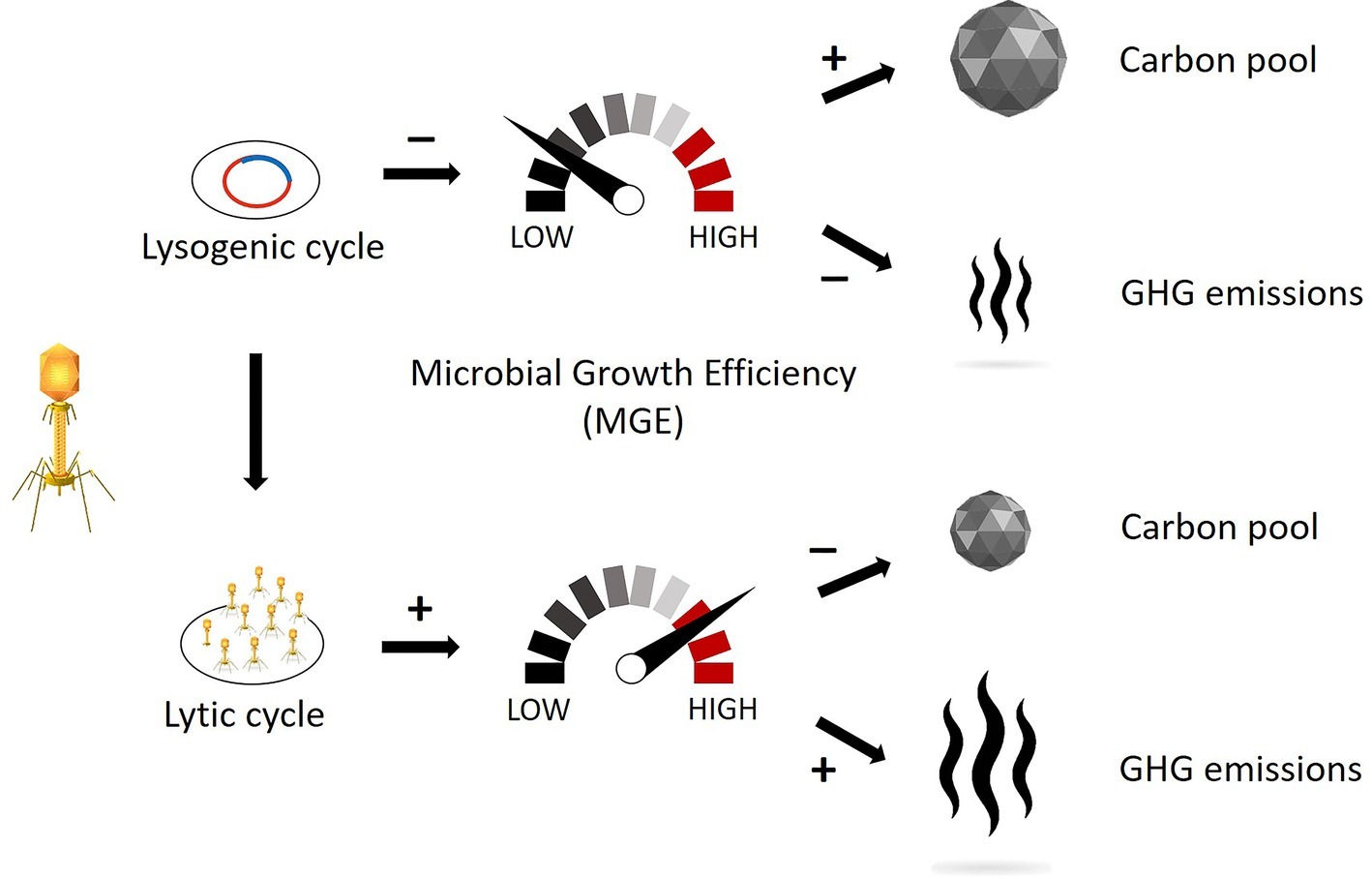
Figure 1. Schematic representation of how different viral replication mechanisms might influence the microbial growth efficiency (MGE) and, consequently, the pool of sequestered carbon and the emissions of greenhouse gas. The lysogenic cycle affects prokaryotic metabolism by decreasing the organic matter usage and thus reducing the carbon decomposition. The lytic cycle affects the carbon turnover by recycling the organic matter already assimilated by prokaryotes and thus increasing the labile carbon available for decomposition. (Vectors used under license from Shutterstock.com).
How Does Flooding Affect Viral Infection and Organic Matter Dynamics?
As outlined above, viruses play a critical role in diverting the carbon flow from the microbial component back to the ecosystem by short-circuiting the microbial loop. However, in aquatic ecosystems, the presence of viruses within sinking organic aggregates also influences the carbon flux (Weinbauer, 2004; Brum et al., 2015; Guidi et al., 2016). Briefly, it is thought that the DOC released by the viral shunt, i.e., cell lysis, is typically consumed by prokaryotes (Azam et al., 1983; del Giorgio and Williams, 2005; Robinson, 2008). However, recent findings have highlighted how part of the carbon released may coagulate, thus increasing the production rate of aggregates (Weinbauer, 2004; Guidi et al., 2016). On the other side, prokaryotes that colonize sinking particles produce more extracellular enzymes compared to the free-living counterpart and would contribute to aggregate degradation (Weinbauer, 2004). The viral shunt has already been proposed in marine ecosystems as one of the most relevant processes in the production of recalcitrant dissolved organic matter (i.e., “The biological carbon pump”; Jiao et al., 2010). In the marine scenario, the carbon released by the viral shunt was primarily chemically labile (i.e., amenable to assimilation by microbes) but ultimately unavailable for remineralization. Microspatial oxygen depletion (Jiao et al., 2010), protist grazing (Strom et al., 1997), and thermal transformation (Benner and Biddanda, 1998) have been shown to be responsible for making the carbon released by the viral shunt resistant to microbial attack. Similarly, the recalcitrant organic matter formation and sequestration in freshwater wetlands could result from the breakdown of the labile organic matter and the DOC turnover.
In contrast to pelagic marine ecosystems, other scenarios and mechanisms could promote the sequestration of carbon released by the viral shunt. First, the interaction between labile organic matter and mineral soil is recognized as a process to make the labile organic matter inaccessible to the prokaryotes, via the formation of recalcitrant soil aggregates or humified organic matter in terrestrial and wetland soils (Davidson and Janssens, 2006; Bridgham et al., 2013a). Second, some wetlands experience regular periods of dryness or drought, e.g., semi-arid wetlands. The resulting reduced water level, soil moisture, and thickness of the water-sediment interface could lead to the reduction in carbon and prokaryotic enzyme diffusion in the porewater, thus reducing the overall metabolic capacity of the soil (Burns and Ryder, 2001). These wetlands starkly contrast to the microbial communities in continually flooded wetlands that do have porewater diffusivity but have to rely on anaerobic metabolic pathways that provide lower energy yields (Davidson and Janssens, 2006).
In dry conditions, soil aggregates produce the spatial heterogeneity that leads to hot spots of microbial diversity. It has been recently proposed how the competitive pressure inside these isolated hot spots might promote viral infections (Pratama and van Elsas, 2018). For instance, lysogeny has been hypothesized as the dominant infection mechanism in soil and, if shown to occur would emphasize the differences between aquatic and soil viral ecology (Kimura et al., 2008; Emerson, 2019). The potential flooding of the soil can disintegrate the microbial aggregates in the dry soil fostering increased virus-host encounters (Pratama and van Elsas, 2018), thus making wetlands unique wet-dry transitional ecosystems. In arctic freshwater wetlands, it has been observed how the thawing permafrost, and the subsequent transition from a dry to a wet state, was driving the shift from soil-like to aquatic-like viruses, which might carry a higher abundance and diversity of AMGs. In addition, given that the glycoside hydrolyze genes involved in polymer hydrolysis could be transferred between hosts through the horizontal gene transfer, this shift likely suggests potential consequences of dry-wet cycles on the carbon cycle (Emerson et al., 2018; Trubl et al., 2018).
However, we still lack comprehensive knowledge and understanding of the organic matter and viral infection dynamics in wetlands that undergo wet-dry and/or dry-wet transitions. We hypothesized that the transition from wet to dry climate will trigger physico-chemical conditions, and a portion of the DOC released by the viral lysis might interact with mineral soil and be removed from the microbial attack, thus available for accumulate into the soils (Figure 2). We also hypothesize that in freshwater wetlands characterized by strong flooding events and dry/wet cycles, there is low metabolic or viral activity in the dry phase, but in the transition or flooded phase, the shift from soil-like to water-like viruses will trigger viral infections and the horizontal gene transfer of AMGs directly involved in carbon metabolism will lead to higher greenhouse gas (GHG) emissions (Figure 2).
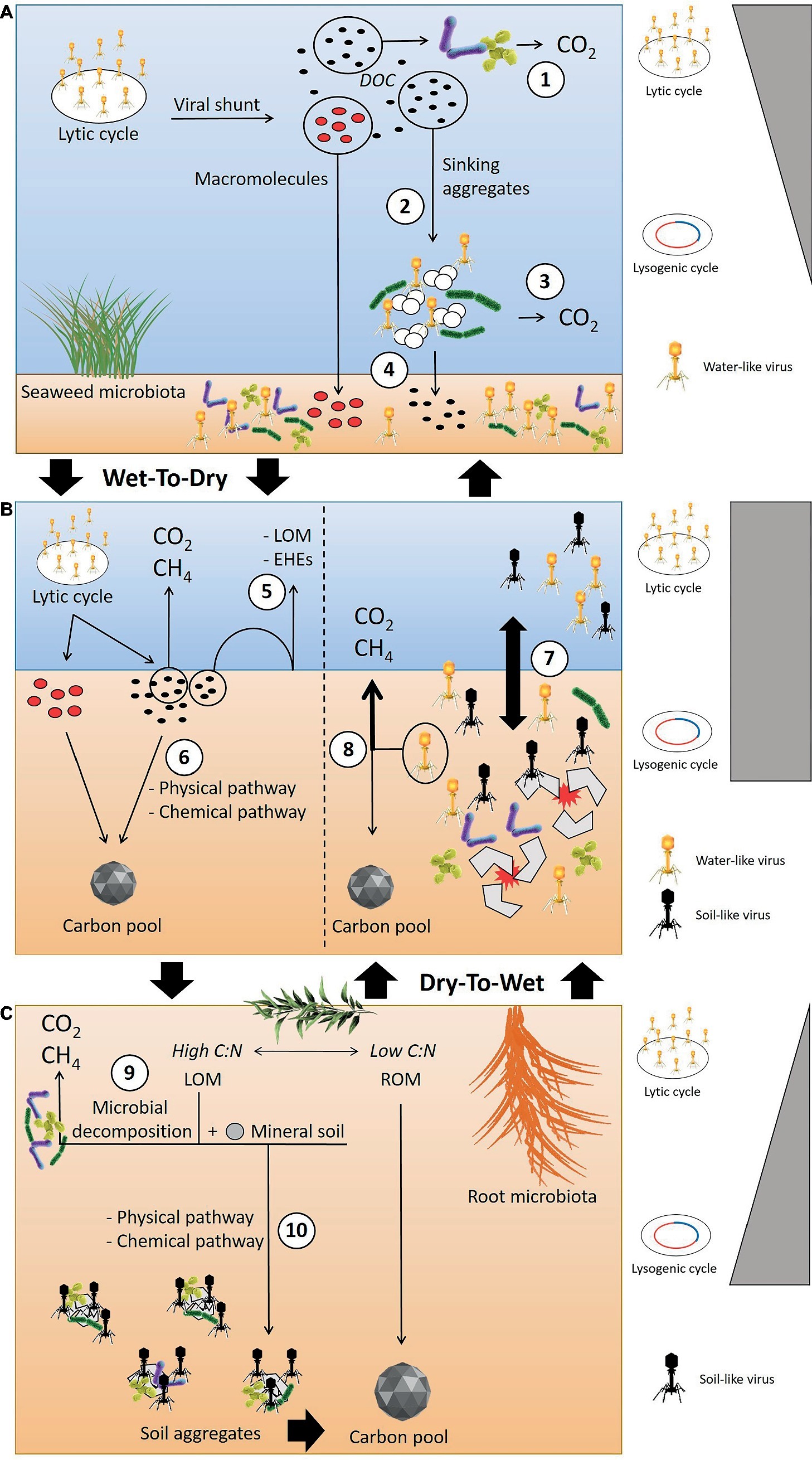
Figure 2. Conceptual model outlining the hypothesized viral infection and organic matter dynamics when in transition from wet-to-dry and dry-to-wet conditions. (A) In the water column, the lytic cycle is recognized as the dominant infection strategy. The viral shunt results in the release of DOC that can be directly decomposed by microbes (1) or might coagulate into sinking aggregates (2). However, the high decomposition activities of the microbiota that colonize the sinking aggregates likely degrade the aggregates, leading to the production of GHG (3). The macromolecules released after the viral lysis can be recalcitrant and bypass microbial consumption. These compounds can reach the soil surface, along with labile organic matter escaping microbial attack within the sinking aggregates (4). (B) During a wet-to-dry transition, the reduction of the water column and the soil-water interface also reduces the diffusion of labile carbon organic matter (LOM) and extracellular hydrolytic enzymes (EHEs) across the water-soil interface (5), leading to increased remineralization of labile matter within the aerobic layer of the soil. Changes in physiochemical conditions triggered by the occurrence of wet-to-dry cycles can also facilitate the transformation of labile carbon into a stable form through the interaction with mineral soil (6). During dry-to-wet transitions, e.g. flooding events, the breakdown of soil aggregates and presence of the porewater matrix promotes virus-host encounters and the shift from soil-like to water-like viruses (7). This shift could affect the direction of carbon flow, possibly increasing GHG emissions (8). (C) In the soil, the fate of LOM can undergo two pathways: (9) microbial decomposition through aerobic or anaerobic reactions, (10) transformation into soil aggregates via physical or chemical reactions with mineral soil, i.e., transformation into recalcitrant organic matter (ROM). The promotion of viral infections and lysogeny through aggregate formation has been hypothesized as the dominant infection mechanism in soil. (Vectors used under license from Shutterstock.com).
How Do Abiotic Factors Affect Virus-Prokaryote Interactions, and Therefore the Carbon Fate, in Freshwater Wetlands?
Alternative to the concept that microbes are the gatekeepers of the carbon cycle, Bridgham et al. (2013b) suggested that microbial communities and their activity are “passive characters” in wetland ecosystems. Instead, the main controls over the biogeochemical cycles are the physiochemical factors, such as dry/wet cycles, hydrology, temperature, and pH. Specifically, shifts in abiotic conditions related to climate change have the potential to enact changes in the microbial members of wetland soils. For instance, a low pH can inhibit the microbial respiration (Benner et al., 1985; Kok et al., 1990; Ye et al., 2012). In Arctic freshwater wetlands, the permafrost thaw progression seems to trigger the GHG production (especially methane) and microbial decomposition rates. By affecting vegetation and hydrology, the permafrost loss causes the increment of nitrogen and labile carbon availability and the transition from hydrogenotrophic to acetoclastic methane production (Hodgkins et al., 2014; McCalley et al., 2014). The virus-host abundance ratios may also be influenced by the increasing thaw and suggest a lineage-specific response to habitat changing (Emerson et al., 2018). In addition, the hydrology in freshwater wetlands can affect the horizontal carbon transport and its dispersion through water flow. In this way, carbon, as well as viral or microbial communities, can be redistributed and accumulated in adjacent systems (Jackson and Jackson, 2008).
Temperature is likely to be a major control in anaerobic biogeochemical processes, such as methanogenesis and denitrification (Gutknecht et al., 2006). In laboratory experiments, it has been demonstrated that microbial sensitivity to temperature might be strongly dependent on the substrate lability (Frey et al., 2013). As we mentioned above, the microbial growth efficiency is a pivotal factor in carbon dynamics, since it determines the journey the carbon will undertake when introduced into the microbial cell. The microbial growth efficiency of relatively more recalcitrant organic matter seems to be inversely correlated to temperature, which may be related to the depletion of labile compounds observed in warmer conditions (Frey et al., 2013). Soil respiration appears to be stimulated by a short-term temperature increases but not by a long-term exposure at higher temperature, suggesting a strong metabolic adaptation that might be explained by the limitation of labile carbon availability (Heimann and Reichstein, 2008; Frey et al., 2013). High soil respiration rates lead to the improvement of the decomposition efficiency that should contribute to an increase in the organic matter remineralization. However, the actual constant organic matter usage with increasing temperature might be justified with a faster carbon consumption and with an increase of new microbial biomass production. This microbial biomass is counterbalanced by the increase of microbial turnover observed at high temperatures and potentially driven by a positive influence of the temperature on grazers (Hagerty et al., 2014) and, possibly, viruses. The positive influence of temperature on viruses might result in high infection rates. The viral lysis of the host cell might cause an initial release of carbon dioxide that added to the carbon dioxide released via the prokaryotic respiration pathway, will increase the total amount of greenhouse gas emissions, and will support the infection mechanism hypotheses presented in Question 1.
While we have an understanding of the physiochemical influences on prokaryotes, we know very little about the effects of different abiotic variables on the viral production, infection strategy, or the virus-host relationship in freshwater wetlands, in vitro or in situ (Figure 3; Middelboe et al., 2008). Some evidence suggests that the viral component might also be a crucial driver in altering the microbial sensitivity to different abiotic factors through the exchange of genetic materials instigated by the unexpected lysis (Boon et al., 2014). In a freshwater microcosm experiment, it has been demonstrated that high levels of nutrients increased the prokaryotic abundance and activity as well as viral production and induced lysogenic cycles by enhancing host cell growth (Mei and Danovaro, 2004; Pradeep Ram and Sime-Ngando, 2008). The effects of temperature changes on viral infection are contradictory, although in general, the lysogenic cycle seems to be induced by temperature increases. On the other hand, stressing the bacterial cell with rapid environmental changes (i.e., temperature, nutrients, and oxygen concentrations) may trigger higher viral replication and the viruses abandoning the host cell before it dies (Boon et al., 2014). In this scenario, failure to complete the lysogenic cycle may cause a reverse to the lytic cycle, leading to increased microbial growth efficiency and thus potentially enhanced carbon utilization.
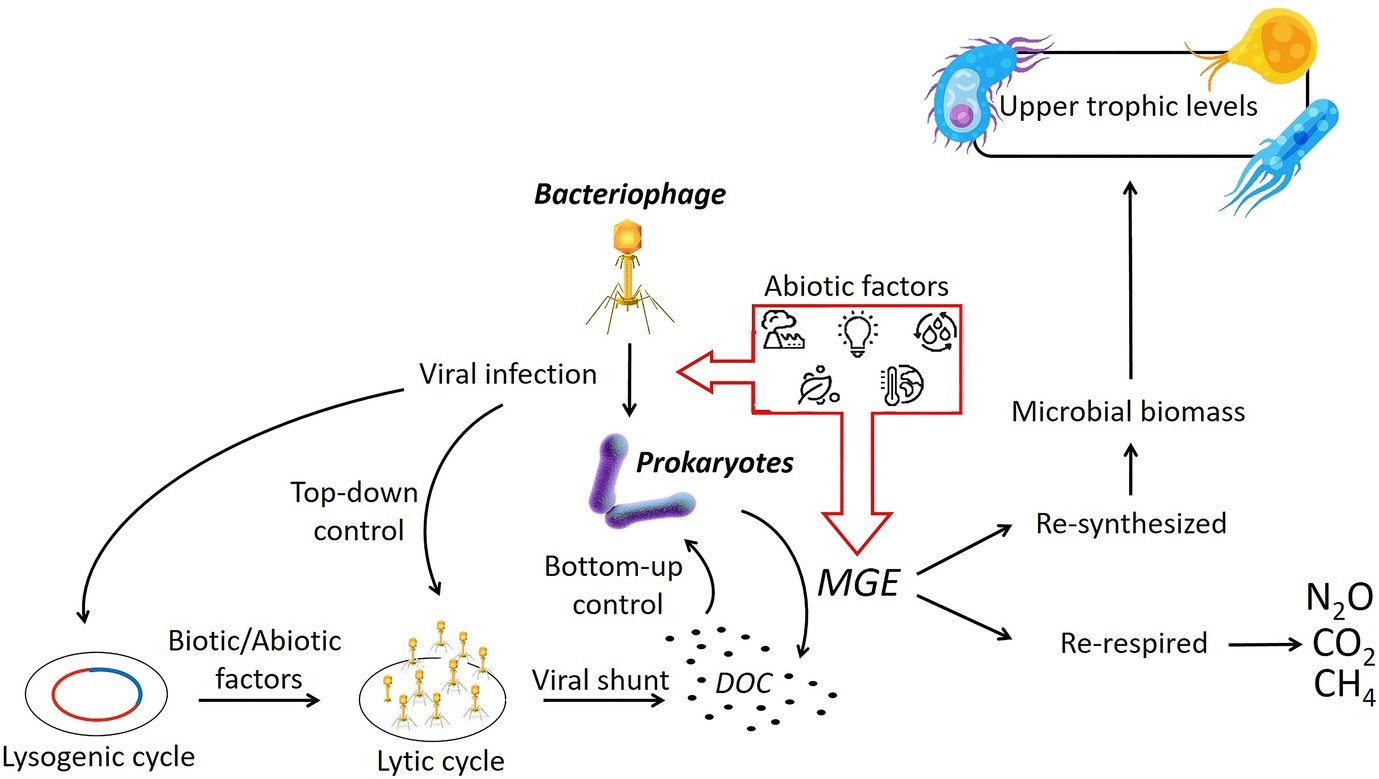
Figure 3. Shifts in abiotic conditions have the potential to enact changes in the viral infection strategy and prokaryotic MGE. The microbial growth efficiency (MGE) defines the proportion of DOC that is being assimilated and then re-synthetized or re-respired with subsequent consequences on the production of carbon emissions. (Vectors used under license from Shutterstock.com).
In light of this evidence, we hypothesize a combined model, whereby the primary factor influencing the virus-prokaryotic dynamic is the abiotic factors actively controlling growth and metabolism, followed by the influence of viral replication mechanisms. A microcosm approach to this question would help to better understand how each abiotic factor separately and collectively influences viral infection and the carbon remineralization activity of infected prokaryotes. Moreover, a controlled approach would allow us to create multiple scenarios that could result in new hypotheses for targets and management strategies to face a changing environment.
Summary
The fundamental role of prokaryotes in regulating carbon sequestration and greenhouse gas (GHG) emissions in wetlands is widely recognized (Bodelier and Dedysh, 2013). However, wide lens of the identity of the microbes involved, their interactions, potential mortality from viruses and susceptibility to external perturbations (i.e., temperature, wet/dry cycles, and hydrology) and how these components work collectively are still considered a “black box” (Brussaard et al., 2008; Woodcroft et al., 2018). To date, research investigating the microbial communities and functions involved in wetland carbon cycling has primarily focused on the prokaryotic component (bacteria and archaea; Boon et al., 2014). In comparison, the role of viruses has been vastly under-studied, although the ecological virus-prokaryote relationship could be fundamentally to the wetland carbon cycling.
As wetlands are important for biodiversity, ecosystem function, and services, management plans have focused on the restoration of a wetland’s original condition prior to disturbance (e.g., repurposing the land from grazing or cropping and/or changing the hydrology for agricultural or development; Carnell et al., 2018). The restoration of the hydrology and ecosystem functionality has its foundation with the aim of rehabilitating the microbial and biogeochemical functions. If wetland restoration aims to improve carbon sequestration, we propose that management actions will need to address environmental variables that trigger viral infectivity. The factors controlling viral production, viral infection rate, and indeed viral involvement in biogeochemical cycles in these ecosystems will be a key management lever. We also expect that an effective management scenario of freshwater wetlands could include targets for viruses and viral infections based on predicted global change scenarios. While ambitious, the ultimate aim would be to create a functional loop where the positive feedbacks promote carbon remineralization and greenhouse gas emission within the context in the range of abiotic and biotic variables that influence the microbial black box.
Data Availability
All datasets generated for this study are included in the manuscript and/or the Supplementary Files.
Author Contributions
GB contributed to manuscript idea and visualization, conducted an investigation process, and wrote the original draft preparation. ST-T, PC, and PM wrote, reviewed, and edited the manuscript and supervised the study.
Funding
We thank the Australian Communities Foundation (Wanless Family Fund) and an Australian Research Council Linkage Project (LP160100242) for funding that made this research possible.
Conflict of Interest Statement
The authors declare that the research was conducted in the absence of any commercial or financial relationships that could be construed as a potential conflict of interest.
Supplementary Material
The Supplementary Material for this article can be found online at: https://www.frontiersin.org/articles/10.3389/fmicb.2019.01962/full#supplementary-material
References
Ackermann, H. W., and DuBow, M. S. (eds.) (1987). “Viruses of prokaryotes” in General Properties of Bacteriophages. Vol. 1 (Boca Raton, CA, USA: CRC Press), 202.
Adriaenssens, E. M., Zyl, L.Van, Maayer, P. De, Rubagotti, E., Rybicki, E., Tuffin, M., et al. (2015). Metagenomic analysis of the viral community in Namib Desert hypoliths. Environ. Microbiol. 17, 480–495. doi: 10.1111/1462-2920.12528
Alexyuk, V., Alexyuk, M., Bogoyavlenskiy, P., Komarnytsky, A., Mirzadinov, S., Zhumanov, R., et al. (2016). Diversity of viral photosystem-II psb A genes in the largest channel reservoir of Kazakhstan. Res. J. Pharm. Biol. Chem. Sci. 7, 2674–2680. ISSN: 09758585.
Arnosti, C. (2011). Microbial extracellular enzymes and the marine carbon cycle. Annu. Rev. Mar. Sci. 3, 401–425. doi: 10.1146/annurev-marine-120709-142731
Azam, F., Fenchel, T., Field, J. G., Gray, J. S., Meyer-Reil, L. A., and Thingstad, F. (1983). The ecological role of water-column microbes in the sea. Mar. Ecol. Prog. Ser. 10, 257–263. doi: 10.3354/meps010257
Basemer, K. (2015). Biodiversity, community structure and function of biofilms in stream ecosystems. Res. Microbiol. 166, 774–781. doi: 10.1016/j.resmic.2015.05.006
Benner, R., and Biddanda, B. (1998). Photochemical transformations of surface and deep marine dissolved organic matter: effects on bacterial growth. Limnol. Oceanogr. 43, 1373–1378. doi: 10.4319/lo.1998.43.6.1373
Benner, R., Moran, M. A., and Hodson, R. E. (1985). Effects of pH and plant source on lignocellulose biodegradation rates in two wetland ecosystems, the Okefenokee Swamp and a Georgia salt marsh. Limnol. Oceanogr. 30, 489–499. doi: 10.4319/lo.1985.30.3.0489
Bochdansky, A. B., Puskaric, S., and Herndi, G. J. (1995). Influence of zooplankton grazing on free dissolved enzymes in the sea. Mar. Ecol. Prog. Ser. 121, 53–63. doi: 10.3354/meps121053
Bodelier, P. L. E., and Dedysh, S. N. (2013). Microbiology of wetlands. Front. Microbiol. 4, 2–5. doi: 10.3389/fmicb.2013.00079
Boon, P. I., Pollard, P. C., and Ryder, D. (2014). “Wetland microbial ecology and biogeochemistry” in Ecology of freshwater and estuarine wetlands. eds. D. P. Batzer and R. R. Sharitz (Oakland, California: University of California Press).
Bridgham, S. D., Cadillo-Quiroz, H., Keller, J. K., and Zhuang, Q. (2013b). Methane emissions from wetlands: biogeochemical, microbial, and modeling perspectives from local to global scales. Glob. Chang. Biol. 19, 1325–1346. doi: 10.1111/gcb.12131
Bridgham, S. D., Ye, R., DeLaune, R. D., Reddy, K. R., Richardson, C. J., and Megonigal, J. P. (2013a). “Carbon dynamics and ecosystem processes” in Organic matter mineralization and decomposition. 185–202.
Brum, J. R., Hurwitz, B. L., Schofield, O., Ducklow, H. W., and Sullivan, M. B. (2016). Seasonal time bombs: dominant temperate viruses affect Southern Ocean microbial dynamics. ISME J. 10, 437–449. doi: 10.1038/ismej.2015.125
Brum, J. R., and Sullivan, M. B. (2015). Rising to the challenge: accelerated pace of discovery transforms marine virology. Nat. Publ. Group 13, 147–159. doi: 10.1038/nrmicro3404
Brum, J. R., Sullivan, M. B., Ignacio-espinoza, J. C., Roux, S., Doulcier, G., Acinas, S. G., et al. (2015). Patterns and ecological drivers of ocean viral communities. Science 348:1261498. doi: 10.1126/science.1261498
Brussaard, C. P. D., Wilhelm, S. W., Thingstad, F., Weinbauer, M. G., Bratbak, G., Heldal, M., et al. (2008). Global-scale processes with a nanoscale drive: the role of marine viruses. ISME J. 2, 575–578. doi: 10.1038/ismej.2008.31
Burns, A., and Ryder, D. S. (2001). Response of bacterial extracellular enzymes to inundation of floodplain sediments. Freshw. Biol. 46, 1299–1307. doi: 10.1046/j.1365-2427.2001.00750.x
Carnell, P. E., Windecker, S. M., Brenker, M., Baldock, J., Masque, P., Brunt, K., et al. (2018). Carbon stocks, sequestration, and emissions of wetlands in south eastern Australia. Glob. Chang. Biol. 24, 4173–4184. doi: 10.1111/gcb.14319
Chen, Y., Golding, I., Sawai, S., Guo, L., and Cox, E. C. (2005). Population fitness and the regulation of Escherichia coli genes by bacterial viruses. PLoS Biol. 3:e229. doi: 10.1371/journal.pbio.0030229
Corinaldesi, C., Dell’Anno, A., and Danovaro, R. (2012). Viral infections stimulate the metabolism and shape prokaryotic assemblages in submarine mud volcanoes. ISME J. 6, 1250–1259. doi: 10.1038/ismej.2011.185
Cowardin, L. M., Carter, V., Golet, F. C., and LaRoe Edward, T. (1979). Classification of wetlands and deepwater habitats of the United States. United States fish and wildlife service (Washington, DC), 131.
Dalcin Martins, P., Danczak, R. E., Roux, S., Frank, J., Borton, M. A., Wolfe, R. A., et al. (2018). Viral and metabolic controls on high rates of microbial sulfur and carbon cycling in wetland ecosystems. Microbiome 6:138. doi: 10.1186/s40168-018-0522-4
Danovaro, R., Corinaldesi, C., Filippini, M., Fischer, U. R., Gessner, M. O., Jacquet, S., et al. (2008a). Viriobenthos in freshwater and marine sediments: a review. Freshw. Biol. 53, 1186–1213. doi: 10.1111/j.1365-2427.2008.01961.x
Danovaro, R., Dell’Anno, A., Corinaldesi, C., Magagnini, M., Noble, R., Tamburini, C., et al. (2008b). Major viral impact on the functioning of benthic deep-sea ecosystems. Nature 454, 1084–1087. doi: 10.1038/nature07268
Davidson, E. A., and Janssens, I. A. (2006). Temperature sensitivity of soil carbon decomposition and feedbacks to climate change. Nature 440, 165–173. doi: 10.1038/nature04514
del Giorgio, P. A., and Williams, P. J. L. (2005). Respiration in aquatic ecosystems. (Oxford: Oxford University Press).
Dise, N. B. (2009). Peatland response to global change. Science 326, 810–811. doi: 10.1126/science.1174268
Ducklow, H. W., Purdie, D. A., Williams, P. J. L. B., and Davies, J. M. (1986). Bacterioplankton: a sink for carbon in a coastal marine plankton community. Science 232, 863–867.
Emerson, J. B., Roux, S., Brum, J. R., Bolduc, B., Woodcroft, B. J., Jang, H. B., et al. (2018). Host-linked soil viral ecology along a permafrost thaw gradient. Nat. Microbiol. 3, 870–880. doi: 10.1038/s41564-018-0190-y
Esteban, D. J., Hysa, B., and Bartow-McKenney, C. (2015). Temporal and spatial distribution of the microbial community of winogradsky columns. PLoS One 10, 1–21. doi: 10.1371/journal.pone.0134588
Fancello, L., Trape, S., Robert, C., Boyer, M., Popgeorgiev, N., Raoult, D., et al. (2013). Viruses in the desert: a metagenomic survey of viral communities in four perennial ponds of the Mauritanian Sahara. ISME J. 7, 359–369. doi: 10.1038/ismej.2012.101
Farnell-Jackson, E. A., and Ward, A. K. (2003). Seasonal patterns of viruses, bacteria and dissolved organic carbon in a riverine wetland. Freshw. Biol. 48, 841–851. doi: 10.1046/j.1365-2427.2003.01052.x
Fierer, N., Leff, J. W., Adams, B. J., Nielsen, U. N., Bates, S. T., Lauber, C. L., et al. (2012). Cross-biome metagenomic analyses of soil microbial communities and their functional attributes. Proc. Natl. Acad. Sci. USA 109, 21390–21395. doi: 10.1073/pnas.1215210110
Fischer, U. R., Wieltschnig, C., Kirschner, A. K. T., and Velimirov, B. (2006). Contribution of virus-induced lysis and protozoan grazing to benthic bacterial mortality estimated simultaneously in microcosms. Environ. Microbiol. 8, 1394–1407. doi: 10.1111/j.1462-2920.2006.01032.x
Frey, S. D., Lee, J., Melillo, J. M., and Six, J. (2013). The temperature response of soil microbial efficiency and its feedback to climate. Nat. Clim. Chang. 3, 395–398. doi: 10.1038/nclimate1796
Fuhrman, J. A. (1999). Marine viruses and their biogeochemica and ecological effects. Nature 399, 541–548. doi: 10.1038/21119
Ghosh, D., Roy, K., Williamson, K. E., White, D. C., Wommack, K. E., Sublette, K. L., et al. (2008). Prevalence of lysogeny among soil bacteria and presence of 16S rRNA and trzN genes in viral-community DNA. Appl. Environ. Microbiol. 74, 495–502. doi: 10.1128/AEM.01435-07
Guidi, L., Chaffron, S., Bittner, L., Eveillard, D., Larhlimi, A., Roux, S., et al. (2016). Plankton networks driving carbon export in the oligotrophic ocean. Nature 532, 465–470. doi: 10.1038/nature16942
Gutknecht, J. L. M., Goodman, R. M., and Balser, T. C. (2006). Linking soil process and microbial ecology in freshwater wetland ecosystems. Plant Soil 289, 17–34. doi: 10.1007/s11104-006-9105-4
Hagerty, S. B., Van Groenigen, K. J., Allison, S. D., Hungate, B. A., Schwartz, E., Koch, G. W., et al. (2014). Accelerated microbial turnover but constant growth efficiency with warming in soil. Nat. Clim. Chang. 4, 903–906. doi: 10.1038/nclimate2361
Heimann, M., and Reichstein, M. (2008). Terrestrial ecosystsem carbon dynamics and climate feedbacks. Nature 451, 289–292. doi: 10.1038/nature06591
Hodgkins, S. B., Tfaily, M. M., McCalley, C. K., Logan, T. A., Crill, P. M., Saleska, S. R., et al. (2014). Changes in peat chemistry associated with permafrost thaw increase greenhouse gas production. Proc. Natl. Acad. Sci. USA 111, 5819–5824. doi: 10.1073/pnas.1314641111
Hurwitz, B. L., Hallam, S. J., and Sullivan, M. B. (2013). Metabolic reprogramming by viruses in the sunlit and dark oceans. Genome Biol. 14:R123. doi: 10.1186/gb-2013-14-11-r123
Jackson, E. F., and Jackson, C. R. (2008). Viruses in wetland ecosystems. Freshw. Biol. 53, 1214–1227. doi: 10.1111/j.1365-2427.2007.01929.x
Jiao, N., Herndl, G. J., Hansell, D. A., Benner, R., Kattner, G., Wilhelm, S. W., et al. (2010). Microbial production of recalcitrant dissolved organic matter: long-term carbon storage in the global ocean. Nat. Rev. Microbiol. 8, 593–599. doi: 10.1038/nrmicro2386
Karner, M., Ferrier-Pages, C., and Rassoulzadegan, F. (1994). Phagotrophic nanoflagellates contribute to occurrence of α-glucosidase and aminopeptidase in marine environments. Mar. Ecol. Prog. Ser. 114, 237–244. doi: 10.3354/meps114237
Kimura, M., Zhong-Jun, J., Nakayama, N., and Susumo, A. (2008). Ecology of viruses in soils: past, present and future perspectives. Soil Sci. Plant Nutr. 54, 1–32. doi: 10.1111/j.1747-0765.2007.00197.x
Kirwan, M. L., and Mudd, S. M. (2012). Response of salt-marsh carbon accumulation to climate change. Nature 489, 550–553. doi: 10.1038/nature11440
Knowles, B., Silveira, C. B., Bailey, B. A., Barott, K., Cantu, V. A., Cobian-Guëmes, A. G., et al. (2016). Lytic to temperate switching of viral communities. Nature 531, 466–470. doi: 10.1038/nature17193
Kok, C. J., Meesters, H. W. G., and Kempers, A. J. (1990). Decomposition rate, chemical composition and nutrient cycling of Nymphea alba L. floating leaf blade detritus as influenced by pH, alkalinity and aluminum in laboratory experiments. Aquat. Bot. 37, 215–227. doi: 10.1016/0304-3770(90)90071-R
Kyle, J. E., and Grant, F. (2013). Geochemistry of virus-prokaryote interactions in freshwater and acid mine drainage environments, Ontario, Canada. Geomicrobiol J. 30, 769–778. doi: 10.1080/01490451.2013.770978
Leroy, M., Prigent, M., Dutertre, M., Confalonieri, F., and Dubow, M. (2008). Bacteriophage morphotype and genome diversity in Seine River sediment. Freshw. Biol. 53, 1176–1185. doi: 10.1111/j.1365-2427.2008.01985.x
Limpens, J., Berendse, F., Blodau, C., Canadell, J. G., Free-man, C., Holden, J., et al. (2008). Peatlands and the carbon cycle: from local processes to global implications— a synthesis. Biogeosciences 5, 1475–1491. doi: 10.5194/bg-5-1475-2008
Mann, N. H., Cook, A., Millard, A., Bailey, S., and Clokie, M. (2003). Marine ecosystems: bacterial photosynthesis genes in a virus. Nature 424:741. doi: 10.1038/424741a
Manzoni, S., Taylor, P., Richter, A., Porporato, A., and Agren, G. I. (2012). Environmental and stoichiometric controls on microbial carbon-use efficiency in soils. New Phytol. 196, 79–91. doi: 10.1111/j.1469-8137.2012.04225.x
McCalley, C. K., Woodcroft, B. J., Hodgkins, S. B., Wehr, R. A., Kim, E. H., Mondav, R., et al. (2014). Methane dynamics regulated by microbial community response to permafrost thaw. Nature 514, 478–481. doi: 10.1038/nature13798
Mei, M. L., and Danovaro, R. (2004). Virus production and life strategies in aquatic sediments. Limnol. Oceanogr. 49, 459–470. doi: 10.4319/lo.2004.49.2.0459
Middelboe, M., Jacquet, S., and Weinbauer, M. (2008). Viruses in freshwater ecosystems: an introduction to the exploration of viruses in new aquatic habitats. Freshw. Biol. 53, 1069–1075. doi: 10.1111/j.1365-2427.2008.02014.x
Mostajir, B., Amblard, C., Buffan-Dubau, E., De Wit, R., Lensi, R., and Sime-Ngando, T. (2015). “Microbial food webs in aquatic and terrestrial ecosystems” in Environmental microbiology: Fundamentals and applications. eds. J. C. Bertrand, P. Caumette, P. Lebaron, R. Matheron, P. Normand, and T. Sime-Ngando (Dordrecht: Springer), 485–509.
Motlagh, A. M., Bhattacharjee, A. S., Coutinho, F. H., Dutilh, B. E., Casjens, S. R., and Goel, R. K. (2017). Insights of phage-host interaction in hypersaline ecosystem through metagenomics analyses. Front. Microbiol. 8, 1–15. doi: 10.3389/fmicb.2017.00352
Neori Amir, A. M. (2017). The functioning of rhizosphere biota in wetlands – a review. Wetlands 37, 615–633. doi: 10.1007/s13157-016-0757-4
Paul, J. H. (2008). Prophages in marine bacteria: dangerous molecular time bombs or the key to survival in the seas? ISME J. 2, 579–589. doi: 10.1038/ismej.2008.35
Pradeep Ram, A. S., and Sime-Ngando, T. (2008). Functional responses of prokaryotes and viruses to grazer effects and nutrient additions in freshwater microcosms. ISME J. 2, 498–509. doi: 10.1038/ismej.2008.15
Pradeep Ram, A. S., and Sime-Ngando, T. (2010). Resources drive trade-off between viral lifestyles in the plankton: evidence from freshwater microbial microcosms. Environ. Microbiol. 12, 467–479. doi: 10.1111/j.1462-2920.2009.02088.x
Pratama, A. A., and van Elsas, J. D. (2018). The ‘neglected’ soil virome – potential role and impact. Trends Microbiol. 26, 649–662. doi: 10.1016/j.tim.2017.12.004
Qin, K., Ji, X., Zhang, C., Ding, Y., Kuang, A., Zhang, S., et al. (2017). Isolation and characterization of wetland VSW-3, a novel lytic cold-active bacteriophage of Pseudomonas fluorescens. Can. J. Microbiol. 63, 110–118. doi: 10.1139/cjm-2016-0368
Robinson, C. (2008). “Heterotrophic bacterial respiration” in Microbial Ecology of the Oceans. 2nd Edn. ed. D. L. Kirchman (New York: John Wiley & Sons Inc.), 299–334.
Rooks, D. J., Smith, D. L., McDonald, J. E., Woodward, M. J., McCarthy, A. J., and Allison, H. E. (2010). 454-pyrosequencing: a molecular battiscope for freshwater viral ecology. Gene 1, 210–226. doi: 10.3390/genes1020210
Roux, S., Brum, J. R., Dutilh, B. E., Sunagawa, S., Duhaime, M. B., Loy, A., et al. (2016). Ecogenomics and potential biogeochemical impacts of globally abundant ocean viruses. Nature 537, 689–693. doi: 10.1038/nature19366
Saxton, M. A., Naqvi, N. S., Rahman, F., Thompson, C. P., Chambers, R. M., Kaste, J. M., et al. (2016). Site-specific environmental factors control bacterial and viral diversity in stormwater retention ponds. Aquat. Microb. Ecol. 77, 23–36. doi: 10.3354/ame01786
Schimel, J. (2013). Soil carbon: microbes and global carbon. Nat. Clim. Chang. 3, 867–868. doi: 10.1038/nclimate2015
Shaw, S. P., and Fredine, C. G. (1956). “Wetlands of the United States, their extent, and their value for waterfowl and other wildlife” in United States department of the interior, fish and wildlife service, circular. Vol. 39 (Washington, DC), 67.
Sherr, B. F., Sherr, E. B., and Fallon, R. D. (1987). Use of monodispersed, fluorescently labeled bacteria to estimate in situ protozoan bacterivory. Appl. Environ. Microbiol. 53, 958–965.
Sherr, B. F., Sherr, E. B., and Rassoulzadegan, F. (1988). Rates of digestion of bacteria by marine phagotrophic protozoa: temperature dependence. Appl. Environ. Microbiol. 54, 1091–1095.
Sieradzki, E. T., Ignacio-Espinoza, J. C., Needham, D. M., Fichot, E. B., and Fuhrman, J. A. (2019). Dynamic marine viral infections and major contribution to photosynthetic processes shown by spatiotemporal picoplankton metatranscriptomes. Nat. Commun. 10:1169. doi: 10.1038/s41467-019-09106-z
Sterner, R. W., and Elser, J. J. (2002). Ecological stoichiometry: The biology of elements from molecules to the biosphere. (NJ, USA & Oxford, UK: Princeton University press).
Strom, S. L., Benner, R., Ziegler, S., and Dagg, M. J. (1997). Planktonic grazers are a potentially important source of marine dissolved organic carbon. Limnol. Oceanogr. 42, 1364–1374. doi: 10.4319/lo.1997.42.6.1364
Stroynov, Y. V., and Philippov, D. A. (2017). Bacterio- and virioplankton in water bodies of a raised bog (Vologda Oblast, Russia). Inland Water Biol. 10, 37–43. doi: 10.1134/S1995082917010175
Sullivan, M. B., Coleman, M. L., Weigele, P., Rohwer, F., and Chisholm, S. W. (2005). Three Prochlorococcus cyanophage genomes: signature features and ecological interpretations. PLoS Biol. 3:e144. doi: 10.1371/journal.pbio.0030144
Suttle, C. A. (2007). Marine viruses - major players in the global ecosystem. Nat. Rev. Microbiol. 5, 801–812. doi: 10.1038/nrmicro1750
Thomas, R., Berdjeb, L., Sime-Ngando, T., and Jacquet, S. (2011). Viral abundance, production, decay rates and life strategies (lysogeny versus lysis) in Lake Bourget (France). Environ. Microbiol. 13, 616–630. doi: 10.1111/j.1462-2920.2010.02364.x
Trubl, G., Jang, H. B., Roux, S., Emerson, J. B., Solonenko, N., Vik, D. R., et al. (2018). Soil viruses are underexplored players in ecosystem carbon processing. MSystems 3, 1–21. doi: 10.1128/msystems.00076-18
Weinbauer, M. G. (2004). Ecology of prokaryotic viruses. FEMS Microbiol. Rev. 28, 127–181. doi: 10.1016/j.femsre.2003.08.001
Weitz, J. S., Beckett, S. J., Brum, J. R., Cael, B. B., and Dushoff, J. (2016). Lysis, lysogeny, and virus-microbe ratios. Nature 549, 3–7. doi: 10.1038/nature23295
Wieder, W. R., Bonan, G. B., and Allison, S. D. (2013). Global soil projections are improved by modelling microbial processes. Nat. Clim. Chang. 3, 909–912. doi: 10.1038/nclimate1951
Wilhelm, S. W., and Matteson, A. R. (2008). Freshwater and marine virioplankton: a brief overview of commonalities and differences. Freshw. Biol. 53, 1076–1089. doi: 10.1111/j.1365-2427.2008.01980.x
Williamson, K. E., Fuhrmann, J. J., Wommack, K. E., and Radosevich, M. (2017). Viruses in soil ecosystems: an unknown quantity within an unexplored territory. Ann. Rev. Virol. 4, 201–219. doi: 10.1146/annurev-virology-101416-041639
Wilson, W. H., and Mann, N. H. (1997). Lysogenic and lytic production in marine microbial communities. Aquat. Microb. Ecol. 13, 95–100. doi: 10.3354/ame013095
Wommack, K. E., and Colwell, R. R. (2000). Virioplankton: viruses in aquatic ecosystems. Microbiol. Mol. Biol. Rev. 64, 69–114. doi: 10.1128/MMBR.64.1.69-114.2000
Woodcroft, B. J., Singleton, C. M., Boyd, J. A., Evans, P. N., Emerson, J. B., Zayed, A. A. F., et al. (2018). Genome-centric view of carbon processing in thawing permafrost. Nature 560, 49–54. doi: 10.1038/s41586-018-0338-1
Ye, R., Jin, Q., Bohannan, B., Keller, J. K., McAllister, S. A., and Bridgham, S. D. (2012). pH controls over anaerobic carbon mineralization, the efficiency of methane production, and methanogenic pathways in peatlands across an ombrotrophic-minerotrophic gradient. Soil Biol. Biochem. 54, 36–47. doi: 10.1016/j.soilbio.2012.05.015
Zheng, C., Wang, G., Liu, J., Song, C., Gao, H., and Liu, X. (2013). Characterization of the major capsid genes (g23) of T4-type bacteriophages in the wetlands of Northeast China. Microb. Ecol. 65, 616–625. doi: 10.1007/s00248-012-0158-z
Glossary
Keywords: wetland, freshwater, virus, prokaryote, infection, biogeochemical cycles, carbon dioxide, methane
Citation: Bonetti G, Trevathan-Tackett SM, Carnell PE and Macreadie PI (2019) Implication of Viral Infections for Greenhouse Gas Dynamics in Freshwater Wetlands: Challenges and Perspectives. Front. Microbiol. 10:1962. doi: 10.3389/fmicb.2019.01962
Edited by:
Rima Franklin, Virginia Commonwealth University, United StatesReviewed by:
Evelyn Jackson, University of Mississippi, United StatesJoanne B. Emerson, University of California, Davis, United States
Copyright © 2019 Bonetti, Trevathan-Tackett, Carnell and Macreadie. This is an open-access article distributed under the terms of the Creative Commons Attribution License (CC BY). The use, distribution or reproduction in other forums is permitted, provided the original author(s) and the copyright owner(s) are credited and that the original publication in this journal is cited, in accordance with accepted academic practice. No use, distribution or reproduction is permitted which does not comply with these terms.
*Correspondence: Giuditta Bonetti, Z2JvbmV0dGlAZGVha2luLmVkdS5hdQ==