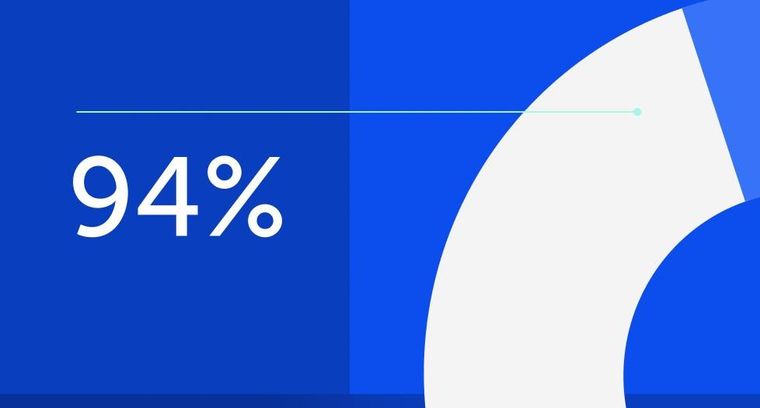
94% of researchers rate our articles as excellent or good
Learn more about the work of our research integrity team to safeguard the quality of each article we publish.
Find out more
ORIGINAL RESEARCH article
Front. Microbiol., 20 August 2019
Sec. Microbial Physiology and Metabolism
Volume 10 - 2019 | https://doi.org/10.3389/fmicb.2019.01926
This article is part of the Research TopicInterdisciplinary Approaches to Face New Challenges on the N-Cycle View all 5 articles
Denitrification in the soybean endosymbiont Bradyrhizobium diazoefficiens is controlled by a complex regulatory network composed of two hierarchical cascades, FixLJ-FixK2-NnrR and RegSR-NifA. In the former cascade, the CRP/FNR-type transcription factors FixK2 and NnrR exert disparate control on expression of core denitrifying systems encoded by napEDABC, nirK, norCBQD, and nosRZDFYLX genes in response to microoxia and nitrogen oxides, respectively. To identify additional genes controlled by NnrR and involved in the denitrification process in B. diazoefficiens, we compared the transcriptional profile of an nnrR mutant with that of the wild type, both grown under anoxic denitrifying conditions. This approach revealed more than 170 genes were simultaneously induced in the wild type and under the positive control of NnrR. Among them, we found the cycA gene which codes for the c550 soluble cytochrome (CycA), previously identified as an intermediate electron donor between the bc1 complex and the denitrifying nitrite reductase NirK. Here, we demonstrated that CycA is also required for nitrous oxide reductase activity. However, mutation in cycA neither affected nosZ gene expression nor NosZ protein steady-state levels. Furthermore, cycA, nnrR and its proximal divergently oriented nnrS gene, are direct targets for FixK2 as determined by in vitro transcription activation assays. The dependence of cycA expression on FixK2 and NnrR in anoxic denitrifying conditions was validated at transcriptional level, determined by quantitative reverse transcription PCR, and at the level of protein by performing heme c-staining of soluble cytochromes. Thus, this study expands the regulon of NnrR and demonstrates the role of CycA in the activity of the nitrous oxide reductase, the key enzyme for nitrous oxide mitigation.
Denitrification is a form of bacterial respiration that occurs under oxygen limitation, in which the reduction of nitrate (NO3–) or nitrite (NO2–) to dinitrogen (N2) provides cellular energy. During this process, nitric oxide (NO) and nitrous oxide (N2O) are generated as free-intermediates (Zumft, 1997). Denitrification consists of four sequential enzymatic reactions catalyzed by nitrate-, nitrite-, nitric oxide-, and nitrous oxide reductases, encoded by nar/nap, nir, nor, and nos genes, respectively (reviewed in van Spanning et al., 2007; Kraft et al., 2011; Richardson, 2011; Torres et al., 2016). Denitrification is widely distributed within the domain of Bacteria and the majority of denitrifiers are found in the phylum Proteobacteria (Shapleigh, 2006). In this phylum, we found the soybean endosymbiont, Bradyrhizobium diazoefficiens (Delamuta et al., 2013), a soil Gram-negative bacterium that is also able to grow anoxically from nitrate respiration and to carry out the complete denitrification pathway. B. diazoefficiens, which is considered a model for studying rhizobial denitrification, possesses a periplasmic nitrate reductase (Nap), a copper-containing nitrite reductase (NirK), a c-type nitric oxide reductase (cNor), and a nitrous oxide reductase (Nos) encoded by napEDABC, nirK, norCBQD, and nosRZDFYLX genes, respectively (Velasco et al., 2001, 2004; Mesa et al., 2002; Delgado et al., 2003; reviewed in Bedmar et al., 2005, 2013).
In B. diazoefficiens, a complex regulatory network comprised by two coordinated cascades (FixLJ-FixK2-NnrR and RegSR-NifA) controls the expression of genes required for microoxic, symbiotic, and denitrifying lifestyles (reviewed in Sciotti et al., 2003; Fernández et al., 2016; Torres et al., 2016). In particular, the FixLJ-FixK2-NnrR cascade is activated at a concentration of ≤5% O2 at the level of the two-component regulatory system FixLJ, where the response regulator FixJ in its active phosphorylated form induces the expression of several genes, including fixK2. In turn, the FixK2 protein activates the expression of about three-hundred genes including those for denitrification and other regulatory genes such as rpoN1, fixK1, or nnrR (Mesa et al., 2008). The product of the latter, NnrR (nitrite and nitric oxide reductase regulator), is also required for maximal expression of key denitrification genes (Mesa et al., 2003; Bueno et al., 2017; Torres et al., 2017). Recently, distinct regulation of denitrifying gene expression in response to microoxia, different nitrogen oxides and the regulatory proteins FixK2 and NnrR was reported in B. diazoefficiens. In this regulatory cascade, expression of napEDABC, nirK, and nosRZDFYLX genes all require microoxic conditions and is directly dependent on FixK2 (Bueno et al., 2017; Torres et al., 2017), while NO is the key signal for the expression of norCBQD, being NnrR the regulator which directly interacts with the norCBQD promoter (Bueno et al., 2017).
Both FixK2 and NnrR proteins belong to the cyclic AMP receptor protein (CRP) and the fumarate and nitrate reductase (FNR) regulator superfamily of transcription factors that mainly act as activators in a wide range of bacteria (reviewed in Körner et al., 2003; Fernández et al., 2016; Torres et al., 2016). These proteins share four functional domains: an N-terminal sensor domain that usually binds a specific cofactor for functional activity, a β-barrel which interacts with the RNA polymerase, an α-helix involved in protein dimerization, and a C-terminal DNA-binding domain. Generally, the active form of this kind of transcription factor consists of a homodimeric protein complex which binds to a twofold symmetric consensus DNA sequence located at distinct distances in the promoters of the target genes. Specifically, in the case of FixK2, the consensus binding site is a 14 bp palindromic sequence, TTGA/C-N6-T/GCAA (Bonnet et al., 2013), based on the FixK2-DNA complex structure as well as the consensus deduced from the alignment of the FixK2 boxes present in the promoter region of not less than 14 FixK2 targets validated by in vitro transcription (IVT) activation assays (Mesa et al., 2008; Reutimann et al., 2010; Bueno et al., 2017). However, a single binding site has been already identified for NnrR which corresponds to the promoter region of the norCBQD genes that harbors a recognition sequence with high similarity to the consensus FixK2 box (Bueno et al., 2017).
The NnrR protein from B. diazoefficiens belongs to the wider NnrR clade of proteins that orchestrate the expression of the nir and nor gene clusters in order to keep the free concentrations of nitrite and NO below cytotoxic levels (Körner et al., 2003). Another subgroup in the CRP/FNR family is the DNR-type proteins such as Dnr from Pseudomonas aeruginosa which has a similar function as the NnrR-like proteins (Giardina et al., 2008). Some global transcriptomic studies have been performed previously to identify the regulons of Dnr from P. aeruginosa (Trunk et al., 2010), NnrR from Rhodobacter sphaeroides 2.4.1 (Arai et al., 2013), and DnrF from the marine bacterium Dinoroseobacter shibae (Ebert et al., 2017). However, such an approach has not been carried out for B. diazoefficiens NnrR.
In the present work, we expanded the NnrR regulon through a comparative transcriptomic analysis of B. diazoefficiens wild type (WT) and an nnrR mutant grown under anoxic denitrifying conditions. Among nnrR targets validated by quantitative reverse transcription PCR (qRT-PCR) analyses, we identified cycA that encodes the soluble cytochrome c550 (CycA). We also demonstrated the requirement of FixK2 for IVT of cycA and nnrR as well as the necessity of FixK2 and NnrR for maximal expression of cycA in vivo. Finally, we reveal the role of CycA in the activity of the nitrous oxide reductase, adding an extra function of this cytochrome in the denitrification pathway of B. diazoefficiens.
Bacterial strains used in this study are listed in Supplementary Table S1 (all references cited in the Supplementary Material are compiled in Supplementary Data Sheet S1). Escherichia coli cells were routinely grown in Luria-Bertani (LB) medium (Miller, 1972) at 37°C. E. coli BL21 (DE3) cells for the overexpression of the recombinant FixK2 protein were incubated at 30°C. Where needed, antibiotics were used at the following concentrations (in μg/ml): ampicillin, 200; kanamycin, 30.
B. diazoefficiens cells were cultured oxically, microoxically (2% O2, 98% N2; headspace exchanged every 8–16 h) and anoxically (filled rubber-stoppered PYREX® bottles without gas exchange) at 30°C, essentially as described previously (Torres et al., 2014; Bueno et al., 2017). While Peptone-Salts-Yeast extract (PSY) (Regensburger and Hennecke, 1983; Mesa et al., 2008) was the standard medium used for B. diazoefficiens culturing, Yeast Extract-Mannitol (YEM) medium supplemented or not with 10 mM KNO3 (Daniel and Appleby, 1972) was routinely employed in our experiments. In the latter case, cells were harvested by centrifugation (5,500 × g for 7 min at 4°C), washed twice with YEM medium and subsequently used for inoculation at an optical density at 600 nm (OD600) of 0.2. Cells were then incubated for 24 h at 30°C under rigorous shaking (170 rpm; oxic cultures) or gentle shaking (80 rpm; microoxic and anoxic cultures). The OD600 of cells at harvesting were: 0.75–0.85 (oxic conditions); 0.7–0.8 (microoxic conditions); 0.3–0.4 (anoxic conditions supplemented with nitrate, WT); 0.28–0.3 (anoxic conditions supplemented with nitrate, fixK2 and nnrR mutants). Antibiotics were added to cultures as required at the following concentrations (μg/ml): chloramphenicol, 20 (solid medium); streptomycin, 200 (solid medium), 100 (liquid medium); kanamycin, 200 (solid medium), 100 (liquid medium); spectinomycin, 200 (solid medium), 100 (liquid medium).
All strains and plasmids used in this work and their corresponding description are compiled in Supplementary Table S1. A cy2 (bll2388) deletion mutant was constructed by markerless mutagenesis using the sacB-system (Schäfer et al., 1994). For that purpose, the 5′ and 3′ flanking regions of the cy2 gene (614 and 609 bp fragments) were amplified by PCR using BLL1F/BLL1R and BLL2F/BLL2R primers pairs (Supplementary Table S2). Both fragments were individually cloned in the pGEM-T easy vector, verified by sequencing and finally cloned in tandem in the suicide vector pK18mobsacB, yielding plasmid pMB2003. This plasmid was transferred via biparental conjugation to B. diazoefficiens by using E. coli S17-1 as donor and transconjugants were firstly selected by kanamycin resistance (single recombination), and secondly by sucrose resistance (double recombination) as previously described (Cabrera et al., 2016). The genomic organization of selected candidates was verified by PCR using gene-specific primers. Double recombination of plasmid pMB2003 with the B. diazoefficiens genome resulted in the replacement of the wild-type cy2 gene encoding a 128 amino acids protein for an in frame truncated version encoding 23 amino acids in the B. diazoefficiens cy2 mutant (Supplementary Table S1).
Plasmids pMB1400 and pMB1401 were individually used as templates in the IVT activation assays. pMB1400 carries the divergent promoter of nnrS and nnrR genes on a BamHI-EcoRI-digested 258-bp fragment amplified by PCR (combination of primers nnrR_6_for and nnrR_6_rev; Supplementary Table S2). The fragment was then cloned into plasmid pRJ8870 (Mesa et al., 2008), that harbors two B. diazoefficiens rrn transcriptional terminators. pMB1401 consists on an EcoRI-HindIII-digested 289-bp cycA promoter fragment that was amplified by PCR, using the cycA_1_for and cycA_1_rev primers (Supplementary Table S2) and then cloned into pRJ9519, which contains a B. diazoefficiens rrn transcriptional terminator (Beck et al., 1997).
The correct nucleotide sequence of all PCR-amplified fragments cloned in the corresponding plasmids was verified by sequencing.
A custom-designed B. diazoefficiens GeneChip BJAPETHa520090 (Affymetrix, Santa Clara, CA, United States; Hauser et al., 2007) was used to analyze the transcriptional profile of the nnrR mutant in comparison to the WT. This GeneChip has successfully been used in a wealth of global transcriptional studies performed with B. diazoefficiens (reviewed in Lardi and Pessi, 2018). Both the nnrR mutant and WT strains were cultivated under anoxic conditions in YEM medium supplemented with nitrate (hereafter named as anoxic denitrifying conditions) as described above. After growth, cells were mixed with 0.1 volume of stop solution (10% buffered phenol in ethanol; Bernstein et al., 2002), and centrifuged at 9,000 rpm, 5 min, 4°C. After centrifugation, the pellet was immediately frozen in liquid nitrogen and stored at −80°C for a further use. For each strain, a minimum of three independent biological replicates were analyzed. RNA isolation, cDNA synthesis, fragmentation, and labeling, and conditions for microarray hybridization were done as described previously (Hauser et al., 2007; Lindemann et al., 2007; Pessi et al., 2007; Mesa et al., 2008; Torres et al., 2014). The details of data processing, normalization, and further analyses were described elsewhere (Pessi et al., 2007; Mesa et al., 2008; Torres et al., 2014). Only the probe sets that were called as “present” or “marginal” in 2 out of the 3 replicates were considered for further analyses. The group of differentially expressed genes comprised those that passed the statistical test (student t-test with a p-value threshold of 0.01) and also that their change in expression [determined as n-fold change (FC)] was ≥ 2 or ≤ − 2 in comparisons between two strains or two conditions. Prediction of putative operons was performed as described elsewhere (Hauser et al., 2007; Mesa et al., 2008).
Expression of norC, nosR, cycA, cy2, and rpoN1 genes was also determined with qRT-PCR experiments using a QuantStudio 3 Real-Time PCR System (Thermo Fisher Scientific). Growth conditions of B. diazoefficiens WT and nnrR and fixK2 mutants, as well as RNA isolation and cDNA synthesis were performed as described above for the samples employed in the microarray experiments. All primers for qRT-PCR experiments are listed in Supplementary Table S2. Design of primers specifically used in this study was done with Clone Manager version 9 (Sci-Ed Software) to generate PCR fragments of about 100 bp and to have a melting temperature of 57°C to 62°C. For each target gene, qRT-PCR reactions were run in triplicate in a total volume of 19 μl containing 1 or 10 ng of cDNA, 2 μM (final concentration) of each primer and 9.5 μl of SYBR Green SuperMix (Bio-Rad). Relative changes in gene expression were calculated according to the methodology described by Pfaffl (2001). mRNA expression levels of each target gene were normalized to that of the sigma factor gene sigA.
Three hundred milliliters of cultures of B. diazoefficiens strains grown oxically, microoxically, and in anoxic denitrifying conditions were harvested by centrifugation (5,000 × g, 7 min), washed with 50 mM Tris-HCl, pH 7.5 (fractionation buffer) and resuspended in one ml of the same buffer containing 1 mM 4-[2-Aminoethyl] benzenesulfonyl fluoride hydrochloride (AEBSF) and DNAse (20 μg/ml). Cell fractionation, sodium dodecyl sulfate gel electrophoresis (SDS-PAGE) and soluble cytochromes c staining were performed essentially as described elsewhere (Delgado et al., 2003; Mesa et al., 2008). Cells were disrupted by three passes through a cold French pressure cell (SLM Aminco, Jessup, MD, United States) at about 120 MPa, and subsequently centrifuged at 20,000 × g for 10 min at 4°C to remove the cells debris. Cell-free extracts were then ultracentrifuged at 140,000 × g for 45 min at 4°C and the resulted supernatant was mixed with one sixth volume of freshly prepared SDS loading dye (350 mM Tris-HCl, pH 6.8, 30% glycerol, 20 mM DTT, 350 mM SDS, 0.05% bromophenol blue). Soluble fractions (50 μg) were loaded without boiling onto 18%-SDS-PAGE polyacrylamide gels, subsequently resolved, and transferred to a nitrocellulose membrane using Trans-Blot Turbo System (Bio-Rad). Heme-dependent peroxidase activity of c-type cytochromes (Vargas et al., 1993) was detected using the ECL SelectTM Western Blotting Detection Reagent Kit (Amersham, GE Healthcare) in a ChemiDoc XRS + System. Image analyses were performed with the Quantity One and Image LabTM softwares (Bio-Rad). Experiments were done with at least two independent biological replicates.
Steady-state levels of NosZ protein were analyzed in the soluble fraction of cultures of B. diazoefficiens grown under microoxic conditions (2% O2) in YEM medium supplemented with nitrate. This growth condition was chosen to compare our results (also nitrous oxide reductase [N2OR] measurements; see below) with previous data described by Torres et al. (2017). At least two independent biological replicates of 25 ml of cells were harvested, washed with 40 mM Tris-HCl, 150 mM KCl, pH 7 (lysis buffer) and resuspended in one ml of the same buffer containing 1 mM AEBSF. Cell fractionation was performed as described above. Twenty microgram of protein was mixed with SDS loading dye (5:1 proportion, v/v), boiled and loaded onto SDS-14% polyacrylamide gels. Immunoblotting was carried out as previously described (Torres et al., 2017) using a heterologous antibody against NosZ of Paracoccus denitrificans (1:1,000 dilution) (Felgate et al., 2012). A horseradish peroxidase (HRP)-conjugated donkey anti-sheep antibody (Sigma-Aldrich) at a 1:3,500 dilution was used as secondary antibody. Immunoreactive proteins were detected and analyzed as described above for the heme-staining experiments.
N2OR activity was measured as N2O consumption in B. diazoefficiens cultures grown microoxically in the presence of nitrate. 200 ml of cells were harvested by centrifugation (5,000 × g, 7 min) and washed at least four times with 50 mM Tris-HCl, pH 7.5, to remove the remaining nitrate and nitrite. Cell pellets were then resuspended in 4 ml of the same buffer prior to N2OR activity determination in the absence (endogenous activity) or in the presence of succinate. Reaction mixtures were individually carried out in 17 ml rubber-stoppered tubes which contained 7 ml of 50 mM Tris-HCl, pH 7.5, cell suspension (0.8–1 mg of protein), and 700 μl of a solution of 600 mM succinate, where needed. 500 μl of the headspace was exchanged by a gas mixture containing 2% N2O in 98% N2, and incubated for 30 min prior to adding cell suspension to ensure equilibrium between headspace and liquid. Four hours after the reactions were set up, aliquots of one ml of the headspace were taken and analyzed for N2O consumption essentially as described previously (Tortosa et al., 2015; Torres et al., 2017). N2O was measured by gas chromatography with a PorapaK Q 80/100 MESH column and N2 as carrier gas at 23 ml/min. The nmoles of N2O consumed were calculated based on a calibration curve done with different volumes of N2O. Activity was expressed as nmol N2O consumed per (mg prot)–1h–1.
Multiple-round IVT activation assays were essentially performed as described earlier (Beck et al., 1997; Mesa et al., 2005, 2008; Torres et al., 2017). Briefly, transcription reactions were carried out in a 50 μl reaction volume containing transcription buffer (40 mM Tris-HCl pH 8, 10 mM MgCl2, 0.1 mM EDTA, 0.1 mM DTT, 150 mM KCl, 0.4 mM K3PO4), 20 U of RNAse inhibitor (Roche), 1 mM of each NTP, 1 μCi [alpha-32P] UTP (800 Ci mmol; PerkinElmer), 750 ng of the corresponding DNA template (pMB1401 or pMB1400) and 1.5 μg of RNA polymerase of B. diazoefficiens purified as described previously (Torres et al., 2017). A C-terminally His-tagged C183S FixK2 protein (insensitive to oxidation; hereafter named as FixK2) (Bonnet et al., 2013) at 1.25 and 2.5 μM was added to the reaction mixtures where needed. Reactions were incubated 30 min at 37°C, placed on ice to stop the reaction, treated with phenol:chloroform:isoamyl alcohol and incubated overnight at −20°C to precipitate RNA. RNA size markers were prepared with T3 RNA polymerase as described elsewhere (Mesa et al., 2005). Samples were resuspended in Sanger solution (95% formamide, 40 mM EDTA pH 8, 0.05% bromophenol blue, 0.05% xylene cyanol), heated at 90°C for 5 min, centrifuged at 13,000 × g for 1 min and loaded onto a 6% polyacrylamide-7 M urea gel, which run at a constant power of 15 W. For the visualization of the radioactive signal transcripts, vacuum dried-gels were exposed to a phosphoimager screen (Molecular Dynamics) for at least 3 days. The images were analyzed with the Image LabTM software (Bio-Rad).
Protein concentration was measured using the Protein assay Dye Reagent Concentrate (Bio-Rad Laboratories) with bovine serum albumin (BSA) as standard protein for the calibration curve.
The comparative transcriptional profile of a B. diazoefficiens nnrR mutant relative to WT, during anoxic denitrifying conditions and using a custom-made GeneChip array (Hauser et al., 2007), revealed 1,242 genes that showed a differential expression in the nnrR mutant (Figure 1). The overlap of this group with those genes differentially controlled in wild-type cells cultivated in anoxic denitrifying conditions in comparison to oxically cultivated cells (1,514 genes) resulted in 298 genes (Figure 1). We next focused on those, among this latter group, which were induced in the WT and, at the same time, showed a downregulated expression in the nnrR mutant (i.e., NnrR exerts its role as an activator). The comparison of both regulons yielded 175 genes (Figure 1 and Supplementary Table S3). Next, in order to narrow down the number of genes for further analysis, we did a systematic search for promoter-associated putative FixK2 binding sites as a first approximation, since no consensus sequence for NnrR binding has been defined. Due to the homology between the consensus FixK2 box (Bonnet et al., 2013), and the only so far known NnrR binding site present in the norCBQD promoter (Bueno et al., 2017), it can argued that the FixK2 box might also serve as recognition site also for NnrR. From this, we then identified 60 genes within the 175 genes group that form part of 42 predicted transcriptional units (mono- or poly-cistronic) associated with a putative FixK2 box (Table 1). Within this group we found: (i) Three of the four structural gene clusters involved in denitrification, i.e., nirK (Velasco et al., 2001), norCBQD (Mesa et al., 2002) and nosRZDFYLX (Velasco et al., 2004), encoding the NirK, cNor, and Nos enzymes, respectively; (ii) genes encoding cytochromes, such as bll2388 (cy2) (Mesa et al., 2008), and cycA (cytochrome c550) (Bott et al., 1995); (iii) regulatory genes as nnrR itself and rpoN1 that encodes one of the two copies of the alternative sigma factor RpoN that collaborates with the transcription factor NifA for activation of genes involved in nitrogen fixation (reviewed in Dixon and Kahn, 2004). The downregulation of the nnrR gene observed in the nnrR mutant indicates, as it was described previously by Mesa et al. (2003), that NnrR does not negatively auto-regulate its own expression; (iv) two copies of the oxygen-independent coproporphyrinogen-III oxidase involved in heme biosynthesis under oxygen limitation (i.e., hemN1 and hemN2); (v) the phbB gene encoding PhaB, one of the two copies of NADP–acetoacetyl-CoA reductases involved in the second step of polyhydroxybutyrate (PHB) biosynthesis (Quelas et al., 2013). We next selected few candidates listed in Table 1 for validation by qRT-PCR of their gene expression profile based on microarray data. In these experiments, we could confirm that norC, nosR, cycA, cy2, and rpoN1 genes are targets positively activated by NnrR (Figure 2), being cycA and its product the focus of our study.
Figure 1. Venn diagram representing the group of differentially expressed genes in the nnrR mutant in comparison to the WT, both grown in anoxic denitrifying conditions (light gray circle), and the group of differentially expressed genes in cells of the WT grown in anoxic denitrifying conditions compared with cells grown in oxic conditions (dark gray circle). Numbers in parentheses indicate the total number of differentially expressed genes. The overlap of both groups represents 298 genes; among them, 175 genes showed a downregulated expression in the nnrR mutant and at the same time are induced in the WT grown in anoxic denitrifying conditions. The latter set contains 60 genes organized in mono-, or polycistronic transcriptional units that contain a putative FixK2 box in the promoter region (42 FixK2 boxes associated to transcriptional units, see Table 1). Up-down arrows refer to increased and decreased gene expression in microarray analyses.
Table 1. List of the 60 genes belonging to 42 putative FixK2 box-associated transcription units whose expression is downregulated in the nnrR mutant in comparison to the WT, both cultured under anoxic denitrifying conditions, and at the same time are induced in the WT in anoxic denitrifying conditions compared to oxic conditions.
Figure 2. Expression data of selected genes determined by microarray (black bars) and qRT-PCR (gray bars). Fold change (FC) refers to the relative expression of norC, nosR, cycA, cy2, norC, and rpoN1 genes in the nnrR mutant in comparison with the WT, both cultured in anoxic denitrifying conditions. qRT-PCRs were performed with two independent experiments, each one with six parallel amplification reactions.
The cycA gene codes for the so-called cytochrome c550, a c-type soluble cytochrome (CycA) (Bott et al., 1995), previously identified as an intermediate electron donor between the cytochrome bc1 membrane complex and the denitrifying nitrite reductase NirK (Bueno et al., 2008). In order to confirm the positive control of NnrR on cycA also at protein level, we monitored the expression of CycA in the WT, and nnrR mutant by using heme-c staining analyses (Figure 3A). A cycA mutant was included as control in the experiments. For this purpose, the soluble fraction was isolated from cells grown under oxic, microoxic and anoxic denitrifying conditions (see Materials and Methods for further details). As shown in Figure 3A (lanes 1, 2 and 6), two stained bands of about 15 and 12 kDa were observed in the soluble fraction of wild-type cells independently of the growth conditions. The 15 kDa band corresponds to the previously identified cytochrome c555 (CycC) encoded by cycC (Bott et al., 1995), and the NapB subunit of the periplasmic nitrate reductase (Delgado et al., 2003) that co-migrate together. The 12 kDa-band was not detected in the cycA mutant (Figure 3A, lanes 5, and 9), indicating that this band corresponds to the CycA holoprotein (processed with covalently bound heme) with a predicted molecular mass of 12.320 kDa. Similarly, Bott et al. (1995) identified the 12 kDa band in the heme c-staining profiling of soluble cytochromes detected in oxic conditions as cytochrome c550 (CycA).
Figure 3. The regulatory proteins FixK2 and NnrR are involved in the control of cycA expression. (A) Profile of heme-stained soluble proteins from B. diazoefficiens WT, and fixK2, nnrR, and cycA mutants. Cells were cultivated during 24 h in YEM medium in the absence (oxic, and microoxic [2% O2] conditions) or in the presence (anoxic denitrifying conditions) of 10 mM of KNO3. 50 μg of soluble proteins were loaded per lane. Heme stained c-type cytochromes identified previously are specified at the right margin and their predicted molecular mass at the left. Each panel corresponds to different sections of the same gel (soluble fractions isolated from oxically and microoxically grown cells; Supplementary Data Sheet S2A) or a different gel (soluble fractions of cells grown in anoxic denitrifying conditions; Supplementary Data Sheet S2A. (B) qRT-PCR analyses of cycA in the WT, and fixK2 and nnrR mutants. Six parallel reactions were performed with cDNA retro-transcribed from RNA isolated from cells grown in anoxic denitrifying conditions (at least two independent biological replicates per strain). Fold change (FC) values refer to the relative expression in the fixK2 and nnrR mutants compared to the WT. anoxia, anoxic denitrifying conditions.
As shown in Figure 3A (lanes 1 and 2), CycA levels were similar in oxic or microoxic cultures of wild-type cells, while an about twofold induction of the CycA band was observed in the soluble fraction of the WT grown under anoxic denitrifying conditions (Figure 3A, comparison of lane 6 and lanes 1, and 2). In addition to the 15- and 12- kDa bands, two smaller cytochromes of about 10 and 9 kDa were also detected in the soluble fraction of wild-type cells cultured microoxically or in anoxic denitrifying conditions (Figure 3A, lanes 2, and 6). While the 10 kDa c-type cytochrome has not been yet identified, the 9 kDa c-type cytochrome corresponds to the soluble cytochrome CycS expressed in anaerobic, nitrate-grown cells (Mesa et al., 2008).
In the pattern of soluble c-type cytochromes detected in the nnrR mutant (Figure 3A, comparison of lanes 4 and 2), CycA expression was similar to that detected in wild-type cells when both strains were grown microoxically. However, under anoxic denitrifying conditions, the levels of CycA in the nnrR mutant were about two to three-fold lower to that of the WT (Figure 3A, lanes 6, and 8). Next, given the dependency of nnrR expression on the transcription factor FixK2 (Mesa et al., 2003, 2008), we also analyzed the expression of CycA in a fixK2 mutant cultured under microoxic and anoxic denitrifying conditions. Interestingly, we detected a similar expression profile for CycA in the fixK2 mutant to the one observed in the nnrR mutant (Figure 3A, lanes 3, and 7), which is consistent with the requirement of both FixK2 and NnrR for the maximal expression of CycA. This mutual dependence of CycA expression on these two CRP/FNR transcription factors was also confirmed at transcriptional levels by monitoring cycA expression by qRT-PCR (Figure 3B) which was about six and fivefold lower in the fixK2 and nnrR mutants, respectively, compared to the WT.
Multiple-round IVT activation assays were performed to investigate whether cycA is directly controlled by the transcriptional regulator FixK2. To achieve this goal, we monitored transcription from the cycA promoter, cloned in plasmid pMB1401, with the native RNA polymerase purified from B. diazoefficiens (Torres et al., 2017) in the presence or in the absence of recombinant FixK2 protein. Without FixK2, B. diazoefficiens RNAP failed to transcribe from the cycA promoter (Figure 4, lane 1) whereas it produced a vector-encoded transcript of 107 nucleotides that it used as an internal control. In the presence of two different concentrations of FixK2 (1.25 and 2.5 μM dimer), B. diazoefficiens RNAP transcribed the cycA promoter efficiently, producing an expectable transcript of 269 nucleotides, with almost no change in the intensity of the 107-nucleotide control (Figure 4, lanes 2 and 3).
Figure 4. IVT activation of nnrR, nnrS and cycA genes by FixK2. Plasmids pMB1400 and pMB1401 were used for a multiple-round IVT activation assay with purified FixK2 protein (no protein, lanes 1, and 4; 1.25 μM, lane 2, and 5; 2.5 μM, lane 3) and RNA polymerase from B. diazoefficiens. RNA size markers (M, M′) were produced as described earlier (Mesa et al., 2005). The positions of the FixK2-dependent transcripts are marked with arrows. A vector-encoded transcript of 107 nucleotides (reference transcript) which serves as internal control appears in all lanes. Transcription products generated from each promoter were run on different gels. nt, nucleotides; rt, reference transcript.
Previous work has shown that the activation of a nnrR′-′lacZ fusion under anoxic denitrifying conditions depends on FixK2 (Mesa et al., 2003). In order to elucidate whether FixK2 exerts direct or indirect control on nnrR, we also performed IVT analyses from the nnrR promoter. nnrR and its divergent oriented gene nnrS share a single predicted FixK2 box located at their intergenic promoter region which was cloned into the template plasmid pRJ8870 (Mesa et al., 2008), that carries two transcriptional terminators. As shown in Figure 4 (lane 5), B. diazoefficiens RNAP transcribed the nnrS and nnrR promoters only in the presence of purified FixK2 protein producing two expectable transcripts of 275 nucleotides (corresponding to nnrR), and 199 nucleotides (corresponding to nnrS). Collectively, these results allowed us to identify three new direct targets for FixK2: cycA, nnrR, and nnrS.
In order to investigate whether CycA could act as a potential electron donor to the nitrous oxide reductase (N2OR) enzyme, we determined N2OR activity in wild-type and cycA mutant cells under microoxic conditions (2% O2) in YEM medium supplemented with nitrate. Cells were cultured for 24 h, harvested by centrifugation and subsequently incubated microoxically in a reaction mixture supplemented with or without succinate as physiological electron donor. The ability of the cells to consume N2O was measured at 2 and 4 h after cell inoculation. As shown in Figure 5A, despite of the fact that the presence of succinate in the medium increased about 1.5-fold N2OR activity in both WT and cycA mutant, values of N2OR activity in cycA cells were about 65% lower than those observed in wild-type cells. As expected, almost no N2OR activity was detected in cells of a nosZ mutant (Figure 5A).
Figure 5. CycA is involved in nitrous oxide reductase activity. (A) N2OR activity determined in the WT and cycA mutant in the absence or in the presence of succinate as physiological electron donor. Activity is expressed as nmol N2O consumed per (mg prot)–1h– 1. Shown are means with standard errors of the three measurements of one representative experiment from at least two independent biological replicates. (B) Immunodetection of NosZ protein in the soluble fraction of the WT and cycA mutant. 20 μg of the soluble fraction of each strain were resolved by SDS-PAGE and immunoblotted with anti-NosZ antibody from P. denitrificans (for a detailed description see section Materials and Methods). The size of the predicted molecular mass of the mature periplasmic NosZ protein (67 kDa) is indicated on the left side. The original image of the immunoblot is shown in Supplementary Data Sheet S2B. In both series of experiments (A,B), cells were grown microoxically (2% O2) for 24 h in YEM medium supplemented with nitrate. A nosZ mutant was included as negative control.
We also analyzed the expression of nosR, the first gene of the nosRZDFYLX operon, by qRT-PCR as well as the steady-state levels of NosZ, the catalytic subunit of N2OR in the WT and the cycA mutant. qRT-PCR analyses showed that nosR expression was not significantly affected in the cycA mutant (FC of −2.9) compared to wild-type cells, both cultivated under microoxic conditions with nitrate. Western blot analysis of NosZ in the soluble fraction of the cycA mutant and wild-type cells using an antibody against purified P. denitrificans NosZ (Felgate et al., 2012) showed that a band of about 67 kDa corresponding to the predicted molecular mass of the mature periplasmic NosZ protein, was detected with a similar intensity in both strains (Figure 5B, lanes 1, and 2). This band was absent in the soluble fraction extracted from B. diazoefficiens nosZ mutant cells which confirmed that corresponds to NosZ (Figure 5B, lane 3).
Denitrification in bacteria (reviewed in Zumft, 1997; van Spanning et al., 2007; Kraft et al., 2011; Richardson, 2011; Bueno et al., 2012; Torres et al., 2016), and specifically in the model organism B. diazoefficiens (reviewed in Bedmar et al., 2005, 2013) has been widely studied to date. The regulatory protein NnrR plays an important role in B. diazoefficiens since it expands the regulatory cascade FixLJ-FixK2 and integrates the nitrogen oxide signal (Mesa et al., 2003). However, the regulon of NnrR has not been yet fully mapped. In this work, a transcriptomic analysis of an nnrR mutant in comparison to the WT, both cultured under anoxic denitrifying conditions, was performed and compared with the group of anoxically-induced genes in the WT. This comparison led the identification of 175 genes induced by anoxia under the positive regulatory control of NnrR (Supplementary Table S3). Since the change in expression of some of these genes might be due to indirect effects (e.g., different grow behavior of the nnrR mutant and the WT, or the putative involvement of another regulatory protein), we focused on a group of 60 genes that contains a FixK2 binding site within their promoter regions (Table 1). Among this group, three targets (nirK, norCBQD, nosRZDFYLX) were previously reported to be under its direct or indirect control (Mesa et al., 2003; Robles et al., 2006; Bueno et al., 2017; Torres et al., 2017). Particularly, our results confirm those that showed a reduced expression of nirK-lacZ, norC-lacZ, or nosR-lacZ fusions in an nnrR mutant (Bueno et al., 2017; Torres et al., 2017). However, the only known direct target for NnrR is norCBQD gene cluster, since purified recombinant NnrR protein was able to bind its promoter region in an anoxic-specific manner (Bueno et al., 2017), while the downregulation of nirK, and nosRZDFYLX was attributed to the toxic effect of NO accumulation in the nnrR mutant (Torres et al., 2017). In this work, we also identified as NnrR targets genes involved in electron transport through the denitrification pathway. This is the case of cycA encoding a cytochrome c550 which acts as an electron donor to NirK (Bueno et al., 2008), and cy2 that encodes cytochrome c2, which was previously described as an indirect FixK2 target (Mesa et al., 2008). Other genes putatively activated by NnrR are hemN1 and hemN2, where the latter encodes a gene product required for denitrification as well as for symbiotic nitrogen fixation as reported by Fischer et al. (2001). In addition to the genes described above, we also found rpoN1 as a target under the positive control of NnrR which points to additional cross-control between the two oxygen-responsive regulatory cascades RegSR-NifA and FixLJ-FixK2-NnrR. In fact, 14 out the 65 genes that constitute the direct NifA + RpoN1+2 regulon (Hauser et al., 2007) showed a downregulated expression in the nnrR mutant (Supplementary Table S3). Taken together, these results expand the B. diazoefficiens NnrR regulon under denitrifying conditions. In other rhizobia such as Ensifer meliloti, transcriptomic studies were consistent with our findings, and identified denitrification genes (nirK and norC), as well as other genes associated to denitrification (azu1, hemN, nnrU, and nnrS) as NnrR targets (Meilhoc et al., 2010). In contrast to B. diazoefficiens, E. meliloti NnrR and FixK are part of two different regulatory cascades (reviewed in Cabrera et al., 2011).
NnrR orthologs are present in other organisms, such as NnrR of R. sphaeroides 2.4.1. In cells exposed to nitrosative stress, the norCBQD gene cluster, other genes encoding putative accessory proteins for the assembly of cNor as well as others involved in NO metabolism were identified (Arai et al., 2013). Similar to our findings for B. diazoefficiens NnrR, microarrays analysis of cells of a P. aeruginosa Dnr mutant cultured under anaerobic conditions revealed that the denitrification structural genes nirS, norCB and nosR as well as hemN, and genes encoding c-type cytochromes are controlled by this protein (Rodionov et al., 2005; Trunk et al., 2010). In the same way, DnrF from the marine bacterium D. shibae is required for the induction of the expression of norCB, hemA encoding the 5-aminolevulinate synthase, and a gene responsible for cytochrome c biosynthesis (Ebert et al., 2017).
We also carried out an in-depth characterization of cycA, one of the targets under the positive control of NnrR. By performing qRT-PCR and heme c-staining analyses, we were able to confirm the requirement of NnrR for the maximal expression of cycA in cells cultivated in anoxic denitrifying conditions. In addition to NnrR, the anoxic induction of cycA was also controlled by FixK2. However, cycA did not appear to be a target for FixK2 in previous microarray analyses of a fixK2 mutant grown microoxically (Mesa et al., 2008). We therefore postulate that anoxia might be required for FixK2-mediated control of cycA induction. In fact, similar heme c-staining levels of CycA were observed in the fixK2 mutant with respect to those in wild-type cells cultured either under microoxic or oxic conditions. However, the significant induction of CycA observed in the WT in response to anoxic denitrifying conditions in comparison to oxic or microoxic conditions was not observed in fixK2 mutant cells. The involvement of FixK2 on cycA induction under anoxic conditions was also confirmed at transcriptional level in qRT-PCR experiments. Moreover, we could also identify cycA as a direct target for FixK2 based on the FixK2-dependent cycA transcript detected in IVT activation experiments.
In previous studies, purified FixK2 protein has been shown to activate transcription in concert with B. diazoefficiens RNA polymerase from napEDABC, nirK, and nosRZDFYLX promoters, but not from the norCBQD promoter (Bueno et al., 2017; Torres et al., 2017), being NnrR the candidate to directly control norCBQD genes expression. In this work, we demonstrated that nnrR and its divergently oriented gene nnrS are two newly identified targets directly activated by FixK2. These results are in agreement with the dependency of both nnrR and nnrS induction on FixK2 (Mesa et al., 2003, 2008) and they also ratified that control of FixK2 on norCBQD genes is indirect via NnrR.
Gene regulation by NnrR could be direct (as proposed for norCBQD; Bueno et al., 2017), indirect (as reported for nosRZDFYLX and nirK; Torres et al., 2017) or with the involvement of another regulatory factor such as FixK2 (as suggested for nirK; Bueno et al., 2017). In principle, FixK2 and NnrR could also act together to regulate other targets identified that showed differential expression in the nnrR mutant. However, the presence of purified NnrR did not increase specific FixK2-mediated transcription from the nirK promoter in an IVT assay (Bueno et al., 2017) implying that the purified NnrR protein does not interact with FixK2 or it is not in the correct conformation to drive transcription. Further, IVT activation of target genes triggered by NnrR seemed to be recalcitrant (Bueno et al., 2017), therefore, at the moment, it is difficult to determine the most plausible mechanism involved in the NnrR-mediated control.
As mentioned above, CycA is a soluble cytochrome involved in anaerobic growth of B. diazoefficiens using nitrate or nitrite as final electron acceptor that suggests a role of this cytochrome in denitrification (Bott et al., 1995; Bueno et al., 2008). Specifically, it is involved in the electrons transfer from cytochrome bc1 to nitrite reductase (NirK) (Bueno et al., 2008). We have now reported, for the first time in B. diazoefficiens, the role of CycA in nitrous oxide reductase functioning, given the reduced N2OR activity observed in the cycA mutant compared to that of the WT. In addition to its involvement in the denitrification process, CycA plays a role in chemolithotrophic growth of B. diazoefficiens when thiosulfate is used as electron donor (Masuda et al., 2017). In this growth condition, electrons are preferentially channeled via cytochrome CycA to the aa3-type heme-copper cytochrome oxidase encoded by coxBAFC genes. Here, it is noteworthy that NirK, NosZ, and CoxB have copper (Cu) centers, particularly NosZ and CoxB contain Cu centers type A (CuA) (Bühler et al., 2010). These observations let us to suggest that CycA might be responsible for electron transfer to Cu-containing proteins belonging to different respiratory pathways.
Importantly, the cycA mutant retained partial N2OR activity, which suggests that alternative electron donors might be also involved in electron transfer to Nos. This has been observed in other model denitrifying bacteria (reviewed in van Spanning, 2011; Torres et al., 2016) and the presence of alternative electron transfer routes was also proposed for chemolithoautotrophic growth as reported by Masuda et al. (2017). To identify other candidates as potential electron donors to Nos, we determined N2OR activity in B. diazoefficiens strains defective in the soluble cytochromes c2 encoded by bll2388 and CycS encoded by bll6062 (Mesa et al., 2008). However, as shown in Supplementary Figure S1, none of these mutants showed a clear negative effect on N2OR activity compared to wild-type activity levels. Thus, we propose the soluble cytochrome CycA is an important but not the only electron donor to the nitrous oxide reductase.
In summary, this work has contributed to increase the understanding of the regulation of denitrification in the model rhizobial denitrifier B. diazoefficiens by expanding the regulon of NnrR, one of the key proteins involved in the control of this process. The cycA gene, one of the identified targets, resulted also to be directly activated by the transcription factor FixK2. Moreover, we showed the direct link between FixK2 and nnrR, further highlighting the role of NnrR as an intermediary in the FixK2-mediated control of some target genes. Finally, we reveal an extra role for the soluble cytochrome CycA in the electron transfer pathway to Nos, adding a new player for nitrous oxide reduction in endosymbiotic soil bacteria.
The microarray expression data are available in the NCBI Gene Expression Omnibus (GEO) database (http://www.ncbi.nlm.nih.gov/geo) under the GEO Series accession number GSE130684.
SM and MD conceived and designed the study. AJ-L, JC, EB, MT, and SS performed the experiments. AJ-L, MD, and SM analyzed the results and wrote the manuscript. AJ-L prepared the figures and tables. JC contributed to the set-up of some methodologies used in this study, the preparation of figures and tables, and the manuscript writing. EJB critically revised the manuscript. All authors read and approved the final version of the manuscript.
This work was funded by the Ministerio de Economía y Competitividad (Spain) under Fondo Europeo de Desarrollo Regional (FEDER)-co-financed grants AGL2011-23383 and AGL2015-63651-P to SM and AGL2013-45087-R and AGL2017-85676-R to MD. Grant P12-AGR-1968 and support from the Junta de Andalucía to Group BIO-275 are also acknowledged. AJ-L was granted by a Ph.D. contract associated to Grant P12-AGR-1968. JC, MT, and SS were supported by contracts funded by Grants AGL2015-63651-P, AGL2013-45087-R, and AGL2011-23383, respectively. EB acknowledges the financial support from the Consejo Superior de Investigaciones Científicas JAE-DOC Programme co-financed by European Social Fund (ESF).
The authors declare that the research was conducted in the absence of any commercial or financial relationships that could be construed as a potential conflict of interest.
We thank the staff of the Functional Genomics Center (Zurich, Switzerland) for the help and assistance in the microarray experiments. Hauke Hennecke (ETH Zurich, Switzerland) is deeply acknowledged for his generous gift of the microarrays used in this work and the cycA mutant, and for his fruitful comments on the manuscript. Hans-Martin Fischer (ETH Zurich, Switzerland) kindly helped in the assistance to deposit microarray data in the GEO database. The expert technical assistance and advices for nitrous oxide reductase activity determination by Germán Tortosa (EEZ, CSIC, Granada, Spain) are highly appreciated. We are grateful to David J. Richardson and Andrew J. Gates (University of East Anglia, Norwich, United Kingdom) for kindly supplying the polyclonal antibody against NosZ from P. denitrificans. Andrew J. Gates is also acknowledged for his critical revision of the manuscript and the improvement of the written English.
The Supplementary Material for this article can be found online at: https://www.frontiersin.org/articles/10.3389/fmicb.2019.01926/full#supplementary-material
FIGURE S1 | Nitrous oxide reductase (N2OR) activity in the absence and in the presence of succinate as electron donor determined in the WT and cy2, and cycS mutants. Activity is expressed in nmol N2O consumed per (mg prot)–1h–1. Cells were grown microoxically (2% O2) for 24 h in YEM medium supplemented with nitrate. A nosZ mutant was included as negative control. Shown are means with standard errors of a representative experiment assayed in triplicates.
TABLE S1 | Strains and plasmids used in this study.
TABLE S2 | Oligonucleotides used in this study.
TABLE S3 | List of the 175 genes induced under anoxic denitrifying conditions and at the same time their expression is downregulated in the nnrR mutant in comparison to the WT, both cultivated in anoxic denitrifying conditions.
DATA SHEET S1 | References cited in the Supplementary Material.
DATA SHEET S2 | Full scans of the entire gels of the blots shown in Figure 3A (A), and Figure 5B (B). Specific details for each image are identical to those described in the corresponding legend to the figure. In (B), the 50 kDa band detected in the WT and cycA mutant corresponds to the C-terminal truncated B. diazoefficiens NosZ protein as previously reported for NosZ from P. denitrificans (Felgate et al., 2012). A 50 kDa band was also present in the soluble fraction of the nosZ insertion mutant which produces a shorter 48.7 kDa polypeptide (deletion at the C-terminal end).
Arai, H., Roh, J. H., Eraso, J. M., and Kaplan, S. (2013). Transcriptome response to nitrosative stress in Rhodobacter sphaeroides 2.4.1. Biosci. Biotechnol. Biochem. 77, 111–118. doi: 10.1271/bbb.120601
Beck, C., Marty, R., Klausli, S., Hennecke, H., and Gottfert, M. (1997). Dissection of the transcription machinery for housekeeping genes of Bradyrhizobium japonicum. J. Bacteriol. 179, 364–369. doi: 10.1128/jb.179.2.364-369.1997
Bedmar, E. J., Bueno, E., Correa, D., Torres, M. J., Delgado, M. J., and Mesa, S. (2013). “Ecology of denitrification in soils and plant-associated bacteria,” in Beneficial Plant-microbial interactions: Ecology and Applications, eds B. Rodelas and J. Gonzalez-López (Boca Ratón, FL: CRC Press), 164–182. doi: 10.1201/b15251-9
Bedmar, E. J., Robles, E. F., and Delgado, M. J. (2005). The complete denitrification pathway of the symbiotic, nitrogen-fixing bacterium Bradyrhizobium japonicum. Biochem. Soc. Trans. 33, 141–144. doi: 10.1042/BST0330141
Bernstein, J. A., Khodursky, A. B., Lin, P. H., Lin-Chao, S., and Cohen, S. N. (2002). Global analysis of mRNA decay and abundance in Escherichia coli at single-gene resolution using two-color fluorescent DNA microarrays. Proc. Natl. Acad. Sci. U.S.A. 99, 9697–9702. doi: 10.1073/pnas.112318199
Bonnet, M., Kurz, M., Mesa, S., Briand, C., Hennecke, H., and Grutter, M. G. (2013). The structure of Bradyrhizobium japonicum transcription factor FixK2 unveils sites of DNA binding and oxidation. J. Biol. Chem. 288, 14238–14246. doi: 10.1074/jbc.M113.465484
Bott, M., Thöny-Meyer, L., Loferer, H., Rossbach, S., Tully, R. E., Keister, D., et al. (1995). Bradyrhizobium japonicum cytochrome c550 is required for nitrate respiration but not for symbiotic nitrogen fixation. J. Bacteriol. 177, 2214–2217. doi: 10.1128/jb.177.8.2214-2217.1995
Bueno, E., Bedmar, E. J., Richardson, D. J., and Delgado, M. J. (2008). Role of Bradyrhizobium japonicum cytochrome c550 in nitrite and nitrate respiration. FEMS Microbiol. Lett. 279, 188–194. doi: 10.1111/j.1574-6968.2007.01034.x
Bueno, E., Mesa, S., Bedmar, E. J., Richardson, D. J., and Delgado, M. J. (2012). Bacterial adaptation of respiration from oxic to microoxic and anoxic conditions: redox control. Antioxid. Redox Signal. 16, 819–852. doi: 10.1089/ars.2011.4051
Bueno, E., Robles, E. F., Torres, M. J., Krell, T., Bedmar, E. J., Delgado, M. J., et al. (2017). Disparate response to microoxia and nitrogen oxides of the Bradyrhizobium japonicum napEDABC, nirK and norCBQD denitrification genes. Nitric Oxide 68, 137–149. doi: 10.1016/j.niox.2017.02.002
Bühler, D., Rossmann, R., Landolt, S., Balsiger, S., Fischer, H. M., and Hennecke, H. (2010). Disparate pathways for the biogenesis of cytochrome oxidases in Bradyrhizobium japonicum. J. Biol. Chem. 285, 15704–15713. doi: 10.1074/jbc.M109.085217
Cabrera, J. J., Salas, A., Torres, M. J., Bedmar, E. J., Richardson, D. J., Gates, A. J., et al. (2016). An integrated biochemical system for nitrate assimilation and nitric oxide detoxification in Bradyrhizobium japonicum. Biochem. J. 473, 297–309. doi: 10.1042/BJ20150880
Cabrera, J. J., Sánchez, C., Gates, A. J., Bedmar, E. J., Mesa, S., Richardson, D. J., et al. (2011). The nitric oxide response in plant-associated endosymbiotic bacteria. Biochem. Soc. Trans. 39, 1880–1885. doi: 10.1042/bst20110732
Daniel, R. M., and Appleby, C. A. (1972). Anaerobic-nitrate, symbiotic and aerobic growth of Rhizobium japonicum: effects on cytochrome P450, other haemoproteins, nitrate and nitrite reductases. Biochim. Biophys. Acta 275, 347–354. doi: 10.1016/0005-2728(72)90215-0
Delamuta, J. R., Ribeiro, R. A., Ormeno-Orrillo, E., Melo, I. S., Martínez-Romero, E., and Hungría, M. (2013). Polyphasic evidence supporting the reclassification of Bradyrhizobium japonicum group Ia strains as Bradyrhizobium diazoefficiens sp. nov. Int. J. Syst. Evol. Microbiol. 63, 3342–3351. doi: 10.1099/ijs.0.049130-0
Delgado, M. J., Bonnard, N., Tresierra-Ayala, A., Bedmar, E. J., and Muller, P. (2003). The Bradyrhizobium japonicum napEDABC genes encoding the periplasmic nitrate reductase are essential for nitrate respiration. Microbiology 149, 3395–3403. doi: 10.1099/mic.0.26620-0
Dixon, R., and Kahn, D. (2004). Genetic regulation of biological nitrogen fixation. Nat. Rev. Microbiol. 2, 621–631. doi: 10.1038/nrmicro954
Ebert, M., Schweyen, P., Broring, M., Laass, S., Hartig, E., and Jahn, D. (2017). Heme and nitric oxide binding by the transcriptional regulator DnrF from the marine bacterium Dinoroseobacter shibae increases napD promoter affinity. J. Biol. Chem. 292, 15468–15480. doi: 10.1074/jbc.M117.798728
Felgate, H., Giannopoulos, G., Sullivan, M. J., Gates, A. J., Clarke, T. A., Baggs, E., et al. (2012). The impact of copper, nitrate and carbon status on the emission of nitrous oxide by two species of bacteria with biochemically distinct denitrification pathways. Environ. Microbiol. 14, 1788–1800. doi: 10.1111/j.1462-2920.2012.02789.x
Fernández, N., Cabrera, J. J., Salazar, S., Parejo, S., Rodríguez, M. C., Lindemann, A., et al. (2016). “Molecular determinants of negative regulation of the Bradyrhizobium diazoefficiens transcription factor FixK2,” in Biological Nitrogen Fixation and Beneficial Plant-Microbe Interactions, eds F. González-Andrés and E. James (Cham: Springer International Publishing), 57–72. doi: 10.1007/978-3-319-32528-6_6
Fernández, N., Cabrera, J. J., Varadarajan, A. R., Lutz, S., Ledermann, R., Roschitzki, B., et al. (2019). An integrated approach unveils new aspects of microoxia-mediated regulation in B. diazoefficiens. Front. Microbiol. 10:924. doi: 10.3389/fmicb.2019.00924
Fischer, H. M., Velasco, L., Delgado, M. J., Bedmar, E. J., Scharen, S., Zingg, D., et al. (2001). One of two hemN genes in Bradyrhizobium japonicum is functional during anaerobic growth and in symbiosis. J. Bacteriol. 183, 1300–1311. doi: 10.1128/jb.183.4.1300-1311.2001
Giardina, G., Rinaldo, S., Johnson, K. A., Di Matteo, A., Brunori, M., and Cutruzzola, F. (2008). NO sensing in Pseudomonas aeruginosa: structure of the transcriptional regulator DNR. J. Mol. Biol. 378, 1002–1015. doi: 10.1016/j.jmb.2008.03.013
Hauser, F., Pessi, G., Friberg, M., Weber, C., Rusca, N., Lindemann, A., et al. (2007). Dissection of the Bradyrhizobium japonicum NifA+sigma54 regulon, and identification of a ferredoxin gene (fdxN) for symbiotic nitrogen fixation. Mol. Genet. Genomics 278, 255–271. doi: 10.1007/s00438-007-0246-9
Kaneko, T., Nakamura, Y., Sato, S., Minamisawa, K., Uchiumi, T., Sasamoto, S., et al. (2002). Complete genomic sequence of nitrogen-fixing symbiotic bacterium Bradyrhizobium japonicum USDA110. DNA Res. 9, 189–197. doi: 10.1093/dnares/9.6.189
Körner, H., Sofia, H. J., and Zumft, W. G. (2003). Phylogeny of the bacterial superfamily of Crp-Fnr transcription regulators: exploiting the metabolic spectrum by controlling alternative gene programs. FEMS Microbiol. Rev. 27, 559–592. doi: 10.1016/s0168-6445(03)00066-4
Kraft, B., Strous, M., and Tegetmeyer, H. E. (2011). Microbial nitrate respiration-genes, enzymes and environmental distribution. J. Biotechnol. 155, 104–117. doi: 10.1016/j.jbiotec.2010.12.025
Lardi, M., and Pessi, G. (2018). Functional genomics approaches to studying symbioses between legumes and nitrogen-fixing rhizobia. High Throughput. 7:E15. doi: 10.3390/ht7020015
Lindemann, A., Moser, A., Pessi, G., Hauser, F., Friberg, M., Hennecke, H., et al. (2007). New target genes controlled by the Bradyrhizobium japonicum two-component regulatory system RegSR. J. Bacteriol. 189, 8928–8943. doi: 10.1128/JB.01088-07
Masuda, S., Hennecke, H., and Fischer, H. M. (2017). Requirements for efficient thiosulfate oxidation in Bradyrhizobium diazoefficiens. Genes 8:E390. doi: 10.3390/genes8120390
Meilhoc, E., Cam, Y., Skapski, A., and Bruand, C. (2010). The response to nitric oxide of the nitrogen-fixing symbiont Sinorhizobium meliloti. Mol. Plant. Microbe Interact. 23, 748–759. doi: 10.1094/mpmi-23-6-0748
Mesa, S., Bedmar, E. J., Chanfon, A., Hennecke, H., and Fischer, H. M. (2003). Bradyrhizobium japonicum NnrR, a denitrification regulator, expands the FixLJ-FixK2 regulatory cascade. J. Bacteriol. 185, 3978–3982. doi: 10.1128/JB.185.13.3978-3982.2003
Mesa, S., Hauser, F., Friberg, M., Malaguti, E., Fischer, H. M., and Hennecke, H. (2008). Comprehensive assessment of the regulons controlled by the FixLJ-FixK2-FixK1 cascade in Bradyrhizobium japonicum. J. Bacteriol. 190, 6568–6579. doi: 10.1128/JB.00748-08
Mesa, S., Ucurum, Z., Hennecke, H., and Fischer, H. M. (2005). Transcription activation in vitro by the Bradyrhizobium japonicum regulatory protein FixK2. J. Bacteriol. 187, 3329–3338. doi: 10.1128/JB.187.10.3329-3338.2005
Mesa, S., Velasco, L., Manzanera, M. E., Delgado, M. J., and Bedmar, E. J. (2002). Characterization of the norCBQD genes, encoding nitric oxide reductase, in the nitrogen fixing bacterium Bradyrhizobium japonicum. Microbiology 148, 3553–3560. doi: 10.1099/00221287-148-11-3553
Miller, J. H. (1972). Experiments in Molecular Genetics. New York, NY: Cold Spring Harbor Laboratory.
Pessi, G., Ahrens, C. H., Rehrauer, H., Lindemann, A., Hauser, F., Fischer, H. M., et al. (2007). Genome-wide transcript analysis of Bradyrhizobium japonicum bacteroids in soybean root nodules. Mol. Plant. Microbe Interact. 20, 1353–1363. doi: 10.1094/MPMI-20-11-1353
Pfaffl, M. W. (2001). A new mathematical model for relative quantification in real-time RT-PCR. Nucleic Acids Res. 29:e45. doi: 10.1093/nar/29.9.e45
Quelas, J. I., Mongiardini, E. J., Pérez-Giménez, J., Parisi, G., and Lodeiro, A. R. (2013). Analysis of two polyhydroxyalkanoate synthases in Bradyrhizobium japonicum USDA 110. J. Bacteriol. 195, 3145–3155. doi: 10.1128/jb.02203-12
Regensburger, B., and Hennecke, H. (1983). RNA polymerase from Rhizobium japonicum. Arch. Microbiol. 135, 103–109. doi: 10.1007/BF00408017
Reutimann, L., Mesa, S., and Hennecke, H. (2010). Autoregulation of fixK2 gene expression in Bradyrhizobium japonicum. Mol. Genet. Genomics 284, 25–32. doi: 10.1007/s00438-010-0547-2
Richardson, D. J. (2011). “Redox complexes of the nitrogen cycle,” in Nitrogen Cycling in Bacteria, ed. J. W. B. Moir (Norkfolk, UK: Caister Academic Press), 23–39.
Robles, E. F., Sánchez, C., Bonnard, N., Delgado, M. J., and Bedmar, E. J. (2006). The Bradyrhizobium japonicum napEDABC genes are controlled by the FixLJ-FixK2-NnrR regulatory cascade. Biochem. Soc. Trans. 34, 108–110. doi: 10.1042/bst0340108
Rodionov, D. A., Dubchak, I. L., Arkin, A. P., Alm, E. J., and Gelfand, M. S. (2005). Dissimilatory metabolism of nitrogen oxides in bacteria: comparative reconstruction of transcriptional networks. PLoS Comput. Biol. 1:e55. doi: 10.1371/journal.pcbi.0010055
Schäfer, A., Tauch, A., Jager, W., Kalinowski, J., Thierbach, G., and Puhler, A. (1994). Small mobilizable multi-purpose cloning vectors derived from the Escherichia coli plasmids pK18 and pK19: selection of defined deletions in the chromosome of Corynebacterium glutamicum. Gene 145, 69–73. doi: 10.1016/0378-1119(94)90324-7
Sciotti, M. A., Chanfon, A., Hennecke, H., and Fischer, H. M. (2003). Disparate oxygen responsiveness of two regulatory cascades that control expression of symbiotic genes in Bradyrhizobium japonicum. J. Bacteriol. 185, 5639–5642. doi: 10.1128/JB.185.18.5639-5642.2003
Shapleigh, J. P. (2006). “The denitrifying prokaryotes”, in The Prokaryotes: A Handbook on the Biology of Bacteria, eds M. Dworkin, S. Flakow, E. Rosenberg, K. H. Schleifer, and E. Stackebrandt (New York, NY: Springer Science+Business Media), 769–792. doi: 10.1007/0-387-30742-7_23
Torres, M. J., Argandoña, M., Vargas, C., Bedmar, E. J., Fischer, H. M., Mesa, S., et al. (2014). The global response regulator RegR controls expression of denitrification genes in Bradyrhizobium japonicum. PLoS One 9:e99011. doi: 10.1371/journal.pone.0099011
Torres, M. J., Bueno, E., Jiménez-Leiva, A., Cabrera, J. J., Bedmar, E. J., Mesa, S., et al. (2017). FixK2 is the main transcriptional activator of Bradyrhizobium diazoefficiens nosRZDYFLX genes in response to low oxygen. Front. Microbiol. 8:1621. doi: 10.3389/fmicb.2017.01621
Torres, M. J., Simon, J., Rowley, G., Bedmar, E. J., Richardson, D. J., Gates, A. J., et al. (2016). Nitrous oxide metabolism in nitrate-reducing bacteria: physiology and regulatory mechanisms. Adv. Microb. Physiol. 68, 353–432. doi: 10.1016/bs.ampbs.2016.02.007
Tortosa, G., Hidalgo, A., Salas, A., Bedmar, E. J., Mesa, S., and Delgado, M. J. (2015). Nitrate and flooding induce N2O emissions from soybean nodules. Symbiosis 67, 125–133. doi: 10.1007/s13199-015-0341-3
Trunk, K., Benkert, B., Quack, N., Munch, R., Scheer, M., Garbe, J., et al. (2010). Anaerobic adaptation in Pseudomonas aeruginosa: definition of the Anr and Dnr regulons. Environ. Microbiol. 12, 1719–1733. doi: 10.1111/j.1462-2920.2010.02252.x
van Spanning, R. J. (2011). “Structure, function, regulation and evolution of the nitrite and nitrousoxide reductase: denitrification enzymes with a b-propeller fold,” in Nitrogen Cycling in Bacteria, ed. J. W. B. Moir (Norkfolk, UK: Caister Academic Press), 135–161.
van Spanning, R. J., Richardson, D. J., and Ferguson, S. J. (2007). “Introduction to the biochemistry and molecular biology of denitrification,” in Biology of the Nitrogen Cycle, eds H. Bothe, S. J. Ferguson, and W. E. Newton (Amsterdam: Elsevier Science), 3–20. doi: 10.1016/b978-044452857-5.50002-3
Vargas, C., Mcewan, A., and Downie, J. A. (1993). Detection of c-type cytochromes using enhanced chemiluminescence. Anal. Biochem. 209, 323–326. doi: 10.1006/abio.1993.1127
Velasco, L., Mesa, S., Delgado, M. J., and Bedmar, E. J. (2001). Characterization of the nirK gene encoding the respiratory, Cu-containing nitrite reductase of Bradyrhizobium japonicum. Biochim. Biophys. Acta 1521, 130–134. doi: 10.1016/S0167-4781(01)00279-2
Velasco, L., Mesa, S., Xu, C. A., Delgado, M. J., and Bedmar, E. J. (2004). Molecular characterization of nosRZDFYLX genes coding for denitrifying nitrous oxide reductase of Bradyrhizobium japonicum. Antonie Van Leeuwenhoek 85, 229–235. doi: 10.1023/B:ANTO.0000020156.42470.db
Keywords: CRP/FNR proteins, in vitro transcription, microoxia, nitrogen oxides, Rhizobia, transcriptomics
Citation: Jiménez-Leiva A, Cabrera JJ, Bueno E, Torres MJ, Salazar S, Bedmar EJ, Delgado MJ and Mesa S (2019) Expanding the Regulon of the Bradyrhizobium diazoefficiens NnrR Transcription Factor: New Insights Into the Denitrification Pathway. Front. Microbiol. 10:1926. doi: 10.3389/fmicb.2019.01926
Received: 26 April 2019; Accepted: 05 August 2019;
Published: 20 August 2019.
Edited by:
Rosa María Martínez-Espinosa, University of Alicante, SpainReviewed by:
Jose Berenguer, Autonomous University of Madrid, SpainCopyright © 2019 Jiménez-Leiva, Cabrera, Bueno, Torres, Salazar, Bedmar, Delgado and Mesa. This is an open-access article distributed under the terms of the Creative Commons Attribution License (CC BY). The use, distribution or reproduction in other forums is permitted, provided the original author(s) and the copyright owner(s) are credited and that the original publication in this journal is cited, in accordance with accepted academic practice. No use, distribution or reproduction is permitted which does not comply with these terms.
*Correspondence: María J. Delgado, bWFyaWFqZXN1cy5kZWxnYWRvQGVlei5jc2ljLmVz; Socorro Mesa, c29jb3Jyby5tZXNhQGVlei5jc2ljLmVz
†Present address: Emilio Bueno, Laboratory for Molecular Infection Medicine Sweden (MIMS), Department of Molecular Biology, Umeå University, Umeå, Sweden María J. Torres, Department of Biochemistry and Molecular Biology, University of Córdoba, Córdoba, Spain Sergio Salazar, Instituto de Medio Ambiente, Recursos Naturales y Biodiversidad (IRENA), Universidad de León, León, Spain
Disclaimer: All claims expressed in this article are solely those of the authors and do not necessarily represent those of their affiliated organizations, or those of the publisher, the editors and the reviewers. Any product that may be evaluated in this article or claim that may be made by its manufacturer is not guaranteed or endorsed by the publisher.
Research integrity at Frontiers
Learn more about the work of our research integrity team to safeguard the quality of each article we publish.