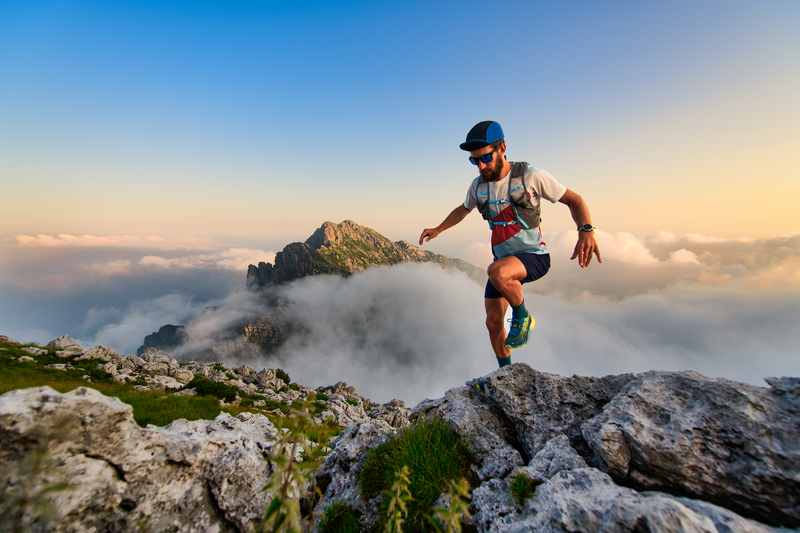
94% of researchers rate our articles as excellent or good
Learn more about the work of our research integrity team to safeguard the quality of each article we publish.
Find out more
MINI REVIEW article
Front. Microbiol. , 24 July 2019
Sec. Aquatic Microbiology
Volume 10 - 2019 | https://doi.org/10.3389/fmicb.2019.01715
The Prodiginine family consists of primarily red-pigmented tripyrrole secondary metabolites that were first characterized in the Gram-negative bacterial species Serratia marcescens and demonstrates a wide array of biological activities and applications. Derivatives of prodiginine have since been characterized in the marine γ-proteobacterium, Pseudoalteromonas. Although biosynthetic gene clusters involved in prodiginine synthesis display homology among genera, there is an evident structural difference in the resulting metabolites. This review will summarize prodiginine biosynthesis, bioactivity, and gene regulation in Pseudoalteromonas in comparison to the previously characterized species of Serratia, discuss the ecological contributions of Pseudoalteromonas in the marine microbiome and their eukaryotic hosts, and consider the importance of modern functional genomics and classic DNA manipulation to understand the overall prodiginine biosynthesis pathway.
The Prodiginine family consists of primarily red-pigmented secondary metabolites that are characterized by their tripyrrole structure. These metabolites are of interest in natural product research due to their wide array of biomedical and industrial applications including algicidal (Zhang et al., 2016), antibacterial (Okamoto et al., 1998), anticancer (Kawauchi et al., 2008), antimalarial (Kim et al., 1999), antiprotozoal (Azambuja et al., 2004), colorants (Alihosseini et al., 2008), immunosuppressive agents (Kawauchi et al., 2007), and insecticides (Suryawanshi et al., 2015). Prodiginine was first extracted from terrestrial bacterium, Serratia marcescens. It consisted of a straight alkyl chain substituent and was named prodigiosin (Rapoport and Holden, 1962). Prodigiosin is ubiquitous throughout various genera within the terrestrial and marine environment, suggesting that the metabolite may be ecologically advantageous to prodiginine-producing bacteria. Due to the diversity among prodiginine-producing bacteria, it is difficult to determine the precise biological and ecological role of the pigment. Based on previous studies, it can be inferred that prodiginine may provide a mode of defense against competing microorganisms or a potential response to environmental stressors (Gallardo et al., 2016).
Since the discovery of prodiginine, analogs have been isolated from marine bacteria, Pseudoalteromonas, including cycloprodigiosin and 2-(p-hydroxybenzyl) prodigiosin (Rapoport and Holden, 1962; Kawauchi et al., 1997; Fehér et al., 2008). Pseudoalteromonas has also been reported to produce tambjamines, a yellow pigment that is structurally like prodiginine, sharing two of the three pyrrole rings (Burke et al., 2007). The presence of pigmented metabolites like prodiginine and tambjamines in species of Pseudoalteromonas is particularly interesting because the genus is commonly associated with macroorganisms, such as algae (Dobretsov and Qian, 2002), fish (Pratheepa and Vasconcelos, 2013), sponges (Sakai-Kawada et al., 2016), and tunicates (Holmström et al., 1998). This host-microbe interaction provides the microorganism with nutrients to flourish, while providing the host with valuable resources that may contribute to nutrient cycling or defense (Webster and Taylor, 2012).
Many mechanisms for signaling and regulation of prodigiosin were identified in Serratia, however, none have been identified within Pseudoalteromonas. It was suggested that prodiginine biosynthesis may prevent primary metabolism overload by utilizing substrates like proline, serine, pyruvate, and NAD(P) (Wasserman et al., 1973; Drummond et al., 1995). PigP was characterized within the quorum sensing regulon as a master regulator of pig biosynthesis, controlling prodigiosin production by modulating pig regulators (Fineran et al., 2005b) Further studies characterized a metalloregulatory repressor (PigS) that responds to stress-induced presence of heavy metals (Gristwood et al., 2011). Independently, a GntR-homolog (PigT) was also identified (Fineran et al., 2005a). Due to the various means for regulation, it is uncertain if Pseudoalteromonas also utilize similar regulatory controls or if it adapted a genus-specific mechanism. Recent studies propose a LuxI/R quorum sensing system associated with pigment production in other Pseudoalteromonas species (Pan et al., 2016; Dang et al., 2017).
Although extensive studies were done to determine the bioactivity and chemical structure of these prodiginine analogs, the means of biological synthesis in Pseudoalteromonas remains elusive. To date, more than 200 Pseudoalteromonas genomes are sequenced and available on genome databases. Of those 200+ genomes, 9 genomes are associated with a prodiginine-producing species and only 3 belong to a tambjamine-producing species.
This review will focus on prodiginine classes that were characterized in Pseudoalteromonas, their bioactivity, and their biosynthesis. These ideas may provide further insight into the role prodiginines play in Pseudoalteromonas, their hosts, and the marine microbiome.
Pseudoalteromonas bacterial strains synthesize prodiginine analogs including prodigiosin, cycloprodigiosin, and 2-(p-hydroxybenzyl) prodigiosin (Figure 1A). These pigments possess a tripyrrole structure composed of two moieties: 2-methyl-3-n-amyl-pyrrole (MAP) and 4-methoxy-2,2′-bipyrrole-5-carbaldehyde (MBC). These analogs demonstrate antibacterial, anticancer, and immunosuppressive bioactivity and each analog exhibits its own specific bioactive properties. The specificity among these microbial pigments emphasizes their potential biomedical application.
Figure 1. (A) Prodiginine biosynthetic pathways: MAP biosynthesis (green), MBC biosynthesis (blue), prodigiosin, cPrG, and HBPG biosynthesis (red), enamine and tambjamine biosynthesis (yellow) (B) Mapping of homologous prodiginine biosynthetic gene clusters in Serratia marcescens (Sm39006), Pseudoalteromonas rubra (Pr6842), Pseudoalteromonas denitrificans (Pd6059), and Pseudoalteromonas tunicata (PtD2). The relationship between pigE and tamH is discussed in the Further insight via functional genomics and DNA manipulation (C) Mapping of homologous cyclizing oxygenases in Streptomyces coelicolor [ScA3(2)], Pseudoalteromonas rubra (Pr6842) and Pseudoalteromonas tunicata (PtD2).
Prodigiosin biosynthesis was first characterized in the γ-proteobacterium, Serratia marcescens and later characterized in Pseudoalteromonas rubra (Fehér et al., 2008; Vynne et al., 2011). This model defines a unidirectional polycistronic pig gene cluster consisting of two enzymatic pathways (Harris et al., 2004; Figure 1A).
The unique pathway involves MAP biosynthesis from 2-octenal (1) and is composed of genes pigB, pigD, and pigE. First, PigD, in the presence of coenzyme thiamine pyrophosphate (TPP), catalyzes the addition of pyruvate (2) to 2-octenal (1). The formation of 3-acetyloctanal (3) occurs with the release of CO2, followed by the transfer of an amino group to the aldehyde by PigE, and cyclization to form H2MAP (4). PigB catalyzes further oxidation to form MAP (5) (Goldschmidt and Williams, 1968; Harris et al., 2004; Williamson et al., 2005; Garneau-Tsodikova et al., 2006).
The common pathway in most prodiginine-producing microorganisms is MBC biosynthesis. This pathway is composed of seven genes (pigA, pigF-J, pigL, and pigM). 4′-phosphopantetheinyl transferase (PigL) activates peptidyl carrier protein (PCP) domain of PigG from apo to holo form through the addition of the 4′-phosphopantetheinyl group. PigI and ATP catalyze the transfer of the L-prolyl group of L-proline (6) to the thiol group of phosphopantetheine to form a L-prolyl-S-PCP intermediate (7). PigA oxidizes the intermediate to pyrrolyl-2-carboxyl-S-PCP (8). This proceeds with a polyketide-type chain extension, which starts with the transfer of the pyrrole-2-carboxyl group from PigG to the cysteine active site of PigJ, generating a pyrrole-2-carboxyl thioester (9). Separately, the ACP domains of PigH are phosphopantetheinylated, providing binding sites for the malonyl group of malonyl-CoA (10). The bound malonyl (11) then undergoes decarboxylation, resulting in a subsequent condensation with the pyrrole-2-carboxyl thioester attached to PigJ and forms pyrrolyl-β-ketothioester on PigH (12). PigH catalyzes another decarboxylation between serine and pyrrolyl-β-ketothioester to generate 4-hydroxy-2,2′-bipyrrole-5-methanol (HBM) (13) (Harris et al., 2004; Garneau-Tsodikova et al., 2006). The alcohol group of HBM is oxidized by PigM to make 4-hydroxy-2,2′-bipyrrole-5-carbaldehyde (HBC) (14). The final step of MBC (15) biosynthesis involves the methylation of the HBC hydroxyl group by methyltransferase (PigF) and oxidoreductase (PigN) (Feitelson and Hopwood, 1983; Williamson et al., 2005).
Once MAP (5) and MBC (15) are synthesized, PigC uses ATP to facilitate the terminal condensation of these two pyrroles to form prodigiosin (16). PigC has shown sequence similarity to an ATP-binding and phosphoryl transfer domains at the N- and C- terminus, respectively (Morrison, 1966; Williams, 1973).
Prodigiosin demonstrated a variety of biological activities, making it a candidate for pharmaceutical development. It exhibited activity against chloroquine-resistant strains of Plasmodium falciparum, which causes cerebral malaria (Singh and Shekhawat, 2012). Although previously considered to have selective activity against Gram-positive bacteria, prodigiosin has shown broad-spectrum antimicrobial activity against bacterial species including Bacillus subtilis, Escherichia coli, Enterobacter aerogenes, Enterococcus avium, Pseudomonas aeruginosa, Staphylococcus aureus, Staphylococcus saprophyticus, Streptococcus pyogenes and Candida albicans (Lapenda et al., 2014; Darshan and Manonmani, 2015; Danevčič et al., 2016a,b; Suryawanshi et al., 2017). Prodigiosin has a variety of mechanisms for antibacterial activity, including inducing autolysin production in B. cereus and inhibiting biofilm production in P. aeruginosa by stimulating the production of reactive oxygen species (ROS) (Danevčič et al., 2016a; Kimyon et al., 2016). Furthermore, prodigiosin demonstrated algicidal activity against Phaeocystis globola via influx of ROS, mitigating harmful algal blooms (Zhang et al., 2017).
Prodigiosin also presented toxigenic activity against chick embryos (Kalesperis et al., 1975) and exhibited cytotoxic activity via oxidative DNA cleavage, particularly against melanoma and liver cancer cells (Manderville, 2001). Additionally, prodigiosin induced apoptosis in human hematopoietic cancer cell lines including acute T-cell leukemia, promyelocytic leukemia, myeloma, Burkitt lymphoma cells, and B- and T-cells from B-cell chronic lymphocytic leukemia (Campàs et al., 2003). It also exhibited cytotoxicity against human gastric and colon cancer cells, and the V79 fibroblast cell line though a specific mechanism of action has yet to be determined (Da Silva Melo et al., 2000; Campàs et al., 2003). However, it does not exhibit genotoxicity, making it a candidate in pharmaceutical development (Guryanov et al., 2013). Trypanocidal activity was also characterized against protozoan Trypanosoma cruzi which causes Chagas disease (Da Silva Melo et al., 2000).
Furthermore, prodigiosin demonstrated selective immunosuppressive properties in the murine models (Tsuji et al., 1990). It was capable of suppressing T-cell proliferation without affecting interleukin-2, transferrin, and antibody expression or response. These selective properties make prodigiosin a prospective probe for the cytotoxic T-lymphocyte activation pathway.
Recently, prodiginine classes were characterized in the marine bacterium genus Pseudoalteromonas, specifically, Pseudoalteromonas denitrificans, Pseudoalteromonas rubra, and Pseudoalteromonas tunicata. Cycloprodigiosin (cPrG) (22) is a red-pigmented secondary metabolite that was isolated from the marine bacteria P. denitrificans and P. rubra (Kim et al., 1999; Xie et al., 2012). The key structural difference between cPrG and prodigiosin is the cyclization between the C-3 pentyl group and the C-4 carbon on pyrrole ring C of MAP (5).
Cyclic prodiginines and their respective oxygenase for cyclization were identified. In Streptomyces, redG and mcpG encode oxygenases that cyclize undecylprodiginine to form butyl-meta-cycloheptylprodiginine and metacycloprodigiosin, respectively (Kimata et al., 2017). Recent studies characterized a homologous gene (PRUB680), encoding an alkylglycerol monooxygenase-like protein away from the pig biosynthetic gene cluster (de Rond et al., 2017). This enzyme shows regiospecificity via C-H activation prompting the cyclization of prodigiosin and formation of cPrG (22).
It is still unclear what sort of advantages, if any, come with prodigiosin cyclization (de Rond et al., 2017). Whether this favorability is dependent on structural stability, heightened bioactivity, or a combination of both has yet to be determined.
cPrG showed higher antimalarial activity against Plasmodium berghei cells than chloroquine and its derivatives (Kim et al., 1999). Other cPrG analogs such as metacycloprodigiosin hydrochloride also demonstrated antimalarial activity against a multidrug-resistant strain of P. falciparum (Isaka et al., 2002). cPrG exhibited antimicrobial activity against S. aureus, E. coli, and C. albicans (Johnson et al., 2015; Offret et al., 2016).
cPrG was also characterized as an immunosuppressant through its ability to induce apoptosis of activated murine splenic T-cells, acute human T-cell leukemia, promyelocytic leukemia, human and rat hepatocellular cancer, human breast cancer, and TNF-stimulated human cervix carcinoma (Darshan and Manonmani, 2015).
2-(p-hydroxybenzyl) prodigiosin (HBPG) (23) was isolated from the marine bacterium P. rubra (Fehér et al., 2008; Offret et al., 2016). The structural difference between HBPG (23) and prodigiosin (16) is the para-addition of phenol to the C-10 carbon of pyrrole ring A of MBC (15), suggesting variations to the MBC pathway and homologs in the MAP pathway and terminal condensation step. Currently, there is no literature describing HBPG biosynthesis. Difficulties in characterization arise when biosynthetic genes are not clustered together, like that of the cPrG pathway. As shown by de Rond et al. (2017) elucidation of the complete biosynthetic pathway may rely on mining for homologous genes in other bacterial genera that function, similarly.
Due to HBPG’s exclusivity in only a few P. rubra strains, little information has been collected regarding its bioactivity. Like other prodiginines, HBPG demonstrated antimicrobial activity against S. aureus, methicillin-resistant S. aureus (MRSA), E. coli, and C. albicans (Fehér et al., 2008; Hu et al., 2016; Offret et al., 2016).
Cytotoxic activity of HBPG was also characterized against SKOV-3, a human ovarian adenocarcinoma cell line, via topoisomerase I inhibition. These results were indistinguishable from the prodigiosin control (Fehér et al., 2008). Additional bioassays will need to be conducted to uncover the significance of the p-hydroxylbenzyl group.
Pseudoalteromonas tunicata has yielded tambjamine, a yellow bipyrrolic bacterial pigment. Despite its divergence from the tripyrrolic structure, tambjamines were characterized to be structurally-related to prodiginines and share sequence homology to the pig biosynthetic pathway (Burke et al., 2007). Like the prodiginine family, tambjamine shares the MBC-moiety and contains the homologous genes responsible for its biosynthesis, however, it lacks the MAP-moiety, instead having an enamine group. Two types of tambjamines were characterized within P. tunicata – tambjamine and tambjamine YP1. The major difference between the two analogs is the substituent group attached to C-4: a methoxy group in tambjamine, where tambjamine YP1 has a methyl group (Franks et al., 2006).
Novel to the tam pathway is the enamine biosynthetic pathway. This pathway is composed of three characterized genes (tamT, tamH, afaA) and begins with dodecenoic acid (17). Until recently, afaA was thought to initiate the enamine biosynthesis (Burke et al., 2007). However, it was later determined that acyl CoA synthetase (TamA) is actually responsible for activating dodecenoic acid (17) (Marchetti et al., 2018). The activated fatty acid (18) undergoes oxidation by dehydrogenase (TamT), introducing a π-bond to C-3 carbon of the fatty acyl side chain (19). Reductase/aminotransferase (TamH) facilitates the reduction of the CoA-ester (19) and transamination to dodec-3-en-1-amine (20). TamQ facilitates the condensation of MBC (15) and enamine (20) to form tambjamine (21) (Burke et al., 2007).
Tambjamines exhibit antifungal, antifouling, and antimicrobial activity against S. aureus, E. coli, C. albicans, and Malassezia furfur (Holmström et al., 2002; Franks et al., 2006; Pinkerton et al., 2010). In addition, the production of tambjamine had inhibitory bioactivity against the bacterivorous nematode Caenorhabditis elegans (Ballestriero et al., 2010).
Since the characterization of the tam pathway, tambjamines analogs were chemically synthesized to explore the antimicrobial and cytotoxic properties associated with the substitution of various alkyl and bromo groups (Pinkerton et al., 2010). Synthetic tambjamine analogs that contain aromatic enamine groups demonstrate efficient transmembrane anion transportation of chloride and bicarbonate (Hernando et al., 2014). These cytotoxic transport properties are a promising treatment of cancer cells. Unlike normal tissue cells, cancer cells contain a reversed pH gradient with alkaline intracellular and acidic extracellular conditions. These conditions provide tumor cells protection from acid-induced apoptosis, allowing them to proliferate (Castaing et al., 2001; Sharma, 2015). Introducing highly effective anion-selective ionophores, like tambjamine, can regulate cellular ion homeostasis by affecting the intracellular pH, triggering mitochondrial dysfunction and lysosomal deacidification, leading to necrosis in lung cancer cell lines and cancer stem cells (Rodilla et al., 2001).
Recent literature suggests that the pig pathway can undergo horizontal gene transfer, which may explain the homology between prodigiosin gene clusters in Serratia and Pseudoalteromonas (Coulhurst et al., 2006; Williamson et al., 2006; Figure 1B). Although Serratia is primarily terrestrial, the genus’s presence in marine and aquatic environments has been reported and may be substantial enough to interact with the exclusively marine Pseudoalteromonas (Joyner et al., 2014). The versatile bioactivity of prodiginines implies an integral role in outcompeting other microorganisms for valuable resources.
Furthermore, a majority of the Pseudoalteromonas species are associated with eukaryotic hosts (Holmström and Kjelleberg, 1999). From the three species described, P. denitrificans and P. rubra were isolated from seawater samples, while P. tunicata was characterized in marine algae and tunicates (Bowman, 2007). There is a close association between pigmented Pseudoalteromonas species and marine macroorganisms (Zheng et al., 2006; Sakai-Kawada et al., 2016). One study isolated P. tunicata from a healthy adult tunicate and Ulva lactuca, a common green alga; both hosts did not independently produce antifouling compounds. P. tunicata produced these bioactive compounds, preventing colonization of algal spores, marine invertebrate larvae, protozoa, bacteria, and fungi, supporting the hypothesis that Pseudoalteromonas species may act as a chemical defense mechanism for its associated host (Holmström et al., 2002; Franks et al., 2006). Although the Pseudoalteromonas genus is associated with a wide array of bioactivities that may provide protection for their respective hosts, difficulties still arise when determining the precise ecological role, they play.
With current breakthroughs in functional genomics, one can now identify homologous prodiginine biosynthetic gene clusters within Pseudoalteromonas to characterize the pathway. 9 of the 200+ Pseudoalteromonas genomes submitted on NCBI are known to synthesize prodiginines. Of the 9 genomes, 8 belong to P. rubra and only 1 to P. denitrificans. Genome sequencing and prodiginine biosynthesis-directed annotation can provide more insight into homologous pathways (Figure 1B and Table 1). Preliminary genome analysis in both Pseudoalteromonas species revealed a gene cluster homologous to the prodiginine biosynthetic gene cluster in Serratia. P. rubra’s gene cluster averaged 63% homology to Serratia, while P. denitrificans averaged 54% (Xie et al., 2012; Li et al., 2016). Although P. tunicata’s tambjamine biosynthetic gene cluster shares a similar MBC pathway with prodiginine biosynthesis in other Pseudoalteromonas species, it showed low identity averaging 46% homology (Burke et al., 2007). However, sequence homology extended beyond the MBC pathway. Putative aminotransferase (tamH) responsible for enamine biosynthesis in P. tunicata showed 40% identity to an aminotransferase (pigE) responsible for MAP biosynthesis in Serratia and the other two Pseudoalteromonas species (Burke et al., 2007).
Table 1. Predicted function of genes involved in prodiginine and tambjamine biosynthesis and their homology among Serratia marcescens (Sm39006), Pseudoalteromonas rubra (Pr6842), Pseudoalteromonas denitrificans (Pd6059), and Pseudoalteromonas tunicata (PtD2).
As previously discussed, there are limitations to relying solely on functional genomics. Genes outside of the primary gene cluster, as demonstrated with PRUB680 in P. rubra, play a key role in both regulating and biosynthesizing prodiginine analogs. However, PRUB680 showed low sequence homology to redG in Streptomyces at 46% (Figure 1C). Although genome sequencing and rapid annotation via localized alignment tools are useful for characterizing numerous biosynthetic gene clusters, proper genetic manipulation of each individual ORF is necessary to confirm function. As previously discussed, Marchetti et al. (2018). demonstrated that tamA was responsible for the initiation of enamine biosynthesis, further feeding into tambjamine biosynthesis. That is not to say that afaA does not still play a role in tambjamine biosynthesis.
Several molecular tools have been used to manipulate Pseudoalteromonas’ genome and explore their numerous biological pathways. Egan et al. (2002) and Huang et al. (2012) both proved that the Pseudoalteromonas genome can be manipulated via Tn10 random mutagenesis, however, this method requires screening hundreds to thousands of mutants before isolating one that disrupts the gene of interest. de Rond et al. (2017) and Wang et al. (2015) utilized a site-directed approach in manipulating P. rubra’s genome via suicide and expression shuttle vectors, respectively. Recently, Pseudoalteromonas phages and prophages belonging to the myoviridae, podoviridae, and siphoviridae families have been isolated and sequenced several instances Duhaime et al., 2017. characterized a Mu-type sipho-myo hybrid capable of incorporating itself into the host genome as well as several phages containing proteins that can control host stress response. Given the proper delivery system, these bacteriophages could prove to be powerful tools for DNA manipulation within Pseudoalteromonas.
In conclusion, a more holistic approach to understanding bioactivity and gene regulation will lay the foundation for answering overarching questions concerning pigmented Pseudoalteromonas species such as their ecological and overall roles within the marine microbiome.
FS-K and JA contributed to the conception of the focus for the study. FS-K contributed to the compilation of all sections, figure and table design, and wrote the first draft of the manuscript. CI performed the compilation of biological activity, ecological and evolutionary significance sections. KH contributed to the biosynthetic pathways and biological activity sections. All authors contributed to revision, read and approved the submitted version of the manuscript.
This research was supported by the United States National Science Foundation grant HRD 0833211.
The authors declare that the research was conducted in the absence of any commercial or financial relationships that could be construed as a potential conflict of interest.
Alihosseini, F., Ju, K. S., Lango, J., Hammock, B. D., and Sun, G. (2008). Antibacterial colorants: characterization of prodiginines and their applications on textile materials. Biotechnol. Prog. 24, 742–747. doi: 10.1021/bp070481r
Azambuja, P., Feder, D., and Garcia, E. S. (2004). Isolation of Serratia marcescens in the midgut of Rhodnius prolixus: impact on the establishment of the parasite Trypanosoma cruzi in the vector. Exp. Parasitol. 107, 89–96. doi: 10.1016/j.exppara.2004.04.007
Ballestriero, F., Thomas, T., Burke, C., Egan, S., and Kjelleberg, S. (2010). Identification of compounds with bioactivity against the nematode Caenorhabditis elegans by a screen based on the functional genomics of the marine bacterium Pseudoalteromonas tunicata D2. Appl. Environ. Microbiol. 76, 5710–5717. doi: 10.1128/AEM.00695-10
Bowman, J. P. (2007). Bioactive compound synthetic capacity and ecological significance of marine bacterial genus Pseudoalteromonas. Mar. Drugs 5, 220–241. doi: 10.3390/md20070017
Burke, C., Thomas, T., Egan, S., and Kjelleberg, S. (2007). The use of functional genomics for the identification of a gene cluster encoding for the biosynthesis of an antifungal tambjamine in the marine bacterium Pseudoalteromonas tunicata: brief report. Environ. Microbiol. 9, 814–818. doi: 10.1111/j.1462-2920.2006.01177.x
Campàs, C., Dalmau, M., Montaner, B., Barragán, M., Bellosillo, B., Colomer, D., et al. (2003). Prodigiosin induces apoptosis of B and T cells from B-cell chronic lymphocytic leukemia. Leukemia 17, 746–750. doi: 10.1038/sj.leu.2402860
Castaing, M., Loiseau, A., and Dani, M. (2001). Designing multidrug-resistance modulators circumventing the reverse pH gradient in tumours. J. Pharm. Pharmacol. 53, 1021–1028. doi: 10.1211/0022357011776270
Coulhurst, S. J., Williamson, N. R., Harris, A. K. P., Spring, D. R., and Salmond, G. P. C. (2006). Metabolic and regulatory engineering of Serratia marcescens: mimicking phage-mediated horizontal acquisition of antibiotic biosynthesis and quorum-sensing capacities. Microbiology 152, 1899–1911. doi: 10.1099/mic.0.28803-0
Da Silva Melo, P., Durán, N., and Haun, M. (2000). Cytotoxicity of prodigiosin and benznidazole on V79 cells. Toxicol. Lett. 116, 237–242. doi: 10.1016/S0378-4274(00)00226-225
Danevčič, T., Vezjak, M. B., Tabor, M., Zorec, M., and Stopar, D. (2016a). Prodigiosin induces autolysins in actively grown Bacillus subtilis cells. Front. Microbiol. 7:27. doi: 10.3389/fmicb.2016.00027
Danevčič, T., Vezjak, M. B., Zorec, M., and Stopar, D. (2016b). Prodigiosin - A multifaceted Escherichia coli antimicrobial agent. PLoS One 11:e0162412. doi: 10.1371/journal.pone.0162412
Dang, H. T., Komatsu, S., Masuda, H., and Enomoto, K. (2017). Characterization of luxi and luxr protein homologs of n-acylhomoserine lactone-dependent quorum sensing system in Pseudoalteromonas sp. 520P1. Mar. Biotechnol. 19, 1–10. doi: 10.1007/s10126-016-9726-4
Darshan, N., and Manonmani, H. K. (2015). Prodigiosin and its potential applications. J. Food Sci. Technol. 52, 5393–5407. doi: 10.1007/s13197-015-1740-4
de Rond, T., Stow, P., Eigl, I., Johnson, R. E., Chan, L. J. G., Goyal, G., et al. (2017). Oxidative cyclization of prodigiosin by an alkylglycerol monooxygenase-like enzyme. Nat. Chem. Biol. 13, 1155–1159. doi: 10.1038/nchembio.2471
Dobretsov, S. V., and Qian, P.-Y. (2002). Effect of bacteria associated with the green alga ulva reticulata on marine micro- and macrofouling. Biofouling 18, 217–228. doi: 10.1080/08927010290013026
Drummond, D., Smith, S., Wood, N. J., and Hodgson, D. A. (1995). Interaction between primary and secondary metabolism in Streptomyces coelicolor A3(2): role of pyrroline-5-carboxylate dehydrogenase. Microbiology 141, 1739–1744. doi: 10.1099/13500872-141-7-1739
Duhaime, M. B., Solonenko, N., Roux, S., Verberkmoes, N. C., Wichels, A., and Sullivan, M. B. (2017). Comparative omics and trait analyses of marine Pseudoalteromonas phages advance the phage OTU concept. Front. Microbiol. 8:124. doi: 10.3389/fmicb.2017.01241
Egan, S., James, S., and Kjelleberg, S. (2002). Identification and characterization of a putative transcriptional regulator controlling the expression of fouling inhibitors in Pseudoalteromonas tunicata. Appl. Environ. Microbiol. 68, 372–378. doi: 10.1128/AEM.68.1.372
Fehér, D., Barlow, R. S., Lorenzo, P. S., and Hemscheidt, T. K. (2008). A 2-Substituted Prodiginine, 2-(p-Hydroxybenzyl)prodigiosin, from Pseudoalteromonas rubra. J. Nat. Prod. 71, 1970–1972. doi: 10.1038/nrm2621
Feitelson, J., and Hopwood, D. (1983). Cloning of a streptomyces gene for an O-methyltransferase involved in antibiotic biosynthesis. Mol. Gen. Genet. MGG 190, 394–398. doi: 10.1007/BF00331065
Fineran, P. C., Everson, L., Slater, H., and Salmond, G. P. C. (2005a). A GntR family transcriptional regulator (PigT) controls gluconate-mediated repression and defines a new, independent pathway for regulation of the tripyrrole antibiotic, prodigiosin, in Serratia. Microbiology 151, 3833–3845. doi: 10.1099/mic.0.28251-0
Fineran, P. C., Slater, H., Everson, L., Hughes, K., and Salmond, G. P. C. (2005b). Biosynthesis of tripyrrole and β-lactam secondary metabolites in Serratia: integration of quorum sensing with multiple new regulatory components in the control of prodigiosin and carbapenem antibiotic production. Mol. Microbiol. 56, 1495–1517. doi: 10.1111/j.1365-2958.2005.04660.x
Franks, A., Egan, S., Holmström, C., James, S., Lappin-Scott, H., and Kjelleberg, S. (2006). Inhibition of fungal colonization by Pseudoalteromonas tunicata provides a competitive advantage during surface colonization. Appl. Environ. Microbiol. 72, 6079–6087. doi: 10.1128/AEM.00559-06
Gallardo, K., Candia, J. E., Remonsellez, F., Escudero, L. V., and Demergasso, C. S. (2016). The ecological coherence of temperature and salinity tolerance interaction and pigmentation in a non-marine Vibrio isolated from salar de atacama. Front. Microbiol. 7:1943. doi: 10.3389/fmicb.2016.01943
Garneau-Tsodikova, S., Dorrestein, P. C., Kelleher, N. L., and Walsh, C. T. (2006). Protein assembly line components in prodigiosin biosynthesis: characterization of PigA,G,H,I,J. J. Am. Chem. Soc. 128, 12600–12601. doi: 10.1021/ja063611l
Goldschmidt, M. C., and Williams, R. P. (1968). Thiamine-induced formation of the monopyrrole moiety of prodigiosin. J. Bacteriol. 96, 609–616.
Gristwood, T., McNeil, M. B., Clulow, J. S., Salmond, G. P. C., and Fineran, P. C. (2011). PigS and PigP regulate prodigiosin biosynthesis in Serratia via differential control of divergent operons, which include predicted transporters of sulfur-containing molecules. J. Bacteriol. 193, 1076–1085. doi: 10.1128/JB.00352-10
Guryanov, I. D., Karamova, N. S., Yusupova, D. V., Gnezdilov, O. I., and Koshkarova, L. A. (2013). Bacterial pigment prodigiosin and its genotoxic effect. Russ. J. Bioorganic Chem. 39, 106–111. doi: 10.1134/S1068162012060040
Harris, A. K. P., Williamson, N. R., Slater, H., Cox, A., Abbasi, S., Foulds, I., et al. (2004). The Serratia gene cluster encoding biosynthesis of the red antibiotic, prodigiosin, shows species- and strain-dependent genome context variation. Microbiology 150, 3547–3560. doi: 10.1099/mic.0.27222-0
Hernando, E., Soto-Cerrato, V., Cortés-Arroyo, S., Pérez-Tomás, R., and Quesada, R. (2014). Transmembrane anion transport and cytotoxicity of synthetic tambjamine analogs. Org. Biomol. Chem. 12, 1771–1778. doi: 10.1039/C3OB42341G
Holmström, C., Egan, S., Franks, A., McCloy, S., and Kjelleberg, S. (2002). Antifouling activities expressed by marine surface associated Pseudoalteromonas species. FEMS Microbiol. Ecol. 41, 47–58. doi: 10.1016/S0168-6496(02)00239-8
Holmström, C., James, S., Neilan, B. A., White, D. C., and Kjelleberg, S. (1998). Pseudoalteromonas tunicata sp. nov., a bacterium that produces antifouling agents. Int. J. Syst. Bacteriol. 48, 1205–1212. doi: 10.1099/00207713-48-4-1205
Holmström, C., and Kjelleberg, S. (1999). Marine Pseudoalteromonas species are associated with higher organisms and produce biologically active extracellular agents. FEMS Microb. Ecol. 30, 285–293. doi: 10.1111/j.1574-6941.1999.tb00656.x
Hu, D. X., Withall, D. M., Challis, G. L., and Thomson, R. J. (2016). Structure, chemical synthesis, and biosynthesis of prodiginine natural products. Chem. Rev. 116, 7818–7853. doi: 10.1021/acs.chemrev.6b00024
Huang, Y., Callahan, S., and Hadfield, M. G. (2012). Recruitment in the sea: bacterial genes required for inducing larval settlement in a polychaete worm. Sci. Rep. 2:228. doi: 10.1038/srep00228
Isaka, M., Jaturapat, A., Kramyu, J., Tanticharoen, M., and Thebtaranonth, Y. (2002). Potent in vitro antimalarial activity of metacycloprodigiosin isolated from Streptomyces spectabilis BCC 4785. Antimicrob. Agents Chemother. 46, 1112–1113. doi: 10.1128/AAC.46.4.1112-1113.2002
Johnson, R. E., De Rond, T., Lindsay, V. N. G., Keasling, J. D., and Sarpong, R. (2015). Synthesis of cycloprodigiosin identifies the natural isolate as a scalemic mixture. Org. Lett. 17, 3474–3477. doi: 10.1021/acs.orglett.5b01527
Joyner, J., Wanless, D., Sinigalliano, C. D., and Lipp, E. K. (2014). Use of quantitative real-time PCR for direct detection of Serratia marcescens in marine and other aquatic environments. Appl. Environ. Microbiol. 80, 1679–1683. doi: 10.1128/AEM.02755-13
Kalesperis, G. S., Prahlad, K. V., and Lynch, D. L. (1975). Toxigenic studies with the antibiotic pigments from Serratia marcescens. Can. J. Microbiol. 21, 213–220. doi: 10.1139/m75-030
Kawauchi, K., Shibutani, K., Yagisawa, H., Kamata, H., Nakatsuji, S., Anzai, H., et al. (1997). A possible immunosuppressant, cycloprodigiosin hydrochloride, obtained from Pseudoalteromonas denitrificans. Biochem. Biophys. Res. Commun. 237, 543–547. doi: 10.1006/bbrc.1997.7186
Kawauchi, K., Tobiume, K., Iwashita, K., Inagaki, H., Morikawa, T., Shibukawa, Y., et al. (2008). Cycloprodigiosin hydrochloride activates the Ras-PI3K-Akt pathway and suppresses protein synthesis inhibition-induced apoptosis in PC12 cells. Biosci. Biot. Echnol. Biochem. Biotechnol. Biochem. 72, 1564–1570. doi: 10.1271/bbb.80064
Kawauchi, K., Tobiume, K., Kaneko, S., Kaneshiro, K., Okamoto, S., Ueda, E., et al. (2007). Suppression of AP-1 activity by cycloprodigiosin hydrochloride. Biol. Pharm. Bull. 30, 1792–1795. doi: 10.1248/bpb.30.1792
Kim, H.-S., Hayashi, M., Shibata, Y., Wataya, Y., Mitamura, T., Horii, T., et al. (1999). Cycloprodigiosin hydrochloride obtained from Pseudoalteromonas denitrificans is a potent antimalarial agent. Biol. Pharm. Bull. 22, 532–534. doi: 10.1248/bpb.22.532
Kimata, S., Izawa, M., Kawasaki, T., and Hayakawa, Y. (2017). Identification of a prodigiosin cyclization gene in the roseophilin producer and production of a new cyclized prodigiosin in a heterologous host. J. Antibiot. 70, 196–199. doi: 10.1038/ja.2016.94
Kimyon,Ö, Das, T., Ibugo, A. I, Kutty, S. K., Ho, K. K., Tebben, J., et al. (2016). Serratia secondary metabolite prodigiosin inhibits Pseudomonas aeruginosa biofilm development by producing reactive oxygen species that damage biological molecules. Front. Microbiol. 7:972. doi: 10.3389/fmicb.2016.00972
Lapenda, J. C., Silva, P. A., Vicalvi, M. C., Sena, K. X. F. R., and Nascimento, S. C. (2014). Antimicrobial activity of prodigiosin isolated from Serratia marcescens UFPEDA 398. World J. Microbiol. Biotechnol. 31, 399–406. doi: 10.1007/s11274-014-1793-y
Li, B., Wang, P., Zeng, Z., Cai, X., Wang, G., and Wang, X. (2016). Complete genome sequence of Pseudoalteromonas rubra SCSIO 6842, harboring a putative conjugative plasmid pMBL6842. J. Biotechnol. 224, 66–67. doi: 10.1016/j.jbiotec.2016.03.010
Manderville, R. A. (2001). Synthesis, proton-affinity and anti-cancer properties of the prodigiosin-group natural products. Curr. Med. Chem. 1, 195–218. doi: 10.2174/1568011013354688
Marchetti, P. M., Kelly, V., Simpson, J. P., Ward, M., and Campopiano, D. J. (2018). The carbon chain-selective adenylation enzyme TamA: the missing link between fatty acid and pyrrole natural product biosynthesis. Org. Biomol. Chem. 16, 2735–2740. doi: 10.1039/c8ob00441b
Morrison, D. A. (1966). Prodigiosin synthesis in mutants of Serratia marcesens. J. Bacteriol. 91, 1599–1604.
Offret, C., Desriac, F., Le Chevalier, P., Mounier, J., Jégou, C., and Fleury, Y. (2016). Spotlight on antimicrobial metabolites from the marine bacteria Pseudoalteromonas: chemodiversity and ecological significance. Mar. Drugs 14:E129. doi: 10.3390/md14070129
Okamoto, H., Sato, Z., Sato, M., Koiso, Y., Iwasaki, S., and Isaki, M. (1998). Identification of Antibiotic red pigments of Serratia marcescens F-1-1, a biocontrol agent of damping-off of cucumber, and antimicrobial activity against other plant pathogens. Japanese J. Phytopathol. 64, 294–298. doi: 10.3186/jjphytopath.64.294
Pan, Y., Wang, Y., Yan, X., Mazumder, A., and Liang, Y. (2016). Draft genome sequence of Pseudoalteromonas tetraodonis Strain MQS005, a bacterium with potential quorum-sensing regulation. Genome Announc 4, e724–e716. doi: 10.1128/genomeA.00724-16
Pinkerton, D. M., Banwell, M. G., Garson, M. J., Kumar, N., De Moraes, M. O., Cavalcanti, B. C., et al. (2010). Antimicrobial and cytotoxic activities of synthetically derived tambjamines C and E-J, BE-18591, and a related alkaloid from the marine bacterium Pseudoalteromonas tunicata. Chem. Biodivers. 7, 1311–1324. doi: 10.1002/cbdv.201000030
Pratheepa, V., and Vasconcelos, V. (2013). Microbial diversity associated with tetrodotoxin production in marine organisms. Environ. Toxicol. Pharmacol. 36, 1046–1054. doi: 10.1016/j.etap.2013.08.013
Rapoport, H., and Holden, K. G. (1962). The synthesis of prodigiosin. J. Am. Chem. Soc. 84, 635–642. doi: 10.1071/CH9732065
Rodilla, A. M., Korrodi-Gregório, L., Hernando, E., Manuel-Manresa, P., Quesada, R., Pérez-Tomás, R., et al. (2001). Synthesis, proton-affinity and anti-cancer properties of the prodigiosin-group natural products. Curr. Med. Chem. 195–218. doi: 10.1016/j.bcp.2016.11.022
Sakai-Kawada, F. E., Yakym, C. J., Helmkampf, M., Hagiwara, K., Ip, C. G., Antonio, B. J., et al. (2016). Draft genome sequence of marine sponge symbiont Pseudoalteromonas luteoviolacea IPB1. Isolated from Hilo, Hawaii. Genome Announc. 4, e1002–e1016. doi: 10.1128/genomeA.01002-16
Sharma, M. (2015). pH gradient reversal: an emerging hallmark of cancers. Recent Pat. Anticancer. Drug Discov. 10, 244–258. doi: 10.2174/1574892810666150708110608
Singh, P., and Shekhawat, N. (2012). Chemometric descriptors in the rationale of antimalarial activity of natural and synthetic prodiginines. J. Curr. Chem. Pharm. Sci. 2, 244–260.
Suryawanshi, R. K., Patil, C. D., Borase, H. P., Narkhede, C. P., Salunke, B. K., and Patil, S. V. (2015). Mosquito larvicidal and pupaecidal potential of prodigiosin from Serratia marcescens and understanding its mechanism of action. Pestic. Biochem. Physiol. 123, 49–55. doi: 10.1016/j.pestbp.2015.01.018
Suryawanshi, R. K., Patil, C. D., Koli, S. H., Hallsworth, J. E., and Patil, S. V. (2017). Antimicrobial activity of prodigiosin is attributable to plasma-membrane damage. Nat. Prod. Res. 31, 572–577. doi: 10.1080/14786419.2016.1195380
Tsuji, R. F., Yamamoto, M., Nakamura, A., Kataoka, T., Magae, J., Nagai, K., et al. (1990). Selective immunosuppresion of Prodigiosin 25-C and FK506 in the Murine Immune System. J. Antibiot. 43, 1293–1301. doi: 10.7164/antibiotics.43.1293
Vynne, N. G., Månsson, M., Nielsen, K. F., and Gram, L. (2011). Bioactivity, chemical profiling, and 16S rRNA-Based Phylogeny of Pseudoalteromonas strains collected on a global research cruise. Mar. Biotechnol. 13, 1062–1073. doi: 10.1007/s10126-011-9369-4
Wang, P., Yu, Z., Li, B., Cai, X., Zeng, Z., Chen, X., et al. (2015). Development of an efficient conjugation-based genetic manipulation system for Pseudoalteromonas. Microb. Cell Fact. 14:11. doi: 10.1186/s12934-015-0194-8
Wasserman, H., Sykes, R. J., Peverada, P., Shaw, C. K., Cushley, R. J., and Lipsky, S. R. (1973). Biosynthesis of prodigiosin. incorporation patterns of 13C-Labeled alanine, proline, glycine, and serine elucidated by fourier transform nuclear magnetic resonance. J. Am. Chem. Soc. 95, 6874–6875. doi: 10.1021/ja00801a080
Webster, N. S., and Taylor, M. W. (2012). Marine sponges and their microbial symbionts: love and other relationships. Environ. Microbiol. 14, 335–346. doi: 10.1111/j.1462-2920.2011.02460.x
Williams, R. P. (1973). Biosynthesis of prodigiosin, a secondary metabolite of Serratia marcescens. Appl. Microbiol. 25, 396–402.
Williamson, N. R., Simonsen, H. T., Ahmed, R. A. A., Goldet, G., Slater, H., Woodley, L., et al. (2005). Biosynthesis of the red antibiotic, prodigiosin, in Serratia: identification of a novel 2-methyl-3-n-amyl-pyrroie (MAP) assembly pathway, definition of the terminal condensing enzyme, and implications for undecylprodigiosin biosynthesis in Streptomyces. Mol. Microbiol. 56, 971–989. doi: 10.1111/j.1365-2958.2005.04602.x
Williamson, N. R., Simonsen, H. T., Harris, A. K. P., Leeper, F. J., and Salmond, G. P. C. (2006). Disruption of the copper efflux pump (CopA) of Serratia marcescens ATCC 274 pleiotropically affects copper sensitivity and production of the tripyrrole secondary metabolite, prodigiosin. J. Ind. Microbiol. Biotechnol. 33, 151–158. doi: 10.1007/s10295-005-0040-9
Xie, B.-B., Shu, Y.-L., Qin, Q.-L., Rong, J.-C., Zhang, X.-Y., Chen, X.-L., et al. (2012). Genome sequence of the cycloprodigiosin-producing bacterial strain Pseudoalteromonas rubra ATCC 29570 T. J. Bacteriol. 194, 1637–1638. doi: 10.1128/JB.06822-11
Zhang, H., Peng, Y., Zhang, S., Cai, G., Li, Y., Yang, X., et al. (2016). Algicidal effects of prodigiosin on the harmful algae Phaeocystis globosa. Front. Microbiol. 7:602. doi: 10.3389/fmicb.2016.00602
Zhang, H., Wang, H., Zheng, W., Yao, Z., Peng, Y., Zhang, S., et al. (2017). Toxic effects of prodigiosin secreted by Hahella sp. KA22 on harmful alga Phaeocystis globosa. Front. Microbiol. 8, 1–12. doi: 10.3389/fmicb.2017.00999
Zheng, L., Yan, X., Han, X., Chen, H., Lin, W., Lee, F. S. C., et al. (2006). Identification of norharman as the cytotoxic compound produced by the sponge (Hymeniacidon perleve)-associated marine bacterium Pseudoalteromonas piscicida and its apoptotic effect on cancer cells. Biotechnol. Appl. Biochem. 44, 135–142. doi: 10.1042/BA20050176
Keywords: Pseudoalteromonas, prodiginines, prodigiosin, secondary metabolites, pigments, marine bacteria
Citation: Sakai-Kawada FE, Ip CG, Hagiwara KA and Awaya JD (2019) Biosynthesis and Bioactivity of Prodiginine Analogs in Marine Bacteria, Pseudoalteromonas: A Mini Review. Front. Microbiol. 10:1715. doi: 10.3389/fmicb.2019.01715
Received: 05 February 2019; Accepted: 11 July 2019;
Published: 24 July 2019.
Edited by:
Marcelino T. Suzuki, Sorbonne Universités, FranceReviewed by:
Christopher Morton Thomas, University of Birmingham, United KingdomCopyright © 2019 Sakai-Kawada, Ip, Hagiwara and Awaya. This is an open-access article distributed under the terms of the Creative Commons Attribution License (CC BY). The use, distribution or reproduction in other forums is permitted, provided the original author(s) and the copyright owner(s) are credited and that the original publication in this journal is cited, in accordance with accepted academic practice. No use, distribution or reproduction is permitted which does not comply with these terms.
*Correspondence: Francis E. Sakai-Kawada, sakai3@hawaii.edu
Disclaimer: All claims expressed in this article are solely those of the authors and do not necessarily represent those of their affiliated organizations, or those of the publisher, the editors and the reviewers. Any product that may be evaluated in this article or claim that may be made by its manufacturer is not guaranteed or endorsed by the publisher.
Research integrity at Frontiers
Learn more about the work of our research integrity team to safeguard the quality of each article we publish.