- 1Department of Microbiology, Faculty of Science and Informatics, University of Szeged, Szeged, Hungary
- 2Department of General and Environmental Microbiology, Faculty of Sciences, and Szentágothai Research Center, University of Pécs, Pécs, Hungary
- 3Department of Plant Biology, Faculty of Science and Informatics, University of Szeged, Szeged, Hungary
- 4Department of Civil Engineering, Aalto University, Espoo, Finland
- 5Research Area Biochemical Technology, Institute of Chemical, Environmental and Bioscience Engineering, TU Wien, Vienna, Austria
- 6Jiangsu Provincial Key Laboratory of Organic Solid Waste Utilization, Nanjing Agricultural University, Nanjing, China
This study examined the structural diversity and bioactivity of peptaibol compounds produced by species from the phylogenetically separated Longibrachiatum Clade of the filamentous fungal genus Trichoderma, which contains several biotechnologically, agriculturally and clinically important species. HPLC-ESI-MS investigations of crude extracts from 17 species of the Longibrachiatum Clade (T. aethiopicum, T. andinense, T. capillare, T. citrinoviride, T. effusum, T. flagellatum, T. ghanense, T. konilangbra, T. longibrachiatum, T. novae-zelandiae, T. pinnatum, T. parareesei, T. pseudokoningii, T. reesei, T. saturnisporum, T. sinensis, and T. orientale) revealed several new and recurrent 20-residue peptaibols related to trichobrachins, paracelsins, suzukacillins, saturnisporins, trichoaureocins, trichocellins, longibrachins, hyporientalins, trichokonins, trilongins, metanicins, trichosporins, gliodeliquescins, alamethicins and hypophellins, as well as eight 19-residue sequences from a new subfamily of peptaibols named brevicelsins. Non-ribosomal peptide synthetase genes were mined from the available genome sequences of the Longibrachiatum Clade. Their annotation and product prediction were performed in silico and revealed full agreement in 11 out of 20 positions regarding the amino acids predicted based on the signature sequences and the detected amino acids incorporated. Molecular dynamics simulations were performed for structural characterization of four selected peptaibol sequences: paracelsins B, H and their 19-residue counterparts brevicelsins I and IV. Loss of position R6 in brevicelsins resulted in smaller helical structures with higher atomic fluctuation for every residue than the structures formed by paracelsins. We observed the formation of highly bent, almost hairpin-like, helical structures throughout the trajectory, along with linear conformation. Bioactivity tests were performed on the purified peptaibol extract of T. reesei on clinically and phytopathologically important filamentous fungi, mammalian cells, and Arabidopsis thaliana seedlings. Porcine kidney cells and boar spermatozoa proved to be sensitive to the purified peptaibol extract. Peptaibol concentrations ≥0.3 mg ml−1 deterred the growth of A. thaliana. However, negative effects to plants were not detected at concentrations below 0.1 mg ml−1, which could still inhibit plant pathogenic filamentous fungi, suggesting that those peptaibols reported here may have applications for plant protection.
Introduction
At present, more than 300 species of the genus Trichoderma (Ascomycota, Hypocreales, Hypocreaceae) have been described (Bissett et al., 2015; Zhang and Zhuang, 2018). The majority of these species were described after the year 2000, as only a few species were initially included in the genus (Bisby, 1939; Rifai, 1969). Section Longibrachiatum of the genus was one of the five Trichoderma sections according to Bissett (1984, 1991a,b,c). It forms a monophyletic group phylogenetically separated from the other four Trichoderma sections (Kuhls et al., 1997; Samuels et al., 1998) and is designated recently as the Longibrachiatum Clade (Samuels et al., 2012). It is one of the youngest clades of the genus (Kubicek et al., 2011) and has the largest number of available whole-genome sequence data. This clade is ecologically highly versatile as it contains prominent clinically relevant and ecologically restricted species. Trichoderma longibrachiatum, T. orientale, and T. citrinoviride are opportunistic human pathogens causing infections, mainly in immunocompromised patients (Kuhls et al., 1999; Kredics et al., 2003; Hatvani et al., 2013). T. longibrachiatum or its transformants have also been suggested for use as biocontrol agents against plant pathogens like Pythium ultimum or members of the Fusarium solani species complex (Migheli et al., 1998; Rojo et al., 2007). T. longibrachiatum and T. orientale are sympatric species but have different reproductive strategies, the former being strictly clonal, whereas the latter recombines sexually (Druzhinina et al., 2008). The cellulase producer T. reesei is also capable of sexual reproduction (Seidl et al., 2009), whereas its sympatric species T. parareesei is genetically isolated and has a clonal lifestyle (Atanasova et al., 2010; Druzhinina et al., 2010). While T. longibrachiatum and T. orientale are cosmopolitan, the related T. pinnatum and T. aethiopicum are rare and restricted species (Druzhinina et al., 2010). Numerous other species, including T. reesei, T. parareesei, T. pseudokoningii, T. sinense, T. effusum, T. konilangbra, T. andinense, or T. novae-zelandiae are also geographically restricted (Druzhinina et al., 2012).
Several secondary metabolites are produced by Trichoderma species from the Longibrachiatum Clade. Probably the best known species is T. reesei, which produces hydrolytic enzymes degrading cellulose or hemicellulose (Harman and Kubicek, 1998; Kubicek et al., 2009). Peptaibols are membrane-active compounds with the ability to aggregate and form ion channels in lipid bilayer membranes. They are usually short peptides of 8–20 residues with non-proteinogenic amino acids and are biosynthesised by non-ribosomal peptide synthetases (NRPSs) (Marahiel, 1997; Marahiel et al., 1997; May et al., 2002; Degenkolb et al., 2003, 2007; Bushley and Turgeon, 2010; Marik et al., 2017b). In the case of NRPSs, a single large protein is responsible for the activation, incorporation and elongation of the peptides. NRPSs can also incorporate non-proteinogenic residues, thus increasing the chemical diversity of the products. The lack of specificity of the recognition sites and the three-dimensional structure of the enzyme lead to the acceptance of closely related residues (such as Vxx vs. Lxx). Consequently, the number of positionally isomeric and homologous peptaibols biosynthesised by a single NRPS can be large. The repair mechanisms, which usually operate during biosynthesis, are also absent in NRPS pathways, thus further increasing the variability of the products. Characteristic residues of peptaibols include α-aminoisobutyric acid (Aib) and isovaline (Iva), as well as 1,2-amino alcohols such as Leuol, Valol, Pheol, Tyrol, Ileol, Alaol, and Prool at the C-terminus (Degenkolb et al., 2008; Stoppacher et al., 2013). Peptaibols usually form short, linear helical structures, several of which aggregate to form ion channels and may damage lipid membranes. Investigation of the structural and dynamic properties of peptaibol molecules is important for the understanding of their biological activities. Computational molecular dynamics-based simulation is a popular technique for investigating a molecule's dynamic behavior and predicting its three-dimensional structure. Peptaibols like trichobrachins (Násztor et al., 2013), harzianins (Putzu et al., 2017), alamethicin (Leitgeb et al., 2007; Kredics et al., 2013), tripleurin (Tyagi et al., 2019), and others have been investigated using such techniques. Knowledge about the structure of peptaibols might also facilitate the design of bioactive peptides for future applications. The characteristic non-proteinogenic amino acid residues of peptaibols (Aib and C-terminal alcohols) can be parameterised quantum-mechanically, and the effects of their presence can be evaluated. In general, long molecular time scales are required to effectively simulate peptide folding processes. An all-atom enhanced sampling technique known as accelerated molecular dynamics (aMD) can be used, which provides a non-negative boost to the potential energy and speeds up the process of peptide folding.
Trichoderma species are widely used against various plant pathogenic fungi as biocontrol agents because of their fast growth and reproduction, their mycoparasitism and their production of secondary metabolites (Chaverri et al., 2015; Degenkolb et al., 2015; Waghunde et al., 2016). Species like T. viride, T. virens, T. atroviride, T. asperellum, and T. harzianum are frequently studied due to their production of enzymes and antibiotics valuable in agriculture (Schuster and Schmoll, 2010; Contreras-Cornejo et al., 2016) and their antagonistic effects against pathogenic fungi such as Botrytis cinerea, Alternaria solani and Rhizoctonia solani (Harman et al., 2004). Incubation of a “T. harzianum” strain later re-identified as T. atroviride (Röhrich et al., 2014) with B. cinerea cell walls resulted in the secretion of cell wall hydrolytic enzymes and antibiotic fractions of peptaibols, which inhibited B. cinerea spore germination, causing a fungicidal effect. Peptaibols and hydrolytic enzymes were found to work synergistically in this antagonistic interaction (Schirmböck et al., 1994).
Trichoderma species also interact with plants through secondary metabolites. Although several studies reported positive effects of Trichoderma species on the physiological and biochemical responses of plants (Contreras-Cornejo et al., 2016), inhibition of plant growth and primary root development have also been described (Rippa et al., 2010; Shi et al., 2016). The most thoroughly investigated model plant, Arabidopsis thaliana, is frequently used to test the bioactivity of the secondary metabolites of Trichoderma species (Kottb et al., 2015). Peptaibols can induce auxin production and disruption of the auxin response gradient in root tips (Shi et al., 2016). The most thoroughly studied peptaibol, alamethicin, was shown to induce resistance in plants (Leitgeb et al., 2007; Kredics et al., 2013) but can also be toxic, causing lesions on Arabidopsis leaves (Rippa et al., 2010). However, it should also be considered that the commercially available alamethicin mixture (Sigma-Aldrich A4665) may also contain the trichothecene-type mycotoxin harzianum A produced by the strain T. brevicompactum used for alamethicin fermentations (Degenkolb et al., 2006).
This study aimed at revealing the genomic background, structural diversity and bioactivity of peptaibol compounds produced by different species from the ecologically diverse Longibrachiatum Clade of the genus Trichoderma.
Materials and Methods
Strains and Culture Conditions
Twenty-two strains from 17 Trichoderma species belonging to the Longibrachiatum Clade of the genus were selected from the TU Collection of Industrially Important Microorganisms, Vienna, Austria (TUCIM, www.vt.tuwien.ac.at/tucim/) and the Szeged Microbiology Collection, Szeged, Hungary (SzMC; www.szmc.hu) for investigation of their peptaibol production (Table 1). For testing the antifungal activity of peptaibol extracts, filamentous fungal strains of clinical relevance (Aspergillus fumigatus SzMC 23245, Fusarium falciforme SzMC 11407 and Fusarium keratoplasticum SzMC 11414 from human keratomycosis, India) or phytopathological relevance (Alternaria alternata SzMC 16085, F. solani species complex SzMC 11467 and Phoma cucurbitacearum SzMC 16088) were selected. The strains were maintained and cultured as described by Marik et al. (2017a).
Peptaibol Extraction
Peptaibols were extracted according to Marik et al. (2017a). For large quantity peptaibol production and purification, T. reesei QM9414 (SzMC 22616) was cultured according to Marik et al. (2018). The samples were purified on a Flash chromatograph (CombiFlash EZ Prep UV-VIS Teledyne Isco). The cartridge (CombiFlash EZ Prep) was filled with 60 cm3 silica (30–40 μm), and 1.5 g of crude peptaibol extract was applied above the septum. The flow rate was set to 35 ml min−1 and the wavelength of the UV detector to 270/320 nm. Solvents A and B were chloroform and methanol, respectively (gradient solvent B: 0%, 0 min; 0%, 5 min; 100%, 15 min; 100%, 18 min). Fractions were automatically collected into collector tubes (18 × 180 mm, 30 ml) based on the slope of the UV signal. Fractions were evaporated, dissolved in methanol (100 mg ml−1) and stored at −20°C. The purity of the samples was checked by HPLC-MS as described by Van Bohemen et al. (2016). For this analysis, the appearing y7-ion fragments were quantified and compared to alamethicin (Sigma-Aldrich A-4665, Hungary) dissolved in methanol (VWR, Hungary).
Analytical Procedures and Data Analysis
Crude peptaibol extracts were subjected to HPLC-ESI-MS using a Varian 500 MS equipment with the parameters described previously (Marik et al., 2018). The excitation storage level (m/z)/excitation amplitude (V) conditions during the MS2 measurements of selected y7 fragments were: m/z of 774.4 (209.4/3.02), m/z of 775.4 (209.7/3.03), m/z of 788.4 (212.9/3.08), and m/z of 789.4 (213.2/3.08). The method of peptaibol identification followed the protocol described previously by Marik et al. (2013, 2017a). The initial Varian 500 MS data were further confirmed by HPLC-Orbitrap-MS: Dionex UltiMate 3000 system (Thermo Scientific, CA, USA) controlled by the Xcalibur 4.2 software (Thermo Scientific, CA, USA) and equipped with a quaternary pump, a vacuum degasser, an autosampler and a column heater. Gemini NX-C18 HPLC column (50 × 2.0 mm, 3 μm; Phenomenex Inc., Torrance, CA, USA) was used for the separation. Solvent A was H2O:MeOH:MeCN 8:1:1 with 10 mM ammonium-acetate and 0.1% (v/v) acetic acid, while solvent B was acetonitrile/methanol 1:1 (v/v) with 10 mM ammonium-acetate and 0.1% (v/v) acetic acid. The flow rate was set to 0.2 ml min−1 and the gradient program for Solvent B was 10%−0 min, 10%−2 min, 78%−3 min, 89%−16 min, 95%−16.5 min, 95%−19.5 min, 10%−20 min, 10%−24 min. The column temperature was kept at 30°C and the injection volume was 5 μl. An Orbitrap-MS: Thermo Scientific Q Exactive Plus (Thermo Scientific, CA, USA) with HESI source in positive mode controlled by Xcalibur 4.2 software (Thermo Scientific, CA, USA) was used for the MS measurements. The HESI parameters were: spray voltage−3 kV, sheath gas flow rate−30 arbitrary units, aux gas flow rate−15 arbitrary units, capillary temperature−350°C, aux gas heater−250°C. The acquisition mode was Full-MS-ddMS2. Full-MS paramteres were: resolution−70,000 at m/z 200, AGC target−3e6, maximum injection time−100 ms, scan range−350-2200 m/z. The ddMS2 parameters: fixed first scan at m/z 80, resolution 17500 at m/z 200, AGC target−1e6, maximum injection time−50 ms, isolation window−1 m/z, collusion energy−30 NCE. The minimum AGC target for ddMS2 triggering was 1e5. As no amino acid analysis was carried out for the determination of the Val/Iva and Leu/Ile isomers, the Vxx/Lxx nomenclature was used in the peptaibol sequences. The newly identified peptaibol compounds were named according to the group to which they belong (A or B) and the elution order of the compounds on the HPLC-Varian MS system (I, II, …, n), appended to “Pept.” Compounds with the same retention time but different sequences were considered as variants and named with small latin letters (a, b, …, n; in decreasing order of amount the variants were produced). Group C peptaibols were named as brevicelsins and numbered according to their elution order.
Peptaibol profiles of individual strains were analyzed using cluster analysis in the ClustVis web tool (Metsalu and Vilo, 2015), and a heat map was constructed using the complete linkage and Euclidian distance settings applied to the columns (strains).
Degenkolb et al. (2006) reported that the Sigma alamethicin standard (A-4665) may be contaminated by the trichothecene mycotoxin harzianum A. In the case of the batch used in this study as a reference compound, the detection of harzianum A was carried out based on a previous article (Nielsen et al., 2005). The flow rate was set to 0.2 ml min−1 on a Phenomenex Gemini 50 × 2 mm, 3 μm HPLC column. The column heater was set to 30°C and the injection volume was 5 μl. An Orbitrap-MS detector was attached to the HPLC system and the parameters were set according to the Orbitrap MS parameters described above. The measurements ran in negative ionization mode, the spray voltage was set to −3 kV.
Bioinformatic Analysis of Peptaibol Synthetase Genes
Peptaibol synthetases of Trichoderma species from the Longibrachiatum Clade with accessible full genome sequences, T. reesei, T. parareesei, and T. citrinoviride (GenBank Assembly accession numbers GCA_000167675.2, GCA_001050175.1 and GCA_003025115.1, respectively) and two strains of T. longibrachiatum (GCA_003025155.1, GCA_000332775.1) were identified using the Secondary Metabolites from InterProScan (SMIPS) online software, and 20 as well as 14 module NRPSs were selected (Wolf et al., 2016). In the case of T. longibrachiatum, T. citrinoviride, T. reesei, and T. parareesei, the extracted sequences were analyzed using the Antibiotics and Secondary Metabolites Analysis Shell (antiSMASH), the PKS-NRPS Analysis Web-site, the NRPS/PKS substrate predictor and the NRPSPredictor3 SVM, as described by Marik et al. (2017a).
Accelerated Molecular Dynamics Simulations of 20- and 19-Residue Peptaibols
Calculation of the partial charges for the non-standard residues Aib and Pheol and the preparation of unfolded conformations of four selected peptaibols in water were carried out as described by Tyagi et al. (2019). The Leu and Val positions in brevicelsin sequences were predicted based on their positionally isomeric 20-residue paracelsin counterparts. For the Paracelsin B system, 3910 water molecules were added with a box size of 55.05 × 46.82 × 62.33 Å and a volume of 160676.0 Å3, whereas 3557 TIP3P water molecules were added with a box size of 55.05 × 42.11 × 63.40 Å and a volume of 147021.35 Å3 to prepare the Paracelsin H system. Similarly, 4725 water molecules were added to the Brevicelsin I system with a box size of 67.57 × 50.93 × 54.97 Å and a volume of 189190.34 Å3, whereas 4536 water molecules were added to the Brevicelsin IV system with a box size of 68.52 × 45.96 × 58.30 Å and a volume of 183623.0 Å3.
The four systems were prepared for aMD simulations used to enhance sampling with a boost to the whole potential energy and an extra boost to torsional energy. The values of coefficients a1 and a2 were set to 4, whereas b1 and b2 were set to 0.16, based on previous studies (Pierce et al., 2012).
Peptaibol Bioactivity Assays
For inhibition tests with filamentous fungi, malt extract agar medium completed with yeast extract was used at 25°C, following the method described by Marik et al. (2018). The purified peptaibol extract of T. reesei QM9414 was tested in an agar plate well-diffusion assay with methanol as a control, as well as alamethicin (Sigma-Aldrich A-4665, Hungary) and nystatin (Nystatin 2-hydrate BioChemica, AppliChem A3811,0025, Germany) as reference compounds. All solutions were prepared in two-step dilution series from 0.4 mg ml−1 to 0.0036125 mg ml−1. The inhibition zones were measured as the distance between the edge of the fungal colonies and the edge of the holes containing the peptaibol solutions at the time when the edge of the colony reached the edge of the control hole filled with methanol. At the same time, plates were photographed with a Coolpix S2600 digital camera (Nikon). Minimum inhibitory concentration (MIC) values were defined as the lowest concentrations where an inhibition zone could be detected. Experiments were carried out in triplicate.
In order to investigate the biological effects of peptaibols on plants, A. thaliana (Col-0 ecotype) seeds were planted on 0.5 × Murashige and Skoog agar (8%) medium (Horváth et al., 2015) with the addition of 0.5% sucrose (w/v) (pH adjusted to 5.5 with NaOH) in plastic Petri dishes (90 × 17 mm) five seeds per Petri dish in one line. Seeds were surface sterilized with 70% ethanol for 1 min, treated with 4% hypochlorite for 15 min and washed with sterile distilled water. After vernalisation at 4°C for 24 h, seeds were sown onto the agar plates. Arabidopsis plants were placed in a greenhouse with a photoperiod of 12 h of light and 12 h of darkness, a light intensity of 300 μmol m−2 s−2 and a temperature of 25 ± 1°C. After the third day post germination, plates were placed at an angle of 50° to allow root growth along the agar surface and to promote aerial growth of the hypocotyls. Four 5 mm holes were bored with a sterile cork borer 0.5 cm from the root tips of 5-day-old Arabidopsis seedlings (five seedlings per plate) and filled with 40 μl of peptaibol extract. The growth of primary roots was measured every 24 h for 4 days. Photographs of 15-day-old plants were taken using a Coolpix S2600 digital camera (Nikon). The fresh weights of the plants from each plate were measured, and photosynthetic pigments were quantified as described by Lichtenthaler (1987). Statistical analyses were performed using Bonferroni's multiple comparison tests with the GraphPad Prism software version 6.00 (GraphPad Software, San Diego, CA, USA; www.graphpad.com) using 25 samples.
Bioassays using porcine kidney cells (PK-15) and assays of cell membrane integrity disruption in boar sperm cells were carried out as described previously (Bencsik et al., 2014; Marik et al., 2017b).
Results
Identification of Peptaibols Produced by Trichoderma Species From the Longibrachiatum Clade
Peptaibols produced by species from the Longibrachiatum Clade of genus Trichoderma were identified using the strategy described by Marik et al. (2013, 2017a). Extracted ion chromatograms (EIC) resulting from full scan measurements of crude extracts from the examined Trichoderma strains are shown in Supplementary Figures 1–22. Singly-charged pseudomolecular ions, such as [M+Na]+ or [M+H]+, were scarcely detectable in the spectra, whereas doubly charged ([M+2Na]2+) ions were present and could be used for identification. Full scan MS spectra contained the series of the fragment ions from the N-terminal part (b1—b6 and b8-b13, Supplementary Figure 23) except for b7, where the stable Gln-Aib bond is present in the compounds (Krause et al., 2006a). The C-terminal y7 fragment was consistently observed and provided a good reference for the quantification of the peptides in the mixture. The first 13 amino acid residues could be identified from the full scan MS spectra, but MS2 experiments were performed for the identification of residues at the C-terminus. The last four residues could be identified directly from the MS2 spectra (Supplementary Figure 24). The y7-AA(19-15) ions were not shown on these spectra, therefore another MS2 fragmentation was performed on an Orbitrap-MS system from the y7 ions, which proved Vxx and Aib in positions 15 and 16, respectively (Supplementary Figure 25). All the detected peaks could also be reidentified at high resolution on the HPLC-Orbitrap-MS system, except for y7-H2O (Supplementary Tables 1–6). Instead of [M+Na]+ and [M+2Na]2+ ions, [M+H]+ could be observed on these spectra.
The peptaibol sequences could be categorized into three groups, designated as A (Table 2; Supplementary Tables 1, 4), B (Table 3; Supplementary Tables 2, 5) and C (Table 4; Supplementary Tables 3, 6). Groups A and B contain 20-residue peptaibols, whereas group C sequences had lost a residue in position R6. The novelty of the sequences was validated according to the “Comprehensive Peptaibiotics Database” (Stoppacher et al., 2013) as well as the last, offline version of the “Peptaibiotics Database.” The former online resource (Neumann et al., 2015) is unavailable since the autumn of 2017, therefore PubMed searches of publications since 2017 were performed with the keyword “peptaibol.” Several sequences proved to be homologous or positionally isomeric to the peptaibol subfamilies of trichobrachins, paracelsins, suzukacillins, saturnisporins, trichoaureocins, trichocellins, longibrachins, hyporientalins, trichokonins, trilongins, metanicins, trichosporins, gliodeliquescins, alamethicins, and hypophellins. Some sequences had amino acid exchanges in comparison with previously described compounds from the peptaibol groups listed above.

Table 2. Sequences of the newly identified group A peptaibol compounds from Trichoderma species of the Longibrachiatum Clade and their similarities to known peptaibols available in the “Comprehensive Peptaibiotics Database.”

Table 3. Sequences of the newly identified group B peptaibol compounds from Trichoderma species of the Longibrachiatum Clade and their similarities to known peptaibols available in the “Comprehensive Peptaibiotics Database.”
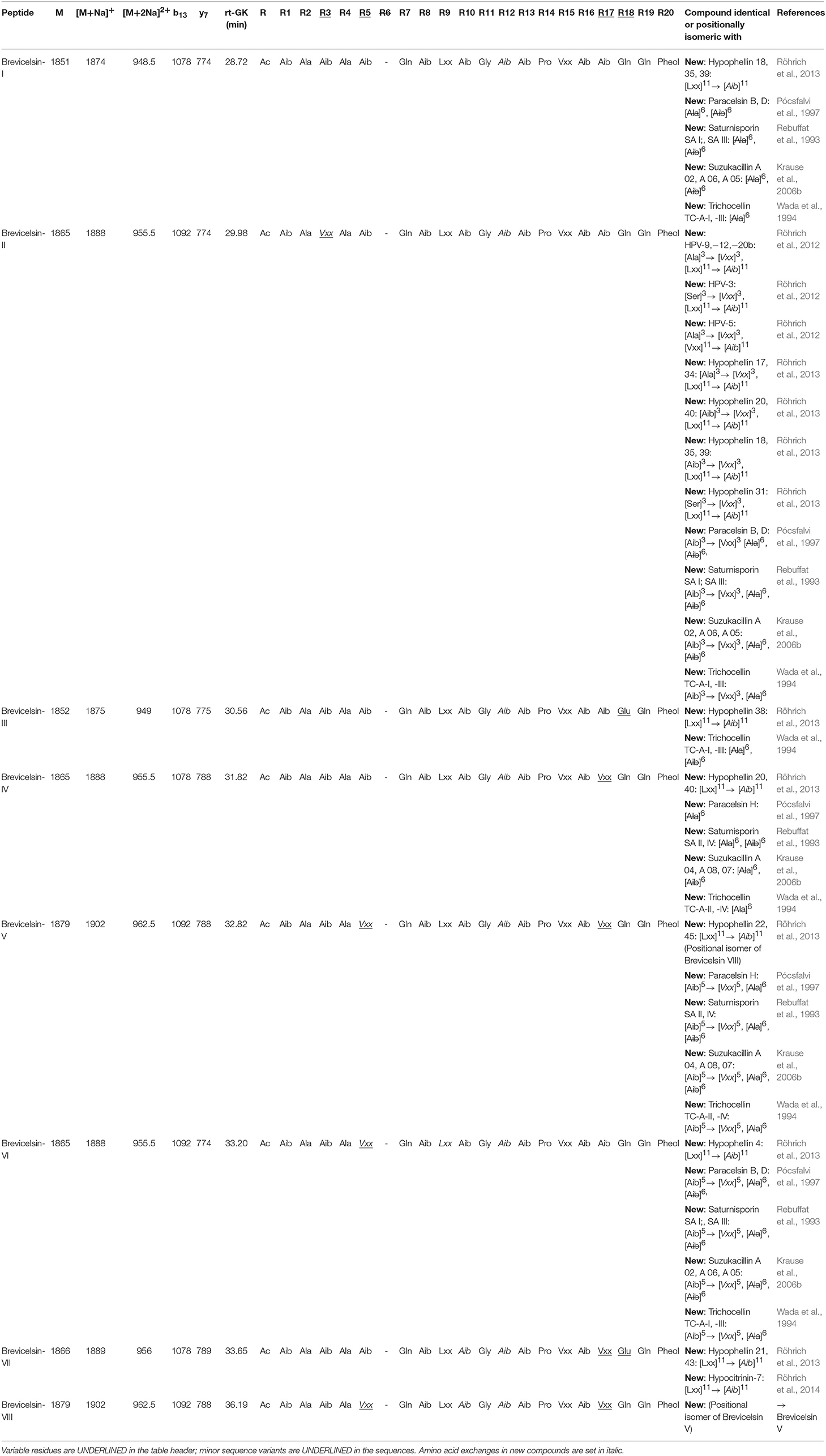
Table 4. Sequences of the newly identified brevicelsins (group C) from Trichoderma species of the Longibrachiatum Clade and their similarities to known peptaibols available in the “Comprehensive Peptaibiotics Database.”
Of the 49 sequences from group A consisting exclusively of 20-residue peptaibols, 27 have been previously described in the literature, and 22 were new, differing by 1–3 amino acids from known sequences (Table 2). Group B also comprises 20-residue sequences (Table 3). The main difference between group B and group A peptaibols is located at the R12 position, where Aib instead of Lxx is present in most of the group B sequences. Another major difference from group A is that the R5 position is not conserved due to a high percentage of Vxx instead of Aib. Of the 86 group B sequences, 37 were identified as new. An entirely new compound, Pept-B-LIX, with a mass of 1992 Da was detected in the crude extracts of three strains (T. konilangbra SzMC 22607, T. flagellatum SzMC 22608 and T. sinensis SzMC 22609). All sequences of group C produced by three strains (T. flagellatum SzMC 22608, T. sinensis SzMC 22609 and T. parareesei SzMC 22615) proved to belong to a new group of peptaibols, which was named brevicelsins, as they are similar to, but one amino acid shorter than paracelsins (Brückner and Graf, 1983; Pócsfalvi et al., 1997) (Table 4).
Qualitative and Semi-quantitative Peptaibol Profiles of the Strains
After investigation of all strains producing peptaibols from group A, “a” and “b” versions of their peptaibol compounds were apparent. Pept-A-XI has a “c” version of the compound, and a few others are represented by only a single sequence (Supplementary Table 7). Compounds such as Pept-A-IV-a and -b were produced constantly in high quantities by all strains. Both Pept-A-IX-a and -b were produced in high quantities by all strains except T. aethiopicum SzMC 22602, T. pinnatum SzMC 22603 and T. longibrachiatum SzMC 1775. Similarly, Pept-A-XVI-a and -b were produced by all strains. In this group, seven mainly produced peptaibol varieties appeared on the spectra, Pept-A-IV-a and -b, Pept-A-VI-a and -b, Pept-A-IX-a and -b, Pept-A-XV-a and -b, Pept-A-XVI-a and -b, Pept-A-XIXa as well as Pept-A-XXI-a and -b.
The analysis of the four T. longibrachiatum strains (SzMC 1773, 1775, 1776, and 12546) revealed similar, but still different, profiles (Supplementary Table 8). Environmental isolates of T. longibrachiatum produced more similar profiles, whereas the peptaibol profile of the clinical isolate was different from those of the three environmental strains. Pept-B-XX and Pept-B-XXVII were produced by all of the strains examined, whereas the other compounds were produced only by certain strains. Five peptaibol compounds (Pept-B-VII, Pept-B-XVII, Pept-B-XX, Pept-B-XXVII, and Pept-XLV-a and b) were produced at high levels. Certain strains could also produce other compounds, such as Pept-B-XXVIII, Pept-B-XXIX-a and b, Pept-B-XXXIIIa, Pept-A-IVb, Pept-XLIb, Pept-XLIII, Pept-B-XLVa, Pept-B-LI, Pept-B-LIV, and Pept-B-LVIb, at high levels. The most diverse peptaibol profile was observed in T. reesei QM6a (SzMC 22614), which produced 41 different peptaibol compounds, whereas the least diverse profiles were that of T. effusum SzMC 22611 and T. konilangbra SzMC 22607, which produced 11 and 12 sequences, respectively. Some species producing mostly group B peptaibols, T. reesei QM6A (SzMC 22614), T. saturnisporum SzMC 22606 and T. konilangbra SzMC 22607 could also produce peptaibols from group A. Interestingly, group A sequences could not be detected from the two mutant strains of T. reesei SzMC 22614 (T. reesei SzMC 22616 and SzMC 22617). Brevicelsins from group C were only produced by three species, T. sinensis, T. flagellatum and, to a lesser extent, T. parareesei. Brevicelsin I and Brevicelsin IV were produced by the examined strains (T. flagellatum SzMC 22608, T. sinensis SzMC 22609 and T. parareesei SzMC 22615) of all three species, but T. parareesei produced only these two compounds of group C in addition to the group B sequences.
We carried out a cluster analysis of the peptaibol diversity profiles in different Trichoderma species of the Longibrachiatum Clade based on the production levels of different peptaibols by various fungal producers (Supplementary Tables 7, 8). According to their peptaibol profiles, members of the Longibrachiatum Clade were divided into two main clusters (Figure 1). The first cluster involves species producing exclusively group A peptaibols. Among them, T. novae-zelandiae is characterized with a relatively poor, but sharply distinct, profile of abundantly produced peptaibol compounds from group A, like Pept-A-XXIb, XVIb, XII, Vb, Ib, and IIIc. Further species in this cluster include members of the phylogenetic subclades Longibrachiatum/Orientale and Citrinoviride/Pseudokoningii, along with the lone lineages T. ghanense and T. capillare (Table 1). This cluster is consisting of three subclusters, the first one containing the closely related species T. aethiopicum and T. pinnatum and the second one involving T. longibrachiatum and T. orientale—all belonging to the phylogenetic subclade Longibrachiatum/Orientale—while the third subcluster is corresponding with the subclade Citrinoviride/Pseudokoningii (Table 1; Figure 1). The second main cluster is comprised of species producing mainly group B peptaibols and includes 2 subclusters, with the first containing the phylogenetic subclades Parareesei/Reesei, Saturnisporum and the lone lineages T. andinense and T. effusum, while the second harboring the three examined species from subclade Konilangbra/Sinensis (Table 1; Figure 1). All three examined members of this subclade produced the entirely new compound Pept-B-LIX (1992 Da).
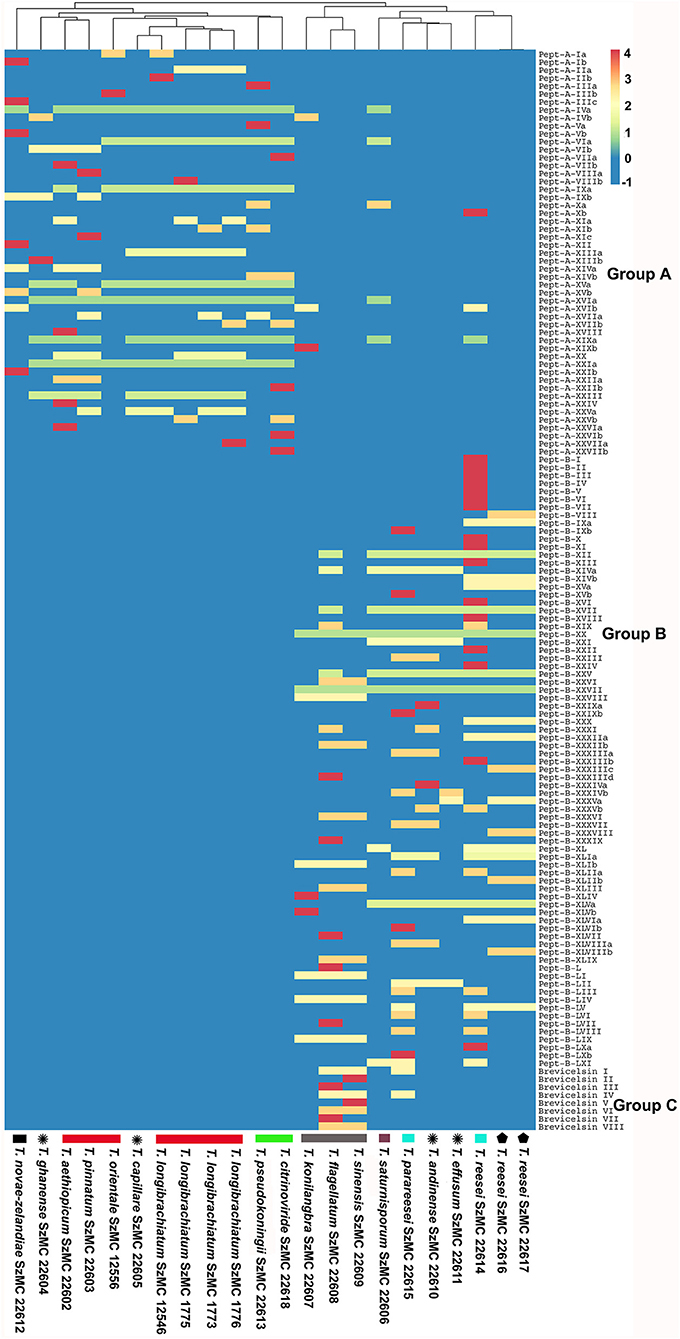
Figure 1. Heatmap showing the correlation between the production of peptaibols and the phylogenetic relationship between the strains. Monophyletic species are indicated by the bottom bar of the same color, species attributed to single phylogenetic lineages are marked with a star, while mutant strains are indicated with a filled pentagon. The color scale denotes production level increasing from zero (deep blue) to high (deep red).
Annotation of NRPS Domains From the Genomes of T. longibrachiatum, T. citrinoviride, T. reesei, and T. parareesei
The NRPS gene sequences from T. longibrachiatum (https://genome.jgi.doe.gov/Trilo1/Trilo1.home.html, Xie et al., 2014), T. citrinoviride (https://genome.jgi.doe.gov/Trici4/Trici4.home.html), T. reesei (https://genome.jgi.doe.gov/Trire2/Trire2.home.html, Martinez et al., 2008) and T. parareesei (NCBI Bioproject Id: PRJNA287603, Yang et al., 2015) predicted by the SMIPS software were analyzed using the fungiSMASH software pipeline (Blin et al., 2017), which was designed to identify gene clusters of secondary metabolite biosynthesis from nucleotide sequences and to predict the products of the clusters identified. The T. longibrachiatum, T. citrinoviride, T. reesei, and T. parareesei genome sequences contain genes encoding 20-module NRPSs of 69.505, 68.508, 69.516, and 69.516 bp, as well as 14-module NRPSs of 43.422, 44.196, 49.386, and 52.395 bp with adenylation, condensation, thiolation, single acyl transferase and thioesterase domains. Figure 2 shows the schematic structure of the 20-mer NRPS gene cluster and the encoded modular enzyme from T. longibrachiatum. The 5′ ends of 20-module synthetase sequences contain a ketide synthase, whereas a Phe-specific permease-like and an aldo/keto reductase-like gene can be found downstream from the NRPS gene cluster. These two genes were also identified in the region downstream of the 18-module peptaibol synthetase gene clusters of the mushroom green mold agents T. aggressivum and T. pleuroti (Marik et al., 2017a). The identification of the presence of Pro in the peptaibol sequences and the close proximity of a Pro-specific permease gene to the NRPS gene cluster in these six Trichoderma species suggests that the permease may have a role in the secretion of these secondary metabolites.
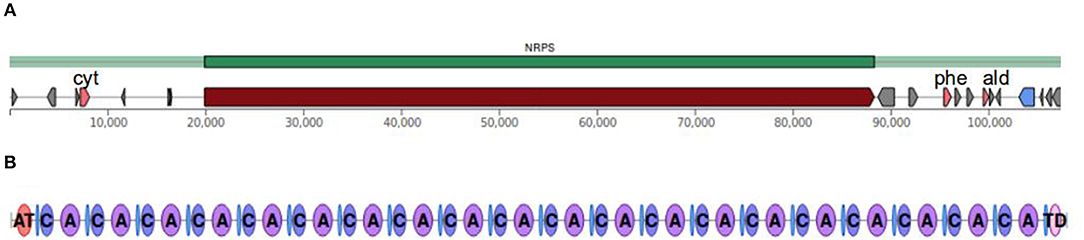
Figure 2. Schematic structure of the 20-residue non-ribosomal peptide synthase (NRPS) from T. longibrachiatum. (A) Up- and downstream regions of the gene encoding the NRPS enzyme; pink pentagons indicate genes of additional putative biosynthetic enzymes (cyt: cytochrome P450, phe: phenylalanine-specific permease, ald: aldo/keto reductase). (B) The 20 modules of the NRPS. AT, acyl transferase; C, condensation module; A, adenylation module; TD, thioesterase domain.
Table 5 shows the incorporated amino acids predicted by the NRPS/PKS substrate predictor and NRPSPredictor3, based on the annotated adenylation domains and the eight amino acid residue signature sequences. The four 20-module NRPSs from the Longibrachiatum Clade were identical in positions R15 and R16 according to the signature sequences and the incorporated amino acids, respectively. Two positions (R6 and R9) were different only in T. longibrachiatum, whereas position R17 showed identity between T. longibrachiatum/T. citrinoviride, and T. reesei/T. parareesei. The most variable position was predicted to be R12, in which all signature sequences differed, and the incorporated amino acid was different in the case of T. citrinoviride.
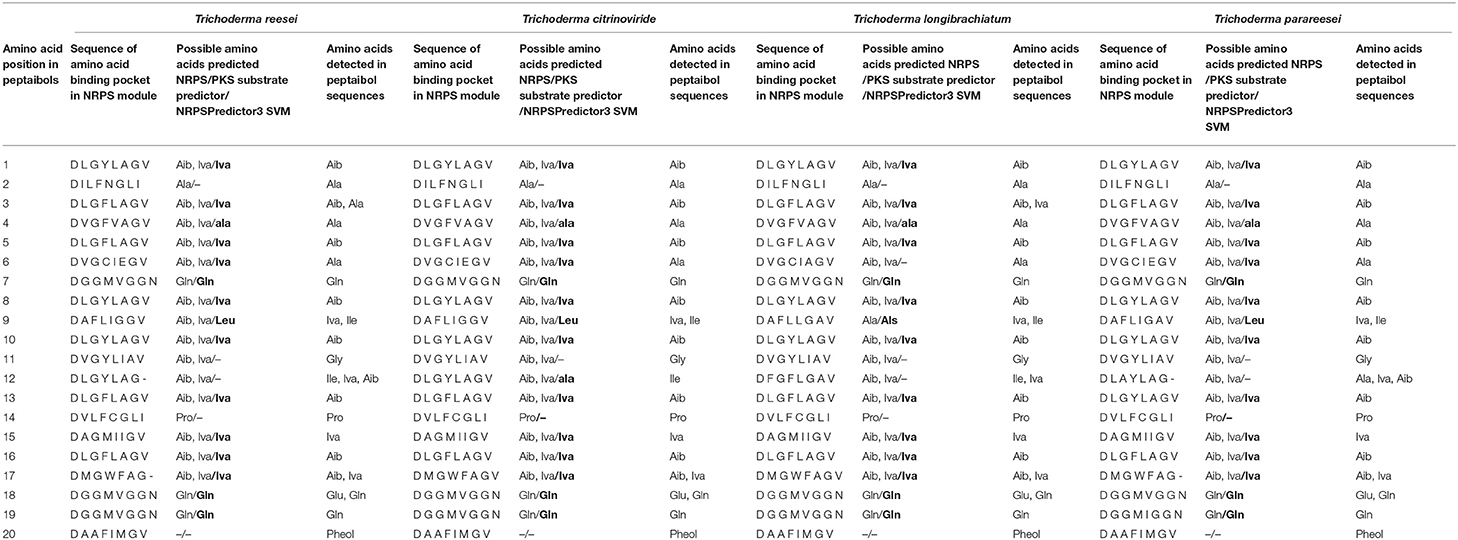
Table 5. Comparison of signature sequences of NRPS modules and predicted amino acid incorporations with the detected amino acid composition of the 20-residue peptaibols in the case of four Trichoderma species from the Longibrachiatum Clade.
Comparison of the amino acids predicted by the NRPS/PKS substrate predictor and the ones detected showed agreement at 11 positions in all four species. In positions R6, R11 and R18, the prediction did not match with the detected Ala, Gly and Glu, respectively. Position R11 of the four species showed identity with position R10 of T. aggressivum (Marik et al., 2017a) in its signature sequence (DVGYLIAV), but the amino acid prediction in these positions was incorrect in all cases. At the last position, the predictor software identified the signature sequence of adenylation domains, but the amino acid prediction failed. These unsuccessful predictions suggest that these signature sequences are missing from the database. Based on the signature sequences, the highest variability is in position R12, where the amino acids detected are also variable.
Structural Characterization of 20- and 19-Residue Peptaibols
Two previously described sequences, Paracelsin B (AcAib-Ala- Aib-Ala-Aib-Ala-Gln-Aib-Leu-Aib-Gly-Aib-Aib-Pro-Val-Aib-Aib-Gln-Gln-Pheol) and Paracelsin H (AcAib-Ala-Aib-Ala-Aib-Ala-Gln-Aib-Leu-Aib-Gly-Aib-Aib-Pro-Val-Aib-Val-Gln-Gln-Pheol), together with their 19-residue counterparts Brevicelsin I (AcAib-Ala-Aib-Ala-Aib-Gln-Aib-Leu-Aib-Gly-Aib-Aib-Pro- Val-Aib-Aib-Gln-Gln-Pheol) and Brevicelsin IV (AcAib-Ala- Aib-Ala-Aib-Gln-Aib-Leu-Aib-Gly-Aib-Aib-Pro-Val-Aib-Val-Gln-Gln-Pheol) were selected for structural characterization. Based on their sequences, Paracelsin B and H appear to correspond with Pept-B-XII and Pept-B-XVIII, respectively, both of which were produced by six examined species (T. reesei, T. saturnisporum, T. andinense, T. effusum, T. parareesei, and T. flagellatum). Our aim was to observe structural differences resulting from the loss of Ala at the R6 position.
All peptides show a strong tendency to form right-handed helical structures with a slight bend at the Aib-Pro position (Figure 3). Cluster analysis of the simulation trajectories of all four peptaibols revealed different energetically stable conformations that occur during folding, and the representative structures of the most populated cluster are provided for each peptaibol. All peptides fold into an energetically favored, highly bent helical conformation along with a linear helical conformation. Based on the reweighted potential of mean force (PMF) values calculated for end-to-end distance (distance in Å from the N-terminus to the C-terminus), it can be speculated that a highly curved conformation for all peptaibols, except for Paracelsin H, lies in the energy minimum and requires an energy “jump” of <1 kcal mol−1 to attain the linear backbone conformation (Figure 4A). Overall, the end-to-end distance values as low as 5 to 27 Å, that lie close to the energy minima, show that all conformations starting from a hairpin-like helix structure to a straight backbone with just a slight bend are easily accessible. The PMF values increase rapidly beyond these two points for all four peptaibols, as shown in the inset image focusing only on PMF values up to 2 kcal mol−1. However, the sequences Paracelsin B and Brevicelsin I, with an Aib residue in position R17, have higher PMF values for higher end-to-end distance values; the energy cost for attaining linearity of the helical backbone is slightly higher than in Paracelsin H and Brevicelsin IV, where a Val residue replaces Aib in the R17 position. The energy minimum for Paracelsin H lies at an end-to-end distance of 22 Å, whereas Brevicelsin IV exhibits a slight fall at this point, even though its energy minimum also lies at 10 Å. The presence of Aib residue in position R17 (in Paracelsin B and Brevicelsin I) results in a highly dynamic folding process, which means that many conformations were visited during the trajectory, whereas Val in the same position (in Paracelsin H and Brevicelsin IV) led to fewer energetically stable conformers. The root-mean-square-atomic fluctuation (RMSF) graph (Figure 4B) shows higher fluctuation of N-terminus region for all peptides. No other significant differences were observed between the RMSF values of the 19-residue peptaibols, Brevicelsins I and IV, in comparison to 20-residue peptaibols, Paracelsins B and H, except that the sequences containing more Aib residues show a slight elevation in atomic fluctuation at the corresponding sequence position. For example, at R16 for Brevicelsin I and R17 for Paracelsin B, also, the R6 Aib in Paracelsins B and H shows higher average atomic fluctuation than the R6 Gln of Brevicelsins I and IV. This observation establishes the fluctuating and dynamic nature of the Aib residue in peptaibol sequences which can be explained by its tendency to oscillate between right- and left-handed helical forms. The Gln residues at R7 and R6 positions of paracelsins and brevicelsins, respectively, show a sharp dip in atomic fluctuation indicating higher stability in comparison to the C-terminal Gln residues and highlights importance of glutamines in ion-channel stabilization (Whitmore and Wallace, 2004).
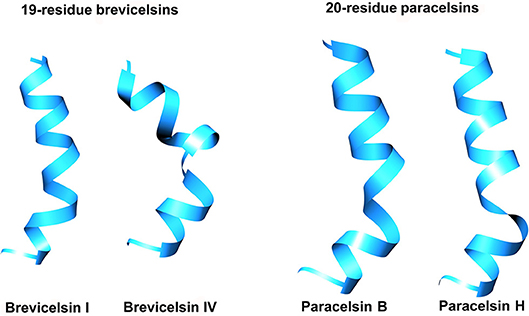
Figure 3. Representative structures of the most populated clusters for each peptaibol simulation. The simulations were carried out for 1,000 ns in each case.
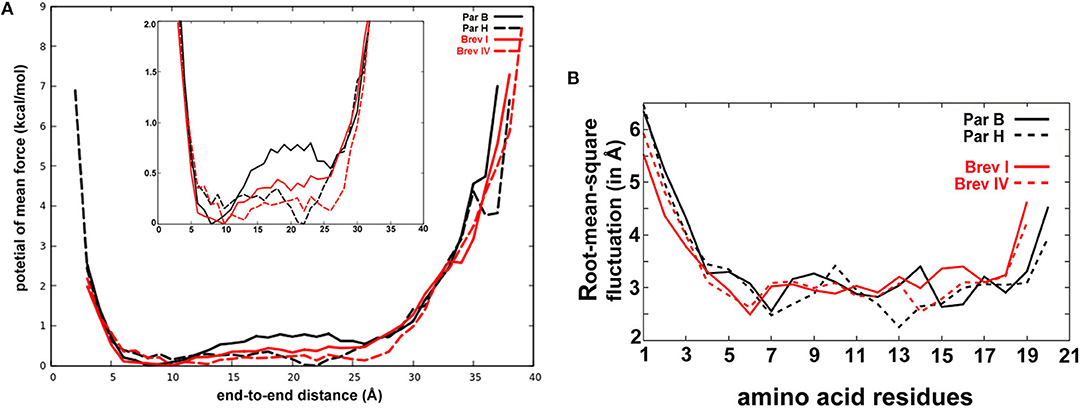
Figure 4. (A) The reweighted potential of mean force (PMF in kcal mol−1) values calculated for end-to-end distance (in Å). The 20-residue paracelsins have been plotted in black, whereas 19-residue brevicelsins are shown in red. The zoomed version of the plot is provided in the inset. The end-to-end values where PMF is zero or close to zero are the energetically stable values and define the most favorable linear conformation. (B) The root-mean-square deviation (in Å) calculated for each residue for all sequences gives an idea of the average fluctuation undergone by the system. Par, Paracelsin; Brev, Brevicelsin.
Antifungal Effects of T. reesei Peptaibols on Filamentous Fungi
The purified peptaibol extracts of T. reesei QM9414 were tested on human and plant pathogenic filamentous fungi, furthermore, the producer strain itself, as well as its Δlae1 mutant (Table 6). Treatment with 0.4 and 0.2 mg ml−1 purified peptaibol solution resulted in growth inhibition of all strains, whereas a weaker, but still notable, inhibition was detected after treatment with the purified extract at a concentration of 0.1 mg ml−1. The peptaibol extract from T. reesei QM9414 exhibited an inhibition profile highly similar to that of alamethicin.
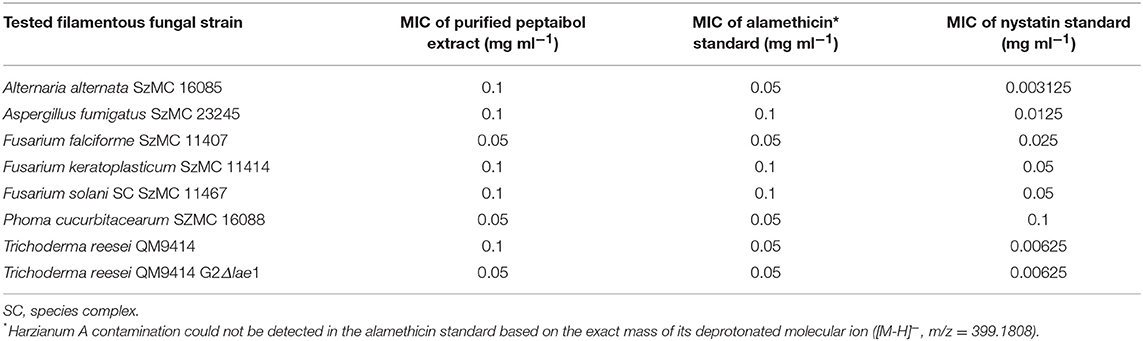
Table 6. Antifungal activity of the purified peptaibol extract from T. reesei QM9414 to filamentous fungi.
Bioactivities of T. reesei Peptaibols on Arabidopsis thaliana Plants
In order to evaluate the value of peptaibols as antifungal agents for plant protection, the purified (98%) peptaibol extract of T. reesei QM9414 was investigated for toxicity in the model plant A. thaliana. The extract was diluted to 50, 10, 5, 1, 0.5, 0.3, 0.1, and 0.05 mg ml−1. All of the treated plants were inhibited after treatment with the peptaibol extract at concentrations of 50, 10, and 5 mg ml−1. Root growth was observed only at concentrations ≤ 1 mg ml−1; however, inhibited growth could be observed down to concentrations of 0.1 mg/ml (Figure 5). Treatment with 1 mg ml−1 peptaibol solution resulted in a hook formation of the primary roots. Chlorophyll-a, -b and carotenoid levels decreased after treatment with extracts of ≥0.3 mg ml−1 (Figure 6). Treatment with a peptaibol solution of 0.1 mg ml−1 resulted in a similar rate of production of photosynthetic pigments but an increased anthocyanin level in 15-day-old plants. The root growth of these plants was suppressed in 6- to 9-day-old plants, although the plants showed normal biomass and could probably eventually survive this minimal toxicity because of the increased levels of anthocyanin (Figure 7).
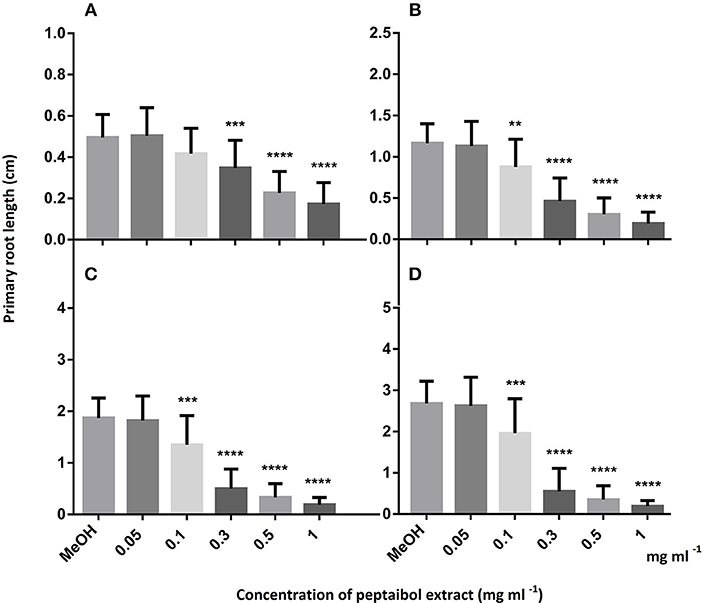
Figure 5. Primary root growth of 6 (A), 7 (B), 8 (C), and 9 (D) days old Arabidopsis thaliana plants after treatment with peptaibol extract from Trichoderma reesei QM9414. Methanol was used for the control plants as all peptaibol extracts were prepared in this solvent. Significance is assessed based on P-values: *P ≤ 0.05; **P ≤ 0.01; ***P ≤ 0.001 and ****P ≤ 0.0001.
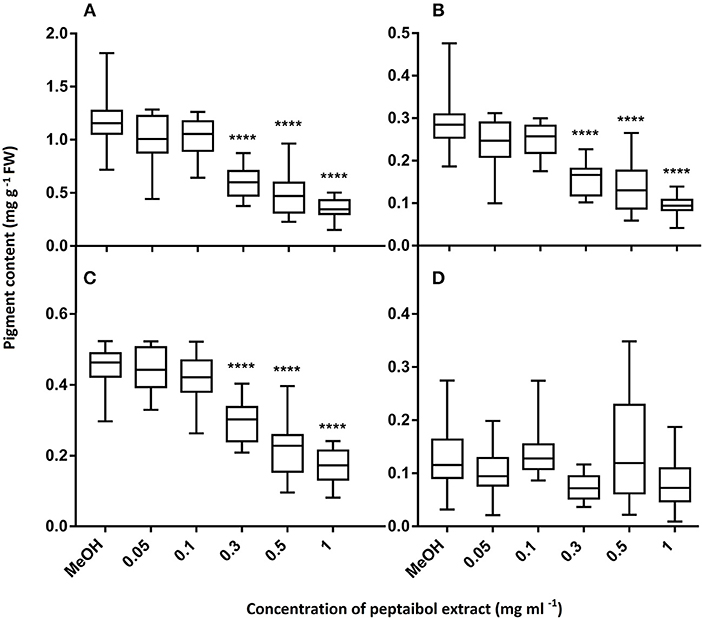
Figure 6. Pigment content of 15-day-old Arabidopsis thaliana leaves after treatment with peptaibol extract from Trichoderma reesei QM9414: chlorophyll-a (A), chlorophyll-b (B), carotenoids (C) and anthocyanins (D). Methanol was used for the control plants. Significance is assessed based on P-values: *P ≤ 0.05; **P ≤ 0.01; ***P ≤ 0.001 and ****P ≤ 0.0001.
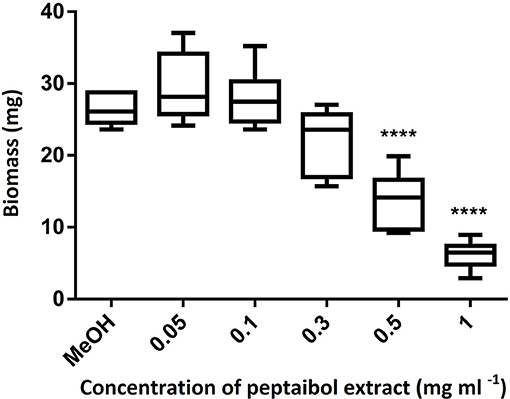
Figure 7. Biomass of 15-day-old Arabidopsis thaliana plants after treatment with peptaibol extract from Trichoderma reesei QM9414. Methanol was used for the control plants. Significance is assessed based on P-values: *P ≤ 0.05; **P ≤ 0.01; ***P ≤ 0.001 and ****P ≤ 0.0001.
Bioactivities of T. reesei Peptaibols on Mammalian Cells
The endpoint of toxic concentration—the last dilution step of the purified peptaibol solution which is toxic to mammalian cells—was determined for the peptaibol extract of T. reesei QM9414 (Table 7). After 20 min incubation at 37°C or 24 h at room temperature, the boar sperm motility inhibition end point was detected after treatment with 3 μg ml−1 peptaibol solution. The acrosome of the exposed sperm cells reacted at the same concentration, which inhibited motility, indicating that the toxic effect involves the plasma membrane. The inhibition end point of proliferation in porcine kidney PK-15 cells was observed at a concentration of 8 μg ml−1 peptaibol solution.
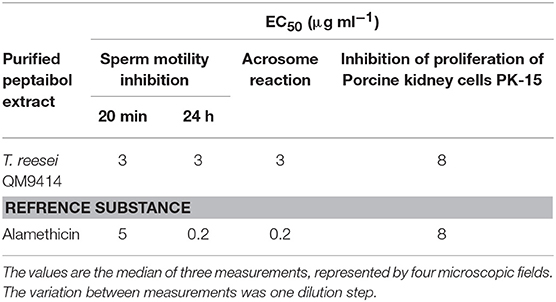
Table 7. Toxicity of the peptaibol extract from T. reesei QM9414 to boar sperm and porcine kidney cells.
Discussion
In this study, the structural diversity and bioactivity of peptaibol compounds produced by Trichoderma species belonging to the Longibrachiatum Clade were investigated and compared. The Longibrachiatum Clade is ecologically highly versatile as it contains both environmental and opportunistically pathogenic species, some of which can be found worldwide, whereas others are ecologically restricted. In total, 143 20-residue peptaibols could be identified from the 17 species examined, including 59 new and 76 recurrent compounds, as well as eight new 19-residue sequences. The peptaibols can be categorized into groups A, B and C, based on their primary structure, where groups A and B consist of 20-residue peptaibols, whereas group C is comprised exclusively of 19-residue sequences. The main difference between peptaibols of group A in relation to group B is in the R12 position. Sequence analysis identified several conserved regions along with some variable positions (R3, R5, R6, R10, R12, and R17), which have also been reported in a previous study (Pócsfalvi et al., 1997). Vxx was usually found instead of Ala and Aib at certain variable positions like R3, R5 and R6, which has never been observed among similar peptaibols. Although all of these amino acids have helix-forming properties, a substitution by Val would render a more linear and less fluctuating helical conformation owing to its bulkier sidechain. The highly curved backbone conformation is not energetically favored with increasing number of Val in peptaibol sequences. It has been hypothesized that the equilibrium between the bent (closed form) and linear conformations (open amphipathic form) may act as a “conformational switch” of voltage gating in ion channels across bilayers (North et al., 1995). Clearly, such substitutions have an important functional relevance, especially at subterminal positions like R3 and R17.
Brevicelsins from group C form a new family of 19-residue peptaibols similar to, but one amino acid shorter than group B sequences. They are not N-terminally truncated derivatives of their full-length precursors—like it is the case for the 16-residue brevikindins deriving from 18-residue trichokindin-like peptaibols (Degenkolb et al., 2016) – but differ from group B sequences by the internal deletion of position 6. This position is critical, since the following Gln plays an important role in the formation of ion channels (Wilson et al., 2011). Brevicelsins could be found only in three species: T. flagellatum, T. sinense and T. parareesei. A full genome sequence is available for T. parareesei, analysis of this sequence, however, revealed no extra 19-module NRPS synthetases but only a 20-module enzyme. The 19-residue peptaibols could be produced by the same, 20-module NRPS via the interaction of non-neighboring modules known as internal module skipping. The mechanisms of this phenomenon resulting in additional classes of 10-, 13-, 18-, and 19-residue peptaibols were proposed by Degenkolb et al. (2012). R6 is also skipped in T. phellinicola peptaibols (Röhrich et al., 2013), which does, however, contain Lxx in position R12, similar to group A peptaibols and unlike brevicelsins with Aib in this position.
The unique group A peptaibol profile of T. novae-zelandiae (Figure 1) may be related to the geographical origin of this species, which is endemic to New Zealand, and to its occupying a basal position in the Longibrachiatum Clade (Samuels et al., 2012). This species has tuberculate conidia, a trait also found in the Viride Clade (Jaklitsch et al., 2006), and it may be an ancestral trait of the Longibrachiatum Clade (Druzhinina et al., 2012). Our results suggest that the production of group A peptaibols may be another ancestral trait of the Longibrachiatum Clade, while the switch to the production of group B peptaibols might have occurred multiple times and seems therefore to be the result of convergent evolution. This switch from group A to group B has not fully completed in certain species: wild-type T. reesei as well as T. saturnisporum and T. konilangbra are also producing some group A compounds in addition to group B peptaibols.
Except from T. reesei, which was separated from its closest relative T. parareesei, the clustering based on peptaibol profiles reflected the close relationships within phylogenetic subclades in most of the cases (e.g., within subclades Longibrachiatum/Orientale, Citrinoviride/Pseudokoningii, or Konilangbra/Sinensis). For example, the species from the Konilangbra/Sinensis subclade are phylogenetically close to each other and are only known from the Paleotropical/Asian areas including Ethiopia (T. flagellatum), Uganda (T. konilangbra) and Taiwan (T. sinensis) (Samuels et al., 2012). The very close relationship of T. sinensis and T. flagellatum is also reflected by their ability to produce group C peptaibols in addition to group B sequences. The phylogenetic relationships between the subclades are less reflected by the clustering based on peptaibol profiles. Distantly related subclades (e.g., Longibrachiatum/Orientale and Citrinoviride/Pseudokoningii) may share similar profiles, while closely related subclades may exhibit substantially different ones—e.g., members of subclade Citrinoviride/Pseudokoningii produce group A peptaibols, while group B compounds are produced by their close relative T. effusum. This could be explained by multiple events of switching from the production of group A to group B during the evolution of the Longibrachiatum Clade.
Based on molecular dynamics simulations, 20-residue peptaibols result in higher linearity of helices than their 19-residue counterparts and are also relatively stable in terms of the atomic fluctuations of each residue. Paracelsins B, H and their 19-residue deletion sequences Brevicelsin I and IV all fold into right-handed helical structures with a slight bend at the Aib-Pro bond, except for Brevicelsin IV where the bend occurs at the Aib11-Aib12 bond. The Aib-Pro bond at R13-R14 in the case of 20-residue sequences is important for the secondary structure of the bent molecule. An important observation was made with respect to Val substitution instead of Aib at R17 which seems to hinder the formation of a bent backbone in close proximity to the N-terminal side-chains, because it is a chiral, hydrophobic amino acid with a bulkier side-chain than that of the achiral Aib. Frequent occurrence of Aib could be detected at the termini of the sequences, which are very important for the determination of the formation of helical structures including α- or 310-helices (De Zotti et al., 2010; Gessmann et al., 2012a,b). The other promotor of the helical structure, D-Iva, is most often found close to the N-terminus, prior to the Gln-Aib bond in position R6, based on different previously described peptaibols such as boletusin 1, chrysospermins, peptaivirins, trichorzianins TA and TB, or the TA1938, 1924, 1910 and 1909a compounds (El-Hajji et al., 1987; Rebuffat et al., 1989; Dornberger et al., 1995; Lee et al., 1999; Yun et al., 2000; Panizel et al., 2013).
The growth of filamentous fungi pathogenic to plants or humans could be inhibited by the purified peptaibol extract of T. reesei QM9414. A stronger inhibition was observed in the case of the Δlae1 mutant of T. reesei than in the case of the other strains, suggesting that the mutation in the methyl transferase gene, which is known as a global epigenetic regulator of gene expression, may also affect tolerance to these metabolites. A previous study (Marik et al., 2018), in which crude peptaibol extracts were tested on several bacterial, yeast and filamentous fungal strains showed similar results. The inhibitory effects of peptaibols to bacteria and filamentous fungi have previously been reviewed (Szekeres et al., 2005; Daniel and Rodrigues Filho, 2007). It has also been demonstrated that purified trichokonin VI triggers a change of fungal membrane permeability and disintegration of subcellular structures, has an effect on mitochondrial membrane permeabilisation and intracellular ROS production, induces phosphatidylserine exposure and eventually triggers metacaspase-independent apoptosis in F. oxysporum (Shi et al., 2012).
Alamethicin, the most studied peptaibol was shown to induce resistance in plants (Leitgeb et al., 2007; Kredics et al., 2013), although it can also be toxic, causing lesions on Arabidopsis leaves (Rippa et al., 2010). At higher concentration, it induces rRNA cleavage-associated rapid death (Rippa et al., 2007). Alamethicin could permeabilise mainly the apical meristem and epidermis cells of the root tips, but not the basal meristem cells, cortex cells or the root cap of A. thaliana (Dotson et al., 2018). If the root was pretreated with cellulase, permeabilisation could not be observed. This study proved cellulose-induced resistance and cell-specific alamethicin permeabilisation of A. thaliana roots. Engelberth et al. (2001) successfully demonstrated the high biological activity of alamethicin that caused emission of volatile compounds from lima beans (Phaseolus lunatus) placed under low concentration of the peptaibol solution. When it was applied to Bryonia dioica tendrils at the same concentration, it elicited jasmonate-induced tendril coiling. Therefore, peptaibols may be used as potential elicitors of plant defense responses. Recently, antiviral activity of trichorzins was also reported on cowpea plants against Cucumber mosaic virus (Kai et al., 2018). In this recent study, bioactivity tests with the selected, purified peptaibol extract of T. reesei QM9414 demonstrated toxicity to A. thaliana plants at higher concentrations. An interesting effect of the peptaibol extract was the induction of hook formation in the root tips. A previous study revealed similar results, where the inoculation of A. thaliana with T. atroviride resulted in shortened primary root growth of the plants and ended in a hook formation, although the lateral root numbers were increased (Pelagio-Flores et al., 2017). An inhibitory effect on primary root growth in A. thaliana was also observed after interaction with T. longibrachiatum SMF2, and its peptaibols induced auxin production and disruption of the auxin response gradients in root tips (Shi et al., 2016).
Boar sperm cells are frequently used for the detection of toxins, which affect plasma membranes (Vicente-Carrillo, 2018; Castagnoli et al., 2018). Due to the high sensitivity of boar sperm cells to toxins, many studies have concluded that these tests are appropriate for toxin detection (Peltola et al., 2004; Andersson et al., 2009, 2010). Similar measurements of peptaibol extracts produced by T. longibrachiatum Thb have been reported, and a mixture of trilongins proved to be a stronger inhibitor of motility than trilongins alone, or any of the crude extracts (Mikkola et al., 2012). Single ion channels remained in an open state for a longer time when exposed to a combination of the long peptaibols (trilongins BI–BIV) with the short ones (trilongin AI), than for the long peptaibols alone. Furthermore, peptaibols (trichokonin VI) could inhibit HepG2 cancer cells by inducing autophagy and apoptosis through an influx of Ca2+, which triggered the activation of μ-calpain and proceeded to the translocation of Bax to mitochondria and the subsequent promotion of apoptosis (Shi et al., 2010). Another peptaibol, emericellipsin A, which is a short lipopeptaibol, exhibited selective cytotoxic activity against HepG2 and HeLa cell lines (Rogozhin et al., 2018), similar to culicinin D, another short linear peptaibol which has been described as a potent anticancer compound (He et al., 2006). In the present study, the partially purified peptaibol extract of T. reesei QM9414 proved to inhibit boar spermatozoa and porcine kidney PK-15 cells at 0.1 mg ml−1, which rises the question of a possible in vivo toxicity. Degenkolb et al. (2008) discussed this issue in detail and suggested that the toxicity of peptaibols may be well below the threshold of human consequence, and it may require direct contact with cell membranes, like in the case of common amphiphilic detergents. This is supported by previous observations demonstrating the very low toxicity of various peptaibols orally administered to rodents and ruminants (Hou et al., 1972; Nayar et al., 1973; Hino et al., 1994).
In conclusion, negative effects on Arabidopsis plants could not be detected below a certain concentration of the purified peptaibol extract from T. reesei QM9414, which could still inhibit plant pathogenic filamentous fungi. This observation suggests that purified peptaibol extracts may have potential value for plant protection. T. reesei is a well-characterized, widely used cellulase producer in the biotechnological industry, and so its peptaibols could be produced as the main product, or a valuable by-product of fermentation.
Data Availability
All datasets generated for this study are included in the manuscript and/or the Supplementary Files.
Author Contributions
LK, TM, AS, and CV designed the study and coordinated the draft of the manuscript. TM and DB took part in the extraction, HPLC separation, sequence determination, and antifungal activity testing of the peptaibol compounds. GE, DR, and AS conducted the mass spectrometry measurements. ID performed the sequence alignments and the comparative sequence analysis of peptaibol profiles. PU performed the annotation and bioinformatic analysis of NRPS gene clusters. CT contributed with the molecular dynamics simulations of peptaibols. TM, ÁS, and LB designed and performed the bioactivity tests on A. thaliana. MA and HS conducted the bioactivity assays on mammalian cells. TM, LK, and CV analyzed the results and designed the figures and tables. All authors read and approved the final manuscript.
Funding
This work was supported by the Hungarian Scientific Research Fund by grant K-116475, by the Széchenyi 2020 Programme (GINOP-2.3.2-15-2016-00052) as well as by the COST action 16110 (HuPlantControl). LK is grantee of the János Bolyai Research Scholarship (Hungarian Academy of Sciences) and the New National Excellence Programme (ÚNKP-18-4). Open access publication was supported by the University of Szeged.
Conflict of Interest Statement
The authors declare that the research was conducted in the absence of any commercial or financial relationships that could be construed as a potential conflict of interest.
Acknowledgments
The authors wish to thank Prof. Cristian P. Kubicek and the Joint Genome Institute for the opportunity to use the genomes of T. longibrachiatum and T. citrinoviride for the bioinformatic analysis of NRPSs, Prof. Rainer Schuhmacher for kindly providing the offline version of the Peptaibiotics Database, as well as Dr. Ekaterina Shelest for her valuable suggestions regarding NRPS sequence mining.
Supplementary Material
The Supplementary Material for this article can be found online at: https://www.frontiersin.org/articles/10.3389/fmicb.2019.01434/full#supplementary-material
References
Andersson, M. A., Mikkola, R., Rasimus, S., Hoornstra, D., Salin, P., Rahkila, R., et al. (2010). Boar spermatozoa as a biosensor for detecting toxic substances in indoor dust and aerosols. Toxicol. In Vitro 24, 2041–2052. doi: 10.1016/j.tiv.2010.08.011
Andersson, M. A., Mikkola, R., Raulio, M., Kredics, L., Maijala, P., and Salkinoja-Salonen, M. S. (2009). Acrebol, a novel toxic peptaibol produced by an Acremonium exuviarum indoor isolate. J. Appl. Microbiol. 106, 909–923. doi: 10.1111/j.1365-2672.2008.04062.x
Antal, Z., Kredics, L., Pakarinen, J., Dóczi, I., Andersson, M., Salkinoja-Salonen, M., et al. (2005). Comparative study of potential virulence factors in human pathogenic and saprophytic Trichoderma longibrachiatum strains. Acta Microbiol. Immunol. Hung. 52, 341–350. doi: 10.1556/AMicr.52.2005.3-4.6
Atanasova, L., Jaklitsch, W. M., Komon-Zelazowska, M., Kubicek, C. P., and Druzhinina, I. S. (2010). Clonal species Trichoderma parareesei sp. nov. likely resembles the ancestor of the cellulase producer Hypocrea jecorina/T. reesei. Appl. Environ. Microbiol. 76, 7259–7267. doi: 10.1128/AEM.01184-10
Belayneh Mulaw, T., Kubicek, C. P., and Druzhinina, I. S. (2010). The rhizosphere of Coffea arabica in its native highland forests of Ethiopia provides a niche for a distinguished diversity of Trichoderma. Diversity 2, 527–549. doi: 10.3390/d2040527
Bencsik, O., Papp, T., Berta, M., Zana, A., Forgó, P., Dombi, G., et al. (2014). Ophiobolin A from Bipolaris oryzae perturbs motility and membrane integrities of porcine sperm and induces cell death on mammalian somatic cell lines. Toxins 6, 2857–2871. doi: 10.3390/toxins6092857
Bisby, G. R. (1939). Trichoderma viride Pers. ex Fries, and notes on Hypocrea. Trans. Br. Mycol. Soc. 23, 149–168. doi: 10.1016/S0007-1536(39)80020-1
Bissett, J. (1984). A revision of the genus Trichoderma. I. Section Longibrachiatum sect. nov. Can. J. Bot. 62, 924–931. doi: 10.1139/b84-131
Bissett, J. (1991a). A revision of the genus Trichoderma. II. Infrageneric classification. Can. J. Bot. 69, 2357–2372. doi: 10.1139/b91-297
Bissett, J. (1991b). A revision of the genus Trichoderma. III. Section Pachybasium. Can. J. Bot. 69, 2373–2417. doi: 10.1139/b91-298
Bissett, J. (1991c). A revision of the genus Trichoderma. IV. Additional notes on section Longibrachiatum. Can. J. Bot. 69, 2418–2420. doi: 10.1139/b91-299
Bissett, J., Gams, W., Jaklitsch, W., and Samuels, G. J. (2015). Accepted Trichoderma names in the year 2015. IMA Fungus 6, 263–295. doi: 10.5598/imafungus.2015.06.02.02
Bissett, J., Szakacs, G., Nolan, C. A., Druzhinina, I., Gradinger, C., and Kubicek, C. P. (2003). New species of Trichoderma from Asia. Can. J. Bot. 81, 570–586. doi: 10.1139/b03-051
Blin, K., Wolf, T., Chevrette, M. G., Lu, X., Schwalen, C. J., Kautsar, S. A., et al. (2017). antiSMASH 4.0—improvements in chemistry prediction and gene cluster boundary identification. Nucl. Acids Res. 45, W36–W41. doi: 10.1093/nar/gkx319
Brückner, H., and Graf, H. (1983). Paracelsin, a peptide antibiotic containing α-aminoisobutyric acid, isolated from Trichoderma reesei Simmons Part A. Experientia 39, 528–530. doi: 10.1007/BF01965190
Brückner, H., Kirschbaum, J., and Jaworski, A. (2002). “Sequences of peptaibol antibiotics trichoaureocins from Trichoderma aureoviride,” in Proceedings of the 27th European Peptide Symposium (Sorrento), 362–363.
Brückner, H., and Przybylski, M. (1984). Methods for the rapid detection, isolation and sequence determination of “peptaibols” and other aib-containing peptides of fungal origin. I. Gliodeliquescin a from Gliocladium deliquescens. Chromatographia 19, 188–189. doi: 10.1007/BF02687737
Bushley, K. E., and Turgeon, B. G. (2010). Phylogenomics reveals subfamilies of fungal nonribosomal peptide synthetases and their evolutionary relationships. BMC Evol. Biol. 10:26. doi: 10.1186/1471-2148-10-26
Castagnoli, E., Marik, T., Mikkola, R., Kredics, L., Andersson, M. A., Salonen, H., et al. (2018). Indoor Trichoderma strains emitting peptaibols in guttation droplets. J. Appl. Microbiol. 125, 1408–1422. doi: 10.1111/jam.13920
Chaverri, P., Branco-Rocha, F., Jaklitsch, W., Gazis, R., Degenkolb, T., and Samuels, G. J. (2015). Systematics of the Trichoderma harzianum species complex and the re-identification of commercial biocontrol strains. Mycologia 107, 558–590. doi: 10.3852/14-147
Contreras-Cornejo, H. A., Macías-Rodríguez, L., del-Val, E., and Larsen, J. (2016). Ecological functions of Trichoderma spp. and their secondary metabolites in the rhizosphere: interactions with plants. FEMS Microbiol. Ecol. 92:fiw036. doi: 10.1093/femsec/fiw036
Daniel, J. F. D. S., and Rodrigues Filho, R. E. (2007). Peptaibols of Trichoderma. Nat. Prod. Rep. 24, 1128–1141. doi: 10.1039/b618086h
De Zotti, M., Damato, F., Formaggio, F., Crisma, M., Schievano, E., Mammi, S., et al. (2010). Total synthesis, characterization, and conformational analysis of the naturally occurring hexadecapeptide integramide A and a diastereomer. Chemistry 16, 316–327. doi: 10.1002/chem.200900945
Degenkolb, T., Berg, A., Gams, W., Schlegel, B., and Gräfe, U. (2003). The occurrence of peptaibols and structurally related peptaibiotics in fungi and their mass spectrometric identification via diagnostic fragment ions. J. Pept. Sci. 9, 666–678. doi: 10.1002/psc.497
Degenkolb, T., Fog Nielsen, K., Dieckmann, R., Branco-Rocha, F., Chaverri, P., Samuels, G. J., et al. (2015). Peptaibol, secondary-metabolite, and hydrophobin pattern of commercial biocontrol agents formulated with species of the Trichoderma harzianum complex. Chem. Biodivers. 12, 662–684. doi: 10.1002/cbdv.201400300
Degenkolb, T., Gräfenhan, T., Nirenberg, H. I., Gams, W., and Brückner, H. (2006). Trichoderma brevicompactum complex: Rich source of novel and recurrent plant-protective polypeptide antibiotics (peptaibiotics). J. Agric. Food Chem. 54, 7047–7061. doi: 10.1021/jf060788q
Degenkolb, T., Karimi Aghcheh, R., Dieckmann, R., Neuhof, T., Baker, S. E., Druzhinina, I. S., et al. (2012). The production of multiple small peptaibol families by single 14-module peptide synthetases in Trichoderma/Hypocrea. Chem. Biodivers. 9, 499–535. doi: 10.1002/cbdv.201100212
Degenkolb, T., Kirschbaum, J., and Brückner, H. (2007). New sequences, constituents, and producers of peptaibiotics: an updated review. Chem. Biodivers. 4, 1052–1067. doi: 10.1002/cbdv.200790096
Degenkolb, T., Röhrich, C. R., Vilcinscas, A., von Döhren, H., and Brückner, H. (2016). A new family of N-terminally truncated peptaibols from the biocontrol fungus Trichoderma harzianum. J. Pept. Sci. 22:S98. doi: 10.1002/psc.2897
Degenkolb, T., von Döhren, H., Nielsen, K. F., Samuels, G. J., and Brückner, H. (2008). Recent advances and future prospects in peptaibiotics, hydrophobin, and mycotoxin research, and their importance for chemotaxonomy of Trichoderma and Hypocrea. Chem. Biodivers. 5, 671–680. doi: 10.1002/cbdv.200890064
Dornberger, K., Ihn, W., Ritzau, M., Gräfe, U., Schlegel, B., Fleck, W. F., et al. (1995). Chrysospermins, new peptaibol antibiotics from Apiocrea chrysosperma Ap101. J. Antibiot. 48, 977–989. doi: 10.7164/antibiotics.48.977
Dotson, B. R., Soltan, D., Schmidt, J., Areskoug, M., Rabe, K., Swart, C., et al. (2018). The antibiotic peptaibol alamethicin from Trichoderma permeabilises Arabidopsis root apical meristem and epidermis but is antagonised by cellulase-induced resistance to alamethicin. BMC Plant Biol. 18:165. doi: 10.1186/s12870-018-1370-x
Druzhinina, I. S., Komon-Zelazowska, M., Atanasova, L., Seidl, V., and Kubicek, C. P. (2010). Evolution and ecophysiology of the industrial producer Hypocrea jecorina (anamorph Trichoderma reesei) and a new sympatric agamospecies related to it. PLoS ONE 5:e9191. doi: 10.1371/journal.pone.0009191
Druzhinina, I. S., Komon-Zelazowska, M., Ismaiel, A., Jaklitsch, W., Mullaw, T., Samuels, G. J., et al. (2012). Molecular phylogeny and species delimitation in the section Longibrachiatum of Trichoderma. Fungal Genet. Biol. 49, 358–368. doi: 10.1016/j.fgb.2012.02.004
Druzhinina, I. S., Komon-Zelazowska, M., Kredics, L., Hatvani, L., Antal, Z., Belayneh, T., et al. (2008). Alternative reproductive strategies of Hypocrea orientalis and genetically close but clonal Trichoderma longibrachiatum, both capable of causing invasive mycoses of humans. Microbiology 154, 3447–3459. doi: 10.1099/mic.0.2008/021196-0
El-Hajji, M., Rebuffat, S., Lecommandeur, D., and Bodo, B. (1987). Isolation and sequence determination of trichorzianines A antifungal peptides from Trichoderma harzianum. Int. J. Pept. Prot. Res. 29, 207–215. doi: 10.1111/j.1399-3011.1987.tb02247.x
Engelberth, J., Koch, T., Schüler, G., Bachmann, N., Rechtenbach, J., and Boland, W. (2001). Ion channel-forming alamethicin is a potent elicitor of volatile biosynthesis and tendril coiling. Cross talk between jasmonate and salicylate signaling in lima bean. Plant Physiol. 125, 369–377. doi: 10.1104/pp.125.1.369
Gessmann, R., Axford, D., Evans, G., Brückner, H., and Petratos, K. (2012b). The crystal structure of samarosporin I at atomic resolution. J. Pept. Sci. 18, 678–684. doi: 10.1002/psc.2454
Gessmann, R., Axford, D., Owen, R. L., Brückner, H., and Petratos, K. (2012a). Four complete turns of a curved 310-helix at atomic resolution: the crystal structure of the peptaibol trichovirin I-4A in a polar environment suggests a transition to α-helix for membrane function. Acta Crystallogr. D Biol. Crystallogr. 68, 109–116. doi: 10.1107/S090744491105133X
Harman, G. E., Howell, C. R., Viterbo, A., Chet, I., and Lorito, M. (2004). Trichoderma species—opportunistic, avirulent plant symbionts. Nat. Rev. Microbiol. 2, 43–56. doi: 10.1038/nrmicro797
Harman, G. E., and Kubicek, C. P. (1998). Trichoderma and Gliocladium. Enzymes, Biological Control and Commercial Applications. London: Taylor & Francis.
Hatvani, L., Antal, Z., Manczinger, L., Szekeres, A., Druzhinina, I. S., Kubicek, C. P., et al. (2007). Green mold diseases of Agaricus and Pleurotus spp. are caused by related but phylogenetically different Trichoderma species. Phytopathology 97, 532–537. doi: 10.1094/PHYTO-97-4-0532
Hatvani, L., Manczinger, L., Vágvölgyi, C., and Kredics, L. (2013). “Trichoderma as a human pathogen,” in Trichoderma - Biology and Applications, eds P. K. Mukherjee, B. A. Horwitz, U. S. Singh, M. Mukherjee, M. Schmoll (Wallingford: CAB International), 292–313. doi: 10.1079/9781780642475.0292
He, H., Janso, J. E., Yang, H. Y., Bernan, V. S., Lin, S. L., and Yu, K. (2006). Culicinin D, an antitumor peptaibol produced by the fungus Culicinomyces clavisporus, strain LL-12I252. J. Nat. Prod. 69, 736–741. doi: 10.1021/np058133r
Hino, T., Saitoh, H., Miwa, T., Kanda, M., and Kumazawa, S. (1994). Effect of aibellin, a peptide antibiotic, on propionate production in the rumen of goats. J. Dairy Sci. 77, 3426–3431. doi: 10.3168/jds.S0022-0302(94)77285-4
Horváth, E., Brunner, S., Bela, K., Papdi, C., Szabados, L., Tari, I., et al. (2015). Exogenous salicylic acid-triggered changes in the glutathione transferases and peroxidases are key factors in the successful salt stress acclimation of Arabidopsis thaliana. Funct. Plant Biol. 42, 1129–1140. doi: 10.1071/FP15119
Hou, C. T., Ciegler, A., and Hesseltine, C. W. (1972). New mycotoxin, trichotoxin A, from Trichoderma viride isolated from southern leaf blight-infected corn. Appl. Microbiol. 23, 183–185.
Huang, Q., Tezuka, Y., Hatanaka, Y., Kikuchi, T., Nishi, A., and Tubaki, K. (1995). Studies on metabolites of mycoparasitic fungi. IV. Minor peptaibols of Trichoderma kiningii. Chem. Pharm. Bull. 43, 1663–1667. doi: 10.1248/cpb.43.1663
Huang, Q., Tezuka, Y., Hatanaka, Y., Kikuchi, T., Nishi, A., and Tubaki, K. (1996). Studies on metabolites of mycoparasitic fungi. V. Ion-spray ionization mass spectrometric analysis of trichokonin-II, a peptaibol mixture obtained from the culture broth of Trichoderma koningii. Chem. Pharm. Bull. 44, 590–593. doi: 10.1248/cpb.44.590
Huang, Q., Tezuka, Y., Kikuchi, T., and Momose, Y. (1994). Trichokonin VI, a new Ca2+ channel agonist in bullfrog cardiac myocytes. Eur. J. Pharmacol. 271, R5–R6. doi: 10.1016/0014-2999(94)90290-9
Iida, A., Okuda, M., Uesato, S., Takaishi, Y., Shingu, T., Morita, M., et al. (1990). Fungal metabolites. Part 3. Structural elucidation of antibiotic peptides, trichosporin-B-IIIb, -IIIc, -IVb, -IVc, -IVd, -VIa and -VIb from Trichoderma polysporum. Application of fast-atom bombardment mass spectrometry/mass spectrometry to peptides containing a unique Aib-Pro peptide bond. J. Chem. Soc. Perkin Trans. 1, 3249–3255. doi: 10.1039/P19900003249
Jaklitsch, W. M., Samuels, G. J., Dodd, S. L., Lu, B. S., and Druzhinina, I. S. (2006). Hypocrea rufa/Trichoderma viride: a reassessment, and description of five closely related species with and without warted conidia. Stud. Mycol. 56, 135–177. doi: 10.3114/sim.2006.56.04
Kai, K., Mine, K., Akiyama, K., Ohki, S., and Hayashi, H. (2018). Anti-plant viral activity of peptaibols, trichorzins HA II, HA V, and HA VI, isolated from Trichoderma harzianum HK-61. J. Pest. Sci. 43, D18–039. doi: 10.1584/jpestics.D18-039
Kimonyo, A., and Brückner, H. (2013). Sequences of metanicins, 20-residue peptaibols from the ascomycetous fungus CBS 597.80. Chem. Biodivers. 10, 813–826. doi: 10.1002/cbdv.201300064
Kottb, M., Gigolashvili, T., Großkinsky, D. K., and Piechulla, B. (2015). Trichoderma volatiles effecting Arabidopsis: from inhibition to protection against phytopathogenic fungi. Front. Microbiol. 6:995. doi: 10.3389/fmicb.2015.00995
Krause, C., Kirschbaum, J., and Brückner, H. (2006a). Peptaibiomics: an advanced, rapid and selective analysis of peptaibiotics/peptaibols by SPE/LC-ES-MS. Amino Acids 30, 435–443. doi: 10.1007/s00726-005-0275-9
Krause, C., Kirschbaum, J., and Brückner, H. (2007). Peptaibiomics: microheterogeneity, dynamics, and sequences of trichobrachins, peptaibiotics from Trichoderma parceramosum Bissett (T. longibrachiatum Rifai). Chem. Biodivers. 4, 1083–1102. doi: 10.1002/cbdv.200790098
Krause, C., Kirschbaum, J., Jung, G., and Brückner, H. (2006b). Sequence diversity of the peptaibol antibiotic suzukacillin-A from the mold Trichoderma viride. J. Pept. Sci. 12, 321–327. doi: 10.1002/psc.728
Kredics, L., Antal, Z., Dóczi, I., Manczinger, L., Kevei, F., and Nagy, E. (2003). Clinical importance of the genus Trichoderma. Acta Microbiol. Immunol. Hung. 50, 105–117. doi: 10.1556/AMicr.50.2003.2-3.1
Kredics, L., Szekeres, A., Czifra, D., Vágvölgyi, C., and Leitgeb, B. (2013). Recent results in alamethicin research. Chem. Biodivers. 10, 744–771. doi: 10.1002/cbdv.201200390
Kubicek, C. P., Herrera-Estrella, A., Seidl-Seiboth, V., Martinez, D. A., Druzhinina, I. S., Thon, M., et al. (2011). Comparative genome sequence analysis underscores mycoparasitism as the ancestral life style of Trichoderma. Genome Biol. 12:R40. doi: 10.1186/gb-2011-12-4-r40
Kubicek, C. P., Mikus, M., Schuster, A., Schmoll, M., and Seiboth, B. (2009). Metabolic engineering strategies for the improvement of cellulase production by Hypocrea jecorina. Biotechnol. Biofuels 2:19. doi: 10.1186/1754-6834-2-19
Kuhls, K., Lieckfeldt, E., Börner, T., and Guého, E. (1999). Molecular reidentification of human pathogenic Trichoderma isolates as Trichoderma longibrachiatum and Trichoderma citrinoviride. Med. Mycol. 37, 25–33. doi: 10.1080/02681219980000041
Kuhls, K., Lieckfeldt, E., Samuels, G. J., Kovacs, W., Meyer, W., Petrini, O., et al. (1996). Molecular evidence that the asexual industrial fungus Trichoderma reesei is a clonal derivative of the ascomycete Hypocrea jecorina. Proc. Natl. Acad. Sci. U.S.A. 93, 7755–7760. doi: 10.1073/pnas.93.15.7755
Kuhls, K., Lieckfeldt, E., Samuels, G. J., Meyer, W., Kubicek, C. P., and Börner, T. (1997). Revision of Trichoderma sect. Longibrachiatum including related teleomorphs based on analysis of ribosomal DNA internal transcribed spacer sequences. Mycologia 89, 442–460. doi: 10.1080/00275514.1997.12026803
Leclerc, G., Rebuffat, S., Goulard, C., and Bodo, B. (1998). Directed biosynthesis of peptaibol antibiotics in two Trichoderma strains. J. Antibiot. 51, 170–177. doi: 10.7164/antibiotics.51.170
Lee, S. J., Yeo, W. H., Yun, B. S., and Yoo, I. D. (1999). Isolation and sequence analysis of new peptaibol, boletusin, from Boletus spp. J. Pept. Sci. 5, 374–378.
Leitgeb, B., Szekeres, A., Manczinger, L., Vágvölgyi, C., and Kredics, L. (2007). The history of alamethicin: a review of the most extensively studied peptaibol. Chem. Biodivers. 4, 1027–1051. doi: 10.1002/cbdv.200790095
Lichtenthaler, H. K. (1987). Chlorophylls and carotenoids: pigments of photosynthetic biomembranes. Methods Enzymol. 148, 350–382. doi: 10.1016/0076-6879(87)48036-1
Maddau, L., Cabras, A., Franceschini, A., Linaldeddu, B. T., Crobu, S., Roggio, T., et al. (2009). Occurrence and characterization of peptaibols from Trichoderma citrinoviride, an endophytic fungus of cork oak, using electrospray ionization quadrupole time-of-flight mass spectrometry. Microbiology 155, 3371–3381. doi: 10.1099/mic.0.030916-0
Marahiel, M. A. (1997). Protein templates for the biosynthesis of peptide antibiotics. Chem. Biol. 4, 561–567. doi: 10.1016/S1074-5521(97)90242-8
Marahiel, M. A., Stachelhaus, T., and Mootz, H. D. (1997). Modular peptide synthetases involved in nonribosomal peptide synthesis. Chem. Rev. 97, 2651–2674. doi: 10.1021/cr960029e
Marik, T., Szekeres, A., Andersson, M. A., Salkinoja-Salonen, M., Tyagi, C., Leitgeb, B., et al. (2017b). “Bioactive peptaibols of forest-derived Trichoderma isolates from section Longibrachiatum,” in Soil Biological Communities and Ecosystem Resilience, eds M. Lukac, P. Grenni, M. Gamboni (Cham: Springer International Publishing), 277–290. doi: 10.1007/978-3-319-63336-7_17
Marik, T., Tyagi, C., Racić, G., Rakk, D., Szekeres, A., Vágvölgyi, C., et al. (2018). New 19-residue peptaibols from Trichoderma clade Viride. Microorganisms 6:85. doi: 10.3390/microorganisms6030085
Marik, T., Urbán, P., Tyagi, C., Szekeres, A., Leitgeb, B., Vágvölgyi, M., et al. (2017a). Diversity profile and dynamics of peptaibols produced by green mould Trichoderma species in interactions with their hosts Agaricus bisporus and Pleurotus ostreatus. Chem. Biodivers. 14:e1700033. doi: 10.1002/cbdv.201700033
Marik, T., Várszegi, C., Kredics, L., Vágvölgyi, C., and Szekeres, A. (2013). Mass spectrometric investigation of alamethicin. Acta Biol. Szeged. 57, 109–112.
Martinez, D., Berka, R. M., Henrissat, B., Saloheimo, M., Arvas, M., Baker, S. E., et al. (2008). Genome sequencing and analysis of the biomass-degrading fungus Trichoderma reesei (syn. Hypocrea jecorina). Nat. Biotechnol. 26, 553–560. doi: 10.1038/nbt1403
May, J. J., Kessler, N., Marahiel, M. A., and Stubbs, M. T. (2002). Crystal structure of DhbE, an archetype for aryl acid activating domains of modular nonribosomal peptide synthetases. Proc. Natl. Acad. Sci. U.S.A. 99, 12120–12125. doi: 10.1073/pnas.182156699
Metsalu, T., and Vilo, J. (2015). Clustvis: a web tool for visualizing clustering of multivariate data using Principal Component Analysis and heatmap. Nucl. Acids Res. 43:W566–W570. doi: 10.1093/nar/gkv468
Migheli, Q., González-Candelas, L., Dealessi, L., Camponogara, A., and Ramón-Vidal, D. (1998). Transformants of Trichoderma longibrachiatum overexpressing the β-1,4-endoglucanase gene egl1 show enhanced biocontrol of Pythium ultimum on cucumber. Phytopathology 88, 673–677. doi: 10.1094/PHYTO.1998.88.7.673
Mikkola, R., Andersson, M. A., Kredics, L., Grigoriev, P. A., Sundell, N., and Salkinoja-Salonen, M. S. (2012). 20-Residue and 11-residue peptaibols from the fungus Trichoderma longibrachiatum are synergistic in forming Na+/K+-permeable channels and adverse action towards mammalian cells. FEBS J. 279, 4172–4190. doi: 10.1111/febs.12010
Násztor, Z., Horváth, J., and Leitgeb, B. (2013). Structural characterization of the short peptaibols trichobrachins by molecular-dynamics methods. Chem. Biodivers. 10, 876–886. doi: 10.1002/cbdv.201200407
Nayar, P. R., Kumar, A., and Thirumalachar, M. J. (1973). Antiamoebin as feed additive for increased lactation in dairy animals. Hindustan Antibiot. Bull. 16, 93–96.
Neumann, N. K., Stoppacher, N., Zeilinger, S., Degenkolb, T., Brückner, H., and Schuhmacher, R. (2015). The peptaibiotics database–a comprehensive online resource. Chem. Biodivers. 12, 743–751. doi: 10.1002/cbdv.201400393
Nielsen, K. F., Gräfenhan, T., Zafari, D., and Thrane, U. J. (2005). Trichothecene production by Trichoderma brevicompactum. Agric. Food Chem. 53, 8190–8196. doi: 10.1021/jf051279b
North, C. L., Barranger-Mathys, M., and Cafiso, D. S. (1995). Membrane orientation of the N-terminal segment of alamethicin determined by solid-state 15N NMR. Biophys. J. 69, 2392–2397. doi: 10.1016/S0006-3495(95)80108-6
Panizel, I., Yarden, O., Ilan, M., and Carmeli, S. (2013). Eight new peptaibols from sponge-associated Trichoderma atroviride. Marine Drugs 11, 4937–4960. doi: 10.3390/md11124937
Pelagio-Flores, R., Esparza-Reynoso, S., Garnica-Vergara, A., López-Bucio, J., and Herrera-Estrella, A. (2017). Trichoderma-induced acidification is an early trigger for changes in Arabidopsis root growth and determines fungal phytostimulation. Front. Plant Sci. 8:822. doi: 10.3389/fpls.2017.00822
Peltola, J., Ritieni, A., Mikkola, R., Grigoriev, P. A., Pócsfalvi, G., Andersson, M. A., et al. (2004). Biological effects of Trichoderma harzianum peptaibols on mammalian cells. Appl. Environ. Microbiol. 70, 4996–5004. doi: 10.1128/AEM.70.8.4996-5004.2004
Pierce, L. C., Salomon-Ferrer, R., Augusto, F., de Oliveira, C., McCammon, J. A., and Walker, R. C. (2012). Routine access to millisecond time scale events with accelerated molecular dynamics. J. Chem. Theory Comput. 8, 2997–3002. doi: 10.1021/ct300284c
Pócsfalvi, G., Ritieni, A., Ferranti, P., Randazzo, G., Vékey, K., and Malorni, A. (1997). Microheterogeneity characterization of a paracelsin mixture from Trichoderma reesei using high-energy collision-induced dissociation tandem mass spectrometry. Rapid Commun. Mass Spectrom. 11, 922–930.
Putzu, M., Kara, S., Afonin, S., Grage, S. L., Bordessa, A., Chaume, G., et al. (2017). Structural behavior of the peptaibol harzianin HK VI in a DMPC bilayer: insights from MD simulations. Biophys. J. 112, 2602–2614. doi: 10.1016/j.bpj.2017.05.019
Rebuffat, S., Conraux, L., Massias, M., Auvin-Guette, C., and Bodo, B. (1993). Sequence and solution conformation of the 20-residue peptaibols, saturnisporins SA II and SA IV. Int. J. Pept. Protein Res. 41, 74–84.
Rebuffat, S., El Hajji, M., Hennig, P., Davoust, D., and Bodo, B. (1989). Isolation, sequence, and conformation of seven trichorzianines from Trichoderma harzianum. Int. J. Pept. Prot. Res. 34, 200–210. doi: 10.1111/j.1399-3011.1989.tb00231.x
Reese, E. T., Levinson, H. S., Downing, M. H., and White, W. L. (1950). Quartermaster culture collection. Farlowia 4, 45–86.
Richter, S., Cormican, M. G., Pfaller, M. A., Lee, C. K., Gingrich, R., Rinaldi, M. G., et al. (1999). Fatal disseminated Trichoderma longibrachiatum infection in an adult bone marrow transplant patient: species identification and review of the literature. J. Clin. Microbiol. 37, 1154–1160.
Rippa, S., Adenier, H., Derbaly, M., and Béven, L. (2007). The peptaibol alamethicin induces an rRNA-cleavage-associated death in Arabidopsis thaliana. Chem. Biodivers. 4, 1360–1373. doi: 10.1002/cbdv.200790116
Rippa, S., Eid, M., Formaggio, F., Toniolo, C., and Béven, L. (2010). Hypersensitive-like response to the pore-former peptaibol alamethicin in Arabidopsis thaliana. ChemBioChem 11, 2042–2049. doi: 10.1002/cbic.201000262
Rogozhin, E. A., Sadykova, V. S., Baranova, A. A., Vasilchenko, A. S., Lushpa, V. A., Mineev, K. S., et al. (2018). A novel lipopeptaibol emericellipsin A with antimicrobial and antitumor activity produced by the extremophilic fungus Emericellopsis alkalina. Molecules 23:2785. doi: 10.3390/molecules23112785
Röhrich, C. R., Iversen, A., Jaklitsch, W. M., Voglmayr, H., Berg, A., Dörfelt, H., et al. (2012). Hypopulvins, novel peptaibiotics from the polyporicolous fungus Hypocrea pulvinata, are produced during infection of its natural hosts. Fung. Biol. 116, 1219–1231. doi: 10.1016/j.funbio.2012.10.003
Röhrich, C. R., Iversen, A., Jaklitsch, W. M., Voglmayr, H., Vilcinskas, A., Nielsen, K. F., et al. (2013). Screening the biosphere: the fungicolous fungus Trichoderma phellinicola, a prolific source of hypophellins, new 17-, 18-, 19-, and 20-residue peptaibiotics. Chem. Biodivers. 10, 787–812. doi: 10.1002/cbdv.201200339
Röhrich, C. R., Jaklitsch, W. M., Voglmayr, H., Iversen, A., Vilcinskas, A., Nielsen, K. F., et al. (2014). Front line defenders of the ecological niche! Screening the structural diversity of peptaibiotics from saprotrophic and fungicolous Trichoderma/Hypocrea species. Fungal Divers. 69, 117–146. doi: 10.1007/s13225-013-0276-z
Rojo, F. G., Reynoso, M. M., Ferez, M., Chulze, S. N., and Torres, A. M. (2007). Biological control by Trichoderma species of Fusarium solani causing peanut brown root rot under field conditions. Crop Prot. 26, 549–555. doi: 10.1016/j.cropro.2006.05.006
Samuels, G. J., Ismaiel, A., Mulaw, T. B., Szakacs, G., Druzhinina, I. S., Kubicek, C. P., et al. (2012). The Longibrachiatum clade of Trichoderma: a revision with new species. Fungal Divers. 55, 77–108. doi: 10.1007/s13225-012-0152-2
Samuels, G. J., Petrini, O., Kuhls, K., Lieckfeldt, E., and Kubicek, C. P. (1998). The Hypocrea schweinitzii complex and Trichoderma sect. Longibrachiatum. Stud. Mycol. 41, 1–54.
Schirmböck, M., Lorito, M., Wang, Y. L., Hayes, C. K., Arisan-Atac, I., Scala, F., et al. (1994). Parallel formation and synergism of hydrolytic enzymes and peptaibol antibiotics, molecular mechanisms involved in the antagonistic action of Trichoderma harzianum against phytopathogenic fungi. Appl. Environ. Microbiol. 60, 4364–4370.
Schuster, A., and Schmoll, M. (2010). Biology and biotechnology of Trichoderma. Appl. Microbiol. Biotechnol. 87, 787–799. doi: 10.1007/s00253-010-2632-1
Seiboth, B., Karimi, R. A., Phatale, P. A., Linke, R., Hartl, L., Sauer, D. G., et al. (2012). The putative protein methyltransferase LAE1 controls cellulase gene expression in Trichoderma reesei. Mol. Microbiol. 84, 1150–1164. doi: 10.1111/j.1365-2958.2012.08083.x
Seidl, V., Seibel, C., Kubicek, C. P., and Schmoll, M. (2009). Sexual development in the industrial workhorse Trichoderma reesei. Proc. Natl. Acad. Sci. U.S.A. 106, 13909–13914. doi: 10.1073/pnas.0904936106
Shi, M., Chen, L., Wang, X. W., Zhang, T., Zhao, P. B., Song, X. Y., et al. (2012). Antimicrobial peptaibols from Trichoderma pseudokoningii induce programmed cell death in plant fungal pathogens. Microbiology 158, 166–175. doi: 10.1099/mic.0.052670-0
Shi, M., Wang, H. N., Xie, S. T., Luo, Y., Sun, C. Y., Chen, X. L., et al. (2010). Antimicrobial peptaibols, novel suppressors of tumor cells, targeted calcium-mediated apoptosis and autophagy in human hepatocellular carcinoma cells. Mol. Cancer 9:26. doi: 10.1186/1476-4598-9-26
Shi, W. L., Chen, X. L., Wang, L. X., Gong, Z. T., Li, S., Li, C. L., et al. (2016). Cellular and molecular insight into the inhibition of primary root growth of Arabidopsis induced by peptaibols, a class of linear peptide antibiotics mainly produced by Trichoderma spp. J. Exp. Bot. 67, 2191–2205. doi: 10.1093/jxb/erw023
Stoppacher, N., Neumann, N. K., Burgstaller, L., Zeilinger, S., Degenkolb, T., Brückner, H., et al. (2013). The comprehensive peptaibiotics database. Chem. Biodivers. 10, 734–743. doi: 10.1002/cbdv.201200427
Szekeres, A., Leitgeb, B., Kredics, L., Antal, Z., Hatvani, L., Manczinger, L., et al. (2005). Peptaibols and related peptaibiotics of Trichoderma. Acta Microbiol. Immunol. Hung. 52, 137–168. doi: 10.1556/AMicr.52.2005.2.2
Tamandegani, P. R., Zafari, D., Marik, T., Szekeres, A., Vágvölgyi, C., and Kredics, L. (2016). Peptaibol profiles of Iranian Trichoderma isolates. Acta Biol. Hung. 67, 431–441. doi: 10.1556/018.67.2016.4.9
Touati, I., Ruiz, N., Thomas, O., Druzhinina, I. S., Atanasova, L., Tabbene, O., et al. (2018). Hyporientalin A, an anti-Candida peptaibol from a marine Trichoderma orientale. World J. Microbiol. Biotechnol. 34:98. doi: 10.1007/s11274-018-2482-z
Tyagi, C., Marik, T., Szekeres, A., Vágvölgyi, C., Kredics, L., and Ötvös, F. (2019). Tripleurin XIIc: Peptide folding dynamics in aqueous and hydrophobic environment mimic using accelerated molecular dynamics. Molecules 24:358. doi: 10.3390/molecules24020358
Van Bohemen, A.-I., Zalouk-Vergnoux, A., Poirier, L., Phuong, N. N., Inguimbert, N., Ben Haj Salah, K., et al. (2016). Development and validation of LC–MS methods for peptaibol quantification in fungal extracts according to their lengths. J. Chromatogr. B Biomed. Sci. Appl. 1009–1010, 25–33. doi: 10.1016/j.jchromb.2015.11.039
Vicente-Carrillo, A. (2018). The usefulness of sperm kinematics in drug-induced toxicity assessment. Bas. Clin. Pharmacol. Toxicol. 123, 3–7. doi: 10.1111/bcpt.12994
Wada, S. I., Nishimura, T., Iida, A., Toyama, N., and Fujita, T. (1994). Primary structures of antibiotic peptides, trichocellins-A and-B from Trichoderma viride. Tetrahedron Lett. 35, 3095–3098. doi: 10.1016/S0040-4039(00)76838-9
Waghunde, R. R., Shelake, R. M., and Sabalpara, A. N. (2016). Trichoderma: A significant fungus for agriculture and environment. Afr. J. Agric. Res. 11, 1952–1965. doi: 10.5897/AJAR2015.10584
Whitmore, L., and Wallace, B. A. (2004). Analysis of peptaibol sequence composition: implications for in vivo synthesis and channel formation. Eur. Biophys. J. 33, 233–237. doi: 10.1007/s00249-003-0348-1
Wilson, M. A., Wei, C., Bjelkmar, P., Wallace, B. A., and Pohorille, A. (2011). Molecular dynamics simulation of the antiamoebin ion channel: linking structure and conductance. Biophys. J. 100, 2394–2402. doi: 10.1016/j.bpj.2011.03.054
Wolf, T., Shelest, V., Nath, N., and Shelest, E. (2016). CASSIS and SMIPS: promoter-based prediction of secondary metabolite gene clusters in eukaryotic genomes. Bioinformatics 32, 1138–1143. doi: 10.1093/bioinformatics/btv713
Xie, B. B., Qin, Q. L., Shi, M., Chen, L. L., Shu, Y. L., Luo, Y., et al. (2014). Comparative genomics provide insights into evolution of Trichoderma nutrition style. Genome Biol. Evol. 6, 379–390. doi: 10.1093/gbe/evu018
Yang, D., Pomraning, K., Kopchinskiy, A., Karimi Aghcheh, R., Atanasova, L., Chenthamara, K., et al. (2015). Genome sequence and annotation of Trichoderma parareesei, the ancestor of the cellulase producer Trichoderma reesei. Genome Announc. 3, e00885–e00815. doi: 10.1128/genomeA.00885-15
Yun, B. S., Yoo, I. D., Kim, Y. H., Kim, Y. S., Lee, S. J., Kim, K. S., et al. (2000). Peptaivirins A and B, two new antiviral peptaibols against TMV infection. Tetrahedron Lett. 41, 1429–1431. doi: 10.1016/S0040-4039(99)02308-4
Keywords: Trichoderma, Longibrachiatum, peptaibol, brevicelsin, mass spectrometry, antifungal activity, Arabidopsis, mammalian cells
Citation: Marik T, Tyagi C, Balázs D, Urbán P, Szepesi Á, Bakacsy L, Endre G, Rakk D, Szekeres A, Andersson MA, Salonen H, Druzhinina IS, Vágvölgyi C and Kredics L (2019) Structural Diversity and Bioactivities of Peptaibol Compounds From the Longibrachiatum Clade of the Filamentous Fungal Genus Trichoderma. Front. Microbiol. 10:1434. doi: 10.3389/fmicb.2019.01434
Received: 25 March 2019; Accepted: 06 June 2019;
Published: 26 June 2019.
Edited by:
Florentine Marx, Innsbruck Medical University, AustriaReviewed by:
Thomas Degenkolb, University of Giessen, GermanyYves Francois POUCHUS, Université de Nantes, France
Copyright © 2019 Marik, Tyagi, Balázs, Urbán, Szepesi, Bakacsy, Endre, Rakk, Szekeres, Andersson, Salonen, Druzhinina, Vágvölgyi and Kredics. This is an open-access article distributed under the terms of the Creative Commons Attribution License (CC BY). The use, distribution or reproduction in other forums is permitted, provided the original author(s) and the copyright owner(s) are credited and that the original publication in this journal is cited, in accordance with accepted academic practice. No use, distribution or reproduction is permitted which does not comply with these terms.
*Correspondence: László Kredics, a3JlZGljc0BiaW8udS1zemVnZWQuaHU=