- 1Grupo de Investigacion en Bioprospeccion (GIBP), Facultad de Ingenieria, Universidad de La Sabana, Chía, Colombia
- 2Department of Food Science, University of Wisconsin-Madison, Madison, WI, United States
- 3Department of Bacteriology, University of Wisconsin-Madison, Madison, WI, United States
Consumption of flavonoids has been associated with protection against cardiovascular and neurodegenerative diseases. Most dietary flavonoids are subjected to bacterial transformations in the gut where they are converted into biologically active metabolites that are more bioavailable and have distinct effects relative to the parent compounds. While some of the pathways involved in the breakdown of flavonoids are emerging, little it is known about the impact of carbon source availability and community dynamics on flavonoid metabolism. This is relevant in the gut where there is a fierce competition for nutrients. In this study, we show that metabolism of one of the most commonly consumed flavonoids, quercetin, by the gut-associated bacterium Eubacterium ramulus is dependent on interspecies cross-feeding interactions when starch is the only energy source available. E. ramulus can degrade quercetin in the presence of glucose but is unable to use starch for growth or quercetin degradation. However, the starch-metabolizing bacterium Bacteroides thetaiotaomicron, which does not metabolize quercetin, stimulates degradation of quercetin and butyrate production by E. ramulus via cross-feeding of glucose and maltose molecules released from starch. These results suggest that dietary substrates and interactions between species modulate the degradation of flavonoids and production of butyrate, thus shaping their bioavailability and bioactivity, and likely impacting their health-promoting effects in humans.
Introduction
Flavonoids are phenolic compounds produced by the secondary metabolism of plants. They are present in fruits, grains, and vegetables. Their basic structure consists of 15 carbon atoms arranged in three rings (A, B, and C). Their consumption is associated with a lower risk of suffering from cardiovascular and neurodegenerative diseases (Hertog et al., 1993; Letenneur et al., 2007; Rendeiro et al., 2015; Matias et al., 2016). Most polyphenols are poorly absorbed in the upper gastrointestinal tract (stomach, duodenum, jejunum, and ileum) and reach the colon where they are metabolized by the gut microbiota into more readily absorbable phenolic acids, increasing bioavailability of these biologically active compounds (Hollman et al., 1999; Manach et al., 2005).
Among the more than 8,000 different flavonoids characterized to date, quercetin is one of the most common in nature. It is found in apples, onions, red wine, tea, lettuce, and tomatoes and is extensively metabolized in the gastrointestinal tract (>90%; Hertog et al., 1992; Chen et al., 2005). Carbon dioxide is a major metabolite derived from quercetin metabolism in humans; a process that starts with the cleavage of the flavonoids C-ring by intestinal bacteria (Walle et al., 2001). Most members of the gut microbiota that are known to cleave the C-ring of quercetin belong to the Clostridia class; these include Flavonifractor plautii, Eubacterium ramulus, and Eubacterium oxidoreducens (Krumholz and Bryant, 1986; Braune et al., 2001; Schoefer et al., 2003). E. ramulus is a prevalent bacterial species commonly found in healthy subjects at levels ranging from 107 to 109 cells/ g of dry feces (Simmering et al., 1999). E. ramulus ferments glucose to butyrate, a major energy source of colonocytes that inhibits colon inflammation and carcinogenesis, and it has systemic effects lowering diet-induced insulin resistance (Roediger, 1982; Segain et al., 2000; Gao et al., 2009; Peng et al., 2009). In vitro studies indicate that E. ramulus requires glucose for the co-metabolization of quercetin (Schneider and Blaut, 2000). Degradation of this flavonoid results in the production of 3,4-dihydroxyphenylacetic acid (DOPAC), which has antiproliferative activity in colon cancer cells (Schneider and Blaut, 2000; Schneider et al., 2000; Gao et al., 2006). Nevertheless, glucose is rapidly absorbed in the small intestine and negligible amounts reach the colon, where E. ramulus resides (Low, 1988).
Dietary compounds that can impact microbial flavonoid metabolism in the colon are those that resist digestion in the upper gastrointestinal tract. Most carbohydrates that reach the lower gastrointestinal tract are of plant origin, including plant cell-wall and storage polysaccharides (Flint et al., 2008). Among these, the fraction of starch that escapes digestion in the small intestine; i.e., resistant starch, represents an important fermentation substrate that boosts bacterial flavonoid metabolism (Tousen et al., 2011) and butyrate production (Schwiertz et al., 2002). Starch that is incompletely digested in the upper digestive tract is naturally present in many foods, including bananas, rice, maize, and potatoes. For example, around 3% of hot potato starch and 12% of cold potato starch are resistant to digestion. It has been estimated that for individuals following a modern diet, the quantity of starch entering the colon is about 10% of starch intake, around 8–40 g/days (Cummings and Englyst, 1991). Previous work indicates that undigested starch enhances bacterial metabolism of daidzein, a soy isoflavone (Tousen et al., 2011). Furthermore, the abundance of the flavonoid-degrading bacterium, E. ramulus, is positively influenced by consumption of resistant starch (Simmering et al., 2002).
In order to get insights into the fate of flavonoids in the presence of polysaccharides in the multi-species environment of the gut, we evaluated in a simplified model of the gut microbiota the interactions between E. ramulus, which has a limited capacity to utilize polysaccharides, and Bacteroides thetaiotaomicron, which has the capacity to degrade many polysaccharides but it is unable to degrade the flavonoid, quercetin. We found that B. thetaiotaomicron liberates glucose and maltose from starch at levels that support the growth of E. ramulus and degradation of quercetin by this bacterium. Our results illustrate how cross-feeding between bacterial taxa can impact the metabolic fate of flavonoids in the gut.
Materials and Methods
Chemicals
Ammonium formate, 98% crystalline (Alfa Aesar, United States), EDTA ≥ 98.5% w/w (Sigma-Aldrich, United States), methanol HPLC-grade (Thermo Fisher Scientific, United States), ultrapure grade water purified to 18.1 MΩ⋅cm using a Barnstead water filtration system (Thermo Fisher Scientific, United States), potato starch (Sigma; 102954), and quercetin dihydrate, 97% w/w (Alfa Aesar, United States).
Bacterial Strains and Culture Conditions
Frozen stocks of E. ramulus strain ATCC 29099 and B. thetaiotaomicron VPI-5482 were diluted 1:50 in 7N minimal medium, consisting of 50 mM MOPS⋅KOH (pH 7.2), 0.2% resazurin, 2 mM tricine, 0.025% tween 80, 20 mM C2H3NaO2, 20 mM NaCl, 14 mM NH4Cl, 0.25 mM K2SO4, 0.5 mM MgCl2⋅6H2O, 0.5 mM CaCl2⋅2H2O, 10 μM FeSO4⋅7H2O, 20 mM NaHCO3, 1 mM KH2PO4, 1 μg ml-1 vitamin K3, 1.9 μM hematin, 0.2 mM histidine, 8 mM L-cysteine, 1 × ATCC trace minerals, 1 × ATCC vitamin supplement, amended with 40 mM glucose. Media were filter-sterilized (0.22 μm pore diameter). Cultures were incubated with constant agitation at 37°C overnight (OD600 = 1.5–1.7 for E. ramulus, 1.3–1.5 for B. thetaiotaomicron), then washed thrice with 10 ml of anaerobic 7N medium without glucose inside an anaerobic chamber. All centrifugations steps were done in Hungate tubes at 3,000 rpm 5 min. After the final centrifugation, E. ramulus cell suspension was resuspended in a sixth of the initial volume and B. thetaiotaomicron in half of the initial volume to produce concentrated cell suspensions with equivalent number of cells for both (an overnight culture of B. thetaiotaomicron has about 3 times more cells than E. ramulus). For a typical assay, about 150 μl of cell suspension was added to a 10 ml medium (this corresponds to about 109–1010 genome equivalents ml-1), cultures were grown at 37°C with agitation. Glucose was filter-sterilized and added at a final concentration of 40 mM. Starch was autoclaved and added at a final concentration of 1%. Quercetin dihydrate was used at a final concentration of 0.25 mg ml-1 in MilliQ water, autoclaved for 20 min, let cooled for 1 h with stirring and dispensed with stirring. Bacteria were handled inside an anaerobic chamber under an atmosphere of nitrogen (75%), carbon dioxide (20%), and hydrogen (5%).
DNA Preparation
DNA extraction was performed as previously described with modifications (Turnbaugh et al., 2009). Briefly, 300 μl aliquot of cultures with starch as sole carbon source was mixed with a solution containing 500 μl of extraction buffer (200 mM Tris pH 8.0, 200 mM NaCl, 20 mM EDTA), 200 μl of 20% SDS, 500 μl of a mixture of phenol:chloroform:isoamyl alcohol (25:24:1, pH 7.9) and 1.2 mg of 0.1-mm diameter zirconia/silica beads (BioSpec Products, United States). The suspension was then subjected to 3 min of bead beating (BioSpec Products, United States) at room temperature (RT), spun at 8,000 rpm for 5 min at RT, and then 750 μl of the top layer was transferred to a 15 ml tube (BD Falcon 12 × 75 mm, #352063) for immediate column purification (NucleoSpin, Macherey-Nagel, Switzerland). Column binding buffer NTl was used at 2.5 vol., 3 washes with washing buffer NT3 were performed and final elution was done with 25 μl of low T(10)E(0.1) buffer.
Real-Time Quantitative PCR (qPCR)
Quantitative PCRs were performed using the SsoAdvanced universal SYBR Green supermix (2X) (BioRad, 172-5270-5275) and the BioRad CFX96 Real-Time PCR Detection System. Species-specific primers for the 16S rRNA gene were used at a final concentration of 0.4 mM. Primer sequences for B. thetaiotaomicron were 5′-GCAAACTGGAGATGGCGA-3′ and 5′-AAGGTTTGGTGAGCCGTTA-3′ (Tm 62.5°C) (Tong et al., 2011) and for E. ramulus, 5′-CGGTACCTGACTAAGAAGC-3′ and 5′-AGTTTCATTCTTGCGAACG-3′ (Tm: 55°C) (Rinttilä et al., 2004). Each culture was analyzed in triplicate. DNA extractions from pure cultures of B. thetaiotaomicron or E. ramulus were used to generate standard curves using 7 serial dilutions ranging from 442 ng ml-1 to 4.42 × 10-4 ng ml-1 and 235 mg ml-1 to 2.35 × 10-4 ng ml-1, respectively (quantified by the Qubit dsDNA HS assay). The qPCR run consisted of a denaturation step (95°C for 30 sec), an amplification step (35 cycles of 95°C for 10 s, Tm for 15 s and 60°C for 30 s), and a melting cycle (65–95°C 0.5°C increment 2–5 s/step).
HPLC Analyses of Quercetin and Metabolites
Samples from 0 and 22 h cultures were thawed in ice, vortexed extensively and 400 μl were mixed with 1000 μl HPLC-grade methanol + 20 μM genistein as internal standard, the suspension was subjected to bead beating (BioSpec Products, Bartlesville) for 2 min at RT, then heated to 56°C for 20 min and spun for 10 min at 18, 000 g at RT. Then 1 ml of the supernatant was transferred to an HPLC vial and 200 μl of 10 mM ammonium formate/0.5 M EDTA buffer (pH 3.5) was added. Quercetin and its metabolites were analyzed using a Dionex UltiMate 3000 HPLC equipped with an LPG-3400 quaternary pump, a WPS-3000 analytical autosampler, a DAD-3000 diode array detector, and a FLD-3100 fluorescence detector. Separations were performed on a Kinetex 5 μm EVO C18, 100 Å, 250 × 4.6 mm column (Phenomenex, Torrance, CA, United States). Injection volumes were 5 μL. A flow rate of 1 ml min-1 was used throughout the 59 min run. The mobile phase was a binary gradient of (A) 10 mM ammonium formate and 0.3 mM ethylenediaminetetraacetic acid in water adjusted to pH 3.5 using concentrated HCl and (B) methanol. Solvents were vacuum filtered with 0.20 μm nylon membrane filters (Merk Millipore Ltd., Cork, Ireland). The gradient began at 5% B for 5 min, increased to 30% B over 30 min, increased to 95% B over 10 min, remained constant at 95% B for 5 min, decreased to 5% B over 2 min, and then re-equilibrated at 5% B for 7 min. Three-dimensional absorbance data were collected using the diode array detector and chromatograms of 280 nm absorbance were analyzed. Reportable values are shown in Supplementary Table S1 and an example chromatogram is shown in Supplementary Figure S1.
Samples were quantitated based on external calibration with injections of 10 μL over the linear range 1–125 μM for protocatechuic acid; 3,4-dihydroxyphenylacetic acid; 3,4-dihydroxyphenylpropionic acid; 3-hydroxybenzoic acid; 3-hydroxyphenylacetic acid; 3-(3-hydroxyphenyl) propionic acid; phenylacetic acid; quercetin; and genistein and 5–125 μM for benzoic acid. Some compounds could not be resolved by this method, namely 3-(3-hydroxyphenyl) propionic acid and phenylacetic acid.
Gas Chromatography–Mass Spectrometry Measurements of Butyrate
Samples were processed as described before (Krautkramer et al., 2016). Briefly, an aliquot of 50 μl of cultures incubated for 12 h with starch and quercetin and E. ramulus monocultures with glucose and quercetin (control) were mixed with 20 mM of a butyric-d7 acid, 99.5 atom % D, CDN isotopes #D-171 as internal standard, acidified with 5 μl of 33% HCl, extracted twice with Diethyl Ether, then 60 μl of each sample was mixed with 2 μl of derivatizing reagent (N-Methyl-N-tert-butyldimethylsilyltrifluoroacetamide, MTBSTFA) and incubated at RT for 2 h. For detection, 1 μl of each sample was injected in a gas chromatography–mass spectrometry (GC-MS) instrument (Agilent 7890B/5977A GC/MSD), and an Agilent DB-1 ms column was used. Oven program was: initial temperature, 40°C for 2.25 min; then 20°C min-1 to 200°C; next 100°C min-1 to 300°C, maintained for 7 min.
Determination of Glucose and Maltose
An aliquot of each culture with starch as sole carbon source was centrifuged at 11,000 ×g 5 min and glucose and maltose levels were determined by a colorimetric method (Maltose and Glucose Assay Kit; Sigma-Aldrich, MO, United States) at 0, 4, and 8 h of incubation following the manufacturer’s instructions. Standard curve: 2–10 nmol of glucose per well.
Statistical Analysis
Data was analyzed using analysis of variance (ANOVA – Minitab 18.1). Differences considered significant at p < 0.05.
Results
Eubacterium ramulus Requires an Energy Source to Metabolize Quercetin
Glucose and starch were evaluated for their capacity to promote quercetin degradation by E. ramulus. The structure of quercetin is shown in Figure 1A. We used media with no addition of a carbon source as a negative control. We found that glucose stimulates the degradation of quercetin by E. ramulus and the production of 3,4-dihydroxyphenylacetic acid (DOPAC) (Figure 1B) as the main metabolite derived from quercetin (Figure 2 and Supplementary Table S1), as previously described (Schneider and Blaut, 2000). In the presence of glucose E. ramulus also generates high levels of butyrate (Table 1). We assessed different concentrations of glucose in order to determine the lowest concentration of this monosaccharide required for the co-metabolization of 0.8 mM of quercetin. Quercetin degradation was checked by visual inspection; i.e., disappearance of the quercetin from the test tube, which is insoluble and has a yellow color. We also quantified quercetin and the main degradation product, DOPAC, by HPLC at the end of the experiment (22 h). We found that concentrations of glucose above 0.3 mM are required to stimulate detectable quercetin degradation (Supplementary Figure S2). Additionally, we observed a decrease in the population of E. ramulus when no carbon source was added but quercetin (Supplementary Table S2), suggesting cell death. Accordingly, under these conditions little production of DOPAC was detected (Figure 2). Monocultures of E. ramulus supplemented only with starch did not show growth (Figure 3A and Supplementary Table S2), as expected they accumulated little butyrate (Table 1) and DOPAC after 22 h of incubation (Figure 2). There were no signs of quercetin degradation after 4 days of incubation (data not shown). E. ramulus did not grow on starch with or without quercetin (Supplementary Figure S3).
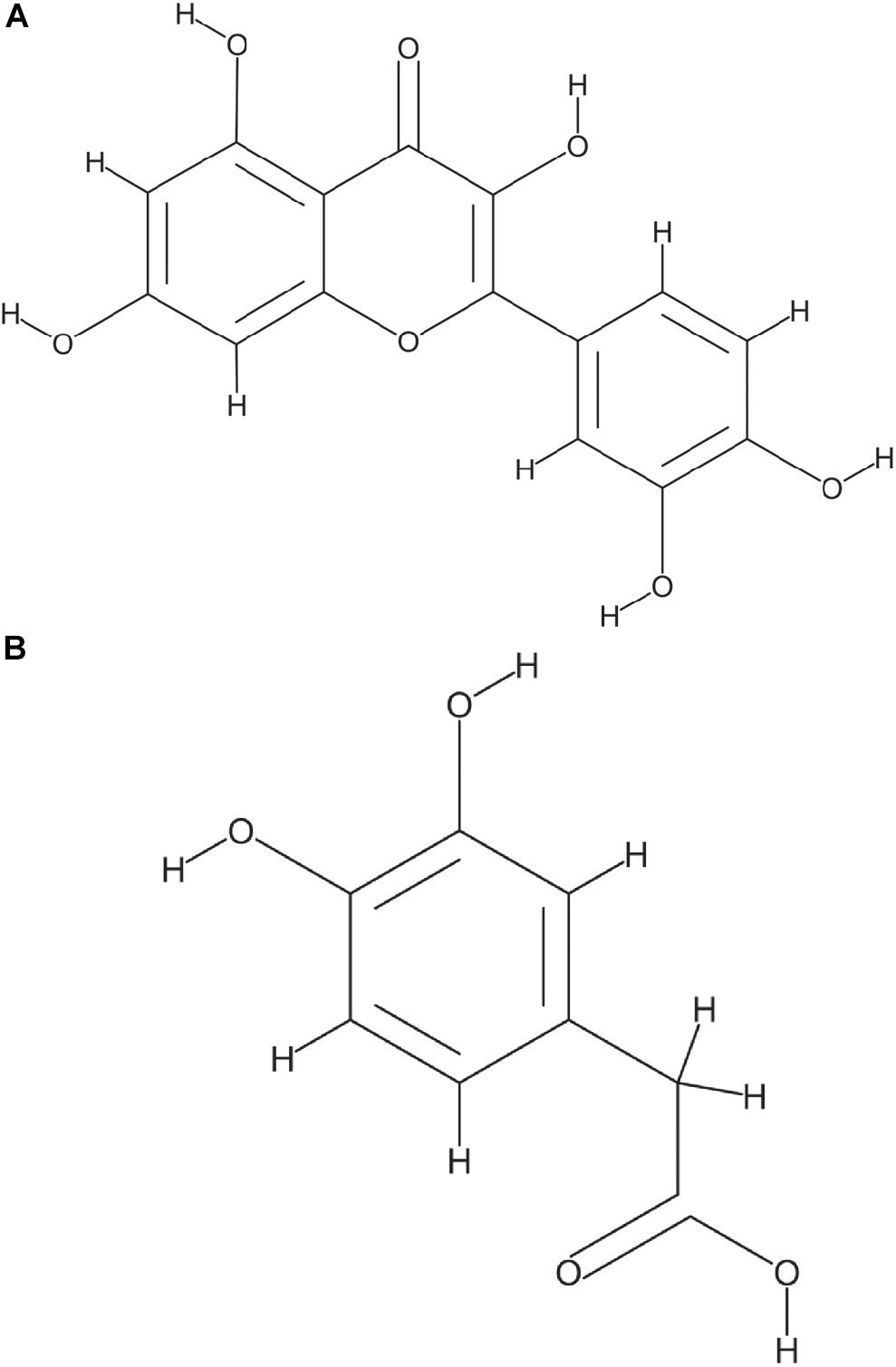
Figure 1. Structure of Quercetin (A) and 3,4-Dihydroxyphenylacetic acid (DOPAC), the main metabolite generated by E. ramulus from quercetin degradation (B) National Center for Biotechnology Information. PubChem Database, compound 5280343 and 547, respectively (https://pubchem.ncbi.nlm.nih.gov/).
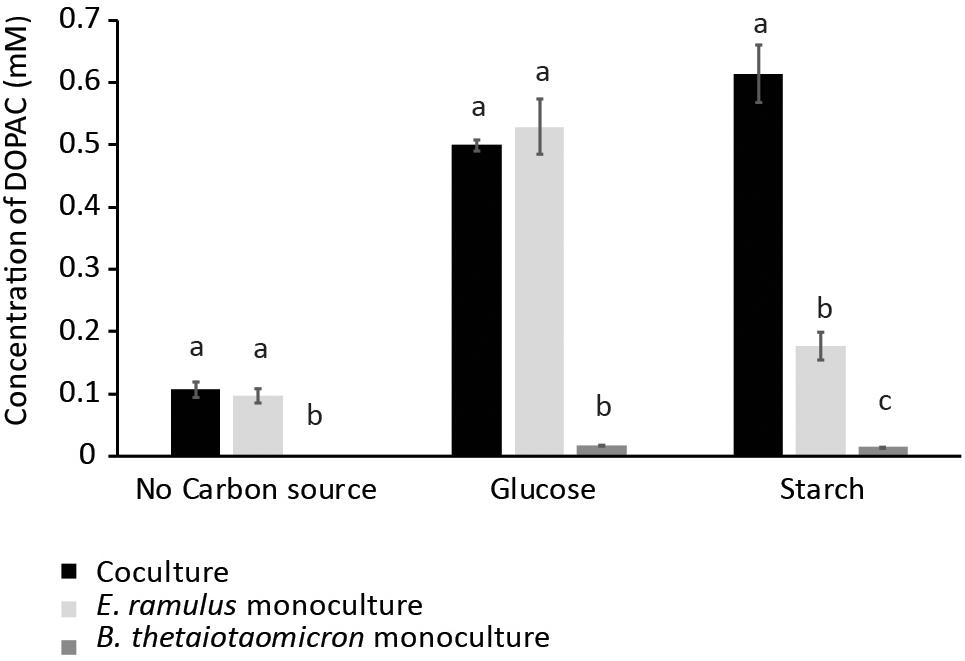
Figure 2. DOPAC concentration as measured by HPLC in cultures with no carbon source, glucose (0.7%), and starch (1%). Culture tubes were inoculated with only B. thetaiotaomicron or E. ramulus or both and incubated for 22 h. Error bars corresponds to 3 replicates. Different letters above bars indicate significant difference between type of culture at p < 0.05 according to Least Significant Difference (LSD).
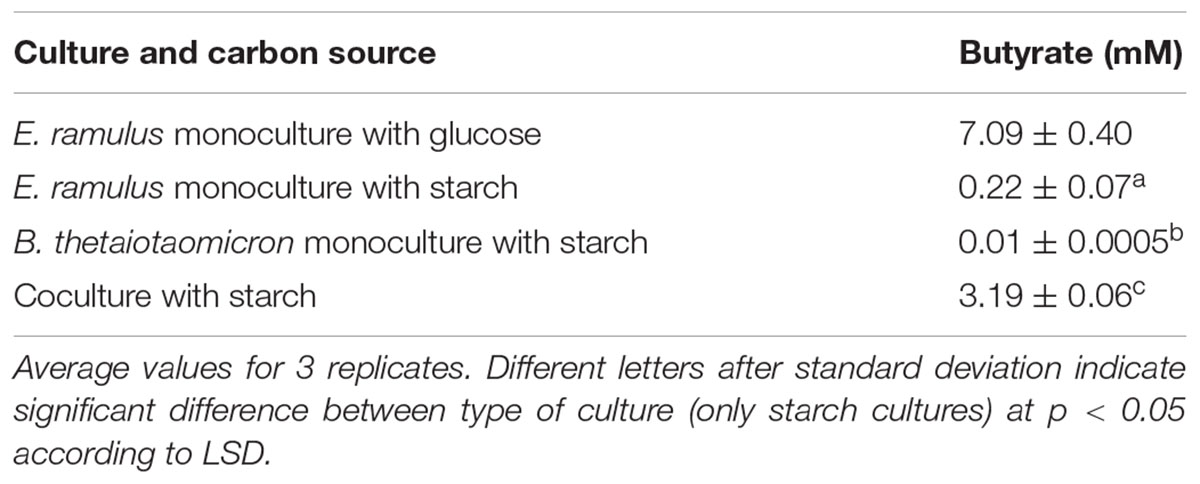
Table 1. Butyrate concentrations in monocultures and co-cultures of E. ramulus and B. thetaiotaomicron.
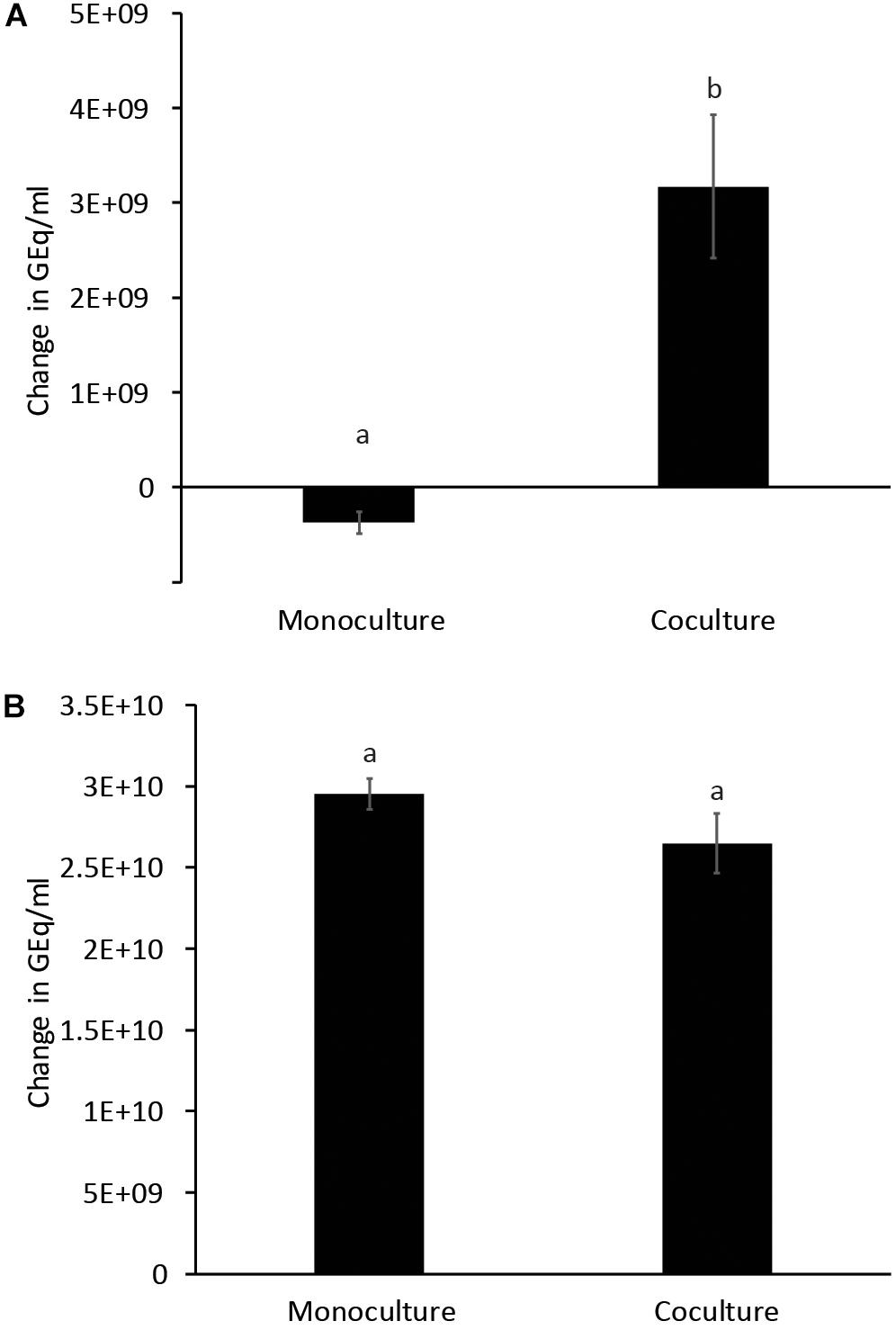
Figure 3. Growth of E. ramulus (A) and B. thetaiotaomicron (B) in cultures with 1% starch as carbon source. GEq, Genome equivalents. Change in Geq/ml between time 0 and 8 h. Results from independent experiments are presented in Supplementary Table S2. Different letters above bars indicate significant differences between type of culture at p < 0.05 according to LSD, data from 2 independent experiments (with 2–3 replicates each).
Bacteroides thetaiotaomicron Starch Utilization Enhances Butyrate Production and Quercetin Degradation by Eubacterium ramulus
We examined whether the presence of the versatile polysaccharide-metabolizing bacterium, B. thetaiotaomicron, influenced the degradation of quercetin and production of butyrate by E. ramulus. We incubated both species with quercetin and starch individually and in co-culture. We did not observe significant degradation of quercetin by either species in monoculture (Figure 2). However, a marked appearance of DOPAC (Figure 2) and enhanced growth of E. ramulus was observed in co-cultures (Figure 3A and Supplementary Table S2). Growth of E. ramulus was not enhanced in co-cultures when glucose was the sole carbon source (Supplementary Figure S4). Additionally, while butyrate was not produced by either species in monoculture incubated with starch, co-cultures accumulated high levels of butyrate, ∼44% of the amount produced by E. ramulus monocultures with 40 mM glucose (Table 1). On the other hand, the presence of E. ramulus did not change significantly the growth yield of B. thetaiotaomicron incubated with 1% starch (Figure 3B and Supplementary Table S2).
Bacteroides thetaiotaomicron Releases Glucose and Maltose From Starch
Lastly, we hypothesized that B. thetaiotaomicron promotes E. ramulus metabolism by releasing free glucose from starch. We quantified free glucose in cultures with starch as the sole carbon source (Table 2). We found that B. thetaiotaomicron releases free glucose at levels that are higher than what we found is necessary to stimulate quercetin degradation (Supplementary Figure S2), whereas co-cultures accumulated approximately 50% of the glucose of B. thetaiotaomicron monocultures (p < 0.05) (Table 2). Additionally, we detected maltose in monocultures of B. thetaiotaomicron incubated with starch at a concentration of 0.2 mM. ( ± 0.04 mM) at 4 h and 0.8 mM ( ± 0.1 mM) at 8 h of incubation. In co-cultures, there were lower concentrations of maltose, 0.08 mM ( ± 0.02 mM) after 4 h of incubation and 0.4 mM ( ± 0.2 mM) after 8 h. Accordingly, maltose also stimulated the degradation of the flavonoid by E. ramulus in monoculture (data not shown). Altogether these results suggest that E. ramulus uses glucose and maltose released by B. thetaiotaomicron from starch to grow, produce butyrate and to degrade the flavonoid.
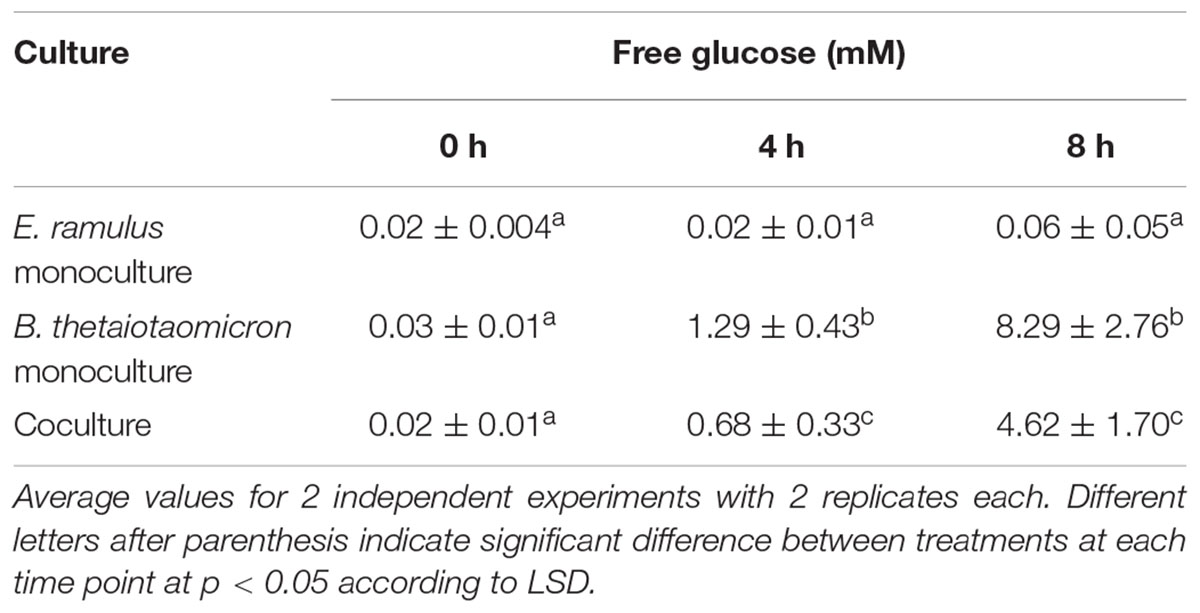
Table 2. Concentration of glucose liberated from starch by E. ramulus or B. thetaiotaomicron (monocultures) or both organisms in coculture.
Discussion
Here we evaluated how the utilization of a common carbohydrate in human diet, starch, by a member of the Bacteriodetes phylum changes the dynamics of flavonoid degradation and production of butyrate by E. ramulus. We found that metabolization of starch by B. thetaiotaomicron enhanced these processes in the Firmicute via cross-feeding of glucose and maltose released from the carbohydrate. Mahowald et al. (2009) observed in gnotobiotic mice co-colonized with Eubacterium rectale and B. thetaiotaomicron that E. rectale is better able to access nutrients and upregulates genes in the central carbon and nitrogen pathways in the presence of the B. thetaiotaomicron (Mahowald et al., 2009). One explanation for this is the observation that B. thetaiotaomicron releases simple saccharides when digesting complex carbohydrates that then Eubacterium can access. We have observed that B. thetaiotaomicron can enhance the growth of E. ramulus when growing on different oligo and polysaccharides (e.g., inulin, FOS, and arabinogalactan; data not shown), however, the most striking stimulation was on starch. B. thetaiotaomicron possess membrane-associated amylase activity, encoded by susG, which may allow the release of products of starch breakdown to the extracellular medium (Shipman et al., 1999), however, not all bacteria can access these public goods (Rakoff-Nahoum et al., 2014). Cross-feeding of starch-derived metabolites has also been reported for Bifidobacterium adolescentis, this bacterium generates resources that can be used by butyrate producers including Roseburia sp., Eubacterium hallii, and Anaerostipes caccae. This cross-feeding involves end-products (lactate or acetate) of B. adolescentis starch fermentation and potentially products released by partial hydrolysis of starch likely to be malto-oligosaccharides (Belenguer et al., 2006), however, it is not clear whether glucose is released from starch by B. adolescentis. In our studies, supplementation of acetate to the media (20 mM), in the absence of a usable carbohydrate, did not prompt the degradation of quercetin or the production of butyrate by E. ramulus.
In this work, we evaluated a soluble form of starch. Soluble starch could reach the colon entrapped in non-soluble cell-wall particles which can be released when cellulose-degrading microorganisms (e.g., Ruminococcus spp. or Enterococcus spp.) act on them releasing the soluble part. It is also possible that cellulose-degrading microorganisms could release free glucose, however, the capacity to degrade cellulose in humans seems to be limited (Robert and Bernalier-Donadille, 2003; Flint et al., 2008). B. thetaiotaomicron has a great ability to ferment soluble starch while its ability to ferment resistant starch depends on the type of resistant starch and treatment. In vitro experiments show an efficiency of >90% for autoclaved or boiled high amylopectin corn starch but <1% for raw high amylose corn starch. Thus, in the gut other players that ferment resistant starch more efficiently may potentially play an important role in providing resources for cross-feeding. It is worth noting that several butyrate-producers including E. ramulus do not have the capacity to degrade starch; non-etheless resistant starch is recognized as a butyrogenic substrate (Schwiertz et al., 2002), thus we suggest that the acquisition of luminal products of starch breakdown (e.g., glucose and maltose) by butyrate-producing species may be important for the production of butyrate.
Several studies indicate that quercetin has antibacterial activity (Rauha et al., 2000; Vaquero et al., 2007). Quercetin increases the permeability of certain bacteria to ions (Mirzoeva et al., 1997). Some species of bacteria have defense mechanisms against quercetin. For example, the plant root-colonizer, Pseudomonas putida, has an efflux pump, TtgABC, that has a high affinity for quercetin, as well as for certain antibiotics (Terán et al., 2006). Cellular targets also include enzymes like DNA gyrase and D-alanine:D-alanine ligase, essential for DNA replication and the assembly of peptidoglycan precursors, respectively, in these enzymes quercetin recognizes the conserved ATP-binding region and compete with ATP (Plaper et al., 2003; Wu et al., 2008). Some sugar transporters depend on ATP (Kaplan and Hutkins, 2003) thus the ability of using starch when quercetin was not present was evaluated for E. ramulus. Under these conditions E. ramulus was also unable to use starch. Thus, our data indicates that E. ramulus is unable to perform the initial breakdown of starch but possess the ability of using public goods generated by the breakdown of this substrate (Rakoff-Nahoum et al., 2014). In the intestine, the ability to degrade quercetin may create a temporal niche where E. ramulus can access nutrients (e.g., luminal glucose) that are in the vicinity of flavonoids and that other flavonoid-sensitive bacteria cannot access while the concentration of the flavonoid is still high.
Microbial-derived biologically active metabolites have a major impact on host’s health. Quercetin is one of the most abundant flavonoids, however, it is still not clear whether its colonic degradation is beneficial for the host since the parent compound and bacterial products derived from its degradation all have bioactivity. Studies that explore the extent to which degradation of quercetin is beneficial for the host are needed. For this goal, carbohydrates that promote more or less the degradation of the flavonoid can be useful. For example, it has been shown that fructooligosaccharides administered to the diet accelerate the use of the flavonoids rutin, quercitrin and quercetin in cecal contents of rats relative to animals supplemented with non-fermentable fiber (Juśkiewicz et al., 2011). The characterization of gut microbes able to metabolize flavonoids will make possible in the future to stratify subjects in clinical trials based on the flavonoid-degrading capacity of their gut microbiotas. Furthermore, understanding how interpersonal or disease-associated differences in gut microbial metabolism of flavonoids impact the potential benefits associated with their consumption, and identifying biomarkers for these processes will help nutritionists formulate dietary recommendations that are matched by the metabolic potential of a subject’s gut microbiota with the ultimate goal of optimizing food function efficacy.
Author Contributions
GR-C and FR conceived and planned the experiments. GR-C, MD, and XL carried out the experiments. GR-C, AA-C, BB, and FR contributed to the interpretation of the results. AA-C and FR helped to supervise the project. GR-C wrote the manuscript with input from all authors. All authors discussed the results and contributed to the final manuscript.
Funding
This research is supported by the National Institute of Food and Agriculture, United States Department of Agriculture, award number 2016-67017-24416.
Conflict of Interest Statement
The authors declare that the research was conducted in the absence of any commercial or financial relationships that could be construed as a potential conflict of interest.
Acknowledgments
We would like to thank Dr. Robert Kerby (University of Wisconsin, Madison, United States) for his support and Dr. Wilson Teran (Javeriana University, Bogota, Colombia) for his help providing resources for the qPCR. We would also like to thank the Doctorate program in Biosciences of La Sabana University and Ph.D. scholarship 647 (Colciencias, Colombia) for supporting GR-C.
Supplementary Material
The Supplementary Material for this article can be found online at: https://www.frontiersin.org/articles/10.3389/fmicb.2019.01145/full#supplementary-material
References
Belenguer, A., Duncan, S. H., Calder, A. G., Holtrop, G., Louis, P., Lobley, G. E., et al. (2006). Two routes of metabolic cross-feeding between Bifidobacterium adolescentis and butyrate-producing anaerobes from the human gut. Appl. Environ. Microbiol. 72, 3593–3599. doi: 10.1128/AEM.72.5.3593-3599.2006
Braune, A., Gütschow, M., Engst, W., and Blaut, M. (2001). Degradation of quercetin and luteolin by eubacterium ramulus. Appl. Environ. Microbiol. 67, 5558–5567. doi: 10.1128/AEM.67.12.5558-5567.2001
Chen, X., Yin, O. Q. P., Zuo, Z., and Chow, M. S. S. (2005). Pharmacokinetics and modeling of quercetin and metabolites. Pharm. Res. 22, 892–901. doi: 10.1007/s11095-005-4584-1
Cummings, J. H., and Englyst, H. N. (1991). Measurement of starch fermentation in the human large intestine. Can. J. Physiol. Pharmacol. 69, 121–129. doi: 10.1139/y91-018
Flint, H. J., Bayer, E. A., Rincon, M. T., Lamed, R., and White, B. A. (2008). Polysaccharide utilization by gut bacteria: potential for new insights from genomic analysis. Nat. Rev. Microbiol. 6, 121–131. doi: 10.1038/nrmicro1817
Gao, K., Xu, A., Krul, C., Venema, K., Liu, Y., Niu, Y., et al. (2006). Of the major phenolic acids formed during human microbial fermentation of tea, citrus, and soy flavonoid supplements, only 3,4-dihydroxyphenylacetic acid has antiproliferative activity. J. Nutr. 136, 52–57. doi: 10.1093/jn/136.1.52
Gao, Z., Yin, J., Zhang, J., Ward, R. E., Martin, R. J., Lefevre, M., et al. (2009). Butyrate improves insulin sensitivity and increases energy expenditure in mice. Diabetes 58, 1509–1517. doi: 10.2337/db08-1637
Hertog, M. G. L., Feskens, E. J. M., Kromhout, D., Hertog, M. G. L., Hollman, P. C. H., Hertog, M. G. L., et al. (1993). Dietary antioxidant flavonoids and risk of coronary heart disease: the Zutphen Elderly Study. Lancet 342, 1007–1011. doi: 10.1016/0140-6736(93)92876-U
Hertog, M. G. L., Hollman, P. C. H., and Katan, M. (1992). Content of potentially anticarcinogenic flavonoids of 28 vegetables and 9 fruits commonly used in the Netherlands. J. Agric. Food Chem. 40, 2379–2383. doi: 10.1021/jf00024a011
Hollman, P. C. H., Bijsman, M. N. C. P., Van Gameren, Y., Cnossen, E. P. J., De Vries, J. H. M., and Katan, M. B. (1999). The sugar moiety is a major determinant of the absorption of dietary flavonoid glycosides in man. Free Radic. Res. 31, 569–573. doi: 10.1080/10715769900301141
Juśkiewicz, J., Milala, J., Jurgoński, A., Król, B., and Zduńczyk, Z. (2011). Consumption of polyphenol concentrate with dietary fructo-oligosaccharides enhances cecal metabolism of quercetin glycosides in rats. Nutrition 27, 351–357. doi: 10.1016/j.nut.2010.02.002
Kaplan, H., and Hutkins, R. W. (2003). Metabolism of fructooligosaccharides by Lactobacillus paracasei 1195. Appl. Environ. Microbiol. 69, 2217–2222. doi: 10.1128/AEM.69.4.2217-2222.2003
Krautkramer, K. A., Kreznar, J. H., Romano, K. A., Vivas, E. I., Barrett-Wilt, G. A., Rabaglia, M. E., et al. (2016). Diet-microbiota interactions mediate global epigenetic programming in multiple host tissues. Mol. Cell 64, 982–992. doi: 10.1016/j.molcel.2016.10.025
Krumholz, L. R., and Bryant, M. P. (1986). Eubacterium oxidoreducens sp. nov. requiring H2 or formate to degrade gallate, pyrogallol, phloroglucinol and quercetin. Arch. Microbiol. 144, 8–14. doi: 10.1007/BF00454948
Letenneur, L., Proust-Lima, C., Le Gouge, A., Dartigues, J. F., and Barberger-Gateau, P. (2007). Flavonoid intake and cognitive decline over a 10-year period. Am. J. Epidemiol. 165, 1364–1371. doi: 10.1093/aje/kwm036
Low, A. (1988). Gut transit and carbohydrate uptake. Proc. Nutr. Soc. 47, 153–159. doi: 10.1079/pns19880025
Mahowald, M. A., Rey, F. E., Seedorf, H., Turnbaugh, P. J., Fulton, R. S., Wollam, A., et al. (2009). Characterizing a model human gut microbiota composed of members of its two dominant bacterial phyla. Proc. Natl. Acad. Sci. U.S.A. 106, 5859–5864. doi: 10.1073/pnas.0901529106
Manach, C., Williamson, G., Morand, C., Scalbert, A., and Rémésy, C. (2005). Bioavailability and bioefficacy of polyphenols in humans. I. Review of 97 bioavailability studies. Am. J. Clin. Nutr. 81, 230S–242S. doi: 10.1093/ajcn/81.1.230S
Matias, I., Buosi, A. S., and Gomes, F. C. A. (2016). Functions of flavonoids in the central nervous system: astrocytes as targets for natural compounds. Neurochem. Int. 95, 85–91. doi: 10.1016/j.neuint.2016.01.009
Mirzoeva, O. K., Grishanin, R. N., and Calder, P. C. (1997). Antimicrobial action of propolis and some of its components: the effects on growth, membrane potential and motility of bacteria. Microbiol. Res. 152, 239–246. doi: 10.1016/S0944-5013(97)80034-1
Peng, L., Li, Z.-R., Green, R. S., Holzman, I. R., and Lin, J. (2009). Butyrate enhances the intestinal barrier by facilitating tight junction assembly via activation of AMP-activated protein kinase in Caco-2 cell monolayers. J. Nutr. 139, 1619–1625. doi: 10.3945/jn.109.104638
Plaper, A., Golob, M., Hafner, I., Oblak, M., Šolmajer, T., and Jerala, R. (2003). Characterization of quercetin binding site on DNA gyrase. Biochem. Biophys. Res. Commun. 306, 530–536. doi: 10.1016/S0006-291X(03)01006-4
Rakoff-Nahoum, S., Coyne, M. J., and Comstock, L. E. (2014). An ecological network of polysaccharide utilization among human intestinal symbionts. Curr. Biol. 24, 40–49. doi: 10.1016/j.cub.2013.10.077
Rauha, J. -P., Remes, S., Heinonen, M., Hopia, A., Kähkönen, M., Kujala, T., et al. (2000). Antimicrobial effects of finnish plant extracts containing flavonoids and other phenolic compounds. Int. J. Food Microbiol. 56, 3–12. doi: 10.1016/S0168-1605(00)00218-X
Rendeiro, C., Rhodes, J. S., and Spencer, J. P. E. (2015). The mechanisms of action of flavonoids in the brain: direct versus indirect effects. Neurochem. Int. 89, 126–139. doi: 10.1016/j.neuint.2015.08.002
Rinttilä, T., Kassinen, A., Malinen, E., Krogius, L., and Palva, A. (2004). Development of an extensive set of 16S rDNA-targeted primers for quantification of pathogenic and indigenous bacteria in faecal samples by real-time PCR. J. Appl. Microbiol. 97, 1166–1177. doi: 10.1111/j.1365-2672.2004.02409.x
Robert, C., and Bernalier-Donadille, A. (2003). The cellulolytic microflora of the human colon: evidence of microcrystalline cellulose-degrading bacteria in methane-excreting subjects. FEMS Microbiol. Ecol. 46, 81–89. doi: 10.1016/S0168-6496(03)00207-1
Roediger, W. E. (1982). Utilization of nutrients by isolated epithelial cells of the rat colon. Gastroenterology 83, 424–429.
Schneider, H., and Blaut, M. (2000). Anaerobic degradation of flavonoids by Eubacterium ramulus. Arch. Microbiol. 173, 71–75. doi: 10.1007/s002030050010
Schneider, H., Simmering, R., Hartmann, L., Pforte, H., and Blaut, M. (2000). Degradation of quercetin-3-glucoside in gnotobiotic rats, associated with human intestinal bacteria. J. Appl. Microbiol. 89, 1027–1037. doi: 10.1046/j.1365-2672.2000.01209.x
Schoefer, L., Mohan, R., Schwiertz, A., Braune, A., and Blaut, M. (2003). Anaerobic degradation of flavonoids by clostridium orbiscindens. Appl. Environ. Microbiol. 69, 5849–5854. doi: 10.1128/AEM.69.10.5849-5854.2003
Schwiertz, A., Lehmann, U., Jacobasch, G., and Blaut, M. (2002). Influence of resistant starch on the SCFA production and cell counts of butyrate-producing Eubacterium spp. in the human intestine. J. Appl. Microbiol. 93, 157–162. doi: 10.1046/j.1365-2672.2002.01679.x
Segain, J. -P., Raingeard de la Blétière, D., Bourreille, A., Leray, V., Gervois, N., Rosales, C., et al. (2000). Butyrate inhibits inflammatory responses through NFκB inhibition: implications for Crohn’s disease. Gut 47, 397–403. doi: 10.1136/gut.47.3.397
Shipman, J. A., Cho, K. H., Siegel, H. A., and Salyers, A. A. (1999). Physiological characterization of SusG, an outer membrane protein essential for starch utilization by Bacteroides thetaiotaomicron. J. Bacteriol. 181, 7206–7211.
Simmering, R., Kleessen, B., and Blaut, M. (1999). Quantification of the flavonoid-degrading bacterium Eubacterium ramulus in human fecal samples with a species-specific oligonucleotide hybridization probe. Appl. Environ. Microbiol. 65, 3705–3709.
Simmering, R., Pforte, H., Jacobasch, G., and Blaut, M. (2002). The growth of the flavonoid-degrading intestinal bacterium, Eubacterium ramulus, is stimulated by dietary flavonoids in vivo. FEMS Microbiol. Ecol. 40, 243–248. doi: 10.1016/S0168-6496(02)00238-6
Terán, W., Krell, T., Ramos, J. L., and Gallegos, M. T. (2006). Effector-repressor interactions, binding of a single effector molecule to the operator-bound TtgR homodimer mediates derepression. J. Biol. Chem. 281, 7102–7109. doi: 10.1074/jbc.M511095200
Tong, J., Liu, C., Summanen, P., Xu, H., and Finegold, S. M. (2011). Application of quantitative real-time PCR for rapid identification of Bacteroides fragilis group and related organisms in human wound samples. Anaerobe 17, 64–68. doi: 10.1016/j.anaerobe.2011.03.004
Tousen, Y., Abe, F., Ishida, T., Uehara, M., and Ishimi, Y. (2011). Resistant starch promotes equol production and inhibits tibial bone loss in ovariectomized mice treated with daidzein. Metab. Clin. Exp. 60, 1425–1432. doi: 10.1016/j.metabol.2011.02.009
Turnbaugh, P. J., Hamady, M., Yatsunenko, T., Cantarel, B. L., Duncan, A., Ley, R. E., et al. (2009). A core gut microbiome in obese and lean twins. Nature 457, 480–484. doi: 10.1038/nature07540
Vaquero, M. J. R., Alberto, M. R., and de Nadra, M. C. M. (2007). Antibacterial effect of phenolic compounds from different wines. Food Control 18, 93–101. doi: 10.1016/j.foodcont.2005.08.010
Walle, T., Walle, U. K., and Halushka, P. V. (2001). Carbon dioxide is the major metabolite of quercetin in humans. J. Nutr. 131, 2648–2652. doi: 10.1093/jn/131.10.2648
Keywords: quercetin degradation, butyrate, Eubacterium ramulus, Bacteroides thetaiotaomicron, cross-feeding, starch
Citation: Rodriguez-Castaño GP, Dorris MR, Liu X, Bolling BW, Acosta-Gonzalez A and Rey FE (2019) Bacteroides thetaiotaomicron Starch Utilization Promotes Quercetin Degradation and Butyrate Production by Eubacterium ramulus. Front. Microbiol. 10:1145. doi: 10.3389/fmicb.2019.01145
Received: 06 February 2019; Accepted: 06 May 2019;
Published: 29 May 2019.
Edited by:
Satoshi Tsuneda,Waseda University, JapanReviewed by:
Nicole Marie Koropatkin, University of Michigan, United StatesDaniela De Biase, Sapienza University of Rome, Italy
Copyright © 2019 Rodriguez-Castaño, Dorris, Liu, Bolling, Acosta-Gonzalez and Rey. This is an open-access article distributed under the terms of the Creative Commons Attribution License (CC BY). The use, distribution or reproduction in other forums is permitted, provided the original author(s) and the copyright owner(s) are credited and that the original publication in this journal is cited, in accordance with accepted academic practice. No use, distribution or reproduction is permitted which does not comply with these terms.
*Correspondence: Federico E. Rey, ZmVyZXlAd2lzYy5lZHU=