- 1Graduate School of Oceanography, The University of Rhode Island, Narragansett, RI, United States
- 2Department of Fisheries, Animal and Veterinary Sciences, The University of Rhode Island, Kingston, RI, United States
- 3Department of Cell and Molecular Biology, The University of Rhode Island, Kingston, RI, United States
- 4Department of Biomedical and Pharmaceutical Sciences, College of Pharmacy, The University of Rhode Island, Kingston, RI, United States
- 5Feinstein School of Social and Natural Sciences, Roger Williams University, Bristol, RI, United States
- 6Division of Research, Florida Atlantic University, Boca Raton, FL, United States
Larval oysters in hatcheries are susceptible to diseases caused by bacterial pathogens, including Vibrio spp. Previous studies have shown that daily addition of the probiotic Bacillus pumilus RI06-95 to water in rearing tanks increases larval survival when challenged with the pathogen Vibrio coralliilyticus. We propose that the presence of probiotics causes shifts in bacterial community structure in rearing tanks, leading to a net decrease in the relative abundance of potential pathogens. During three trials spanning the 2012–2015 hatchery seasons, larvae, tank biofilm, and rearing water samples were collected from control and probiotic-treated tanks in an oyster hatchery over a 12-day period after spawning. Samples were analyzed by 16S rRNA sequencing of the V4 or V6 regions followed by taxonomic classification, in order to determine bacterial community structures. There were significant differences in bacterial composition over time and between sample types, but no major effect of probiotics on the structure and diversity of bacterial communities (phylum level, Bray–Curtis k = 2, 95% confidence). Probiotic treatment, however, led to a higher relative percent abundance of Oceanospirillales and Bacillus spp. in water and oyster larvae. In the water, an increase in Vibrio spp. diversity in the absence of a net increase in relative read abundance suggests a likely decrease in the abundance of specific pathogenic Vibrio spp., and therefore lower chances of a disease outbreak. Co-occurrence network analysis also suggests that probiotic treatment had a systemic effect on targeted members of the bacterial community, leading to a net decrease in potentially pathogenic species.
Introduction
Diseases caused by bacterial pathogens result in losses in aquaculture and wild populations of commercially important shellfish and finfish (Lafferty et al., 2015; Groner et al., 2016; Pérez-Sánchez et al., 2018). World aquaculture production is valued at $243.5 billion USD, and disease is a primary limiting factor on its growth and economic worth (Stentiford et al., 2012; FAO, 2018). Larval oysters are especially susceptible to disease, often by etiological agents from the genus Vibrio (Beaz-Hidalgo et al., 2010a; Richards et al., 2015; Le Roux et al., 2016; Dubert et al., 2017; King et al., 2018). Pathogenic Vibrio spp. are naturally occurring microbes in coastal waters, which makes them difficult to avoid. In an effort to maintain a healthy environment, hatcheries work toward optimum water quality by controlling larval culture density and the use of water treatment systems (Mckindsey et al., 2007; Pérez-Sánchez et al., 2018).
An alternative method for the management of disease in aquaculture involves the use of probiotics, microorganisms that provide health benefits to the host, including protection against bacterial pathogens. Probiotics exert their beneficial effects through a variety of mechanisms, including direct pathogen inhibition, competition for nutrients, secretion of antibacterial substances, and improvement of water quality (Kesarcodi-Watson et al., 2008, 2012; Prado et al., 2010). Previous studies have shown that treatment of larval oysters in the laboratory or the hatchery with the probiotic bacterium Bacillus pumilus RI06-95 significantly increases their survival when challenged with the pathogen Vibrio coralliilyticus (Karim et al., 2013; Sohn et al., 2016a). Additionally, administration of this probiotic in a hatchery setting results in reductions in total Vibrio abundance in tank water and surfaces, compared to the control tanks (Sohn et al., 2016b).
However, there is a lack of knowledge regarding the effects of probiotics on the systems in which they are used. There are concerns about using probiotic bacteria to combat disease in open aquaculture systems, as they will eventually disperse into the environment and may thus affect bacterial diversity in these systems (Newaj-Fyzul et al., 2014). Improper selection of probiotics may result in bacterial dysbiosis, which could ultimately impact host health (Verschuere et al., 2000). As filter feeders that process large volumes of seawater daily, bivalves are especially susceptible to changes in bacterial community composition in the water (Burge et al., 2016). Moreover, bacteria both contribute to and serve as indicators of oyster health and function of the microbial community (Le Roux et al., 2016) and likely mediate the effects of probiotics on the host. Therefore, it is important to assess the effects of probiotics not only on the health and protection of the host, but also on the bacterial communities in the systems in which oysters are grown.
Previous studies of microbiomes in adult oysters have shown differences in microbiota according to tissue type, geographic location, season, and environmental conditions (King et al., 2012; Chauhan et al., 2014; Lokmer and Wegner, 2015; Lokmer et al., 2016b; Pierce et al., 2016; Pierce and Ward, 2018). Additionally, the oyster microbiomes are distinct from those of the surrounding water and are often dominated by Proteobacteria, Cyanobacteria, and Firmicutes (Lokmer et al., 2016a). Three independent microbiome studies of larval cultures of the Pacific oyster, Crassostrea gigas found that, even though the microbiome in the rearing water changes throughout the year, there is little effect from direct manipulation of rearing conditions themselves, including salinity and temperature (Powell et al., 2013; Trabal Fernández et al., 2014; Asmani et al., 2016). Microbiome studies of juvenile Kumamoto oysters treated with Streptomyces N7 and RL8 showed an increase in species diversity and changes in the relative abundances of taxa, compared to control oysters (García Bernal et al., 2017). However, the effect of probiotics on bacterial communities in an oyster hatchery has not yet been determined.
In this study, we analyzed the structure and diversity of bacterial communities in larval oysters, their rearing water, and in tank biofilms over a 12-day period following treatment with the probiotic B. pumilus RI06-95. We hypothesized that probiotic treatment has a cascading effect on the bacterial community structure that alters the microbiomes of the rearing water, tank biofilms, and larvae, leading to a net decrease in potentially pathogenic species.
Materials and Methods
Bacterial Strain and Culture Conditions
The probiotic strain B. pumilus RI06-95, previously isolated from a marine sponge from the Pettaquamscutt River in Rhode Island (Karim et al., 2013), was cultured in yeast peptone with 3% salt (mYP30) media [5 g L-1 of peptone, 1 g L-1 of yeast extract, and 30 g L-1 of ocean salt (Red Sea Salt, Ohio, United States)] at 28°C with shaking at 170 rpm. The bacterial cell concentration was estimated by OD550 measurements using a spectrophotometer (Synergy HT, BioTek, United States) and confirmed using serial dilution and spot plating on mYP30 agar plates to determine colony forming units (CFU).
Experimental Design and Sample Collection
Samples for microbiome analysis were collected during 3 hatchery trials performed at the Blount Shellfish Hatchery at Roger William University (Bristol, RI, United States) (Table 1). Eastern oysters (Crassostrea virginica) were spawned following standard procedures (Helm and Bourne, 2004). Spawning day is referred to as Day 0 throughout the manuscript. Larvae (1-day old) were distributed and maintained in static conditions in triplicate 120 L conical tanks for each treatment containing filtered and UV sterilized seawater at 21 – 23 °C and a salinity of 28 psu. Tanks were randomly assigned to treatments including no probiotics (control) and probiotic treatment with probiotic B. pumilus RI06-95. The probiotic was administered daily at 104 CFU/mL, regardless of the length of the trial, to treatment tanks after being mixed with the microalgal feed. The microalgae strains used for feeding included Chaetoceros muelleri (CCMP1316), Isochrysis galbana (CCMP1323), Tisochrysis lutea (CCMP1324), Pavlova lutheri (CCMP1325), Tetraselmis sp. (CCMP892), and Thalassiosira weissflogii (CCMP1336). Experimental tanks were drained every other day to perform larval counts and grading. Tanks were washed thoroughly with a diluted bleach solution, rinsed, and replenished with filtered and UV-treated water prior to restocking the larvae. Sampling timepoints and trial lengths varied according to the hatchery-scheduled drain down days, so that extensive larval counts would coincide with sampling days.
Rearing water (volumes in Table 1) was collected from each of the triplicate tanks during drain-down and filtered through a 0.22 μm Sterivex filter (Millipore, Milford, MA, United States). The Sterivex filters were immediately frozen and stored at -80°C until DNA extraction. Biofilm swab samples were collected from the surface inside of each tank after drain-down of the water by swabbing a line of approximately 144 cm in length with sterile cotton swabs. The cotton tips of the swabs were stored in RNAlater (Ambion, Inc., Foster City, CA, United States). Oyster larvae were collected on a 55 μm sieve after drain-down of tank water and resuspended in 5 L of seawater. 10 mL of oyster larvae (from each tank, about 150 – 1500 larvae) were then placed into a sterile tube. In the laboratory, oyster larvae were collected on a 40 μm nylon membrane and rinsed with filtered sterile seawater (FSSW) to reduce loosely attached environmental bacteria. Swab and larvae samples were flash frozen in liquid nitrogen and stored at -80°C until DNA extraction. Extracts from swab, larvae, and water samples were cultured on selective media to perform culturable Vibrio counts following methods in Sohn et al. (2016b). All sample types were collected during Trials 1 and 2, but only water samples were collected during Trial 3 (Table 1). In Trial 3, water (1 – 2 L) was also collected from the inflow (water piped directly from the environment) and outflow (water collected after filtration and UV-treatment prior to reaching the hatchery tanks) and processed as described above for tank water.
DNA Extraction, Amplification, and Sequencing
Total DNA from water samples was extracted from the filters using the PowerWater Sterivex DNA Isolation Kit (Mo Bio Laboratories, United States) according to manufacturer recommendations (Trials 1 and 2) or Gentra Puregene Reagents (Qiagen, Hilden, Germany) with an added proteinase K-lytic enzyme digestion step (Sinigalliano et al., 2007; Trial 3). In addition, total bacterial DNA from the tank biofilm swabs and oyster larvae were extracted using the PowerSoil DNA Isolation Kit (Mo Bio) with slight modifications detailed below. In brief, frozen pooled oyster larvae were ground in a mortar with a sterile pestle and then placed into bead tubes for extraction (Qiagen). The RNAlater samples containing the cotton tops of the swabs were placed directly into bead tubes. Bead tubes were incubated at 65°C for 10 min and then shaken horizontally at maximum speed for 10 min using the Mo Bio vortex adaptor. Following extraction, DNA concentration was quantified with both a Nanodrop 2000 instrument and a Qubit Fluorometer (Thermo Fisher Scientific, Wilmington, DE, United States). The performance and quality of DNA extractions was comparable between trials and sample types.
16S rRNA gene amplicon analysis was performed using 515F/806R primers to amplify the V4 region (Trials 1 and 2) or 967F/1064R primers to amplify the V6 region (Trial 3). The V4 region was used in Trials 1 and 2 for better taxonomic resolution of all sample types and the V6 region was used in Trial 3 for independent confirmation with greater sequencing depth. A two-step PCR reaction following Illumina’s 16S Metagenomic Sequencing Library Preparation Protocol was performed on the samples from Trials 1 and 2 (Illumina Inc., San Diego, CA, United States). The PCR products were then analyzed with 250 bp paired-end sequencing to obtain fully overlapping reads on an Illumina MiSeq at the Genomics and Sequencing Center at the University of Rhode Island. The samples from Trial 3 were prepared with a 2-step fusion primer PCR amplification according to the protocols from the Keck Sequencing Center at the Marine Biological Laboratory (MBL1). Paired-end sequencing was performed at the MBL on an Illumina HiSeq 2500 to generate 100 bp paired-end reads with full overlap of the V6 region.
Processing of Sequencing Data
Sequences from Trials 1 and 2 were demultiplexed using FastQC v0.11.4 (Andrews, 2010), then merged and trimmed using Trimmomatic v0.32 (Bolger et al., 2014). All sequences shorter than 200 bp were removed from the dataset. Sequences from Trial 3 were demultiplexed and quality filtered following standard protocols at the MBL Bay Paul Center that remove reads where forward and reverse sequences do not match perfectly (Eren et al., 2013b). All sequences were uploaded to VAMPS (Visualization and Analysis of Microbial Population Structure) and classified directly using the GAST pipeline with the SILVA database, in order to compare between the three trials (Huse et al., 2014). The taxonomy data from each trial were separately normalized to the total reads of each sample to provide relative abundance of each taxa in percentage, and then exported as a matrix or BIOM file for analysis in R (Version 3.3.1). Vibrio spp. sequences in water samples from Trial 3 were processed through the oligotyping pipeline described in Eren et al. (2013a) as implemented in VAMPS, and annotated using SILVA.
Statistical and Network Analysis
All descriptive and statistical analyses were performed in the R statistical computing environment with the vegan and phyloseq packages (Dixon, 2003; McMurdie and Holmes, 2013). Simpson’s diversity values were calculated for each sample at the order level using the vegan package Version 2.4-1 and analyzed using the non-parametric Kruskal–Wallis rank sum test in R. Non-metric dimensional analysis (NMDS) was used to determine the influence of time, probiotic treatment, or sample type on the bacterial community composition, based on methods by Torondel et al. (2016) and implemented using vegan. The Bray-Curtis dissimilarity metric was calculated with k = 2 for max 50 iterations and 95% confidence intervals (standard deviation) were plotted. Statistical testing of the beta-diversity was done using the adonis2 test implemented in vegan (method = ”bray”, k = 2) (Mcardle and Anderson, 2010; Warton et al., 2012). Additionally, relative percent abundances of specific taxa were extracted and plotted according to treatment and time, and analyzed using the Kruskal–Wallis test in R.
A co-occurrence network was generated with normalized taxa counts at the Order level from water samples in Trial 3 (n = 18) to determine hypothetical relationships resulting from each treatment. The make_network() command from the phyloseq package was used with the Bray–Curtis dissimilarity metric, max distance = 0.5. The mean resulting relationship table including 123 taxa (nodes) and 670 relationships (edges) was exported to Cytoscape Version 3.6.0 for visualization and analysis (Shannon et al., 2003). Nodes were assigned continuous size attributes based on the number of normalized reads in all samples per taxa (2 to 2,720,021), and discrete shape and continuous color according to whether the taxa were more abundant in the control or probiotic-treated samples (0 to 3.6 times).
Results
Bacterial Structure and Diversity Over Time
In order to determine the effect of probiotics on the microbial community dynamics in an oyster hatchery, we needed to first characterize bacterial structure and diversity in different environmental niches within the hatchery (water, tank surfaces, and larvae) over time. A total of 18,103,647 quality-controlled 16S rRNA gene amplicon sequences were analyzed from 42 rearing water samples, 24 tank biofilm swabs, and 21 pooled larvae samples from three hatchery trials. There was an average of 208,087 reads for each of the 87 samples, ranging between 961 and 1,117,380 depending on the sequencing method and sample type (Figure 1, top). Direct taxonomical classification resulted in the detection of a total of 168 Orders across 29 Phyla in all samples. Overall, bacterial communities for each trial and sample type shared many of the most dominant phyla, although differences in relative abundance were seen between trials, timepoints, and sample types (Figure 1, bottom). The most dominant phyla in the water community, averaged from all samples, were Proteobacteria (53 ± 6%), Bacteroidetes (26 ± 10%), Cyanobacteria (12 ± 10%), Actinobacteria (5 ± 5%), and Planctomycetes (2 ± 1%) (Figure 1, bottom right). The larval samples were dominated by Proteobacteria (87 ± 12%) and the swab samples by Proteobacteria (68 ± 17%), Cyanobacteria (19 ± 16%), and Bacteroidetes (8 ± 4%) (Figure 1, bottom left). Percent abundance of Cyanobacteria was significantly higher in swab than in water samples (p < 0.001, Supplementary Table S1). Larval and swab samples showed a significantly higher proportion of Proteobacteria, and lower percent abundance of Bacteroidetes, as compared to water samples (p < 0.001, Supplementary Table S1). No significant effect of probiotic treatment was observed on the relative abundance of dominant phyla (p > 0.38).
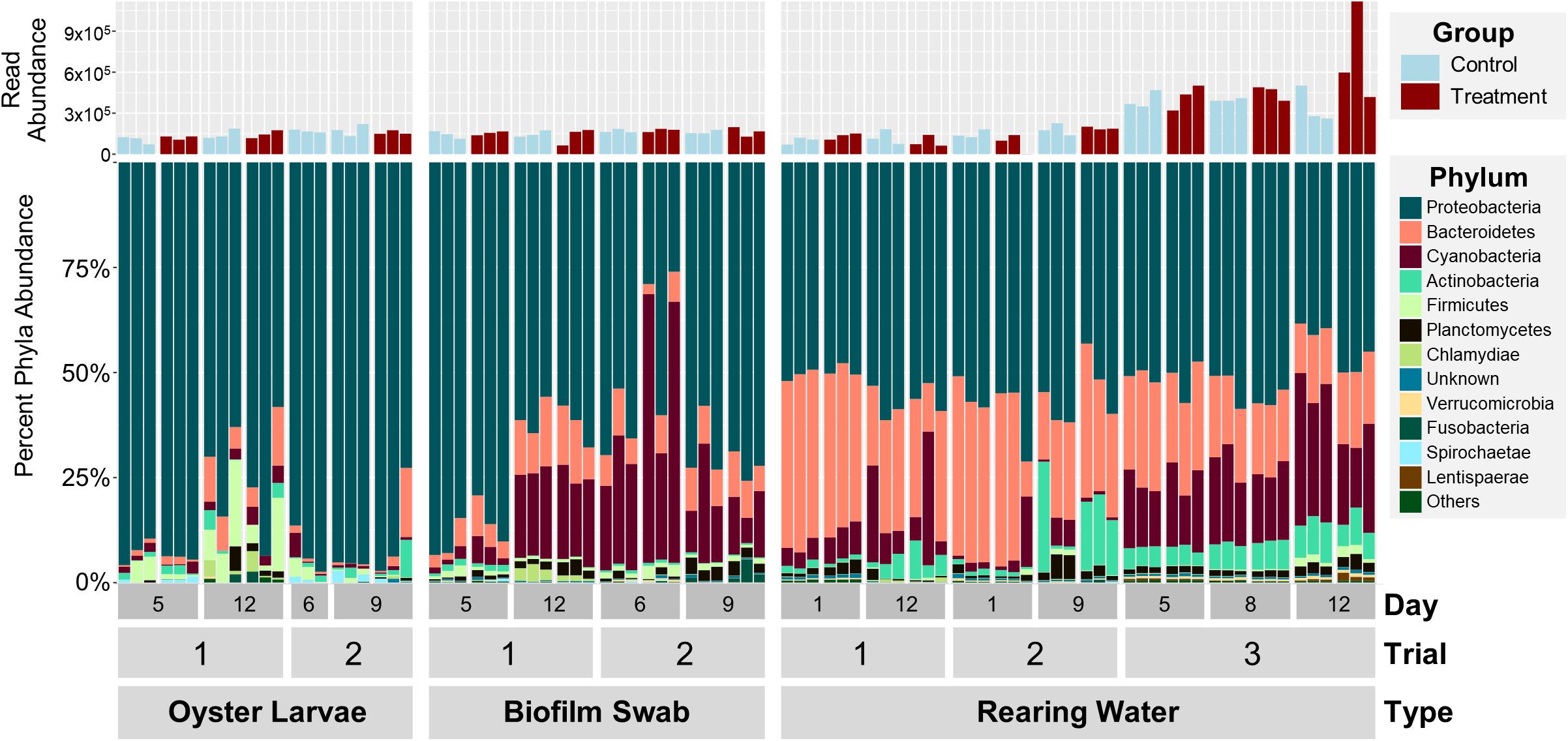
Figure 1. Percent abundances of the 12 most abundant phyla in oyster larvae, biofilm swab, and rearing water samples from all 3 trials based on 16S rRNA amplicon sequencing data (bottom). The total abundance of quality filtered sequencing reads is shown in the bar graph (top). The 12 dominant phyla include Actinobacteria, Bacteroidetes, Cyanobacteria, Deferribacteres, Firmicutes, Fusobacteria, Lentisphaerae, Planctomycetes, Proteobacteria, Spirochaetae, Verrucomicrobia, and Unknown. Note: there are no treated oyster larvae samples from Trial 2, Day 6.
Overall, the bacterial communities in rearing water were significantly more diverse than the communities in oyster larvae and tank biofilm swab samples (Simpson’s Diversity Index, p < 0.001, Figure 2 and Supplementary Table S2), reflecting an enrichment in specific community members in larvae and tank surfaces from the more diverse rearing water community (Figure 1). Simpson’s Diversity Index indicated significantly higher diversity in rearing water samples from Trial 3 (0.66 ± 0.04), than from Trials 1 (0.59 ± 0.3) and 2 (0.53 ± 0.5; p < 0.001, Figure 2, Supplementary Table S2), most probably due to the greater sequencing depth and different target 16S variable region in Trial 3 (Supplementary Figure S1), but potentially also due to seasonal and yearly differences in bacterial composition of the rearing water source (Table 1). There was high variability among replicate samples from each timepoint and treatment, especially in oyster larvae samples (Figure 2 and Supplementary Figure S2). Significant increases in bacterial diversity over time were detected in the oyster larvae and biofilm swabs in Trial 1 (p < 0.01, Supplementary Table S3), and in the rearing water in Trials 2 and 3 (p < 0.01, Figure 2 and Supplementary Tables S4, S5). No significant differences in Simpson’s Diversity Index were detected between control and treated samples at any timepoints for any of the sample types (p = 0.52).
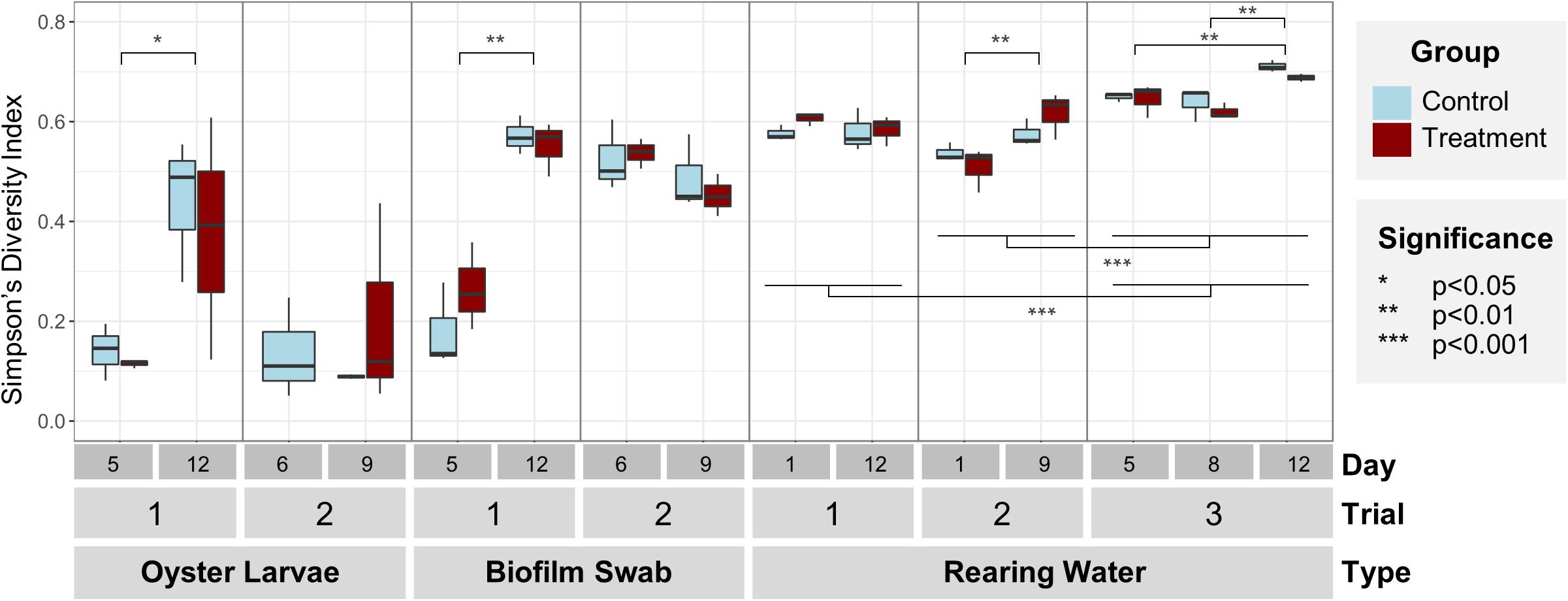
Figure 2. Simpson’s index of diversity of bacterial communities by sample (larvae, swab, and water) and trial (n = 3 tanks). No significant differences in diversity were found between control (light blue) and treatment (dark red) within each sample type and trial. Bacterial community diversity significantly increased over time in larvae and swab samples from Trial 1, and water samples from Trials 2 and 3. Diversity in water was significantly higher in Trial 3 than Trials 1 and 2. Note: there are no treated oyster larvae samples from Trial 2, Day 6.
The bacterial community structures of the water and oyster larvae samples were significantly different (Bray–Curtis, k = 2, 95% confidence, adonis2 p = 0.001) in both Trial 1 and Trial 2 (Figure 3A and Supplementary Table S6). The community structure of microbiomes in tank biofilms (swab samples) was not significantly different from the structure of either the water or oyster larvae samples, suggesting an intermediate microbiome stage. Bacterial communities in the rearing water were significantly different between sampling timepoints (Bray–Curtis, k = 2, 95% confidence, adonis2 p < 0.02) in all three trials (Figure 3B and Supplementary Table S6). Moreover, the bacterial community in samples of inflow and outflow seawater, which were collected on Days 5, 8, and 12 during Trial 3, was distinct from that of the water in rearing tanks (Supplementary Figure S3, adonis2 p = 0.001, and Supplementary Table S6). These results suggest that hatchery tanks containing oyster larvae have dynamically developing microbiomes, despite the fact that they are all receiving the same inflow seawater. There was no significant effect of treatment on the beta-diversity in water samples from all timepoints (Figure 3C and Supplementary Table S6).
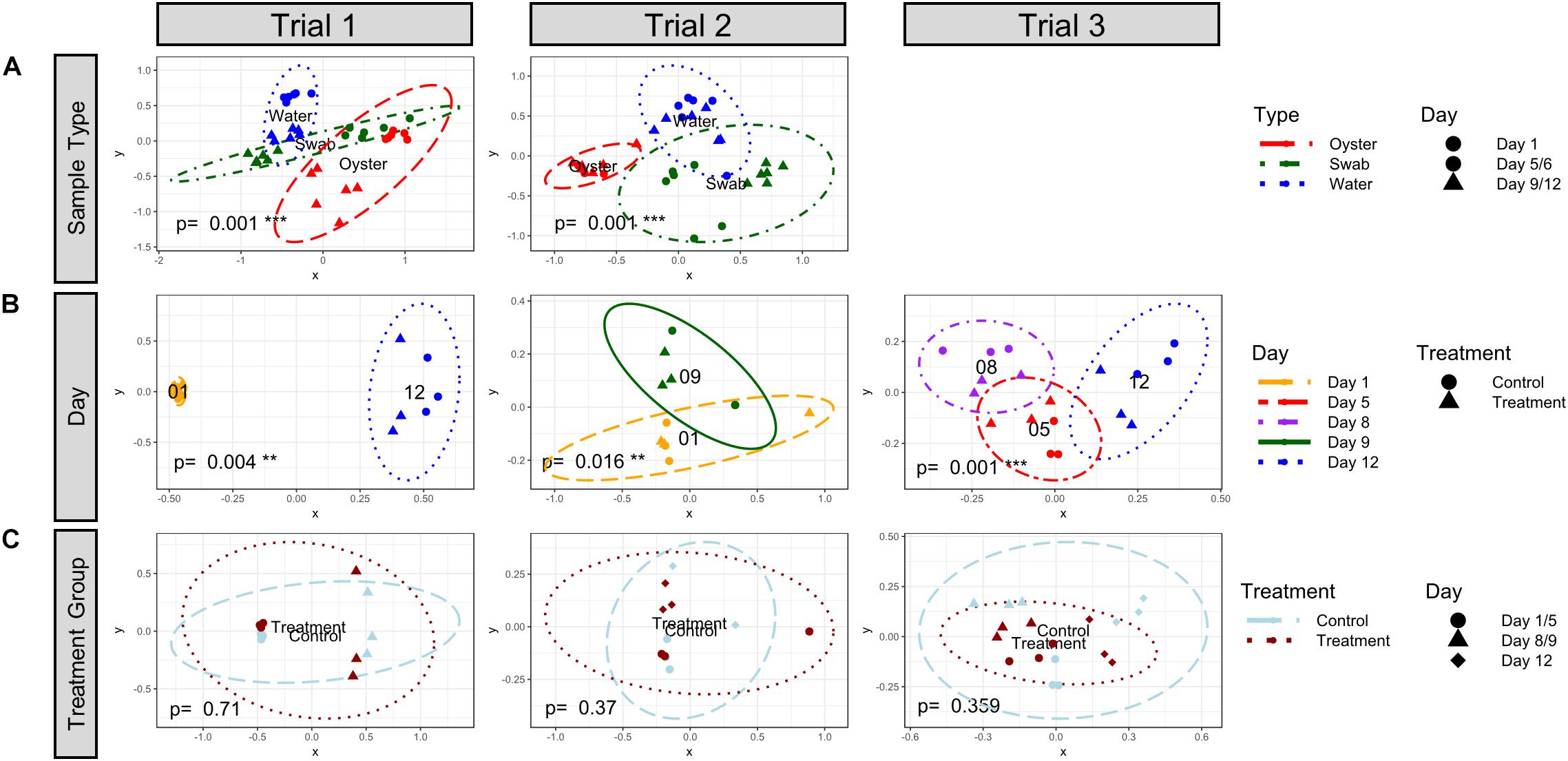
Figure 3. NMDS plot visualization of Bray-Curtis beta-diversity (k = 2) at the Order level by (A) sample type, (B) sampling day, and (C) treatment. The ellipse lines show the 95% confidence interval (standard deviation). p-values indicate significance of grouping with adonis2 Permutational Multivariate Analysis of Variance Using Distance Matrices test. (A) The different types of samples are indicated by colors (Oyster, dashed red; Swab, dashdot green; and Water, dotted blue) and the days are indicated by symbols (Timepoint 1, circle; Timepoint 2, triangle). The water and oyster communities were significantly distinct from each other in both trials. (B) The sampling timepoints are indicated by colors (1, longdash yellow; 5, shortdash red; 8, dashdot purple; 9, solid green; and 12, dotted blue) and the treatment group is indicated by symbols (control, circle; probiotic treatment, triangle). The water community was significantly different between timepoints. (C) The treatment group is indicated by colors (control, light blue dashed; probiotic treatment, dark red dotted) and sampling timepoints are indicated by symbols. No significant differences in community structure in water from control and probiotic-treated tanks was detected when samples from all timepoints were analyzed together.
Effects of the Probiotic on the Selected Members of the Bacterial Community
Although control and probiotic-treated tanks showed no significant differences in diversity and structure of bacterial communities overall (Figure 3C), significant differences in the relative read abundance of several specific taxa were detected. In all trials, Bacillales reads in the probiotic-treated water samples increased through time, and were significantly more abundant in samples from treated tanks than in the control tanks by the final sampling day in all trials (p < 0.05, Figure 4A and Supplementary Table S7). These consistent results suggest that the relative increase in reads corresponded to the added probiotic. The relative percent of Oceanospirillales reads was also significantly higher by 20–34% at all but one timepoint in probiotic-treated rearing water as compared to control water in all trials (p < 0.05, Figure 4B and Supplementary Table S8). The relative percent abundance of Oceanospirillales reads in the water significantly decreased over time by 41–62% (depending on the trial; p < 0.05, Figure 4B and Supplementary Table S8). No significant changes in relative percent read abundance of these two selected members of the bacterial community were detected in larval oysters or swabs, but percent abundance was low in these sample types (Trials 1 and 2; not shown).
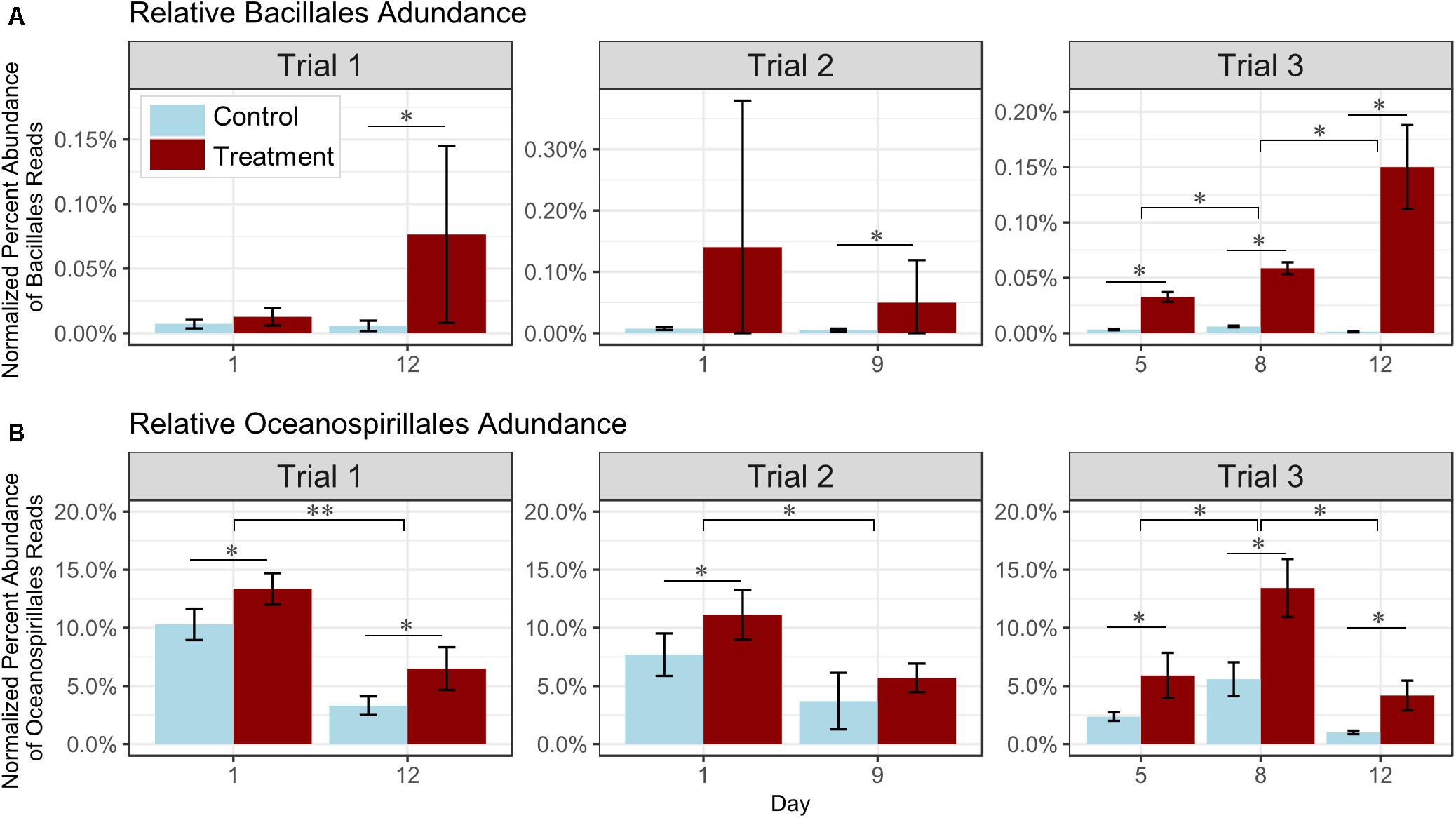
Figure 4. Effect of probiotic treatment on relative percent read abundance of (A) Bacillales and (B) Oceanospirillales in water. Number of reads in treated (dark red) and control (light blue) samples (n = 3 tanks per treatment) are represented for each sampling day and trial. (A) Bacillales was relatively significantly higher in the treated than the control water after 5 days of treatment, and (B) Oceanospirillales were consistently more abundant in probiotic-treated tank rearing water, and decreased with time. ∗p < 0.05, ∗∗p < 0.01, ∗∗∗p < 0.001.
Vibrio is a taxon that comprises a significant number of larval oyster pathogens, therefore we evaluated the effect of probiotic treatment on changes in Vibrio spp. diversity, relative abundance, and culturable colonies on selective media, over time during each of the hatchery trials (Figure 5 and Supplementary Figures S4, S5). Probiotic treatment led to a significant increase in Vibrio diversity (as measured using the Simpson’s Index of Diversity) in water samples collected on Day 12 in Trial 1 (p < 0.05; Figure 5A and Supplementary Table S9). No significant differences in relative percent abundance of Vibrio spp. between control and probiotic-treated tanks were detected for any of the sample types (Figure 5B and Supplementary Table S10). Colony counts of culturable Vibrios, however, were significantly lower in probiotic-treated tanks, relative to control tanks (p < 0.05, Figure 5C and Supplementary Table S11). When considering the effect of sample type, Vibrio relative abundance was significantly lower in water samples than in swabs or oysters (all timepoints) and in swabs than in oysters (p < 0.05, Figure 5B and Supplementary Table S10). When considering data from all timepoints together, the diversity of Vibrio spp. as detected using 16S rRNA gene sequencing was significantly higher in swab and oyster samples than in water samples (p < 0.05, Figure 5A and Supplementary Table S9). An evaluation of the effect of time on Vibrio relative abundance and diversity showed a significant increase in the diversity of Vibrio spp. in swab and water samples (Trial 1, p < 0.005, Figure 5A and Supplementary Table S9), and a significant decrease in relative abundance in all sample types (Trial 1, p < 0.005, Figure 5B and Supplementary Table S10). This decrease in abundance was further seen in colony counts of culturable Vibrios in the water samples (Trial 1, p < 0.05, Figure 5C and Supplementary Table S11).
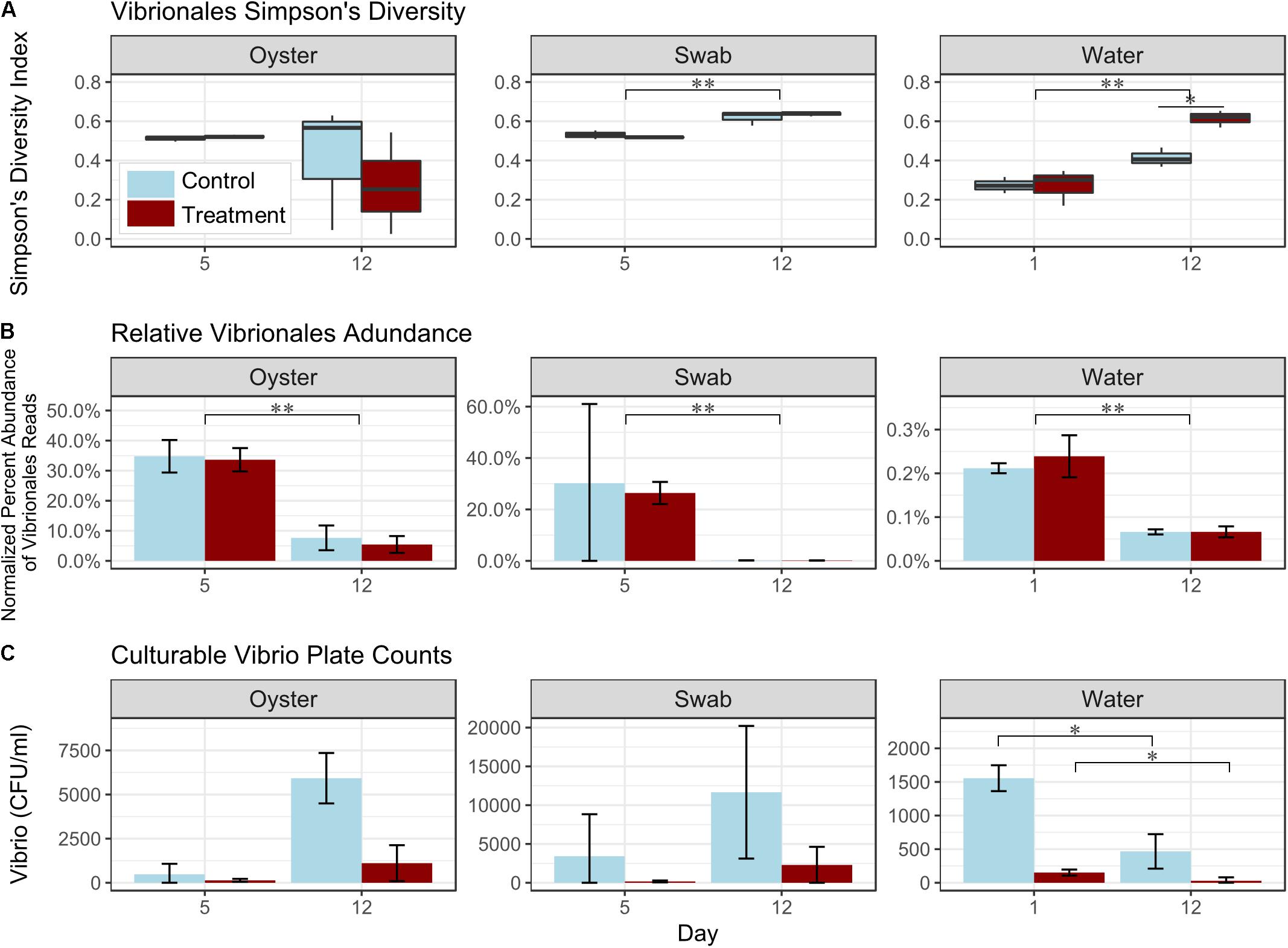
Figure 5. Effect of treatment, time, and sample type on Simpson’s Index of Diversity for Vibrionales (A, boxplots), total Vibrionales relative percent read abundance (B, bar graph), and culturable Vibrio plate counts (C, bar graph). Representative data from Trial 1 (n = 3 tanks per treatment). Note different scales for (B) and (C). ∗p < 0.05, ∗∗p < 0.01, ∗∗∗p < 0.001.
Since the V6 region of the 16S rRNA gene was deeply sequenced in Trial 3, we were able to perform an oligotyping analysis – a method that detects genetic variants within a taxon – of the 1,727 Vibrio reads in the 18 water samples. Changes in the overall composition of the Vibrio community over time and by treatment were observed by oligotyping (Figure 6). On Day 5, while the Vibrio community in control tanks was dominated by an oligotype most closely related to V. alginolyticus WW1 (64 ± 6%), probiotic tanks showed a mix of V. alginolyticus WW1 (31 ± 3%) and Halovibrio sp. 5F5 (31 ± 3%). By Day 12, the Vibrio composition in water in control tanks was dominated by V. celticus 5OM18 (75 ± 3%), while a mix of V. orientalis LK2HaP4 (51 ± 10%), and V. celticus 5OM18 (35 ± 8%) was detected in probiotic tanks.
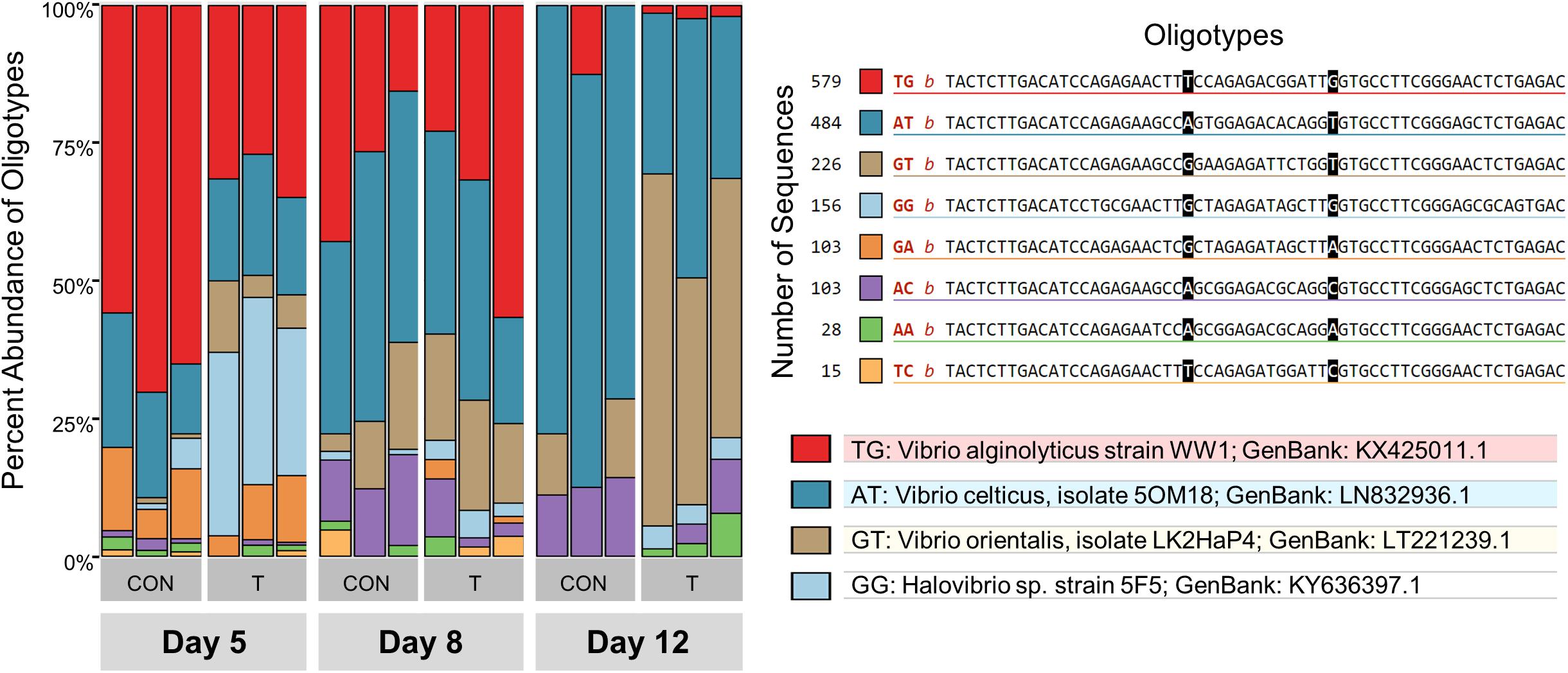
Figure 6. Vibrio spp. oligotypes in Control (CON) and Treatment (T) water samples on Days 5, 8, and 12 from Trial 3. These 8 oligotypes were generated from changes in positions 23 and 37 in a total of 1727 sequences, represented with the 2 letter abbreviations in the legend. The taxonomy of the 4 most abundant oligotypes is shown. Vibrio oligotypes showed differences in succession of species over time between control and treatment rearing water.
Bacterial Relationships With Co-occurrence Analysis
A co-occurrence analysis of members of the bacterial community (Figure 7) in the 18 water samples from Trial 3 was performed to illustrate: (a) how abundance of each Order changed relative to others (edge connections); (b) which Orders were relatively most abundant in the system (node size); and (c) how probiotic treatment affected their relative abundances (node color and shape). The most abundant taxa (Rhodobacterales, Micrococcales, Sphingobacteriales, Flavobacteriales, Deferribacterales, and Oceanospirillales) changed in similar fashion, but had different occurrence ratios between control and treatment samples. Orders that were more abundant in the treatment samples than in control samples included Oceanospirillales, Caulobacterales, Lentispherales, Acidithiobacillales, Chrococcales, and Bacillales. These nodes were scattered throughout the network and did not share direct edges, but were within 3–5 edges of each other.
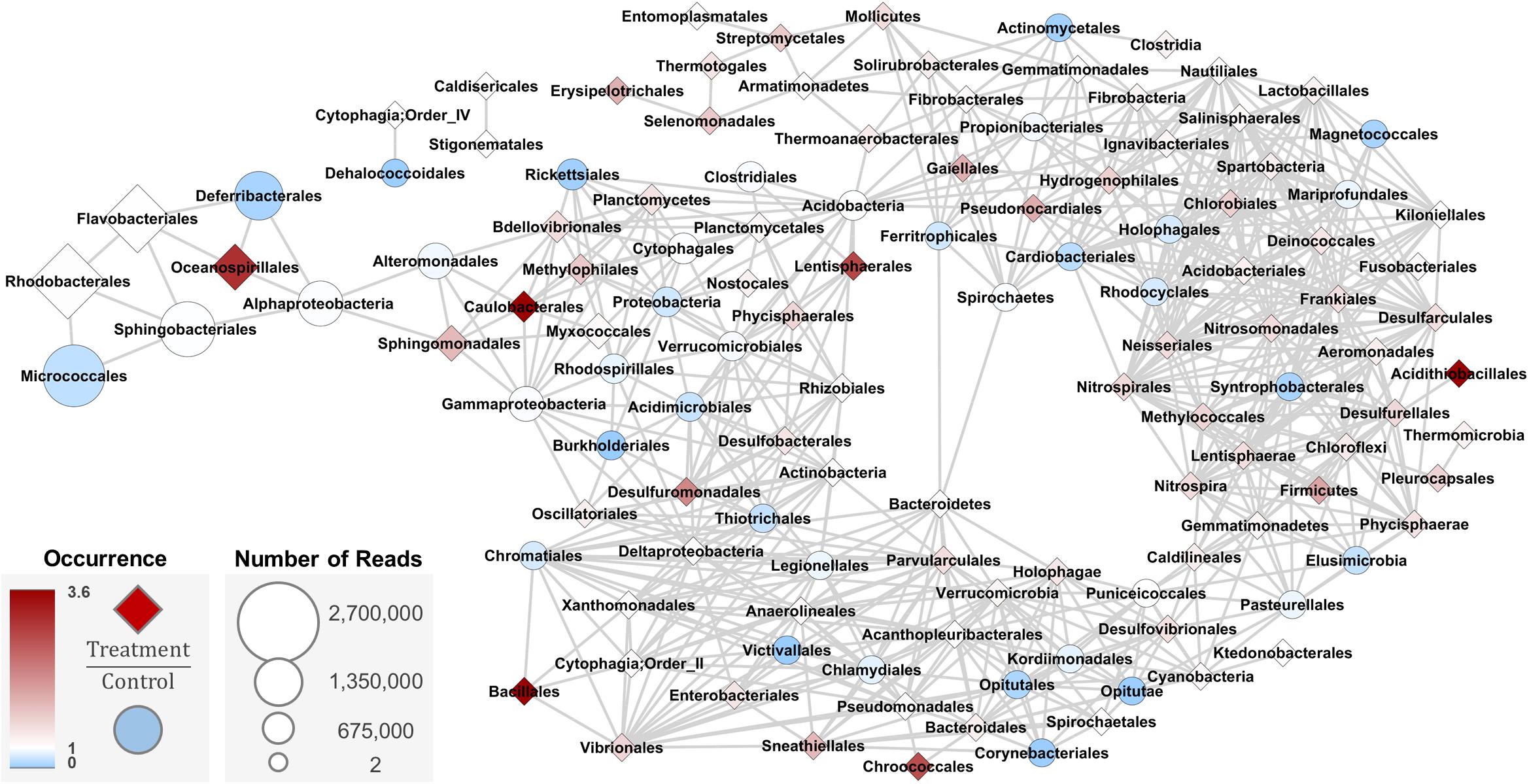
Figure 7. Co-occurrence network analysis based on Bray-Curtis dissimilarity metric (max distance = 0.5, Order level) for water samples from Trial 3 (n = 3 tanks per treatment and day, total of 18). Taxa that change in the same way share an edge; nodes that have edges occur in the same proportions and in the same samples. Darker blue circle nodes indicate taxa that occur in the Control significantly more than Treated water samples. White nodes have equal occurrence in treated and control water samples. Darker red diamond nodes indicated taxa that occur in the Treated significantly more than Control water samples.
Bacillales, the Order to which the probiotic used in these experiments belongs, was shown to be most directly associated in the network with four other Orders that changed in relative abundance between control and treatment samples: Chromatiales, Xanthomonadales, Cytophagia Order II, and Vibrionales. This direct connection between Bacillales and Vibrionales in the network indicated that the probiotic may have directly affected members of Vibrionales. Oceanospirillales was placed in the network 5 edges away from Bacillales, sharing an edge with the treatment-abundant Flavobacteriales, a common environmental bacteria taxon. This network suggests that the probiotic did not directly alter the overall bacterial community in the rearing water in an oyster hatchery, but targeted specific members of the community.
Discussion
A better understanding of bacterial community dynamics in aquaculture systems is critical for optimizing disease management strategies such as probiotic treatment. This study characterized: (a) changes in microbial communities in an oyster hatchery through the rearing process; and (b) the effect of probiotic treatment on those communities. To our knowledge, this is the first study to characterize the effects of probiotics on microbiomes in a bivalve hatchery. Despite the high spatial (by sample type and replicate tank) and temporal variability in bacterial composition at the hatchery detected in this research, results support the hypothesis that probiotic treatment leads to shifts in the microbial community in the hatchery from a state promoting the growth of potential pathogens to one that inhibits it.
Our results showed high variability in bacterial composition between replicate samples within trials and between trials, especially among the bacterial communities of oyster larvae. Variability between the 3 trials, conducted in July, January, and June in different years, is consistent with natural seasonal variation in microbial communities in Narragansett Bay (Staroscik and Smith, 2004). High variability in microbial communities in oysters from a single location is consistent with past studies, and is most probably driven by genetic and environmental effects on host-microbe interactions (King et al., 2012; Wegner et al., 2013). Moreover, variability between replicates (tanks within the hatchery) and between trials, may have been due to inevitable variance in husbandry and handling techniques at the hatchery (Elston et al., 1981, 2008).
Despite the high variability observed in these trials, our study observed clear differences in diversity and bacterial community structure between the rearing water, the biofilms on tank surfaces (swabs), and the oyster larvae. In particular, oyster larvae microbiomes were a subset of taxa present in the water and in biofilms, including Firmicutes and Proteobacteria, while tank biofilms showed a diversity and composition state that was intermediate between water and larvae. Lower diversity indices in the larvae and tank biofilms (swabs) than the water indicates niche selection of larval and biofilm colonizers, particularly Cyanobacteria in tank biofilms and Proteobacteria in oyster larvae. The dominance of Proteobacteria in the system, the most abundant phylum in all samples (up to 87% in larvae), is consistent with previous studies where it was shown to make up the largest and most diverse phylum in oyster-associated microbiota (Hernández-Zárate and Olmos-Soto, 2006; Trabal Fernández et al., 2014; Dittmann et al., 2018). Bacteria are an essential component of aquaculture nutrition, as a source of both nutrients and growth factors for the microalgae, and as food for the larvae (Kamiyama, 2004; Natrah et al., 2014; Nevejan et al., 2016). Factors such as size, nutrient availability, metabolites, and accompanying bacteria lead to differential ingestion of algae and associated microbes in eastern oysters (Newell and Jordan, 1983; Baldwin, 1995; Pales Espinosa et al., 2009; Nevejan et al., 2016). Interestingly, strong temporal changes were seen in the structure of microbial communities of oyster larvae, tank surface biofilms, and/or rearing water in each of the trials. Considering the short duration of the trials (less than 15 days), this indicates that temporal changes in bacterial communities in the tanks may be driven by developmental and health changes in the oyster larvae, since it is unlikely that these major changes are due to transient changes in the microbial composition of incoming water (as observed in Trial 3). More research is needed to evaluate the role of oyster-microbial interactions on the dynamics of microbial communities in rearing tanks in hatcheries.
There was no effect of probiotics on bacterial community diversity or structure in any of the sample types, suggesting that the primary probiotic effect of B. pumilus RI06-95 is exerted directly on larval health (e.g., by modulation of the immune system) and/or that it is mediated by subtle, targeted changes in the oyster microbiomes that are obscured by larger temporal effects and/or by homogenization of large pools of larvae from each tank. The presence of the probiotic was confirmed with higher relative abundance of Bacillales in the probiotic-treated water and increased relative abundance throughout the duration of each trial, suggesting that the probiotic accumulates in the larvae through time (tanks were scrubbed and water was changed every other day). Previous studies of the impact of probiotics on microbiota in humans and fish also showed subtle changes of certain taxa, but no consistent effect on the diversity of the host’s bacterial community (Boutin et al., 2013; Merrifield and Carnevali, 2014; Standen et al., 2015; Laursen et al., 2017; Schmidt et al., 2017). However, other studies report dramatic changes in fish intestinal microbiomes as a result of prebiotic treatment (Geraylou et al., 2013; Gonçalves and Gallardo-Escárate, 2017).
In addition to Bacillales, significant amplification of taxa was observed in probiotic-treated water samples compared to the control samples, most notably in the Oceanospirillales order. Oceanospirillales are heterotrophs commonly associated with mollusks and are found in the gills of many bivalves (Jensen et al., 2010; Zurel et al., 2011; Costa et al., 2012; Beinart et al., 2014). Additionally, they are recognized for their ability to degrade organic compounds in the environment and their abundance in oil plume microbial communities (Hazen et al., 2010; Dubinsky et al., 2013). These observations indicate that Oceanospirillales may confer a beneficial effect to the oyster host and contribute to the mechanism of oyster larval protection by the B. pumilus RI06-95 probiotic. Additionally, this suggests that the presence of B. pumilus RI06-95 has targeted effects on specific members of the microbial community in larval tanks in the hatchery.
Previous research showed that probiotic treatment with B. pumilus RI06-95 decreases levels of Vibrio spp. in the hatchery (Sohn et al., 2016a). This may be due to the production of antimicrobial secondary metabolites produced by B. pumilus RI06-95, as well as other Bacillus spp., that inhibit the growth of Vibrio spp. (Vaseeharan and Ramasamy, 2003; Karim et al., 2013; Sohn et al., 2016a). In the current study, a similar trend (as determined by a reduction in relative abundance, with overall trends confirmed using Vibrio spp. colony counts on selective media) was observed in treated tanks, but high variability and small sample sizes may have hindered detecting statistically significant differences. Moreover, failure to detect a significant decrease in Vibrio reads in Trial 2 (performed in January) was most probably due to the low abundance of Vibrio spp. in this trial, which is consistent with low levels of these species in coastal waters of the North Atlantic during winter (Staroscik and Smith, 2004). Interestingly, our research indicates that probiotic treatment leads to increased Vibrio diversity in rearing water through time. This increase in diversity in the absence of a net increase in relative abundance signifies a likely decrease in the relative abundance of specific pathogenic Vibrio spp., and therefore lower chances of a disease outbreak. Moreover, 16SrRNA oligotyping of the Vibrio species in the water samples revealed a transition in the Vibrio community in probiotic-treated tanks from a predominance of potentially pathogenic species [V. alginolyticus, a virulent pathogen originally isolated from amphioxus (Zou et al., 2016) and V. celticus, a virulent anaerobic clam pathogen (Beaz-Hidalgo et al., 2010b)] to a predominance of a likely non-pathogenic species [V. orientalis, a species that has been associated with adaptive functions (Tangl, 1983; Mukhta et al., 2016)]. This trend further confirms that addition of B. pumilus RI06-95 causes targeted changes in certain taxa, especially Vibrios, which is highly relevant for decreasing infective doses and, consequently, disease dynamics (Chauhan and Singh, 2018).
This interpretation is consistent with results from the co-occurrence network analysis, a tool used to identify associations, patterns, roles, and inform hypotheses from 16S abundance data (Barberán et al., 2012). This analysis suggests a negative association between Bacillales with Vibrionales in the trials performed in summer months (Trials 1 and 3), when Vibrionales are more abundant in the environment and oysters. Previous research and sequencing of the genome of B. pumilus RI06-95 show that potential mechanisms of probiotic action can include direct competition with other species and biofilm formation (Karim et al., 2013; Hamblin et al., 2015). Competition between B. pumilus RI06-95 and other bacteria (including Vibrionales) could open niches in the oyster microbiome for potentially beneficial microbes.
In summary, the bacterial community dynamics observed in this study indicate a variety of interactions between larval oysters and specific members of the microbiome, such as Vibrio spp. and the Bacillus probiotic. First, Vibrio spp., as well as other Proteobacteria, appear to be particularly capable of colonizing and surviving within oyster larvae (Romalde et al., 2014). These opportunistic Vibrios may be outcompeted by pre-colonization of other bacteria in the system, leading to a decrease in Vibrio abundance and/or an increase in diversity over time (Beaz-Hidalgo et al., 2010a; Zhao et al., 2016, 2018). We hypothesize that inhibition of Vibrio spp. by probiotic B. pumilus RI06-95 may allow for potentially beneficial Oceanospirillales to become more abundant in the system. Additional research is needed to examine the specific interactions between Oceanospirillales symbionts, the Bacillus probiotic, Vibrio pathogens, and the oyster host. Elucidating such interactions will require more targeted 16S rRNA and functional metagenomic analyses to track specific species over time, as well as functional studies using in vitro and in vivo competition experiments.
Conclusion
This study investigated the effects of time and probiotic treatment on bacterial communities in an oyster hatchery. Understanding how probiotic treatment affects microbiota in aquaculture systems may help in optimizing their benefits and preventing undesirable side-effects (Kesarcodi-Watson et al., 2008). Our results show that there is a strong effect of time on the microbiomes within oyster larvae, on tank walls and in the rearing water, and that probiotic treatment leads to subtle changes in certain bacterial taxa, including an increase in the relative abundance of Oceanospirillales in the rearing water and changes in the Vibrio community. These results inform how probiotics may influence bacterial communities in an oyster hatchery over temporal and spatial scales, leading to an overall improvement in larval health.
Data Availability
The raw sequences generated for this study can be found in the NCBI Short Read Archive under BioProject no. PRJNA518081. All processed 16S rRNA amplicon percent abundances at the Phylum and Order level and the R-markdown file to reproduce the figures in the manuscript can be found on Zenodo (https://doi.org/10.5281/zenodo.2658685). In addition, further public analysis and exploration of Trial 3 data are possible on the VAMPS website (https://vamps.mbl.edu/) using software under the project name AFP_RWU1_Bv6.
Author Contributions
RJS, SS, DRN, DCR, AFP, and MG-C contributed conception and design of the study. SS, THM, KT, RS, and KML contributed to performance of the hatchery trials. RJS and SS collected and prepared the samples for sequencing. RJS performed the sequence analysis and wrote the manuscript. All authors contributed to manuscript revision, read and approved the submitted version.
Funding
This work was supported by NSF Graduate Research Fellowship 1244657 to RJS, USDA AFRI 2016-67016-24905 and USDA NRAC 2258-Z55106 to DCR, DRN, and MG-C. This material is based upon work conducted at a Rhode Island NSF EPSCoR research facility, the Genomics and Sequencing Center, supported in part by the National Science Foundation EPSCoR Cooperative Agreements 0554548, EPS-1004057, and OIA-1655221.
Conflict of Interest Statement
The authors declare that the research was conducted in the absence of any commercial or financial relationships that could be construed as a potential conflict of interest.
Acknowledgments
We would like to thank the RWU Blount Shellfish Hatchery, the URI Genomics and Sequencing Center, and the MBL Keck Sequencing Center. We gratefully acknowledge several undergraduate students at URI and RWU for their assistance during this study.
Supplementary Material
The Supplementary Material for this article can be found online at: https://www.frontiersin.org/articles/10.3389/fmicb.2019.01060/full#supplementary-material
Footnotes
References
Andrews, S. (2010). FASTQC. A Quality Control Tool for High Throughput Sequence Data. Available at: http://www.bioinformatics.babraham.ac.uk/projects/fastqc/ (accessed September 15, 2014).
Asmani, K., Petton, B., Le Grand, J., Mounier, J., Robert, R., and Nicolas, J. L. (2016). Establishment of microbiota in larval culture of Pacific oyster, Crassostrea gigas. Aquaculture 464, 434–444. doi: 10.1016/j.aquaculture.2016.07.020
Baldwin, B. S. (1995). Selective particle ingestion by oyster larvae (Crassostrea virginica) feeding on natural seston and cultured algae. Mar. Biol. 123, 95–107. doi: 10.1007/BF00350328
Barberán, A., Bates, S. T., Casamayor, E. O., and Fierer, N. (2012). Using network analysis to explore co-occurrence patterns in soil microbial communities. ISME J. 6, 343–351. doi: 10.1038/ismej.2011.119
Beaz-Hidalgo, R., Balboa, S., Romalde, J. L., and Figueras, M. J. (2010a). Diversity and pathogenecity of vibrio species in cultured bivalve molluscs. Environ. Microbiol. Rep. 2, 34–43. doi: 10.1111/j.1758-2229.2010.00135.x
Beaz-Hidalgo, R., Diéguez, A. L., Cleenwerck, I., Balboa, S., Doce, A., de Vos, P., et al. (2010b). Vibrio celticus sp. nov., a new Vibrio species belonging to the Splendidus Clade with pathogenic potential for clams. Syst. Appl. Microbiol. 33, 311–315. doi: 10.1016/j.syapm.2010.06.007
Beinart, R. A., Nyholm, S. V., Dubilier, N., and Girguis, P. R. (2014). Intracellular Oceanospirillales inhabit the gills of the hydrothermal vent snail Alviniconcha with chemosynthetic, γ-Proteobacterial symbionts. Environ. Microbiol. Rep. 6, 656–664. doi: 10.1111/1758-2229.12183
Bolger, A. M., Lohse, M., and Usadel, B. (2014). Trimmomatic: a flexible trimmer for Illumina sequence data. Bioinformatics 30, 2114–2120. doi: 10.1093/bioinformatics/btu170
Boutin, S., Audet, C., and Derome, N. (2013). Probiotic treatment by indigenous bacteria decreases mortality without disturbing the natural microbiota of Salvelinus fontinalis. Can. J. Microbiol. 59, 662–670. doi: 10.1139/cjm-2013-0443
Burge, C. A., Closek, C. J., Friedman, C. S., Groner, M. L., Jenkins, C. M., Shore-Maggio, A., et al. (2016). The use of filter-feeders to manage disease in a changing world. Integr. Comp. Biol. 56, 573–587. doi: 10.1093/icb/icw048
Chauhan, A., and Singh, R. (2018). Probiotics in aquaculture: a promising emerging alternative approach. Symbiosis 77, 99–113. doi: 10.1007/s13199-018-0580-1
Chauhan, A., Wafula, D., Lewis, D. E., and Pathak, A. (2014). Metagenomic assessment of the eastern oyster-associated microbiota. Genome Announc. 2:e1083–14. doi: 10.1128/genomeA.01083-14
Costa, P. M., Carreira, S., Lobo, J., and Costa, M. H. (2012). Molecular detection of prokaryote and protozoan parasites in the commercial bivalve Ruditapes decussatus from southern Portugal. Aquaculture 370–371, 61–67. doi: 10.1016/j.aquaculture.2012.10.006
Dittmann, K., Sonnenschein, E. C., Egan, S., Gram, L., and Bentzon-Tilia, M. (2018). Impact of Phaeobacter inhibens on marine eukaryote associated microbial communities. Environ. Microbiol. Rep. [Epub ahead of print].
Dixon, P. (2003). VEGAN, a package of R functions for community ecology. J. Veg. Sci. 14, 927–930. doi: 10.1111/j.1654-1103.2003.tb02228.x
Dubert, J., Barja, J. L., and Romalde, J. L. (2017). New insights into pathogenic vibrios affecting bivalves in hatcheries: present and future prospects. Front. Microbiol. 8:762. doi: 10.3389/fmicb.2017.00762
Dubinsky, E. A., Conrad, M. E., Chakraborty, R., Bill, M., Borglin, S. E., Hollibaugh, J. T., et al. (2013). Succession of hydrocarbon-degrading bacteria in the aftermath of the deepwater horizon oil spill in the gulf of Mexico. Environ. Sci. Technol. 47, 10860–10867. doi: 10.1021/es401676y
Elston, R., Hasegawa, H., Humphrey, K., Polyak, I., and Häse, C. (2008). Re-emergence of Vibrio tubiashii in bivalve shellfish aquaculture: severity, environmental drivers, geographic extent and management. Dis. Aquat. Organ. 82, 119–134. doi: 10.3354/dao01982
Elston, R., Leibovitz, L., Relyea, D., and Zatila, J. (1981). Diagnosis of vibriosis in a commercial oyster hatchery epizootic: diagnostic tools and management features. Aquaculture 24, 53–62. doi: 10.1016/0044-8486(81)90043-0
Eren, A. M., Maignien, L., Sul, W. J., Murphy, L. G., Grim, S. L., Morrison, H. G., et al. (2013a). Oligotyping: differentiating between closely related microbial taxa using 16S rRNA gene data. Methods Ecol. Evol. 4, 1111–1119. doi: 10.1111/2041-210X.12114
Eren, A. M., Vineis, J. H., Morrison, H. G., and Sogin, M. L. (2013b). A filtering method to generate high quality short reads using illumina paired-end technology. PLoS One 8:e66643. doi: 10.1371/journal.pone.0066643
FAO (2018). The State of World Fisheries and Aquaculture 2018 - Meeting the Sustainable Development Goals. Available at: www.fao.org/publications (accessed November 14, 2018).
García Bernal, M., Trabal Fernández, N., Saucedo Lastra, P. E., Medina Marrero, R., and Mazón-Suástegui, J. M. (2017). Streptomyces effect on the bacterial microbiota associated to Crassostrea sikamea oyster. J. Appl. Microbiol. 122, 601–614. doi: 10.1111/jam.13382
Geraylou, Z., Souffreau, C., Rurangwa, E., De Meester, L., Courtin, C. M., Delcour, J. A., et al. (2013). Effects of dietary arabinoxylan-oligosaccharides (AXOS) and endogenous probiotics on the growth performance, non-specific immunity and gut microbiota of juvenile Siberian sturgeon (Acipenser baerii). Fish Shellfish Immunol. 35, 766–775. doi: 10.1016/j.fsi.2013.06.014
Gonçalves, A. T., and Gallardo-Escárate, C. (2017). Microbiome dynamic modulation through functional diets based on pre- and probiotics (mannan-oligosaccharides and Saccharomyces cerevisiae) in juvenile rainbow trout (Oncorhynchus mykiss). J. Appl. Microbiol. 122, 1333–1347. doi: 10.1111/jam.13437
Groner, M. L., Maynard, J., Breyta, R., Carnegie, R. B., Dobson, A., Friedman, C. S., et al. (2016). Managing marine disease emergencies in an era of rapid change. Philos. Trans. R. Soc. B Biol. Sci. 371:20150364. doi: 10.1098/rstb.2015.0364
Hamblin, M., Spinard, E., Gomez-Chiarri, M., Nelson, D. R., and Rowley, D. C. (2015). Draft genome sequence of the shellfish larval probiotic Bacillus pumilus RI06-95. Genome Announc. 3:e0858–15. doi: 10.1128/genomeA.00858-15
Hazen, T. C., Dubinsky, E. A., DeSantis, T. Z., Andersen, G. L., Piceno, Y. M., Singh, N., et al. (2010). Deep-sea oil plume enriches indigenous oil-degrading bacteria. Science 330, 204–208. doi: 10.1126/science.1195979
Helm, M., and Bourne, N. (2004). Hatchery Culture of Bivalves. A Practical Manual. Rom: Food and Agriculture Organization of the United Nations.
Hernández-Zárate, G., and Olmos-Soto, J. (2006). Identification of bacterial diversity in the oyster Crassostrea gigas by fluorescent in situ hybridization and polymerase chain reaction. J. Appl. Microbiol. 100, 664–672. doi: 10.1111/j.1365-2672.2005.02800.x
Huse, S. M., Mark Welch, D. B., Voorhis, A., Shipunova, A., Morrison, H. G., Eren, A. M., et al. (2014). VAMPS: a website for visualization and analysis of microbial population structures. BMC Bioinformatics 15:41. doi: 10.1186/1471-2105-15-41
Jensen, S., Duperron, S., Birkeland, N.-K., and Hovland, M. (2010). Intracellular oceanospirillales bacteria inhabit gills of Acesta bivalves. FEMS Microbiol. Ecol. 74, 523–533. doi: 10.1111/j.1574-6941.2010.00981.x
Kamiyama, T. (2004). The microbial loop in a eutrophic bay and its contribution to bivalve aquaculture. Fish. Res. 1, 41–50. doi: 10.3892/or.2013.2813
Karim, M., Zhao, W., Rowley, D., Nelson, D., and Gomez-Chiarri, M. (2013). Probiotic strains for shellfish aquaculture: protection of eastern oyster, crassostrea virginica, larvae and juveniles against bacterial challenge. J. Shellfish Res. 32, 401–408. doi: 10.2983/035.032.0220
Kesarcodi-Watson, A., Kaspar, H., Lategan, M. J., and Gibson, L. (2008). Probiotics in aquaculture: the need, principles and mechanisms of action and screening processes. Aquaculture 274, 1–14. doi: 10.1016/j.aquaculture.2007.11.019
Kesarcodi-Watson, A., Miner, P., Nicolas, J. L., and Robert, R. (2012). Protective effect of four potential probiotics against pathogen-challenge of the larvae of three bivalves: pacific oyster (Crassostrea gigas), flat oyster (Ostrea edulis) and scallop (Pecten maximus). Aquaculture 344–349, 29–34. doi: 10.1016/j.aquaculture.2012.02.029
King, G. M., Judd, C., Kuske, C. R., and Smith, C. (2012). Analysis of stomach and gut microbiomes of the eastern oyster (Crassostrea virginica) from Coastal Louisiana, USA. PLoS One 7:e51475. doi: 10.1371/journal.pone.0051475
King, W. L., Jenkins, C., Seymour, J. R., and Labbate, M. (2018). Oyster disease in a changing environment: decrypting the link between pathogen, microbiome and environment. Mar. Environ. Res. 143, 124–140. doi: 10.1016/J.MARENVRES.2018.11.007
Lafferty, K. D., Harvell, C. D., Conrad, J. M., Friedman, C. S., Kent, M. L., Kuris, A. M., et al. (2015). Infectious diseases affect marine fisheries and aquaculture economics. Ann. Rev. Mar. Sci. 7, 471–496. doi: 10.1146/annurev-marine-010814-015646
Laursen, M. F., Laursen, R. P., Larnkjær, A., Michaelsen, K. F., Bahl, M. I., and Licht, T. R. (2017). Administration of two probiotic strains during early childhood does not affect the endogenous gut microbiota composition despite probiotic proliferation. BMC Microbiol. 17:175. doi: 10.1186/s12866-017-1090-7
Le Roux, F., Wegner, K. M., and Polz, M. F. (2016). Oysters and vibrios as a model for disease dynamics in wild animals. Trends Microbiol. 24, 568–580. doi: 10.1016/j.tim.2016.03.006
Lokmer, A., Goedknegt, M. A., Thieltges, D. W., Fiorentino, D., Kuenzel, S., Baines, J. F., et al. (2016a). Spatial and temporal dynamics of pacific oyster hemolymph microbiota across multiple scales. Front. Microbiol. 7:1367. doi: 10.3389/fmicb.2016.01367
Lokmer, A., Kuenzel, S., Baines, J. F., and Wegner, K. M. (2016b). The role of tissue-specific microbiota in initial establishment success of Pacific oysters. Environ. Microbiol. 18, 970–987. doi: 10.1111/1462-2920.13163
Lokmer, A., and Wegner, K. M. (2015). Hemolymph microbiome of pacific oysters in response to temperature, temperature stress and infection. ISME J. 9, 670–682. doi: 10.1038/ismej.2014.160
Mcardle, B. H., and Anderson, M. J. (2010). Fitting multivariate models to community data?: a comment on distance-based redundancy analysis. Ecology 82, 290–297. doi: 10.1890/0012-9658
Mckindsey, C. W., Landry, T., Beirn, F. X. O., and Davies, I. A. N. M. (2007). Bivalve aquaculture and exotic species?: a review of ecological considerations and management issues. J. Shellfish Res. 26, 281–294. doi: 10.2983/0730-8000(2007)26[281:BAAESA]2.0.CO;2
McMurdie, P. J., and Holmes, S. (2013). Phyloseq: an R package for reproducible interactive analysis and graphics of microbiome census data. PLoS One 8:e61217. doi: 10.1371/journal.pone.0061217
Merrifield, D. L., and Carnevali, O. (2014). Probiotic modulation of the gut microbiota of fish. Aquac. Nutr. 588, 185–222. doi: 10.1002/9781118897263.ch8
Mukhta, S., Mehnaz, S., Mirza, M. S., Mirza, B., and Malik, K. A. (2016). Accession No. LT221239, Vibrio Orientalis Partial 16S rRNA gene, isolate LK2HaP4 - Nucleotide - NCBI. Available at: https://www.ncbi.nlm.nih.gov/nuccore/LT221239.1 (accessed December 19, 2017).
Natrah, F. M. I., Bossier, P., Sorgeloos, P., Yusoff, F. M., and Defoirdt, T. (2014). Significance of microalgal-bacterial interactions for aquaculture. Rev. Aquac. 6, 48–61. doi: 10.1111/raq.12024
Nevejan, N., De Schryver, P., Wille, M., Dierckens, K., Baruah, K., and Van Stappen, G. (2016). Bacteria as food in aquaculture: do they make a difference? Rev. Aquac. 10, 180–212. doi: 10.1111/raq.12155
Newaj-Fyzul, A., Al-Harbi, A. H., and Austin, B. (2014). Review: developments in the use of probiotics for disease control in aquaculture. Aquaculture 431, 1–11. doi: 10.1016/j.aquaculture.2013.08.026
Newell, R. I., and Jordan, S. J. (1983). Preferential ingestion of organic material by the American oyster Crassostrea virginica. Mar. Ecol. Prog. Ser. 13, 47–53. doi: 10.3354/meps013047
Pales Espinosa, E., Mickael, P., Evan Ward, J., Shumway, S. E., and Bassem, A. (2009). Lectins associated with the feeding organs of the oyster Crassostrea virginica can mediate particle selection. Biol. Bull. 217, 130–141. doi: 10.1086/bblv217n2p130
Pérez-Sánchez, T., Mora-Sánchez, B., and Balcázar, J. L. (2018). Biological approaches for disease control in aquaculture: advantages, limitations and challenges. Trends Microbiol. 26, 896–903. doi: 10.1016/j.tim.2018.05.002
Pierce, M. L., and Ward, J. E. (2018). Microbial ecology of the bivalvia, with an emphasis on the family ostreidae. J. Shellfish Res. 37, 793–806. doi: 10.2983/035.037.0410
Pierce, M. L., Ward, J. E., Holohan, B. A., Zhao, X., and Hicks, R. E. (2016). The influence of site and season on the gut and pallial fluid microbial communities of the eastern oyster, crassostrea virginica (Bivalvia, Ostreidae): community-level physiological profiling and genetic structure. Hydrobiologia 765, 97–113. doi: 10.1007/s10750-015-2405-z
Powell, S. M., Chapman, C. C., Bermudes, M., and Tamplin, M. L. (2013). Dynamics of seawater bacterial communities in a shellfish hatchery. Microb. Ecol. 66, 245–256. doi: 10.1007/s00248-013-0183-6
Prado, S., Romalde, J. L., and Barja, J. L. (2010). Review of probiotics for use in bivalve hatcheries. Vet. Microbiol. 145, 187–197. doi: 10.1016/j.vetmic.2010.08.021
Richards, G. P., Watson, M. A., Needleman, D. S., Church, K. M., and Häse, C. C. (2015). Mortalities of eastern and pacific oyster larvae caused by the pathogens Vibrio coralliilyticus and Vibrio tubiashii. Appl. Environ. Microbiol. 81, 292–297. doi: 10.1128/AEM.02930-14
Romalde, J. L., Diéguez, A. L., Lasa, A., and Balboa, S. (2014). New vibrio species associated to molluscan microbiota: a review. Front. Microbiol. 4:413. doi: 10.3389/fmicb.2013.00413
Schmidt, V., Gomez-Chiarri, M., Roy, C., Smith, K., and Amaral-Zettler, L. (2017). Subtle microbiome manipulation using probiotics reduces antibiotic-associated mortality in fish. mSystems 2:e133–17. doi: 10.1128/mSystems.00133-17
Shannon, P., Markiel, A., Ozier, O., Baliga, N. S., Wang, J. T., Ramage, D., et al. (2003). Cytoscape: a software environment for integrated models of biomolecular interaction networks. Genome Res. 13, 2498–2504. doi: 10.1101/gr.1239303
Sinigalliano, C. D., Gidley, M. L., Shibata, T., Whitman, D., Dixon, T. H., Laws, E., et al. (2007). Impacts of hurricanes Katrina and Rita on the microbial landscape of the New Orleans area. Proc. Natl. Acad. Sci. U.S.A. 104, 9029–9034. doi: 10.1073/pnas.0610552104
Sohn, S., Lundgren, K. M., Tammi, K., Karim, M., Smolowitz, R., Nelson, D. R., et al. (2016a). Probiotic strains for disease management in hatchery larviculture of the eastern oyster Crassostrea virginica. J. Shellfish Res. 35, 307–317. doi: 10.2983/035.035.0205
Sohn, S., Lundgren, K. M., Tammi, K., Smolowitz, R., Nelson, D. R., Rowley, D. C., et al. (2016b). Efficacy of probiotics in preventing vibriosis in the larviculture of different species of bivalve shellfish. J. Shellfish Res. 35, 319–328. doi: 10.2983/035.035.0206
Standen, B. T., Rodiles, A., Peggs, D. L., Davies, S. J., Santos, G. A., and Merrifield, D. L. (2015). Modulation of the intestinal microbiota and morphology of tilapia, Oreochromis niloticus, following the application of a multi-species probiotic. Appl. Microbiol. Biotechnol. 99, 8403–8417. doi: 10.1007/s00253-015-6702-2
Staroscik, A. M., and Smith, D. C. (2004). Seasonal patterns in bacterioplankton abundance and production in Narragansett Bay, Rhode Island, USA. Aquat. Microb. Ecol. 35, 275–282. doi: 10.3354/ame035275
Stentiford, G. D., Neil, D. M., Peeler, E. J., Shields, J. D., Small, H. J., Flegel, T. W., et al. (2012). Disease will limit future food supply from the global crustacean fishery and aquaculture sectors. J. Invertebr. Pathol. 110, 141–157. doi: 10.1016/j.jip.2012.03.013
Torondel, B., Ensink, J. H. J., Gundogdu, O., Ijaz, U. Z., Parkhill, J., Abdelahi, F., et al. (2016). Assessment of the influence of intrinsic environmental and geographical factors on the bacterial ecology of pit latrines. Microb. Biotechnol. 9, 209–223. doi: 10.1111/1751-7915.12334
Trabal Fernández, N., Mazón-Suástegui, J. M., Vázquez-Juárez, R., Ascencio-Valle, F., and Romero, J. (2014). Changes in the composition and diversity of the bacterial microbiota associated with oysters (Crassostrea corteziensis, Crassostrea gigas and Crassostrea sikamea) during commercial production. FEMS Microbiol. Ecol. 88, 69–83. doi: 10.1111/1574-6941.12270
Vaseeharan, B., and Ramasamy, P. (2003). Control of pathogenic Vibrio spp. by Bacillus subtilis BT23, a possible probiotic treatment for black tiger shrimp Penaeus monodon. Lett. Appl. Microbiol. 36, 83–87. doi: 10.1046/j.1472-765X.2003.01255.x
Verschuere, L., Rombaut, G., Sorgeloos, P., and Verstraete, W. (2000). Probiotic bacteria as biological control agents in aquaculture. Microbiol. Mol. Biol. Rev. 64, 655–671. doi: 10.1128/MMBR.64.4.655-671.2000
Warton, D. I., Wright, S. T., and Wang, Y. (2012). Distance-based multivariate analyses confound location and dispersion effects. Methods Ecol. Evol. 3, 89–101. doi: 10.1111/j.2041-210X.2011.00127.x
Wegner, K., Volkenborn, N., Peter, H., and Eiler, A. (2013). Disturbance induced decoupling between host genetics and composition of the associated microbiome. BMC Microbiol. 13:252. doi: 10.1186/1471-2180-13-252
Zhao, W., Dao, C., Karim, M., Gomez-Chiarri, M., Rowley, D., and Nelson, D. R. (2016). Contributions of tropodithietic acid and biofilm formation to the probiotic activity of Phaeobacter inhibens. BMC Microbiol. 16:1. doi: 10.1186/s12866-015-0617-z
Zhao, W., Yuan, T., Piva, C., Spinard, E. J., Schuttert, C., Rowley, D. C., et al. (2018). The probiotic bacterium, Phaeobacter inhibens, down-regulates virulence factor transcription in the shellfish pathogen, Vibrio coralliilyticus, by N -acyl homoserine lactone production. Appl. Environ. Microbiol. 85:e1545–18. doi: 10.1128/AEM.01545-18
Zou, Y., Ma, C., Zhang, Y., Du, Z., You, F., Tan, X., et al. (2016). Isolation and characterization of Vibrio alginolyticus from cultured amphioxus Branchiostoma belcheri tsingtauense. Biology 71, 757–762. doi: 10.1515/biolog-2016-0102
Keywords: microbiome, 16S rRNA sequencing analysis, oyster hatchery, probiotics, Vibrio, Crassostrea virginica, larvae
Citation: Stevick RJ, Sohn S, Modak TH, Nelson DR, Rowley DC, Tammi K, Smolowitz R, Markey Lundgren K, Post AF and Gómez-Chiarri M (2019) Bacterial Community Dynamics in an Oyster Hatchery in Response to Probiotic Treatment. Front. Microbiol. 10:1060. doi: 10.3389/fmicb.2019.01060
Received: 07 February 2019; Accepted: 26 April 2019;
Published: 15 May 2019.
Edited by:
Jean-Christophe Avarre, Institut de Recherche pour le Développement (IRD), FranceReviewed by:
Jesus L. Romalde, University of Santiago de Compostela, SpainSophie Gaudriault, Institut National de la Recherche Agronomique, France
Copyright © 2019 Stevick, Sohn, Modak, Nelson, Rowley, Tammi, Smolowitz, Markey Lundgren, Post and Gómez-Chiarri. This is an open-access article distributed under the terms of the Creative Commons Attribution License (CC BY). The use, distribution or reproduction in other forums is permitted, provided the original author(s) and the copyright owner(s) are credited and that the original publication in this journal is cited, in accordance with accepted academic practice. No use, distribution or reproduction is permitted which does not comply with these terms.
*Correspondence: Marta Gomez-Chiarri, Z29tZXpjaGlAdXJpLmVkdQ==