- 1STLO, Agrocampus Ouest, Institut National de la Recherche Agronomique, Paris, France
- 2Bioprox, Levallois-Perret, France
- 3Science de la Vie et de la Terre, Université de Rennes 1, Rennes, France
- 4Pôle Agronomique Ouest, Bba, Rennes, France
This review deals with beneficial bacteria, with a focus on lactobacilli, propionibacteria, and bifidobacteria. As being recognized as beneficial bacteria, they are consumed as probiotics in various food products. Some may also be used as starters in food fermentation. In either case, these bacteria may be exposed to various environmental stresses during industrial production steps, including drying and storage, and during the digestion process. In accordance with their adaptation to harsh environmental conditions, they possess adaptation mechanisms, which can be induced by pretreatments. Adaptive mechanisms include accumulation of compatible solutes and of energy storage compounds, which can be largely modulated by the culture conditions. They also include the regulation of energy production pathways, as well as the modulation of the cell envelop, i.e., membrane, cell wall, surface layers, and exopolysaccharides. They finally lead to the overexpression of molecular chaperones and of stress-responsive proteases. Triggering these adaptive mechanisms can improve the resistance of beneficial bacteria toward technological and digestive stresses. This opens new perspectives for the improvement of industrial processes efficiency with regard to the survival of beneficial bacteria. However, this bibliographical survey evidenced that adaptive responses are strain-dependent, so that growth and adaptation should be optimized case-by-case.
Introduction
Bacteria may constitute useful fermentation starters, healing probiotics, or both for the so-called “2-in-1” bacteria. These beneficial bacteria, within fermented foods as starter or within functional foods supplements as probiotic are ingested in high amount and it is a means to modulate the activity of the human gut microbiota (Collins and Gibson, 1999; Parvez et al., 2006; Moens et al., 2017). The ingested bacteria are essential for normal development of the immune system (Marco et al., 2017). Indeed, gut microbiota dysbiosis is increasingly correlated to various diseases, such as inflammatory bowel diseases and obesity, which are on the rise and constitute a public health problem linked to the Western diet (David et al., 2014; Plé et al., 2016). In order to decrease the consequences of such health problems, probiotics and fermented foods could be part of the solution. Indeed, the size of the probiotic market exceeded US$42 billion in 2016 and is expected to exceed US$64 billion in 2022 (Marketsandmarkets.com, 2017). Probiotics are also increasingly incorporated into non-fermented functional foods such as infant formula, ice creams, and cereal bars (Homayouni et al., 2008; Braegger et al., 2011; Bampi et al., 2016). These products constitute a growing market as well. The starter culture market, worth US$1.0 billion by 2018, is projected to grow at a CAGR of 5.6% (Marketsandmarkets.com, 2014).
Starters and probiotics are usually dried to produce easy-to-use ingredients that are stable and flexible for different applications like food, feed, and pharmaceutical products. At the industrial scale, two types of drying processes are implemented: freeze-drying and spray-drying. In addition, drying is a way to keep bacteria alive on the long term at ambient temperature and to facilitate their storage and transport. At the industrial level, the use of frozen starter culture has the disadvantage of high energy costs during transportation and storage. Therefore, frozen starters go hand-in-hand with high operating costs (Santivarangkna et al., 2007). During powder production, storage, and digestion, bacteria of interest may encounter multiple stresses, which may affect its survival and its beneficial effects. Indeed, they must survive during powder production and storage in a first time, and in a second time, during fermentation (starter) and digestion (probiotics) (Picot and Lacroix, 2004). A stress is defined as “any change in the genome, proteome or environment that imposes either reduced growth or survival potential. Such changes lead to attempts by a cell to restore a pattern of metabolism that either fits it for survival or faster growth” (Booth, 2002). Different pretreatments can induce an enhanced tolerance to various stresses, which may occur during bacterial powders production and consumption. In this paper, we reviewed stresses encountered during drying processes, storage, and digestion. Then, we summarized the main molecular adaptation mechanisms induced by different pretreatments described in propionibacteria, lactobacilli, and bifidobacteria, recognized as beneficial bacteria. We then describe the impact of the adaptation mechanisms induced by pretreatments on tolerance toward technological and digestive stresses. These data should contribute to making an informed choice of the best treatments for reinforcing bacteria during drying, storage, and digestion.
Beneficial Effects of Propionibacteria, Bifidobacteria and Lactobacilli
General Features of Propionibacteria, Bifidobacteria, and Lactobacilli
This review is focused in propionibacteria, lactobacilli, and bifidobacteria, which are generally recognized as safe (GRAS) (Avalljaaskelainen and Palva, 2005; Picard et al., 2005; Cousin et al., 2010). They constitute, to the best of our knowledge, the main bacterial genera considered as starter or/ and as probiotics.
Propionibacteria and bifidobacteria both belong to the Actinobacteria class, which comprises non-sporulating Gram-positive bacteria with a high G+C content (Cousin et al., 2010). Propionibacteria are currently described as non-motile pleomorphic rods. Their optimal growth temperature is 30°C with a pH of 7.0. They are anaerobic aerotolerant, and many of them are catalase-positive. Propionibacteria can use a wide range of carbon sources such as organic acids (lactate), carbohydrates (lactose, glucose, galactose, and fructose), and alcohol (glycerol) (Cousin et al., 2010). They are hetero-fermentative bacteria and their favorite substrate is lactate. For a consumption of 3 moles of lactate, propionibacteria produce 1 mole of acetate, 2 moles of propionate, and 1 mole of carbon dioxide, according to the Fitz equation (Gagnaire et al., 2015). Bifidobacteria require cysteine in their growth medium, consume carbohydrates, and produce lactate and acetate at molar ratio of 2:3, respectively, thus decreasing the pH of the culture medium. Their optimal growth temperature is 37°C (Hsu et al., 2007). Bifidobacteria are bifid or multiple-branched rods, and are strictly anaerobic and catalase-negative. They are naturally present in the gastrointestinal tract and vagina of animals (Schell et al., 2002; Savijoki et al., 2005; De Dea Lindner et al., 2007).
Lactobacilli are Gram-positive, firmicute bacteria with a low G+C content. Their natural habitat includes the digestive or reproductive tract of animals, raw milk, decomposing plants, and fermented products. They are anaerobic, non-sporuling, and facultative heterofermentative. Lactobacilli require rich growth media that contains carbohydrates, amino acids, peptides, fatty acid esters, salts, nucleic acid derivatives, and vitamins (Chervaux et al., 2000; Elli et al., 2000). Lactobacilli optimal growth temperature is generally between 30 and 40°C (Hammes and Hertel, 2015). Like bifidobacteria, lactobacilli use carbohydrates (as carbon and energy source) and produce lactic acid, therefore inducing a significant decrease in the pH of the medium. Among lactic acid bacteria, members of the genera Streptococcus, Lactococcus, Leuconostoc, and Pediococcus are also widely used as starters. The majority of available literature on probiotic effects and/or studies deals with lactobacilli, the largest genus within the group of lactic acid bacteria. In addition to lactobacilli, this review also deals with propionibacteria and bifidobacteria, used as probiotics and/or as starters.
Potential of Propionibacteria, Bifidobacteria, and Lactobacilli as Probiotics
Probiotics are defined as “live microorganisms that, when administered in adequate amounts, confer a health benefit on the host” (FAO/WHO, 2001). Potential probiotics can be isolated from many sources (Mills et al., 2011). A minimum of 107 live probiotic bacterial cells per gram or milliliter of product at the time of consumption is recommended by the International Dairy Federation (IDF) (Huang et al., 2017). Since these bacteria are included in probiotic preparations, the amount of live bacteria in these latter (tablets or capsules) has to be optimized (Gagnaire et al., 2015; Huang et al., 2017), as does their viability during digestion (Kailasapathy and Chin, 2000; Rabah et al., 2018). Probiotic bacteria can enhance or preserve health, in strain-dependent manner among probiotic species (Lebeer et al., 2008; Le Maréchal et al., 2015; Plé et al., 2016).
As mentioned previously, the consumption of probiotics modulates the gut microbiota, which is a pivotal effect. Indeed, the presence of bifidobacteria in the intestine is very important, especially during the first years of life (De Dea Lindner et al., 2007), when they represent a majority of the intestinal microbiota. This part decreases with time over a lifetime (Sánchez et al., 2013).
The probiotic effects include also immunomodulation, described in propionibacteria, bifidobacteria, and lactobacilli, which prevent and help to treat various immunes diseases as inflammatory bowel diseases or allergy (Picard et al., 2005; Steed et al., 2010; DuPont et al., 2014; Rong et al., 2015; Saez-Lara et al., 2015). Indeed, this early colonization of the gut by bifidobacteria and propionibacteria seems to prevent necrotizing enterocolitis (Colliou et al., 2017; Young et al., 2017). In addition, the manipulation of the gut microbiota with probiotics has been considered as a possible manner to prevent and treat obesity and cancers (Saez-Lara et al., 2015; Dasari et al., 2017; Rabah et al., 2017; Brusaferro et al., 2018). Propionibacterium freudenreichii inhibits pathogens such as Salmonella Heidelberg (Nair FIM 2018) and meticillin-resistant Staphylococcus aureus (Sikorska and Smoragiewicz, 2013). Lactobacillus amylovorus inhibits Escherichia coli adhesion to intestinal cells (Hynönen et al., 2014) while Lactobacillus plantarum inhibits E. coli 0157:H7 adhesion to collagen (Yadav et al., 2013). Selected strains of bifidobacteria were reported to inhibit growth and toxicity of Clostridium difficile (Valdés-Varela et al., 2016), or to affect the virulence of Listeria monocytogenes in vitro (Rios-Covian et al., 2018). They may protect against gastrointestinal disorders (Sanchez et al., 2007) and prevent diarrhea through their effects on the immune system and/or through enhanced resistance to colonization by pathogens, as C. difficile (Cantero et al., 2018). Therefore, alleviation of lactose intolerance symptoms is demonstrated by lactobacilli, which provide the missing enzyme for lactose-intolerant people and, therefore, are complementary to a host that is deficient in β-galactosidase (Levri et al., 2005; Pakdaman et al., 2015). The preclinical studies are promising and data highlight the strain-dependent aspect of these effects among propionibacteria, bifidobacteria, and lactobacilli species. However, more investigations are needed to assess the probiotic effectiveness at clinical level and to determine the precise molecular mechanisms involved (Khalesi et al., 2018).
The molecular mechanisms identified in probiotics effects seem to be similar among propionibacteria, lactobacillia, and bifidobacteria species. They include the production of secreted metabolites as short fatty acids (Lan et al., 2008; LeBlanc et al., 2017; Rabah et al., 2017), and the presence of key surface components (Konstantinov et al., 2008; Foligné et al., 2013; Sengupta et al., 2013; Taverniti et al., 2013; Le Maréchal et al., 2015; Lightfoot et al., 2015; Sarkar and Mandal, 2016; Louis and Flint, 2017; do Carmo et al., 2018). In the dairy propionibacterium P. freudenreichii, the immunomodulatory properties are linked to the ability of selected strains to induce the release of the regulatory IL-10 by immune cells (Foligne et al., 2010). This property is mediated by surface proteins of the S-layer family (Le Maréchal et al., 2015; Deutsch et al., 2017). Accordingly, propionibacteria belonging to the human gut microbiota protect new-borns from necrotizing enterocolitis via Th17 cell regulation (Colliou et al., 2017). This property is also dependent on proteins of the surface layer and induces the generation of bacteria-specific Th17 cells, while maintaining IL-10+ regulatory T cells (Ge et al., 2019). In Lactobacillus acidophilus, immunomodulatory properties are dependent on the surface-layer protein SlpA, it occurs via binding to DC-SIGN receptors on dendritic cells and inducing a concentration-dependent production of IL-10 (Konstantinov et al., 2008). This binding plays a pivotal role in L. acidophilus ability to mitigate induced colitis (Lightfoot et al., 2015). By contrast, in the probiotic Bifidobacterium bifidum, such a protective ability, as well as the immunomodulatory properties, are linked to the presence of cell surface polysaccharides. These last also act via regulatory dendritic cells, but through a partially Toll-like receptor 2-mediated mechanism, inducing the generation of Foxp3+ regulatory T cells (Verma et al., 2018). The strain-dependent nature of the probiotics beneficial effects is closely correlated to the strain-dependent nature of the ability of probiotics to express or produce these probiotics effectors.
Potential of Propionibacteria, Bifidobacteria, and Lactobacilli as Starters
Fermented foods have been produced and consumed worldwide for centuries (Ebner et al., 2014; Marco et al., 2017). Fermented products can be produced from dairy, meat, or plant matrices and then used to produce a large diversity of fermented foods. As a result, there is a multitude of food matrix-starter combinations. Some fermentations can be spontaneous, but many products require inoculation by starters (Rong et al., 2015; Walsh et al., 2016; Marco et al., 2017). To produce fermented foods, a large number of bacteria may be used. This number was shown to be stable over time and between countries (Fortina et al., 1998; De Dea Lindner et al., 2007), although the diversity of fermented foods tends to decrease because of industrialization (Marco et al., 2017).
Lactobacilli are extensively used as starters in the fermentation of dairy products, e.g., L. acidophilus in cheese, Lactobacillus delbrueckii in yogurt, and Lactobacillus kefiranofaciens in kefir. L. acidophilus is also used in fermented plant products such as kimchi (Meira et al., 2015; Marco et al., 2017; Wang et al., 2018), that also provides other lactobacilli such as Lactobacillus sakei (Kwon et al., 2018), L. plantarum (Lim et al., 2018), and Lactobacillus fermentum (Yoo et al., 2017). Propionibacteria are widely used as ripening starters in the manufacture of Swiss-type cheeses (Thierry et al., 2011) and also contribute to the fermentation of vegetable products (Yu et al., 2015). Although not really considered as fermentation starters, bifidobacteria can be added before the fermentation process, with an impact on the final organoleptic properties of the product (Kailasapathy, 2006).
During fermentation, bacteria modify the matrices and contribute to the final flavor, texture, nutrition, and organoleptic qualities (Marco et al., 2017). They offer an increased availability of bioactive molecules, vitamins, and other constituents due to the process of fermentation. Thus, fermentation leads to increased digestibility of dairy products and plant matrices (Marco et al., 2017; Moens et al., 2017). Fermented dairy products have a low lactose content. Indeed, this carbohydrate is digested by the starter (Guarner et al., 2005), like other oligosaccharides (Sánchez et al., 2013; Moens et al., 2017). Furthermore, starters improve food storage and preservation, and may thus make it possible to decrease the use of additives (Mende et al., 2016).
Traditional fermented foods products can have a probiotic effect per se (Levri et al., 2005). Combining selected strains of probiotic bacteria with two-in-one abilities (both efficient starters and probiotics) leads to new functional fermented foods (Dimitrellou et al., 2016). Probiotics can be added to foods like in the case of bifidobacteria in yogurt (Picot and Lacroix, 2004), but these type of processes require adequate technologies to keep bacteria alive (Racioppo et al., 2017). The use of probiotics in food is growing, but mechanisms used by bacteria to exert health benefits are not fully elucidated (Savijoki et al., 2005). The main troubles targeted are antibiotic-associated diarrhea, traveler’s diarrhea, pediatric diarrhea, inflammatory bowel disease, and irritable bowel disease. Although there is a limited number of clinical studies with fermented foods (Marco et al., 2017), preclinical studies show promising positive effects (Chen et al., 2014; Gao et al., 2015; Kato-Kataoka et al., 2016; Plé et al., 2016). All the above-described probiotic effects should be considered as potential effects. Indeed, the European Food Safety Authority (EFSA) requires substantial clinical proof before allowing a functional claim.
For all probiotics and starters, survival during industrial production processes, storage step, and supply chain is a prerequisite. For in situ probiotic efficacy, effects that rely on the local production of beneficial metabolites such as short-chain fatty acids or vitamins require live bacteria capable of surviving digestive tract constraints. In contrast, for effects that rely on cellular fractions such as cell wall immunomodulating compounds, viability may be less crucial. Anyway, the probiotic bacterium should adapt industrial constraints to keep alive and then used.
Stress Encountered During Industrial Production and Digestion Processes
Industrial Drying Process and Storage
Freeze-Drying Process
Frozen starter culture is benchmark, as a high cooling rate and very low temperature (-80°C) permit to increase bacteria viability. However, the use of frozen starter culture at the industrial level has the disadvantage of requiring negative temperatures during transportation and storage. Therefore, frozen starters go hand-in-hand with high operating costs (Santivarangkna et al., 2007). Freeze-drying is the most conventional process, i.e., the most frequently used with regard to its efficiency. The drying process of bacteria is conducted by sublimation, with the advantage of providing high bacterial viability (Santivarangkna et al., 2007). However, this process is discontinuous and expensive. Moreover, the ice crystals produced during freezing in the intracellular and extracellular compartments may be responsible for cell damage: compromised cellular integrity, broken DNA strands, altered transcription and replication, and reduced membrane fluidity. Freeze-drying favors also the appearance of holes in the cell membrane, which may cause cell death if another stress occurs (Carvalho et al., 2004; Giulio et al., 2005). During the freezing step, the cooling rate is an important factor for maintaining bacterial viability. In addition, bacteria suffer from osmotic stress (decrease in surrounding water activity) during freeze-drying, that represents a common constraint encountered during the drying of bacteria. The composition of drying media is highly studied in the aim of improving bacterial viability during the process. As an example, skim milk can be used as a drying matrix since it has the advantage of stabilizing the cell membrane constituents and contains proteins that build a protective coating for the bacteria (Carvalho et al., 2004). Protective agents can be added to the drying matrix in order to improve bacterial viability during storage. These molecules need to have an amino group, a secondary alcohol group, or both (Carvalho et al., 2004). Suitable protective agents should provide cryoprotection for the bacteria during freezing, be easily dried, and improve drying matrix stability and rehydration (Zhao and Zhang, 2005). Several sugars are used to protect bacteria, e.g., glucose, fructose, lactose, and trehalose. Sugar alcohols like sorbitol and inositol can also be used. Monosodium glutamate can stabilize protein structure. Antioxidants such as ascorbate can be also added in order to protect membrane lipids against damage caused by the freeze-drying process (Carvalho et al., 2004; Kurtmann et al., 2009). The efficiency of these different agents to limit freeze-drying stresses is bacteria dependent (Zhao and Zhang, 2005).
Spray-Drying Process
Spray-drying decreases the cost of water removal by a factor of six, in comparison to freeze-drying (Paéz et al., 2012), while producing powder with a yield four to seven times higher than that of freeze-drying (Golowczyc et al., 2011; Huang et al., 2016a). Spray-drying has the advantage of being a continuous process, drying and encapsulating bacteria occurred in the same time (Peighambardoust et al., 2011). This process constitutes thus an emerging alternative to freeze-drying (Huang et al., 2016b).
Spray-drying can be divided into two steps: an initial constant rate evaporation stage, which is at the wet-bulb temperature, and the falling rate evaporation stage, which leads to product temperature increases toward the outlet air temperature at the end of drying (Huang et al., 2017). The time–temperature combination is an important factor that determines the extent of cell death, in particular depending on the outlet temperature value (Santivarangkna et al., 2007). The spray-drying process usually leads to high temperatures over a short time. Heat stress is a commonly encountered stress, affecting bacterial viability during spray-drying. Cell death is due to the destruction of more than one critical component (Peighambardoust et al., 2011), including major damage to membrane lipids and/or aggregation of membrane protein (Mills et al., 2011). Indeed, the membrane bilayer structure is thermodynamically unstable (Peighambardoust et al., 2011) so that damage caused to the membrane is the first cause of viability losses (Simpson et al., 2005). Other damage may occur inside the cell during drying. It affects DNA (Simpson et al., 2005), intracellular proteins, and ribosomes (Mills et al., 2011). RNAs may also be impaired as a result of the escape of Mg2+ (Huang et al., 2017). Indeed, the use of lower temperatures during spray-drying is possible to enhance viability, but the high water activity (aw) obtained in the powder limits its stability during storage. Moreover, bacteria suffer from oxidative and osmotic stresses that are coupled to heat stress during spray-drying (Huang et al., 2017), first, from the drying air and, second, because of the concomitant increase in osmotic pressure with the loss of water (Desmond et al., 2001).
Survival rate during spray-drying is species and strain-dependent, which is related to species- and strain-dependent heat tolerance ability (Peighambardoust et al., 2011). P. freudenreichii has a better chance to survive than Lactobacillus casei (Huang et al., 2016a), and thermotolerant S. thermophilus survives better than lactobacilli (L. delbrueckii ssp bulgaricus and L. acidophilus) (Huang et al., 2017). One of the most important factors for spray-drying survival is the intrinsic resistance to heat that is species- and strain-dependent and determine the survival of the bacteria during the process. The difference in heat resistance is more important when the heat challenge increases (Paéz et al., 2012). The thermic intrinsic sensitivity of a strain is essential to determine survival (Simpson et al., 2005) and can be optimized to enhance viability (Santivarangkna et al., 2007). In contrast, the oxygen tolerance of bifidobacteria is not correlated with a good viability during drying (Simpson et al., 2005).
Statistical models were developed to predict survival during spray-drying based on the hypothesis that bacterial death during spray-drying followed a probability distribution (Perdana et al., 2014). However, this prediction was not very precise because bacteria can be pre-adapted to technological stress, and because the drying medium may play a protective role. Viability can be improved by adding either trehalose, which stabilizes membranes and proteins and decreases membrane phase transition temperatures, or gelatin, gum Arabic, or fruit juice (Huang et al., 2017). It is thus possible to modulate the drying media composition to enhance bacterial survival (Sollohub and Cal, 2010). However, adding protective agents to the drying medium will not necessarily lead to optimal bacteria survival during storage (Santivarangkna et al., 2007).
Storage
During storage, bacterial viability generally remains higher for freeze-dried powders compared to spray-dried powders (Santivarangkna et al., 2007), which may be due to heat stress (Huang et al., 2017). Viability indeed decreases during storage, particularly at ambient temperature (Simpson et al., 2005). During storage, the water activity of the dry product, the glass transition temperature, the environmental relative humidity, temperature, and light are important factors for maintaining cell viability (Santivarangkna et al., 2007; Huang et al., 2017). For freeze-dried powders, stability is greater at low temperatures and in oxygen-free environments (Santivarangkna et al., 2007). Viability is inversely correlated to temperature (Paéz et al., 2012), but industry generally looks for stable dry cultures at ambient temperature for marketing purposes. The presence of oxygen decreases viability because of lipid oxidation. Some authors therefore attempted to add antioxidants to decrease the impact of oxidative stress, but the results were not convincing for long-term storage (Santivarangkna et al., 2007).
Digestion
Some probiotic effects require live bacteria in sufficient amounts in the large intestine. Probiotic bacteria have to pass the stomach, which seems to be the most difficult part of the gastrointestinal tract to cross during digestion. Bacteria have to survive the acid conditions and the presence of bile salts (Mainville et al., 2005). The harsh conditions of the gastrointestinal tract also include variations in the redox potential, and the presence of hydrolytic enzymes such as lysozyme, of various proteases and lytic compounds found in pancreatin, and of bile salts. Acid stress is a major limit to fermentation by beneficial bacteria. A decrease in the pH is generally the reason why fermentation stops. Probiotics such as bifidobacteria are also added to acidic yogurt-type products and then stored at 4°C before consumption. Finally, all the beneficial bacteria face severe acid stress during the first step of digestion (i.e., stomach), which limits the in situ activity. Viability in the intestinal compartment is specie and strain-dependent (Picot and Lacroix, 2004; Alcantara and Zuniga, 2012). For example, L. casei and other lactobacilli have low pH tolerance (Broadbent et al., 2010), although it is still higher than that of some bifidobacteria (except for Bifidobacterium infantis). The capacity to resist bile salts is strain-dependent, and probiotic strains can thus be selected for their tolerance to bile salts. Bile salts act as detergents (Alcantara and Zuniga, 2012; Papadimitriou et al., 2016) and cause cell damage and cytotoxicity (Arnold et al., 2018). Molecular mechanisms leading to bile adaptation were evidenced in dairy propionibacteria (Leverrier AEM2003, arch 2004), in lactobacilli (Ruiz FIM 2013, Goh MolCelFact 2014, Vinusha 2018), and in probiotic bifidobacteria (Ruiz FIM 2013, Sanchez AEM 2007).
Lactobacilli and bifidobacteria have bile salts hydrolase (bsh) genes, which can have a positive or negative impact on bile salts tolerance, so the selection requires a careful examination of microbial physiology (Arnold et al., 2018). Since osmolarity is constantly changing in the gastrointestinal tract, bacteria survival may be affected byf osmotic stress (De Dea Lindner et al., 2007).
Mechanisms of Stress Adaptation Triggered by Pretreatements
Beneficial bacteria used as technological starters will have to face technological stresses such as heat, cold, oxidative, and osmotic stresses. Probiotic bacteria should also survive digestive stress, in addition to the above-mentioned stresses. Digestive stresses include acid, bile salts, and osmotic constraints. The main adaptive mechanisms triggered by bacteria to deal with such damage are DNA repair, metabolic pathways of lipid modification, chaperones and proteases, accumulation of compatible solutes, and reactive oxygen specices (ROS) detoxification (Desmond et al., 2001; Sheehan et al., 2006; Li et al., 2009). The medium culture choice is crucial since its composition may induce different adaptation mechanisms, as detailed below. General adaptive mechanisms induced by different pretreatments of propionibacteria, bifidobacteria, and lactobacilli are presented in Figure 1. Adaptive mechanisms are induced by specific pretreatments reported in Table 1.
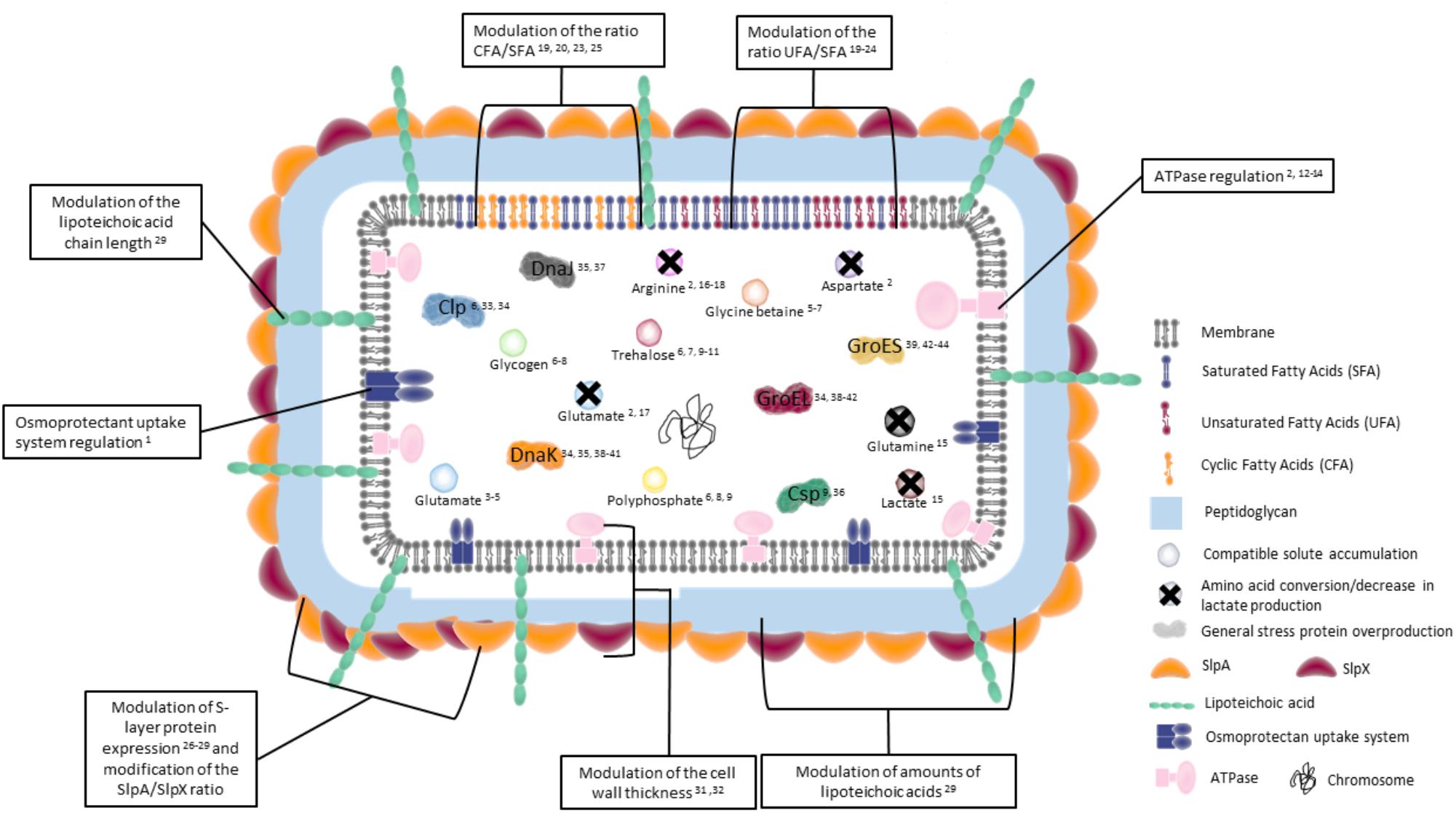
Figure 1. Key actors of adaptive mechanisms in bacteria during osmotic, acid, oxidative, heat, cold, and bile salts adaptation. General adaptive bacterial mechanisms during osmotic, acid, oxidative, heat, cold, and bile salts treatment are represented. Peptidoglycan is represented in blue. Membrane lipids under normal growth are represented in gray. Amounts of saturated (blue), unsaturated (red), and cyclic (yellow) fatty acids are modulated by treatments. S-layer proteins, which may be involved in adaptation, are represented in yellow and red outside the peptidoglycan. Liptechoic acids, whose length is modulated, are presented in green. Inducible transmembrane ATPase and osmoprotectant uptake systems are represented in pink and blue, respectively. In the cytoplasm, general stress proteins are represented by different colors. Colored circles represent different osmoprotectant and energy storage compounds. Crosses on circles mean the conversion of the molecule. The chromosome is represented in black. The numbers indicate corresponding references in the tables.
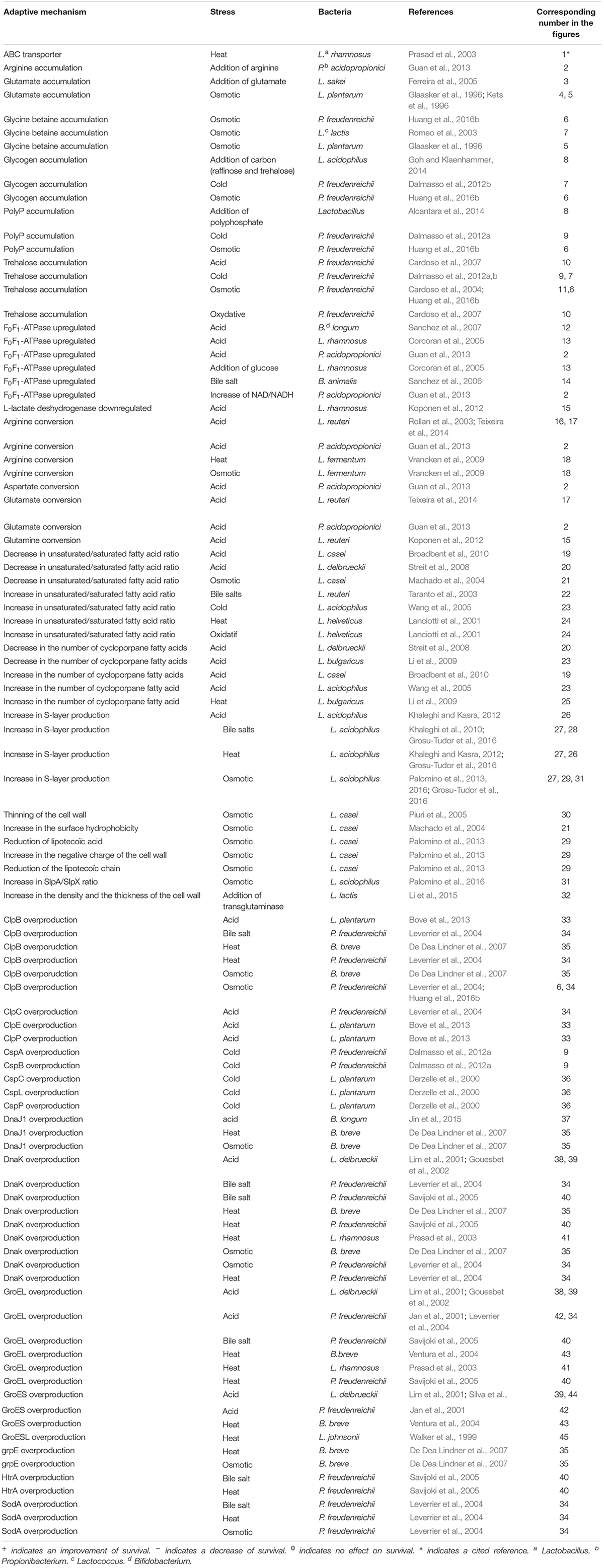
Table 1. Adaptive mechanisms induced by stressing conditions or by modifications of the growth medium in bifidobacteria, propionibacteria, and lactobacilli.
Accumulation of Compatible Solute and Energy Storage
A compatible solute is a small organic molecule that is polar, highly soluble in water, and that has a neutral isoelectric point. It behaves like an osmolyte, allowing a live cell to adapt to an osmotic stress (Csonka, 1989). During osmotic stress, bacteria accumulate compatible solutes (Csonka, 1989), either transported from the external medium, or synthesized de novo, to restore turgescent pressure and enable cell growth and division (Csonka and Hanson, 1991). Compatible solutes are unable to rapidly cross bacterial membranes without the involvement of a transport system and, for the most part, do not carry an electrical charge at a neutral pH. Uncharged molecules can be accumulated at a high concentration without disturbing the metabolism (Csonka, 1989). There are a limited number of molecules considered to be compatible solutes, and they can be divided into two categories: the first one corresponds to sugars and polyols and the second is composed of alpha and beta amino acids and their derivatives (Roesser and Müller, 2001). Compatible solutes preserve the conformation of proteins submitted to osmotic constraint. Generally, compatible solutes are excluded from the immediate vicinity of the proteins by an unfavorable interaction between the protein surface and the compatible solutes. This mechanism is known as “preferential excluding” (Roesser and Müller, 2001).
Accumulation of Sugar and Polyol
Accumulation of trehalose
The versatile role of trehalose accumulation in stresses adaptation is schematized in Figure 2. Indeed, trehalose is a stable molecule (Giulio et al., 2005) which prevents protein aggregation, facilitates refolding, and protects cells and cellular proteins from damages caused by oxygen (Cardoso et al., 2004). It is also suggested that trehalose plays a role in the stabilization of cell membranes (Cardoso et al., 2007). Trehalose can act as an intracellular carbon stock and is consumed after exhaustion of the external carbon source (Cardoso et al., 2004). High sugar concentration in the culture medium promotes trehalose accumulation. Osmotic stress triggers accumulation of trehalose in P. freudenreichii and L. casei (Cardoso et al., 2004; Huang et al., 2016b). The accumulation of compatible solutes is facilitated in a rich medium as opposed to a chemically defined mediaTrehalose accumulation by P. freudenreichii may also be induced by other stresses such as cold (Dalmasso et al., 2012b), oxidative, and acid stresses (Cardoso et al., 2004, 2007). Moreover, trehalose can decrease the loss of viability during storage after freeze-drying (Giulio et al., 2005).
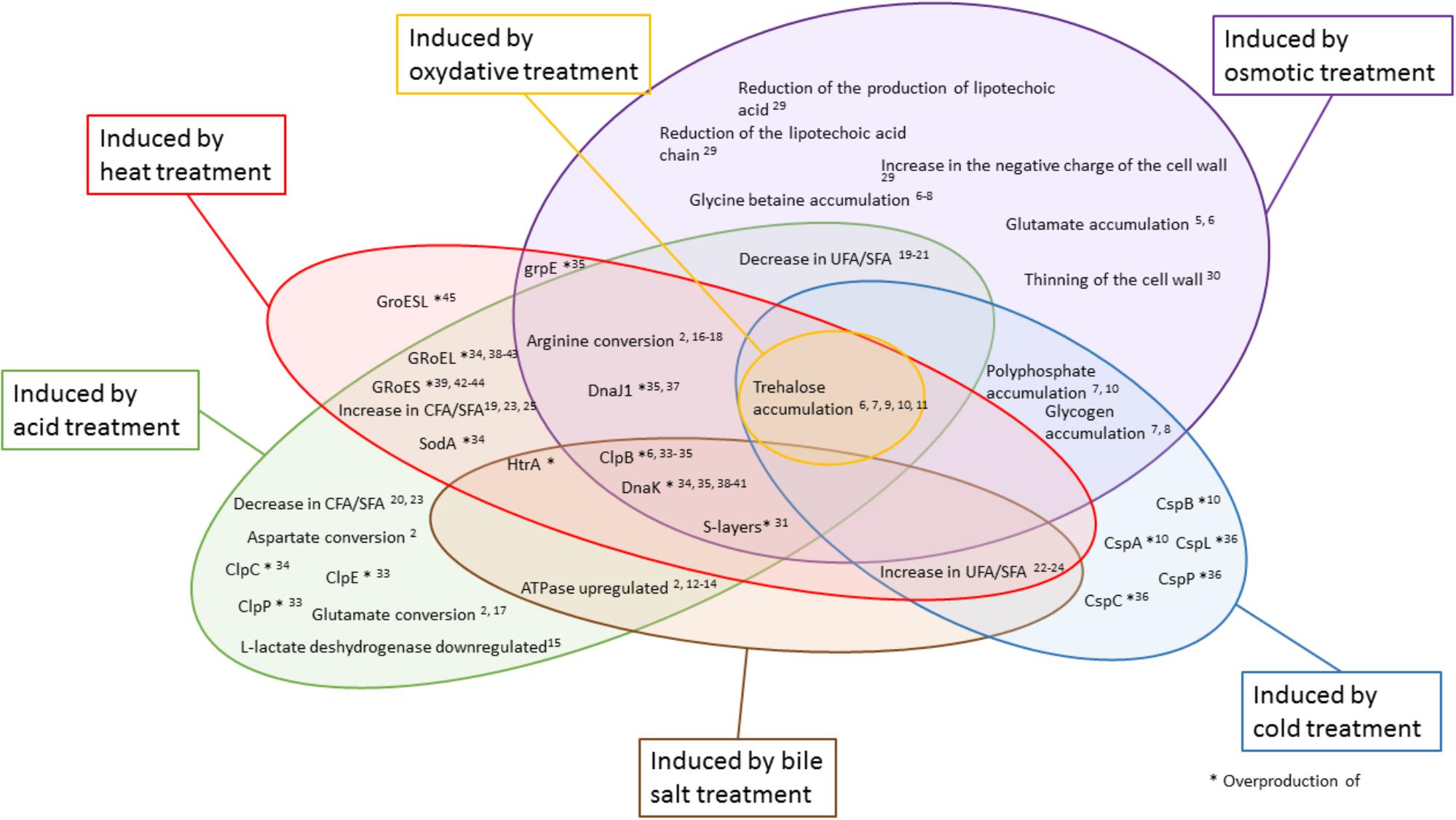
Figure 2. Different treatments modulate the key actors of adaptive mechanisms. Colored areas represent the different treatments studied. In yellow: oxidative; in red: heat; in green: acid; in brown: bile salts; in blue: cold; and in purple: osmotic treatment. The key actors of adaptive mechanisms indicated inside a bubble are modulated by the corresponding treatment. The numbers indicate corresponding references in the tables.
Accumulation of glycerol
Huang et al. (2016b) observed that Glycerol 3–phosphate dehydrogenase was overexpressed by P. freudenreichii in hyperconcentrated sweet whey medium. During osmotic stress, glycerol modification on oligosaccharides is increased. This phenomenon induces a possible increase of hydroxyl groups available number in saccharide molecules and replaces water molecules for cellular interaction, during osmotic stress, for example (Prasad et al., 2003).
Accumulation of Amino Acids
In osmotic adaptation, lactobacilli regulate the intracellular concentration of amino acids like proline and glutamate (Papadimitriou et al., 2016). Glutamate is a key metabolite which plays a role in various cell stress responses (Feehily and Karatzas, 2013). This amino acid is crucial for the primary response to hyper-osmotic shock (Glaasker et al., 1996). The first response to a high osmolarity is the accumulation of K+ and its counterion, glutamate. Glutamate has a minor influence on the acid tolerance capacity of Propionibacterium acidipropionici (Guan et al., 2013). The addition of glutamate in the growth medium enhanced glutamate accumulation by L. sakei, even in the absence of stressing conditions (Ferreira et al., 2005).
An increase in lysine production during stress has been observed in lactobacilli (Papadimitriou et al., 2016). In Propionibacterium acidopropionicii, a similar phenomenon was observed for other amino acids such as arginine and aspartate, which were accumulated during acid adaptation (Guan et al., 2013). GABA (decarboxylation of glutamate to γ-aminobutyrate) is involved in acid tolerance (Bron et al., 2006; Guan et al., 2013). The lysine degradation pathway is activated under acidic conditions in L. plantarum (Heunis et al., 2014).
Glycine betaine is known to protect different bacterial species against high osmolarity and is considered to be the most effective osmoprotectant (Roesser and Müller, 2001). During osmotic stress, P. freudenreichii accumulation of glycine betaine occurs via the OpuABC (or Bus ABC) transporter, which is osmotically induced (Figure 1) (Huang et al., 2016b). The glycine betaine transporter OpuABC of Lactococcus lactis was also well described by Romeo et al. (2003), as well the QacT transporter in L. plantarum by Glaasker et al. (1998).
Accumulation of Energy Storage Compounds
Accumulation of phosphates
Polyphosphates are not only used for energy storage but they are also accumulated by P. freudenreichii during cold (Dalmasso et al., 2012a) and osmotic stresses (Huang et al., 2016b). For lactobacilli, the accumulation of polyphosphates is dependent on high phosphate concentration in the growth medium (Alcantara et al., 2014). Polyphosphates act as chaperones in other bacteria and interact with misfolded proteins in oxidative stress conditions (Gray and Jakob, 2015). Polyphosphate accumulation is recognized as a key factor of stress tolerance in L. casei (Huang et al., 2018).
The polyphosphate kinase, responsible for polyphosphate (Ppk) synthesis, catalyzes the ATP-dependent formation of a phosphoanhydride bond between a polyphosphate chain and orthophosphate. Some lactobacilli have more than one ppk gene involved in polyphosphate synthesis. The number of ppk genes was shown to be correlated with the accumulation of elevated phosphate concentrations (Alcantara et al., 2014).
Accumulation of glycogen
Low temperatures induce glycogen accumulation in P. freudenreichii (Dalmasso et al., 2012b). This bacterium also accumulates glycogen in hyper-concentrated sweet whey medium, which has a high osmotic pressure and provides an abundance of carbon substrate (Huang et al., 2016b). Glycogen accumulation depends on the type of sugar substrate present in the culture medium. Raffinose and trehalose activate the accumulation of glycogen, whereas glucose represses it in lactobacilli (Goh and Klaenhammer, 2014).
Regulation of Energy Production
Intracellular pH (pHi) homeostasis is a prerequisite to normal growth or to survival during stress (Guan et al., 2013). Bacteria triggered different mechanisms to regulate their pHi, such as the upregulation of ATPase activity and the conversion of different substrates.
Regulation of ATPase Activity
Lactobacilli acid tolerance is attributed to the presence of a constant gradient between extracellular and pHi. The ATPase protein is a known mechanism used for protection against acid stress (Figure 1). This protein generates a proton driving force via proton expulsion (Corcoran et al., 2005; Guan et al., 2013). The mechanism is similar for bifidobacteria during acid (Sanchez et al., 2007) and bile salts stresses (Sanchez et al., 2006). The regulation of ATPase activity occurs at the transcriptional level (Broadbent et al., 2010). There is a correlation between APTase activity and acid tolerance (Guan et al., 2013): the higher the ATPase activity is, the higher the acid tolerance will be. However, some lactobacilli species (like L. casei) do not use ATPase as a tolerance response to acid stress, instead, they keep the pHi low and reduce the energy demand for proton translocation, in addition to preventing intracellular accumulation of organic acid (Broadbent et al., 2010).
Regulation of Substrate Conversion
During an acid stress, lactobacilli decrease the production of lactate (Papadimitriou et al., 2016). In cold stress conditions, propionibacteria decrease the production of propionate and acetate from lactate substrate via a redirection of pyruvate from the Wood-Werkman pathway to other metabolic pathways (Dalmasso et al., 2012a). To limit the decrease of the pHi, P. acidopropionici and lactobacilli increase the activity of the arginine deaminase (ADI) system by a factor of three to five. This system allows the degradation of arginine, producing ATP, NH4+, and CO2 (Rollan et al., 2003; Guan et al., 2013; Teixeira et al., 2014). The production of NH4+ and CO2 allows pH homeostasis (Guan et al., 2013), and the ATP produced leads to an exclusion of protons by the APTase. Guan et al. (2013) showed that the conversion of aspartic acid into alanine makes it possible for the latter to contribute to the ADI system. In addition, ADI induction can also occur during osmotic and heat stress in L. fermentum (Vrancken et al., 2009). During acid treatment, Lactobacillus reuteri overexpresses glutamine deaminase and glutamate decarboxylase (Su et al., 2011; Teixeira et al., 2014) (Figure 1). These enzymes also generate NH4+ and CO2 and are involved in pHi homeostasis.
At a low pH, enzymes involved in catabolism and energy production are overproduced (Sanchez et al., 2007). This includes enzymes involved in the consumption of complex carbohydrates that contribute to the bifid shunt (Sanchez et al., 2007). Carbon utilization is more efficient and produces more ATP, which could contribute to the proton exclusion via ATPase (Sanchez et al., 2007). The addition of glucose in the growth medium can improve acid tolerance by providing the ATP pool required for proton extrusion by ATPase (Corcoran et al., 2005). NAD is used in glycolysis so that the NAD/NADH ratio has to be optimized for high ATP production (Guan et al., 2013).
Impact on the Bacterial Envelope
Lactobacilli, propionibacteria, and bifidobacteria encountered membranes damages to during various stresses (Papadimitriou et al., 2016). Indeed, the cytoplasmic membrane acts as a barrier for most solutes. The cell membrane also plays a role in other stresses like acid, cold, heat, and bile salts (Table 1). The cell envelope plays also a key role in the regulation of osmotic stress; and maintains cell shape and counteracts the high intracellular osmotic pressure (Papadimitriou et al., 2016). To restore membrane and cell wall integrity, different adaptation mechanisms are adopted by bacteria.
Regulation of Membrane Fluidity
The membrane has such an important role that the relationship between membrane fluidity and stress tolerance has been used to predict the outcome of cell tolerance to stress (Muller et al., 2011). Indeed, stability and permeability of membranes are both key parameters of adaptation and tolerance toward various stresses (Machado et al., 2004). Modulation of membranes composition as adaptation mechanism, which can occur under stressing conditions, tends to counteract variations of fluidity in order to maintain the structure of the bilayer (Figure 1).
Changes in membrane lipids composition are strain-dependent. As presented in Figure 2, the cyclic/saturated fatty acid ratio can be increased or decreased during osmotic stress. During osmotic adaptation, an increase in the amount of cyclopropane fatty acids is observed in L. lactis, while the unsaturated/saturated ratio in membrane fatty acids remains unchanged (Guillot et al., 2000). However, for other bacteria, a decrease in the unsaturated/saturated lipid ratio in the membrane composition can be observed during osmotic adaptation (Machado et al., 2004). L. casei can do both during acid stress, increasing the number of cyclopropane fatty acids and decreasing the unsaturated/saturated ratio. In acidic conditions, bacteria have to counteract proton influx by increasing the rigidity and compactness of the cytoplasmic membrane (Broadbent et al., 2010). The unsaturated/saturated ratio and the cyclic/saturated ratio decrease in L. delbreuckii subsp. bulgaricus in membrane under acidic conditions, thus leading to a decrease in membrane fluidity (Streit et al., 2008). During heat stress, L. helveticus decreases its membrane fluidity by increasing the number of unsaturated fatty acids (Lanciotti et al., 2001). P. freudenreichii, which contains a majority of odd-numbered membrane unsaturated fatty acids, changes its fatty acid composition under cold stress conditions were observed. It reduces the amount of iso-fatty acids in favor of anteiso-fatty acids (Dalmasso et al., 2012a). As an example, in propionibacteria, branched-chain fatty acids are synthesized due to the activity of branched alpha-keto acid dehydrogenase under cold stress during cheese storage (Dalmasso et al., 2012a). P. freudenreichii uses different enzymes to synthesize branched-chain fatty acids, which result from the catabolism of branched amino acids (Gagnaire et al., 2015), maintaining the fluidity of the membrane in order to counteract the cold stress.
The role of cyclic fatty acids (poorly understood) includes the modulation of the fluidity in order to increase the tolerance to different stresses (Machado et al., 2004). Cyclic fatty acid concentration increases during the stationary phase (Muller et al., 2011). The number of double bonds in unsaturated fatty acids is important: linoleic and linolenic acids have two and three double bonds, respectively, causing more steric hindrances than oleic acid (one double bond). Several double bonds could result in the loss of membrane integrity and cell death (Muller et al., 2011). Indeed, Lactobacillus johnsonii NCC533 supplemented with unsaturated fatty acid shows a higher sensitivity to heat and acid stress.
Fatty acid biosynthesis, or neosynthesis, requires a great deal of energy, but bacteria have the possibility of modifying existing fatty acids. For example L. casei ATCC 334 possesses an enzyme that can add a methylene residue across the cis double bond of C16:1n(9), C18:1n(9), or C18:1n(11) unsaturated fatty acids to form a cyclopropane derivative, thus allowing bacterial adaptation with a minimal energy requirement (Broadbent et al., 2010).
Modulation of the membrane bilayer fatty acid composition seems to be stress-dependent as well as strain-dependent (Figure 2). Different bacteria reach the same goal, environmental adaptation, in different ways.
Regulation of the Cell Wall
Peptidoglycan is a key element for the stability of bacteria. It is composed of glycan chains of repeating N-acetyl-glucosamine and N-acetyl-muramic acid residues, and cross-linked by peptide side chains (Piuri et al., 2005). During osmotic treatment, bacteria are affected by an enlargement of the cell (Piuri et al., 2005). During enlargement, the cell wall loses a layer. Two layers can be observed in high salt conditions, whereas three layers are observed under normal conditions. The cell wall is thus thinned (Figure 1). Under salt stress adaptation, the structure is irregular and seems to be detached from the cytoplasmic membrane. This phenomenon can be attributed to plasmolysis. The presence of protein and teichoic acid in controlled conditions may be responsible for the presence of a third layer (Piuri et al., 2005).
Cells grown in high osmolarity increase the hydrophobicity of their surface, revealed by a higher adherence to the organic solvent. In Gram-positive bacteria, lipoteichoic acids and proteins are the most important cell wall components responsible for surface hydrophobicity. This high hydrophobicity helps bacteria to tolerate the osmotic stress (Machado et al., 2004). L. casei grown in high salt conditions limits its production of lipoteichoic acids. In addition, lipoteichoic acids exhibit a lower mean chain length and a lower D-alanine substitution during osmotic treatment. D-alanine substitution increases negative charges in the cell wall and contributes to bacteria tolerance by helping to evacuate toxic Na+ from the cell wall (Palomino et al., 2013).
Regulation of the Different S-Layers
S-layer proteins are the outer layer component and have several different functions; they maintain cell shape, provide a protective coating, and adhere to the host cell. Lactobacilli overexpress S-layer proteins under stress conditions like bile salts, acid, heat, and salt stresses (Khaleghi et al., 2010; Khaleghi and Kasra, 2012; Grosu-Tudor et al., 2016). Moreover, they are involved in osmoadaptation (Palomino et al., 2016). During osmotic treatment, there is an overproduction of the surface layer proteins A (SlpA) and X (SlpX), and the SlpA/SlpX ratio is modified in L. acidophilus (Palomino et al., 2016). S-layer proteins may have a role as a protective sheath and protect cells against mechanical and osmotic insults. Bacteria may increase the S-layer gene expression in order to maintain the integrity of the cell envelope structure (Figure 1), mainly because of the decrease of the cell wall thickness (Palomino et al., 2016).
Regulation of Exopolysaccharide
Exopolysaccharides include various forms of polysaccharides and are located outside the microbial cell wall. They consist of repeating units of homo- or heteropolysaccharides (Caggianiello et al., 2016). The exopolysaccharides can be strongly or weakly bound to the cell surface and protect bacteria against high temperature, acid, bile salts, and osmotic stress (Alp and Aslim, 2010; Stack et al., 2010; Caggianiello et al., 2016). Exopolysaccharide production is improved under acidic conditions (Torino et al., 2001) and enhances thus the bacterial tolerance response.
Overexpression of Molecular Chaperones and Stress-Responsive Proteases
The synthesis of chaperones and proteases is quickly induced under various stressing conditions (Figure 2) to decrease the deleterious impact of the aggregation of denatured proteins and to refold misfolded ones. Proteases act like the last line of defense when damage is irreversible and leads to amino acids recycling. Proteolysis of cellular proteins, which is a regulated process, can greatly contribute to homeostasis by degrading proteins whose functions are no longer required after modification of environmental parameters (Papadimitriou et al., 2016). DnaK (Hsp70) is one of the well-conserved bacterial chaperones that can refold misfolded proteins (Papadimitriou et al., 2016).
One of the ways to counteract stress is the regulation of membrane fluidity. This rather complex regulation involves GroEL and HSPs universal protein chaperones (Papadimitriou et al., 2016). Indeed, small heat shock proteins regulate membrane lipid polymorphism (Tsvetkova et al., 2002). Accordingly, inactivation of small heat shock proteins affects membrane fluidity in L. plantarum (Capozzi et al., 2011). GroEL and GroES (Hsp60) proteins are overproduced under various stress conditions (Papadimitriou et al., 2016) (Figure 2). It has been shown that Lactobacillus paracasei overproduces GroESL during heat stress (Santivarangkna et al., 2007). GroEL and GroES work in tandem to ensure the correct folding of proteins in an ATP-regulated manner (Mills et al., 2011) (Figure 2).
De Dea Lindner et al. (2007) showed that there are two types of stress protein regulation. In the first type, GroEL, GroES, and ClpC are rapidly induced to a high level after moderate heat stress (+5°C) but are not induced by severe heat treatment (+13°C). In the second type, DnaK, GrpE, DnaJ1, and ClpB are strongly induced in a high heat treatment but not in moderate one (De Dea Lindner et al., 2007). Heat shock proteins are highly conserved but it is not the case for their expression regulation mechanisms. The heat shock response is controlled by a combination system like transcriptional repression and activation (De Dea Lindner et al., 2007).
Bifidobacterium breve overexpresses DnaK, DnaJ, and ClpB in high salt conditions and during high heat treatment, which probably means that there is an overlapping regulatory network that controls both osmotic and severe heat stress (De Dea Lindner et al., 2007). This is consistent with observed cross-protections between heat and salt treatments. These proteins are also overproduced during bile and acid stresses (Leverrier et al., 2004; Bove et al., 2013; Jin et al., 2015).
In addition, P. freudenreichii overproduces HtrA, DnaK, and GroEL during bile salts stress, and HtrA is known to have protease and chaperone activities (Leverrier et al., 2003, 2004). This suggests that bile and salt adaptation are closely related (Savijoki et al., 2005).
The Clp protease family constitutes a major system for general protein turnover in lactobacilli. These proteins work with ATPase to degrade proteins (Papadimitriou et al., 2016). Chaperones and Clp proteins are stress-induced, with induction occurring at the transcription level, and can be regulated by the transcriptional repressors CtsR and HrcA (Papadimitriou et al., 2016).
Cold shock proteins (Csp) are produced in cold conditions (Figure 2) and are involved in mRNA stabilization (Mills et al., 2011). These proteins are essential for growth in cold temperatures (Dalmasso et al., 2012a). The production of three Csps, CspL, CspP, and CspC, in cold conditions resulted in an increased of bacterial tolerance toward temperature downshift (Mills et al., 2011). In cold and other stress conditions, P. feudenreichii upregulates genes coding for two Csp (Dalmasso et al., 2012a). Bifidobacteria have less different chaperones and proteases than other bacteria (De Dea Lindner et al., 2007), in accordance with their great sensitivity to stress.
Chaperones can also behave as moonlight proteins. Moonlighting DnaK and GroEL, for example, have adhesive functions. The overexpression of these proteins during bile or acid stresses can help the bacteria to adhere to intestinal cells and to persist within the digestive tract (Bergonzelli et al., 2006; Candela et al., 2010).
The whole studies reveal that some adaptive mechanisms are common to different treatments. They may be induced by several treatments and explain the observed cross-protections (Figure 2). Osmotic and acid treatments induce large changes in bacterial cells, and only a few adaptation mechanisms are common to both treatments. Oxidative treatment has been less studied than the other treatments.
Stress Adaptation Improves Bacterial Tolerance to Technological and Digestive Stress
Understanding and mastering bacterial adaptation is crucial for strains selection and of pretreatments to improve viability during technological stresses, storage, and digestion (Figure 3). Bacterial survival during technological and digestive stresses is strain-dependent. Some bacteria are naturally more resistant and have the ability to adapt to various stresses. Some growth medium modifications and some physicochemical treatments can improve bacterial survival during technological stresses, thanks to adaptation (Tables 2, 3 and Figure 3), but only a few studies have focused on which adaptive mechanisms are responsible for enhanced survival.
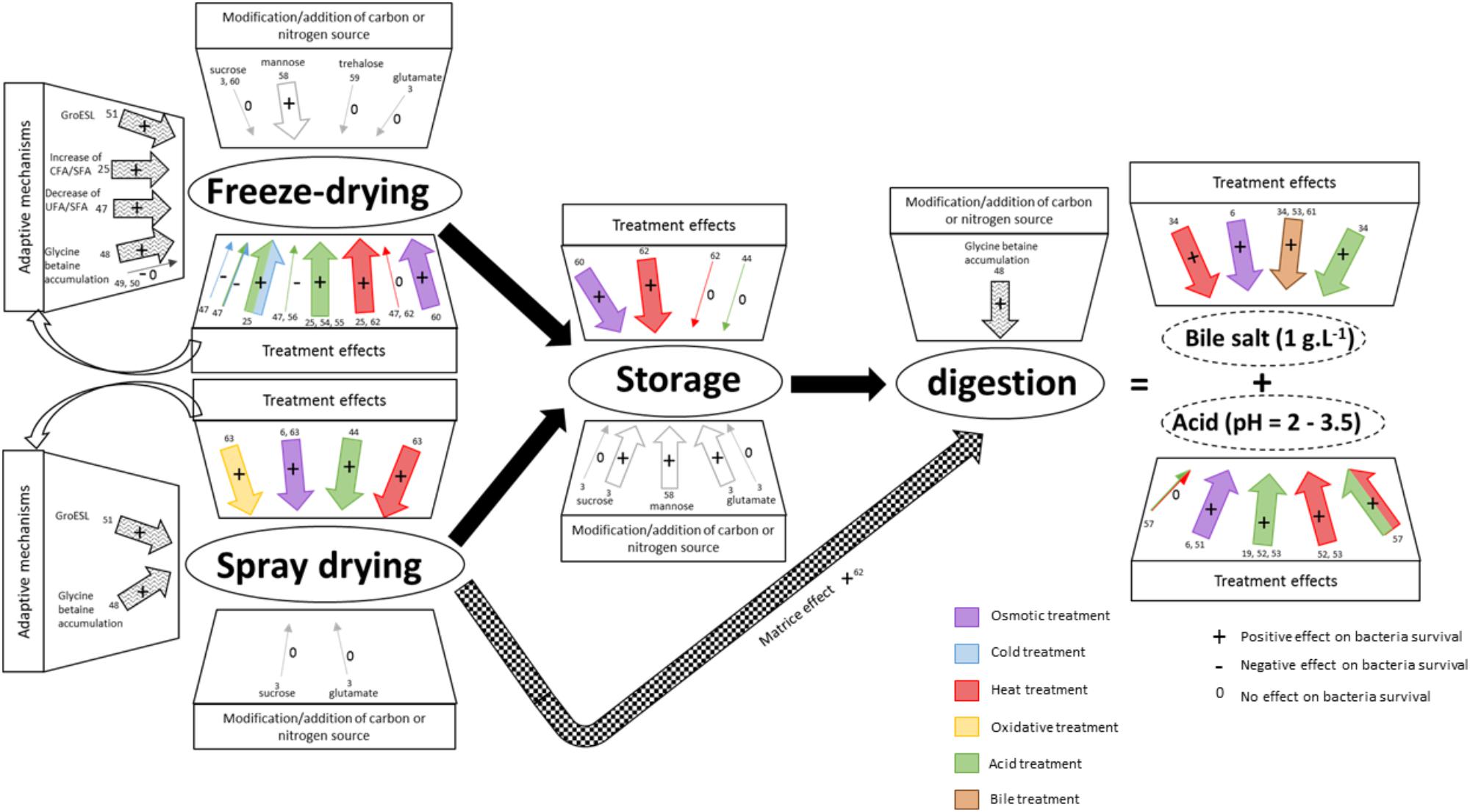
Figure 3. Stressing pretreatments and modifications of the growth medium modulate survival during technological and digestive stresses. Technological and digestive stresses are represented in the figure. Digestion triggers two main stresses: bile salts and acid stress. For each stress, the impact of stressing pretreatments and of modifications of the growth medium on bacteria survival is indicated (+: positive; -: negative; and 0: no effect). Strain-dependent modulations are represented by arrows (purple: osmotic; blue: cold; red: heat; yellow: oxidative; green: acid; brown: bile salts treatment). Large arrows indicate a positive effect and thin arrows indicate either no effect or a negative effect. The numbers indicate corresponding references in the tables.
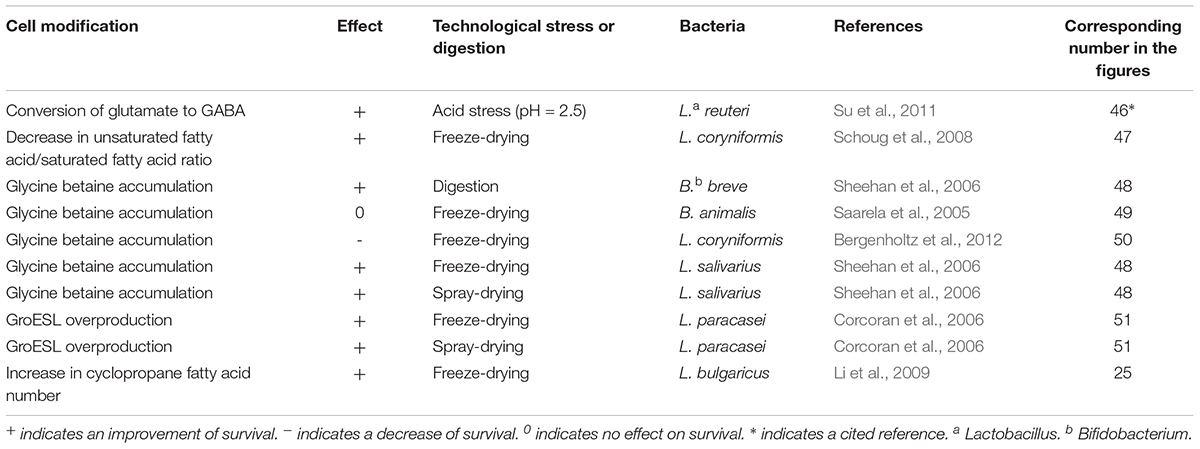
Table 2. Adaptive mechanisms reported to modulate the survival of propionibacteria, bifidobacteria, and lactobacilli under technological and digestive stresses.
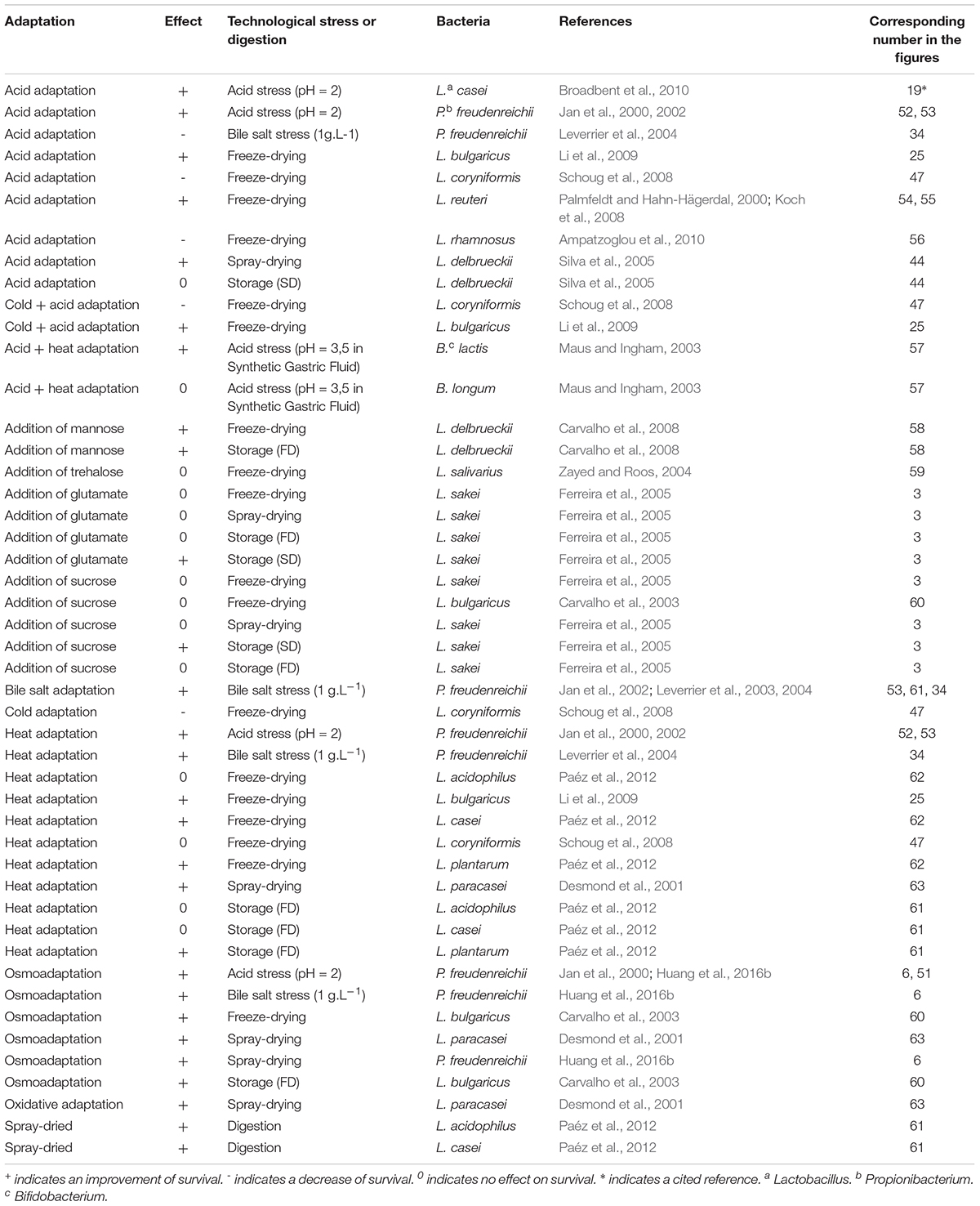
Table 3. Treatments and modifications of the growth medium that modulate the survival of propionibacteria, bifidobacteria, and lactobacilli under technological and digestive stresses.
Enhanced Tolerance Toward Drying Process and Storage
Freeze-Drying Process
Bacteria survival during freeze-drying has been well studied. Many studies presenting the best way to increase survival are available. However, only few studies have focused on the role of bacterial adaptive mechanisms on bacterial survival during this process.
The accumulation of glycine betaine leads to an enhanced survival during freeze-drying for Lactobacillus salivarus (Sheehan et al., 2006). Unfortunately, this mechanism is strain-dependent, as shown in Figure 3. Glycine betaine accumulation can be triggered by osmotic stress (Table 1; Glaasker et al., 1996; Romeo et al., 2003; Huang et al., 2016b). Indeed, as listed in Table 3, the osmoadaptation enhanced Lacotbacillus bulgaricus survival during freeze-drying (Carvalho et al., 2003). Osmotic constraint induces bacterial adaptation, and glycine betaine accumulation can be responsible for the better survival of L. bulgaricus and of other species. Figure 3 shows that acid, heat, and osmotic treatments can improve bacterial survival during freeze-drying in strain-dependent way. Osmotic stress can be also induced by the addition of either salt or sugar. The advantage of a sugar like mannose is that it can increase bacterial survival during freeze-drying (Carvalho et al., 2008). In fact, a growth medium with a high sugar concentration leads to the accumulation of trehalose (Cardoso et al., 2004). In addition, sugars have a positive impact on bacterial survival when they are added at a high concentration to the drying medium (Giulio et al., 2005).
A strain of L. paracasei which overexpresses GroESL presents an improved survival rate after extreme stresses challenges than other L. paracasei strains (Mills et al., 2011). GroESL is a chaperone protein which can be induced during heat treatment (Walker et al., 1999). The overproduction of GroESL or of other general stress proteins is induced by various treatments (Schoug et al., 2008) (Table 1), and their synthesis probably has a considerable impact on bacterial survival during freeze-drying.
Modulation of membrane lipids composition may contribute to enhancing bacterial survival during freeze-drying. In fact, lipid membrane modifications depend on the strain-treatment couple, like other adaptive mechanisms. In the literature, two lipid modifications have been reported to increase bacterial survival during freeze-drying. It includes the increase of the cyclic fatty/saturated fatty acid ratio and the decrease of the unsaturated/saturated membrane fatty acid ratio, these ratios being key features of stress adaptation (see section “Regulation of Membrane Fluidity”) (Table 2 and Figure 3).
Combinations of stress seem to be a promising way to induce multiple adaptation mechanisms, which unfortunately are strain-dependent. The combination of cold and acid treatment was tested, but this association decreased Lactobacillus coryniformis survival during freeze-drying (Schoug et al., 2008). However, Li et al. (2009) demonstrated that L. bulgaricus viability is enhanced during freeze-drying, when it is grown in cold and acid conditions (30°C; pH = 5) or when it is submitted to a mild cold treatment (30°C).
Spray-Drying Process
To enhance the latter, it is possible first to select strains with high intrinsic stress tolerance and the ability to adapt upon pretreatment, which is strain-dependent. Indeed, bifidobacteria species with high heat and moderate oxygen tolerance have a better survival rate after spray-drying (68–102%) compared to species with no intrinsic tolerance to oxygen and to heat stress (Santivarangkna et al., 2007). In addition, it is important to remember that grow phase harvesting may influence the bacterium viability during spray-drying. The stationary phase seems to be a favorable phase to harvest bacteria for drying (Peighambardoust et al., 2011).
The three treatments – osmotic, heat, and acid – can improve bacterial survival during spray-drying or freeze-drying (Figure 3). Although these two drying processes impose opposite stresses – cold and heat stress – the treatments that improve bacterial survival are indeed the same. It is possible to rank treatments according to their efficiency toward protection during spray-drying for L. paracasei NFBC 338. Decreasing efficiency is observed with heat > salt > hydrogen peroxide > and bile treatment (Desmond et al., 2001). Obviously, this ranking is strain-dependent and the addition of another treatment may make more effective bacterial adaptation possible.
The heat tolerance of bacteria is defined by the decimal reduction value (D𝜃 value) that represents the time needed to kill 90% of the bacteria at a given temperature 𝜃. Upon heat adaptation, the D60 value of L. paracasei can increase from 1.7 to 3.1 min (Desmond et al., 2001), in accordance with enhanced tolerance toward spray-drying. Survival of L. salivarius and L. paracasei is also enhanced by heat or oxidative treatments before spray-drying (Desmond et al., 2001). Moreover, during heat stress, GroESL can be overproduced and enhance bacterial survival during spray-drying (Walker et al., 1999; Corcoran et al., 2006). Silva et al. (2005) showed that acid-adapted L. delbrueckii achieves a better tolerance to heat and spray-drying due to the production of heat shock proteins.
In addition, the accumulation of glycine betaine improves lactic acid bacteria viability during spray-drying (Sheehan et al., 2006). High fermentable sugar concentrations in the growth medium permit the production of metabolites like mannitol. Non-fermentable sugars increase the osmotic pressure of the medium and induce osmoadaptation of bacteria, so that both types of sugars improve cell survival during spray-drying (Peighambardoust et al., 2011). Osmoadaptation increases the survival of several species during spray-drying (Desmond et al., 2001; Huang et al., 2016a,b).
Spray-drying is a stressful process that affects the bacterial membrane. Acid, osmotic, heat, and oxidative treatments lead to the modification of the membrane composition (Figure 2). Membrane fluidity should be optimized to increase bacteria survival. If the fluidity is too high or too low, bacterial survival may decrease.
Pretreatments prior to drying are thus promising tools to improve bacterial viability during technological processes, but must be adapted for each strain. It can be observed that bacterial adaptation has its own limits. As an example, the heat adaptation of L. paracasei would be useful in order to deal with high drying outlet temperatures (higher than 95°C) and, conversely, less useful for lower outlet temperatures (85–90°C) because the temperature would cause less damage in this case (Desmond et al., 2001). Moreover, the outlet temperature can be decreased while optimizing the drying parameters. It seems that the best pretreatment would be acid and osmotic stresses for spray-drying.
Storage
The challenge is not only to maintain the viability of bacteria during the drying process, but also during storage. Relative humidity and temperature are two key factors controlling the loss of viability upon storage. Since the oxidation of lipids increases with relative humidity (Golowczyc et al., 2011) and temperature, the stability is negatively correlated with these two factors. Cells have the best stability during storage with a relative humidity of 0%, even if the temperature is 30°C (Golowczyc et al., 2011).
To increase the stability of cells during storage, a protective agent can be added during growth. When monosodium glutamate or fructo-oligosaccharides (FOS) are added during the growth of Lactobacillus kefir, the cells have a higher stability after drying and during storage at 0–11% relative humidity (Golowczyc et al., 2011). Sugars like glucose, fructose, mannose, and sorbitol can provide protection (Peighambardoust et al., 2011). Protection through the addition of sucrose is strain-dependent (Golowczyc et al., 2011). Storage at relative humidity higher than 23% resulted in low stability, regardless of the protective agent, with a correspondingly low D20 value (Golowczyc et al., 2011). This highlights the importance of decreasing the relative humidity of the storage room.
Another way to prepare bacteria to storage conditions is cross-protection. Cells cultivated with a moderate stress can have better stability during storage. Only heat and osmotic treatments are reported to increase bacterial survival upon storage (Figure 3). Indeed, mild heat treatment enhances the stability of L. paracasei (Paéz et al., 2012) and of Lactobacillus rhamnosus (Prasad et al., 2003) during storage, these results being strain-dependent (Paéz et al., 2012).
Osmotic adaptation can also improve stability during storage for L. rhamnosus (Prasad et al., 2003). In powders, the absence of water may have deleterious effects on bacteria. Osmotic treatment during growth induces the accumulation of compatible solutes like glycine betaine, glycerol, and trehalose, and leads to changes in carbohydrate metabolism, resulting in the accumulation of glycerol linked to polysaccharides. These molecules interact with macromolecules instead of water. This protection increases the stability of L. rhamnosus during storage (Prasad et al., 2003). The modification of membrane composition during heat and osmotic treatments may reduce membrane damage, which occurs during storage.
Lactobacillus delbrueckii spp. bulgaricus grown under uncontrolled pH, thus experiencing acid stress, do not have a higher stability during storage (Silva et al., 2005).
Enhance Tolerance Toward Digestive Stresses
During digestion, bile salts and acid stresses are two major stresses which affect probiotics survival and thus their beneficial effects. The effect of adaptation on bacterial survival during digestion is not well known (Figure 3).
Pre-adaptation of bifidobacteria to bile salts induces many metabolic changes. The first is the more efficient use of maltose by Bifidobacterium animalis. In addition, Bifidobacterium longum over-produces mucin-binding protein in acid pH conditions. This should facilitate its efficient targeting to the colon, which is its natural habitat. Moreover, B. animalis overproduces the membrane-bound ATPase that controls the pHi (Sánchez et al., 2013). Finally, adapted bifidobacteria are then able to consume raffinose and maltose, in addition to glucose (Sanchez et al., 2007). All these elements lead to a better survival within the gut, particularly because acid and bile salts adaptation prepare cells to use carbon sources, which cannot be used by the indigenous microbiota (Collado and Sanz, 2007; Sánchez et al., 2013).
Acid response is well documented for bifidobacteria. More than 20 bifidobacteria showed an increase of their tolerance to a simulated GIT after acid adaptation (Sánchez et al., 2013). Acid and heat adaptation can be used together at the same time to improve Bifidobacterium lactis survival to acid stress (Maus and Ingham, 2003).
Heat and osmotic treatments can be used to adapt bacteria to acid stress. In addition, L. casei can be acid-adapted with a treatment at pH 4.5 for 10 or 20 min, with an enhanced viability to acid challenge (Broadbent et al., 2010) and, consequently, to digestion. During bile salts adaptation, some probiotics express a range of bile salts hydrolases which lead a better bacterial viability (Papadimitriou et al., 2016). The spray-drying process can improve the tolerance to simulated gastrointestinal digestion as a result of encapsulation (Paéz et al., 2012) (Figure 3).
The probiotic delivery vehicle, used as drying matrix, has a high impact on viability during digestion. Several studies reported that spray-drying is the best method to maintain and improve the effect of probiotics. The protection of bacteria during digestion, both by encapsulation or by a food matrix, seems to be an important factor and has to be further studied in order to improve the effect of probiotics.
Conclusion
Treatments used to adapt bacteria before technological and digestive challenges offer promising opportunities for improvement. Osmotic and heat treatments reportedly enhance bacteria survival during stressing challenges, via accumulation of compatible solutes and overexpression of key stress proteins, respectively. In the literature, these treatments are reported to have positive impacts on bacterial survival during freeze-drying, spray-drying, storage, and, to a lesser extent, during digestion. Acid treatment also seems promising because it can have a positive impact during drying, bile salts, and acid stress challenges. Treatments including bile salts have not been well studied, particularly in terms of their impact on bacterial survival during technological stresses. The combination of two or three of these stresses could be very interesting, especially the osmotic-acid combination. Indeed, it can be observed that these two treatments can induce a high number of adaptive mechanisms. Starter bacteria apart from two-in-one bacteria do not require an adaptation to digestive stress because they will grow in a food matrix. For starters, osmotic or heat treatment, or the combination thereof, seem to afford protection toward technological constraints. To trigger the adaptation of beneficial bacteria, the growth medium should be adapted, as carbon (saccharides) and nitrogen (amino acids) sources can be modulated. Growth conditions have to be chosen according to the subsequent probiotic/starter use and the strain since this review shows that most of the treatment effects are strain-dependent.
Author Contributions
All authors contributed to the writing of the manuscript. GJ supervised the work.
Conflict of Interest Statement
FG, PM, and PB were employed by the company Bioprox.
The remaining authors declare that the research was conducted in the absence of any commercial or financial relationships that could be construed as a potential conflict of interest.
Acknowledgments
FG is the recipient of a doctoral fellowship from Bioprox and ANRT (Association National de la Recherche et de la Technologie), and HR is the recipient of a BBA (Bretagne Biotechnologie Alimenetaire) and ANRT. The authors would like to thank Alphonsin Marcelin for useful discussions and advice. English was corrected by Gail Wagman.
References
Alcantara, C., Blasco, A., Zuniga, M., and Monedero, V. (2014). Accumulation of polyphosphate in Lactobacillus spp. and its involvement in stress resistance. Appl. Environ. Microbiol. 80, 1650–1659. doi: 10.1128/AEM.03997-13
Alcantara, C., and Zuniga, M. (2012). Proteomic and transcriptomic analysis of the response to bile stress of Lactobacillus casei BL23. Microbiology 158, 1206–1218. doi: 10.1099/mic.0.055657-0
Alp, G., and Aslim, B. (2010). Relationship between the resistance to bile salts and low pH with exopolysaccharide (EPS) production of Bifidobacterium spp. isolated from infants feces and breast milk. Anaerobe 16, 101–105. doi: 10.1016/j.anaerobe.2009.06.006
Ampatzoglou, A., Schurr, B., Deepika, G., Baipong, S., and Charalampopoulos, D. (2010). Influence of fermentation on the acid tolerance and freeze drying survival of Lactobacillus rhamnosus GG. Biochem. Eng. J. 52, 65–70. doi: 10.1016/j.bej.2010.07.005
Arnold, J. W., Simpson, J. B., Roach, J., Kwintkiewicz, J., and Azcarate-Peril, M. A. (2018). Intra-species genomic and physiological variability impact stress resistance in strains of probiotic potential. Front. Microbiol. 9:242. doi: 10.3389/fmicb.2018.00242
Avalljaaskelainen, S., and Palva, A. (2005). surface layers and their applications. FEMS Microbiol. Rev. 29, 511–529. doi: 10.1016/j.femsre.2005.04.003
Bampi, G. B., Backes, G. T., Cansian, R. L., de Matos, F. E., Ansolin, I. M. A., Poleto, B. C., et al. (2016). Spray chilling microencapsulation of Lactobacillus acidophilus and Bifidobacterium animalis subsp. lactis and its use in the preparation of savory probiotic cereal bars. Food Bioproc. Technol. 9, 1422–1428. doi: 10.1007/s11947-016-1724-z
Bergenholtz,ÅS., Wessman, P., Wuttke, A., and Håkansson, S. (2012). A case study on stress preconditioning of a Lactobacillus strain prior to freeze-drying. Cryobiology 64, 152–159. doi: 10.1016/j.cryobiol.2012.01.002
Bergonzelli, G. E., Granato, D., Pridmore, R. D., Marvin-Guy, L. F., Donnicola, D., and Corthesy-Theulaz, I. E. (2006). GroEL of Lactobacillus johnsonii La1 (NCC 533) is cell surface associated: potential role in interactions with the host and the gastric pathogen Helicobacter pylori. Infect. Immun. 74, 425–434. doi: 10.1128/IAI.74.1.425-434.2006
Booth, I. R. (2002). Stress and the single cell: intrapopulation diversity is a mechanism to ensure survival upon exposure to stress. Int. J. Food Microbiol. 78, 19–30. doi: 10.1016/S0168-1605(02)00239-8
Bove, P., Russo, P., Capozzi, V., Gallone, A., Spano, G., and Fiocco, D. (2013). Lactobacillus plantarum passage through an oro-gastro-intestinal tract simulator: carrier matrix effect and transcriptional analysis of genes associated to stress and probiosis. Microbiol. Res. 168, 351–359. doi: 10.1016/j.micres.2013.01.004
Braegger, C., Chmielewska, A., Decsi, T., Kolacek, S., Mihatsch, W., Moreno, L., et al. (2011). Supplementation of infant formula with probiotics and/or prebiotics: a systematic review and comment by the ESPGHAN committee on nutrition. J. Pediatr. Gastroenterol. Nutr. 52, 238–250. doi: 10.1097/MPG.0b013e3181fb9e80
Broadbent, J. R., Larsen, R. L., Deibel, V., and Steele, J. L. (2010). Physiological and transcriptional response of Lactobacillus casei ATCC 334 to acid stress. J. Bacteriol. 192, 2445–2458. doi: 10.1128/JB.01618-09
Bron, P. A., Molenaar, D., Vos, W. M., and Kleerebezem, M. (2006). DNA micro-array-based identification of bile-responsive genes in Lactobacillus plantarum. J. Appl. Microbiol. 100, 728–738. doi: 10.1111/j.1365-2672.2006.02891.x
Brusaferro, A., Cozzali, R., Orabona, C., Biscarini, A., Farinelli, E., Cavalli, E., et al. (2018). Is it time to use probiotics to prevent or treat obesity? Nutrients 10:E1613. doi: 10.3390/nu10111613
Caggianiello, G., Kleerebezem, M., and Spano, G. (2016). Exopolysaccharides produced by lactic acid bacteria: from health-promoting benefits to stress tolerance mechanisms. Appl. Microbiol. Biotechnol. 100, 3877–3886. doi: 10.1007/s00253-016-7471-2
Candela, M., Centanni, M., Fiori, J., Biagi, E., Turroni, S., Orrico, C., et al. (2010). DnaK from Bifidobacterium animalis subsp. lactis is a surface-exposed human plasminogen receptor upregulated in response to bile salts. Microbiology 156, 1609–1618. doi: 10.1099/mic.0.038307-0
Cantero, C., Schrenzel, J., and Darbellay Farhoumand, P. (2018). [Current status of probiotics usage for the prevention of a first episode of Clostridium difficile infection]. Rev. Med. Suisse 14, 1834–1837.
Capozzi, V., Weidmann, S., Fiocco, D., Rieu, A., Hols, P., Guzzo, J., et al. (2011). Inactivation of a small heat shock protein affects cell morphology and membrane fluidity in Lactobacillus plantarum WCFS1. Res. Microbiol. 162, 419–425. doi: 10.1016/j.resmic.2011.02.010
Cardoso, F. S., Castro, R. F., Borges, N., and Santos, H. (2007). Biochemical and genetic characterization of the pathways for trehalose metabolism in Propionibacterium freudenreichii, and their role in stress response. Microbiology 153, 270–280. doi: 10.1099/mic.0.29262-0
Cardoso, F. S., Gaspar, P., Hugenholtz, J., Ramos, A., and Santos, H. (2004). Enhancement of trehalose production in dairy propionibacteria through manipulation of environmental conditions. Int. J. Food Microbiol. 91, 195–204. doi: 10.1016/S0168-1605(03)00387-8
Carvalho, A. S., Silva, J., Ho, P., Teixeira, P., Malcata, F. X., and Gibbs, P. (2003). Effects of addition of sucrose and salt, and of starvation upon thermotolerance and survival during storage of freeze-dried Lactobacillus delbrueckii ssp bulgaricus. J. Food Sci. 68, 2538–2541. doi: 10.1111/j.1365-2621.2003.tb07057.x
Carvalho, A. S., Silva, J., Ho, P., Teixeira, P., Malcata, F. X., and Gibbs, P. (2004). Relevant factors for the preparation of freeze-dried lactic acid bacteria. Int. Dairy J. 14, 835–847. doi: 10.1016/j.idairyj.2004.02.001
Carvalho, A. S., Silva, J., Ho, P., Teixeira, P., Malcata, F. X., and Gibbs, P. (2008). Effects of various sugars added to growth and drying media upon thermotolerance and survival throughout storage of freeze-dried Lactobacillus delbrueckii ssp. bulgaricus. Biotechnol. Prog. 20, 248–254. doi: 10.1021/bp034165y
Chen, M., Sun, Q., Giovannucci, E., Mozaffarian, D., Manson, J. E., Willett, W. C., et al. (2014). Dairy consumption and risk of type 2 diabetes: 3 cohorts of US adults and an updated meta-analysis. BMC Med. 12:215. doi: 10.1186/s12916-014-0215-1
Chervaux, C., Ehrlich, S. D., and Maguin, E. (2000). Physiological study of Lactobacillus delbrueckii subsp. bulgaricus strains in a novel chemically defined medium. Appl. Environ. Microbiol. 66, 5306–5311. doi: 10.1128/AEM.66.12.5306-5311.2000
Collado, M. C., and Sanz, Y. (2007). Induction of acid resistance in Bifidobacterium: a mechanism for improving desirable traits of potentially probiotic strains. J. Appl. Microbiol. 103, 1147–1157. doi: 10.1111/j.1365-2672.2007.03342.x
Collins, M. D., and Gibson, G. R. (1999). Probiotics, prebiotics, and synbiotics: approaches for modulating the microbial ecology of the gut. Am. J. Clin. Nutr. 69, 1052S–1057S. doi: 10.1093/ajcn/69.5.1052s
Colliou, N., Ge, Y., Sahay, B., Gong, M., Zadeh, M., Owen, J. L., et al. (2017). Commensal Propionibacterium strain UF1 mitigates intestinal inflammation via Th17 cell regulation. J. Clin. Invest. 127, 3970–3986. doi: 10.1172/JCI95376
Corcoran, B. M., Ross, R. P., Fitzgerald, G. F., Dockery, P., and Stanton, C. (2006). Enhanced survival of GroESL-overproducing Lactobacillus paracasei NFBC 338 under stressful conditions induced by drying. Appl. Environ. Microbiol. 72, 5104–5107. doi: 10.1128/AEM.02626-05
Corcoran, B. M., Stanton, C., Fitzgerald, G. F., and Ross, R. P. (2005). Survival of probiotic Lactobacilli in acidic environments is enhanced in the presence of metabolizable sugars. Appl. Environ. Microbiol. 71, 3060–3067. doi: 10.1128/AEM.71.6.3060-3067.2005
Cousin, F. J., Mater, D. D. G., Foligne, B., and Jan, G. (2010). Dairy propionibacteria as human probiotics: a review of recent evidence. Dairy Sci. Technol. 96, 1–26. doi: 10.1051/dst/2010032
Csonka, L. N. (1989). Physiological and genetic responses of bacteria to osmotic stress. Microbiol. Rev. 53, 121–147.
Csonka, L. N., and Hanson, A. D. (1991). Prokaryotic osmoregulation: genetics and physiology. Annu. Rev. Microbiol. 45, 569–606. doi: 10.1146/annurev.mi.45.100191.003033
Dalmasso, M., Aubert, J., Even, S., Falentin, H., Maillard, M.-B., Parayre, S., et al. (2012b). Accumulation of intracellular glycogen and trehalose by Propionibacterium freudenreichii under conditions mimicking cheese ripening in the cold. Appl. Environ. Microbiol. 78, 6357–6364. doi: 10.1128/AEM.00561-12
Dalmasso, M., Aubert, J., Briard-Bion, V., Chuat, V., Deutsch, S.-M., Even, S., et al. (2012a). A temporal -omic study of Propionibacterium freudenreichii CIRM-BIA1T adaptation strategies in conditions mimicking cheese ripening in the cold. PLoS One 7:e29083. doi: 10.1371/journal.pone.0029083
Dasari, S., Kathera, C., Janardhan, A., Praveen Kumar, A., and Viswanath, B. (2017). Surfacing role of probiotics in cancer prophylaxis and therapy: a systematic review. Clin. Nutr. 36, 1465–1472. doi: 10.1016/j.clnu.2016.11.017
David, L. A., Maurice, C. F., Carmody, R. N., Gootenberg, D. B., Button, J. E., Wolfe, B. E., et al. (2014). Diet rapidly and reproducibly alters the human gut microbiome. Nature 505, 559–563. doi: 10.1038/nature12820
De Dea Lindner, J., Canchaya, C., Zhang, Z., Neviani, E., Fitzgerald, G. F., van Sinderen, D., et al. (2007). Exploiting Bifidobacterium genomes: the molecular basis of stress response. Int. J. Food Microbiol. 120, 13–24. doi: 10.1016/j.ijfoodmicro.2007.06.016
Derzelle, S., Hallet, B., Francis, K. P., Ferain, T., Delcour, J., and Hols, P. (2000). Changes in cspL, cspP, and cspC mRNA abundance as a function of cold shock and growth phase in Lactobacillus plantarum. J. Bacteriol. 182, 5105–5113. doi: 10.1128/JB.182.18.5105-5113.2000
Desmond, C., Stanton, C., Fitzgerald, G. F., Collins, K., and Paul Ross, R. (2001). Environmental adaptation of probiotic Lactobacilli towards improvement of performance during spray drying. Int. Dairy J. 11, 801–808. doi: 10.1016/S0958-6946(01)00121-2
Deutsch, S.-M., Mariadassou, M., Nicolas, P., Parayre, S., Le Guellec, R., Chuat, V., et al. (2017). Identification of proteins involved in the anti-inflammatory properties of Propionibacterium freudenreichii by means of a multi-strain study. Sci. Rep. 7:46409. doi: 10.1038/srep46409
Dimitrellou, D., Kandylis, P., Petrović, T., Dimitrijević-Branković, S., Lević, S., Nedović, V., et al. (2016). Survival of spray dried microencapsulated Lactobacillus casei ATCC 393 in simulated gastrointestinal conditions and fermented milk. LWT-Food Sci. Technol. 71, 169–174. doi: 10.1016/j.lwt.2016.03.007
do Carmo, F. L. R., Rabah, H., De Oliveira Carvalho, R. D., Gaucher, F., Cordeiro, B. F., da Silva, S. H., et al. (2018). Extractable bacterial surface proteins in probiotic–host interaction. Front. Microbiol. 9:645. doi: 10.3389/fmicb.2018.00645
DuPont, A. W., Richards, D. M., Jelinek, K. A., Krill, J. T., Rahimi, E. F., and Ghouri, Y. A. (2014). Systematic review of randomized controlled trials of probiotics, prebiotics, and synbiotics in inflammatory bowel disease. Clin. Exp. Gastroenterol. 7, 473–487. doi: 10.2147/CEG.S27530
Ebner, S., Smug, L. N., Kneifel, W., Salminen, S. J., and Sanders, M. E. (2014). Probiotics in dietary guidelines and clinical recommendations outside the European Union. World J. Gastroenterol. 20, 16095–16100. doi: 10.3748/wjg.v20.i43.16095
Elli, M., Zink, R., Rytz, A., Reniero, R., and Morelli, L. (2000). Iron requirement of Lactobacillus spp. in completely chemically defined growth media. J. Appl. Microbiol. 88, 695–703. doi: 10.1046/j.1365-2672.2000.01013.x
FAO/WHO (2001). Evaluation of Health and Nutritional Properties of Powder Milk and Live Lactic Acid Bacteria. Food and Agriculture Organization of the United Nations and World Health Organization Expert Consultation Report. Rome: FAO.
Feehily, C., and Karatzas, K. A. G. (2013). Role of glutamate metabolism in bacterial responses towards acid and other stresses. J. Appl. Microbiol. 114, 11–24. doi: 10.1111/j.1365-2672.2012.05434.x
Ferreira, V., Soares, V., Santos, C., Silva, J., Gibbs, P. A., and Teixeira, P. (2005). Survival of Lactobacillus sakei during heating, drying and storage in the dried state when growth has occurred in the presence of sucrose or monosodium glutamate. Biotechnol. Lett. 27, 249–252. doi: 10.1007/s10529-004-8351-x
Foligné, B., Breton, J., Mater, D., and Jan, G. (2013). Tracking the microbiome functionality: focus on Propionibacterium species. Gut 62, 1227–1228. doi: 10.1136/gutjnl-2012-304393
Foligne, B., Deutsch, S.-M., Breton, J., Cousin, F. J., Dewulf, J., Samson, M., et al. (2010). Promising immunomodulatory effects of selected strains of dairy Propionibacteria as evidenced in vitro and in vivo. Appl. Environ. Microbiol. 76, 8259–8264. doi: 10.1128/AEM.01976-10
Fortina, M. G., Nicastro, G., Carminati, D., Neviani, E., and Manachini, P. L. (1998). Lactobacillus helveticus heterogeneity in natural cheese starters: the diversity in phenotypic characteristics. J. Appl. Microbiol. 84, 72–80. doi: 10.1046/j.1365-2672.1997.00312.x
Gagnaire, V., Jardin, J., Rabah, H., Briard-Bion, V., and Jan, G. (2015). Emmental cheese environment enhances Propionibacterium freudenreichii stress tolerance. PLoS One 10:e0135780. doi: 10.1371/journal.pone.0135780
Gao, C., Major, A., Rendon, D., Lugo, M., Jackson, V., Shi, Z., et al. (2015). Histamine H2 receptor-mediated suppression of intestinal inflammation by probiotic Lactobacillus reuteri. MBio 6:e1358-15. doi: 10.1128/mBio.01358-15
Ge, Y., Gong, M., Colliou, N., Zadeh, M., Li, J., Jones, D. P., et al. (2019). Neonatal intestinal immune regulation by the commensal bacterium, P. UF1. Mucosal Immunol. 12, 434–444. doi: 10.1038/s41385-018-0125-1
Giulio, B. D., Orlando, P., Barba, G., Coppola, R., Rosa, M. D., Sada, A., et al. (2005). Use of alginate and cryo-protective sugars to improve the viability of lactic acid bacteria after freezing and freeze-drying. World J. Microbiol. Biotechnol. 21, 739–746. doi: 10.1007/s11274-004-4735-2
Glaasker, E., Heuberger, E. H., Konings, W. N., and Poolman, B. (1998). Mechanism of osmotic activation of the quaternary ammonium compound transporter (QacT) of Lactobacillus plantarum. J. Bacteriol. 180, 5540–5546.
Glaasker, E., Konings, W. N., and Poolman, B. (1996). Osmotic regulation of intracellular solute pools in Lactobacillus plantarum. J. Bacteriol. 178, 575–582. doi: 10.1128/jb.178.3.575-582.1996
Goh, Y. J., and Klaenhammer, T. R. (2014). Insights into glycogen metabolism in Lactobacillus acidophilus: impact on carbohydrate metabolism, stress tolerance and gut retention. Microb. Cell Fact. 13:94. doi: 10.1186/s12934-014-0094-3
Golowczyc, M. A., Gerez, C. L., Silva, J., Abraham, A. G., De Antoni, G. L., and Teixeira, P. (2011). Survival of spray-dried Lactobacillus kefir is affected by different protectants and storage conditions. Biotechnol. Lett. 33, 681–686. doi: 10.1007/s10529-010-0491-6
Gouesbet, G., Jan, G., and Boyaval, P. (2002). Two-dimensional electrophoresis study of Lactobacillus delbrueckii subsp. bulgaricus Thermotolerance. Appl. Environ. Microbiol. 68, 1055–1063. doi: 10.1128/AEM.68.3.1055-1063.2002
Gray, M. J., and Jakob, U. (2015). Oxidative stress protection by polyphosphate—new roles for an old player. Curr. Opin. Microbiol. 24, 1–6. doi: 10.1016/j.mib.2014.12.004
Grosu-Tudor, S.-S., Brown, L., Hebert, E. M., Brezeanu, A., Brinzan, A., Fadda, S., et al. (2016). S-layer production by Lactobacillus acidophilus IBB 801 under environmental stress conditions. Appl. Microbiol. Biotechnol. 100, 4573–4583. doi: 10.1007/s00253-016-7355-5
Guan, N., Liu, L., Shin, H., Chen, R. R., Zhang, J., Li, J., et al. (2013). Systems-level understanding of how Propionibacterium acidipropionici respond to propionic acid stress at the microenvironment levels: mechanism and application. J. Biotechnol. 167, 56–63. doi: 10.1016/j.jbiotec.2013.06.008
Guarner, F., Perdigon, G., Corthier, G., Salminen, S., Koletzko, B., and Morelli, L. (2005). Should yoghurt cultures be considered probiotic? Br. J. Nutr. 93, 783–786. doi: 10.1079/BJN20051428
Guillot, A., Obis, D., and Mistou, M.-Y. (2000). Fatty acid membrane composition and activation of glycine-betaine transport in Lactococcus lactis subjected to osmotic stress. Int. J. Food Microbiol. 55, 47–51. doi: 10.1016/S0168-1605(00)00193-8
Hammes, W. P., and Hertel, C. (2015). “Lactobacillus,” in Bergey’s Manual of Systematics of Archaea and Bacteria, eds W. B. Whitman, F. Rainey, P. Kämpfer, M. Trujillo, J. Chun, P. DeVos, et al. (Chichester: John Wiley & Sons Ltd.),1–76.
Heunis, T., Deane, S., Smit, S., and Dicks, L. M. T. (2014). Proteomic profiling of the acid stress response in Lactobacillus plantarum 423. J. Proteome Res. 13, 4028–4039. doi: 10.1021/pr500353x
Homayouni, A., Azizi, A., Ehsani, M. R., Yarmand, M. S., and Razavi, S. H. (2008). Effect of microencapsulation and resistant starch on the probiotic survival and sensory properties of synbiotic ice cream. Food Chem. 111, 50–55. doi: 10.1016/j.foodchem.2008.03.036
Hsu, C. A., Yu, R. C., Lee, S. L., and Chou, C. C. (2007). Cultural condition affecting the growth and production of β-galactosidase by Bifidobacterium longum CCRC 15708 in a jar fermenter. Int. J. Food Microbiol. 116, 186–189. doi: 10.1016/j.ijfoodmicro.2006.12.034
Huang, S., Gaucher, F., Cauty, C., Jardin, J., Le Loir, Y., Jeantet, R., et al. (2018). Growth in hyper-concentrated sweet whey triggers multi stress tolerance and spray drying survival in Lactobacillus casei BL23: from the molecular basis to new perspectives for sustainable probiotic production. Front. Microbiol. 9:2548. doi: 10.3389/fmicb.2018.02548
Huang, S., Rabah, H., Jardin, J., Briard-Bion, V., Parayre, S., Maillard, M.-B., et al. (2016b). Hyperconcentrated sweet whey, a new culture medium that enhances Propionibacterium freudenreichii stress tolerance. Appl. Environ. Microbiol. 82, 4641–4651. doi: 10.1128/AEM.00748-16
Huang, S., Cauty, C., Dolivet, A., Le Loir, Y., Chen, X. D., Schuck, P., et al. (2016a). Double use of highly concentrated sweet whey to improve the biomass production and viability of spray-dried probiotic bacteria. J. Funct. Foods 23, 453–463. doi: 10.1016/j.jff.2016.02.050
Huang, S., Vignolles, M.-L., Chen, X. D., Le Loir, Y., Jan, G., Schuck, P., et al. (2017). Spray drying of probiotics and other food-grade bacteria: a review. Trends Food Sci. Technol. 63, 1–17. doi: 10.1016/j.tifs.2017.02.007
Hynönen, U., Kant, R., Lähteinen, T., Pietilä, T. E., Beganović, J., Smidt, H., et al. (2014). Functional characterization of probiotic surface layer protein-carrying Lactobacillus amylovorus strains. BMC Microbiol. 14:199. doi: 10.1186/1471-2180-14-199
Jan, G., Leverrier, P., Pichereau, V., and Boyaval, P. (2001). Changes in protein synthesis and morphology during acid adaptation of Propionibacterium freudenreichii. Appl. Environ. Microbiol. 67, 2029–2036. doi: 10.1128/AEM.67.5.2029-2036.2001
Jan, G., Leverrier, P., Proudy, I., and Roland, N. (2002). Survival and beneficial effects of propionibacteria in the human gut: in vivo and in vitro investigations. Le Lait 82, 131–144. doi: 10.1051/lait:2001012
Jan, G., Rouault, A., and Maubois, J.-L. (2000). Acid stress susceptibility and acid adaptation of Propionibacterium freudenreichii subsp. shermanii. Le Lait 80, 325–336. doi: 10.1051/lait:2000128
Jin, J., Qin, Q., Guo, H., Liu, S., Ge, S., Zhang, H., et al. (2015). Effect of pre-stressing on the acid-stress response in Bifidobacterium revealed using proteomic and physiological approaches. PLoS One 10:e0117702. doi: 10.1371/journal.pone.0117702
Kailasapathy, K. (2006). Survival of free and encapsulated probiotic bacteria and their effect on the sensory properties of yoghurt. LWT-Food Sci. Technol. 39, 1221–1227. doi: 10.3168/jds.2012-5710
Kailasapathy, K., and Chin, J. (2000). Survival and therapeutic potential of probiotic organisms with reference to Lactobacillus acidophilus and Bifidobacterium spp. Immunol. Cell Biol. 78, 80–88. doi: 10.1046/j.1440-1711.2000.00886.x
Kato-Kataoka, A., Nishida, K., Takada, M., Kawai, M., Kikuchi-Hayakawa, H., Suda, K., et al. (2016). Fermented milk containing Lactobacillus casei strain shirota preserves the diversity of the gut microbiota and relieves abdominal dysfunction in healthy medical students exposed to academic stress. Appl. Environ. Microbiol. 82, 3649–3658. doi: 10.1128/AEM.04134-15
Kets, E., Teunissen, P., and de Bont, J. (1996). Effect of compatible solutes on survival of lactic acid bacteria subjected to drying. Appl. Environ. Microbiol. 62, 259–261.
Khaleghi, M., and Kasra, R. (2012). “Effect of environmental stresses on S-layer production in Lactobacillus acidophilus ATCC 4356,” in Advances in Applied Biotechnology, ed. M. Petre (Shanghai: InTech).
Khaleghi, M., Kermanshahi, R. K., Yaghoobi, M. M., Zarkesh-Esfahani, S. H., and Baghizadeh, A. (2010). Assessment of bile salt effects on s-layer production, slp gene expression and some physicochemical properties of Lactobacillus acidophilus ATCC 4356. J. Microbiol. Biotechnol. 20, 749–756.
Khalesi, S., Bellissimo, N., Vandelanotte, C., Williams, S., Stanley, D., and Irwin, C. (2018). A review of probiotic supplementation in healthy adults: helpful or hype? Eur. J. Clin. Nutr. 73, 24–37. doi: 10.1038/s41430-018-0135-9
Koch, S., Eugster-Meier, E., Oberson, G., Meile, L., and Lacroix, C. (2008). Effects of strains and growth conditions on autolytic activity and survival to freezing and lyophilization of Lactobacillus delbrueckii ssp. lactis isolated from cheese. Int. Dairy J. 18, 187–196. doi: 10.1016/j.idairyj.2007.07.009
Konstantinov, S. R., Smidt, H., de Vos, W. M., Bruijns, S. C. M., Singh, S. K., Valence, F., et al. (2008). S layer protein A of Lactobacillus acidophilus NCFM regulates immature dendritic cell and T cell functions. Proc. Natl. Acad. Sci. U.S.A. 105, 19474–19479. doi: 10.1073/pnas.0810305105
Koponen, J., Laakso, K., Koskenniemi, K., Kankainen, M., Savijoki, K., Nyman, T. A., et al. (2012). Effect of acid stress on protein expression and phosphorylation in Lactobacillus rhamnosus GG. J. Proteom. 75, 1357–1374. doi: 10.1016/j.jprot.2011.11.009
Kurtmann, L., Carlsen, C. U., Risbo, J., and Skibsted, L. H. (2009). Storage stability of freeze–dried Lactobacillus acidophilus (La-5) in relation to water activity and presence of oxygen and ascorbate. Cryobiology 58, 175–180. doi: 10.1016/j.cryobiol.2008.12.001
Kwon, M.-S., Lim, S. K., Jang, J.-Y., Lee, J., Park, H. K., Kim, N., et al. (2018). Lactobacillus sakei WIKIM30 ameliorates atopic dermatitis-like skin lesions by inducing regulatory T Cells and altering gut microbiota structure in mice. Front. Immunol. 9:1905. doi: 10.3389/fimmu.2018.01905
Lan, A., Bruneau, A., Bensaada, M., Philippe, C., Bellaud, P., Rabot, S., et al. (2008). Increased induction of apoptosis by Propionibacterium freudenreichii TL133 in colonic mucosal crypts of human microbiota-associated rats treated with 1,2-dimethylhydrazine. Br. J. Nutr. 100, 1251–1259. doi: 10.1017/S0007114508978284
Lanciotti, R., Guerzoni, M. E., and Cocconcelli, P. S. (2001). Alteration in cellular fatty acid composition as a response to salt, acid, oxidative and thermal stresses in Lactobacillus helveticus. Microbiology 147, 2255–2264. doi: 10.1099/00221287-147-8-2255
Le Maréchal, C., Peton, V., Plé, C., Vroland, C., Jardin, J., Briard-Bion, V., et al. (2015). Surface proteins of Propionibacterium freudenreichii are involved in its anti-inflammatory properties. J. Proteomics 113, 447–461. doi: 10.1016/j.jprot.2014.07.018
Lebeer, S., Vanderleyden, J., and De Keersmaecker, S. C. J. (2008). Genes and molecules of Lactobacilli supporting probiotic action. Microbiol. Mol. Biol. Rev. 72, 728–764. doi: 10.1128/MMBR.00017-08
LeBlanc, J. G., Chain, F., Martín, R., Bermúdez-Humarán, L. G., Courau, S., and Langella, P. (2017). Beneficial effects on host energy metabolism of short-chain fatty acids and vitamins produced by commensal and probiotic bacteria. Microb. Cell Fact. 16:79. doi: 10.1186/s12934-017-0691-z
Leverrier, P., Dimova, D., Pichereau, V., Auffray, Y., Boyaval, P., and Jan, G. (2003). Susceptibility and adaptive response to bile salts in Propionibacterium freudenreichii: physiological and proteomic analysis. Appl. Environ. Microbiol. 69, 3809–3818. doi: 10.1128/AEM.69.7.3809-3818.2003
Leverrier, P., Vissers, J. P. C., Rouault, A., Boyaval, P., and Jan, G. (2004). Mass spectrometry proteomic analysis of stress adaptation reveals both common and distinct response pathways in Propionibacterium freudenreichii. Arch. Microbiol. 181, 215–230. doi: 10.1007/s00203-003-0646-0
Levri, K. M., Ketvertis, K., Deramo, M., Merenstein, J. H., and D’Amico, F. (2005). Do probiotics reduce adult lactose intolerance? A systematic review. J. Fam. Pract. 54, 613–620.
Li, C., Zhao, J.-L., Wang, Y.-T., Han, X., and Liu, N. (2009). Synthesis of cyclopropane fatty acid and its effect on freeze-drying survival of Lactobacillus bulgaricus L2 at different growth conditions. World J. Microbiol. Biotechnol. 25, 1659–1665. doi: 10.1007/s11274-009-0060-0
Li, Y., Kan, Z., You, Y., Gao, X., Wang, Z., and Fu, R. (2015). Exogenous transglutaminase improves multiple-stress tolerance in Lactococcus lactis and other lactic acid bacteria with glutamine and lysine in the cell wall. Biotechnol. Lett. 37, 2467–2474. doi: 10.1007/s10529-015-1942-x
Lightfoot, Y. L., Selle, K., Yang, T., Goh, Y. J., Sahay, B., Zadeh, M., et al. (2015). SIGNR3-dependent immune regulation by Lactobacillus acidophilus surface layer protein A in colitis. EMBO J. 34, 881–895. doi: 10.15252/embj.201490296
Lim, E. M., Lafon, A., Dridi, L., Boudebbouze, S., Dusko Ehrlich, S., and Maguin, E. (2001). Identification de protéines de stress chez Lactobacillus delbrueckii bulgaricus par électrophorèse bidimensionnelle. Le Lait 81, 317–325. doi: 10.1051/lait:2001135
Lim, J., Yoon, S., Tan, P.-L., Yang, S., Kim, S., and Park, H. (2018). Probiotic properties of Lactobacillus Plantarum LRCC5193, a plant-origin lactic acid bacterium isolated from Kimchi and its use in chocolates: properties of chocolate of L. plantarum. J. Food Sci. 83, 2802–2811. doi: 10.1111/1750-3841.14364
Louis, P., and Flint, H. J. (2017). Formation of propionate and butyrate by the human colonic microbiota: propionate and butyrate producing gut microbes. Environ. Microbiol. 19, 29–41. doi: 10.1111/1462-2920.13589
Machado, M. C., López, C. S., Heras, H., and Rivas, E. A. (2004). Osmotic response in Lactobacillus casei ATCC 393: biochemical and biophysical characteristics of membrane. Arch. Biochem. Biophys. 422, 61–70. doi: 10.1016/j.abb.2003.11.001
Mainville, I., Arcand, Y., and Farnworth, E. R. (2005). A dynamic model that simulates the human upper gastrointestinal tract for the study of probiotics. Int. J. Food Microbiol. 99, 287–296. doi: 10.1016/j.ijfoodmicro.2004.08.020
Marco, M. L., Heeney, D., Binda, S., Cifelli, C. J., Cotter, P. D., Foligné, B., et al. (2017). Health benefits of fermented foods: microbiota and beyond. Curr. Opin. Biotechnol. 44, 94–102. doi: 10.1016/j.copbio.2016.11.010
Marketsandmarkets.com (2014). Starter Culture Market Worth $1.0 Billion By 2018. Novi, MI: MarketsandMarkets Research Private Ltd.
Marketsandmarkets.com (2017). Probiotics Market by Application (Functional Food & Beverages (Dairy, Non-dairy Beverages, Baked Goods, Meat, Cereal), Dietary Supplements, Animal Feed), Source (Bacteria, Yeast), Form (Dry, Liquid), End User (Human, Animal), and Region - Forecast to 2022. Novi, MI: MarketsandMarkets Research Private Ltd.
Maus, J. E., and Ingham, S. C. (2003). Employment of stressful conditions during culture production to enhance subsequent cold- and acid-tolerance of bifidobacteria. J. Appl. Microbiol. 95, 146–154. doi: 10.1046/j.1365-2672.2003.01954.x
Meira, Q. G. S., Magnani, M., de Medeiros Júnior, F. C., Queiroga, R., de, C. R., do, E., et al. (2015). Effects of added Lactobacillus acidophilus and Bifidobacterium lactis probiotics on the quality characteristics of goat ricotta and their survival under simulated gastrointestinal conditions. Food Res. Int. 76, 828–838. doi: 10.1016/j.foodres.2015.08.002
Mende, S., Rohm, H., and Jaros, D. (2016). Influence of exopolysaccharides on the structure, texture, stability and sensory properties of yoghurt and related products. Int. Dairy J. 52, 57–71. doi: 10.1016/j.idairyj.2015.08.002
Mills, S., Stanton, C., Fitzgerald, G. F., and Ross, R. P. (2011). Enhancing the stress responses of probiotics for a lifestyle from gut to product and back again. Microb. Cell Fact. 10(Suppl. 1):S19. doi: 10.1186/1475-2859-10-S1-S19
Moens, F., Verce, M., and De Vuyst, L. (2017). Lactate- and acetate-based cross-feeding interactions between selected strains of lactobacilli, bifidobacteria and colon bacteria in the presence of inulin-type fructans. Int. J. Food Microbiol. 241, 225–236. doi: 10.1016/j.ijfoodmicro.2016.10.019
Muller, J. A., Ross, R. P., Sybesma, W. F. H., Fitzgerald, G. F., and Stanton, C. (2011). Modification of the technical properties of Lactobacillus johnsonii NCC 533 by supplementing the growth medium with unsaturated fatty acids. Appl. Environ. Microbiol. 77, 6889–6898. doi: 10.1128/AEM.05213-11
Paéz, R., Lavari, L., Vinderola, G., Audero, G., Cuatrin, A., Zaritzky, N., et al. (2012). Effect of heat treatment and spray drying on lactobacilli viability and resistance to simulated gastrointestinal digestion. Food Res. Int. 48, 748–754. doi: 10.1016/j.foodres.2012.06.018
Pakdaman, M. N., Udani, J. K., Molina, J. P., and Shahani, M. (2015). The effects of the DDS-1 strain of lactobacillus on symptomatic relief for lactose intolerance - a randomized, double-blind, placebo-controlled, crossover clinical trial. Nutr. J. 15:56. doi: 10.1186/s12937-016-0172-y
Palmfeldt, J., and Hahn-Hägerdal, B. (2000). Influence of culture pH on survival of Lactobacillus reuteri subjected to freeze-drying. Int. J. Food Microbiol. 55, 235–238. doi: 10.1016/S0168-1605(00)00176-8
Palomino, M. M., Allievi, M. C., Grundling, A., Sanchez-Rivas, C., and Ruzal, S. M. (2013). Osmotic stress adaptation in Lactobacillus casei BL23 leads to structural changes in the cell wall polymer lipoteichoic acid. Microbiology 159, 2416–2426. doi: 10.1099/mic.0.070607-0
Palomino, M. M., Waehner, P. M., Fina Martin, J., Ojeda, P., Malone, L., Sánchez Rivas, C., et al. (2016). Influence of osmotic stress on the profile and gene expression of surface layer proteins in Lactobacillus acidophilus ATCC 4356. Appl. Microbiol. Biotechnol. 100, 8475–8484. doi: 10.1007/s00253-016-7698-y
Papadimitriou, K., Alegría,Á, Bron, P. A., de Angelis, M., Gobbetti, M., Kleerebezem, M., et al. (2016). Stress physiology of lactic acid bacteria. Microbiol. Mol. Biol. Rev. 80, 837–890. doi: 10.1128/MMBR.00076-15
Parvez, S., Malik, K. A., Ah Kang, S., and Kim, H.-Y. (2006). Probiotics and their fermented food products are beneficial for health. J. Appl. Microbiol. 100, 1171–1185. doi: 10.1111/j.1365-2672.2006.02963.x
Peighambardoust, S. H., Golshan Tafti, A., and Hesari, J. (2011). Application of spray drying for preservation of lactic acid starter cultures: a review. Trends Food Sci. Technol. 22, 215–224. doi: 10.1016/j.tifs.2011.01.009
Perdana, J., Fox, M. B., Siwei, C., Boom, R. M., and Schutyser, M. A. I. (2014). Interactions between formulation and spray drying conditions related to survival of Lactobacillus plantarum WCFS1. Food Res. Int. 56, 9–17. doi: 10.1016/j.foodres.2013.12.007
Picard, C., Fioramonti, J., Francois, A., Robinson, T., Neant, F., and Matuchansky, C. (2005). Review article: bifidobacteria as probiotic agents - physiological effects and clinical benefits. Aliment. Pharmacol. Ther. 22, 495–512. doi: 10.1111/j.1365-2036.2005.02615.x
Picot, A., and Lacroix, C. (2004). Encapsulation of bifidobacteria in whey protein-based microcapsules and survival in simulated gastrointestinal conditions and in yoghurt. Int. Dairy J. 14, 505–515. doi: 10.1016/j.idairyj.2003.10.008
Piuri, M., Sanchez-Rivas, C., and Ruzal, S. M. (2005). Cell wall modifications during osmotic stress in Lactobacillus casei. J. Appl. Microbiol. 98, 84–95. doi: 10.1111/j.1365-2672.2004.02428.x
Plé, C., Breton, J., Richoux, R., Nurdin, M., Deutsch, S.-M., Falentin, H., et al. (2016). Combining selected immunomodulatory Propionibacterium freudenreichii and Lactobacillus delbrueckii strains: reverse engineering development of an anti-inflammatory cheese. Mol. Nutr. Food Res. 60, 935–948. doi: 10.1002/mnfr.201500580
Prasad, J., McJarrow, P., and Gopal, P. (2003). Heat and osmotic stress responses of probiotic Lactobacillus rhamnosus HN001 (DR20) in relation to viability after drying. Appl. Environ. Microbiol. 69, 917–925. doi: 10.1128/AEM.69.2.917-925.2003
Rabah, H., Ménard, O., Gaucher, F., do Carmo, F. L. R., Dupont, D., and Jan, G. (2018). Cheese matrix protects the immunomodulatory surface protein SlpB of Propionibacterium freudenreichii during in vitro digestion. Food Res. Int. 106, 712–721. doi: 10.1016/j.foodres.2018.01.035
Rabah, H., Rosa do Carmo, F., and Jan, G. (2017). Dairy Propionibacteria: versatile probiotics. Microorganisms 5:E24. doi: 10.3390/microorganisms5020024
Racioppo, A., Corbo, M. R., Piccoli, C., Sinigaglia, M., Speranza, B., and Bevilacqua, A. (2017). Ultrasound attenuation of Lactobacilli and Bifidobacteria: effect on some technological and probiotic properties. Int. J. Food Microbiol. 243, 78–83. doi: 10.1016/j.ijfoodmicro.2016.12.011
Rios-Covian, D., Nogacka, A., Salazar, N., Hernández-Barranco, A. M., Cuesta, I., Gueimonde, M., et al. (2018). Bifidobacterium breve IPLA20005 affects in vitro the expression of hly and luxS genes, related to the virulence of Listeria monocytogenes Lm23. Can. J. Microbiol. 64, 215–221. doi: 10.1139/cjm-2017-0625
Roesser, M., and Müller, V. (2001). Osmoadaptation in bacteria and archaea: common principles and differences. Environ. Microbiol. 3, 743–754. doi: 10.1046/j.1462-2920.2001.00252.x
Rollan, G., Lorca, G. L., and Font de Valdez, G. (2003). Arginine catabolism and acid tolerance response in Lactobacillus reuteri isolated from sourdough. Food Microbiol. 20, 313–319. doi: 10.1016/S0740-0020(02)00139-9
Romeo, Y., Obis, D., Bouvier, J., Guillot, A., Fourçans, A., Bouvier, I., et al. (2003). Osmoregulation in Lactococcus lactis: BusR, a transcriptional repressor of the glycine betaine uptake system BusA. Mol. Microbiol. 47, 1135–1147. doi: 10.1046/j.1365-2958.2003.03362.x
Rong, J., Zheng, H., Liu, M., Hu, X., Wang, T., Zhang, X., et al. (2015). Probiotic and anti-inflammatory attributes of an isolate Lactobacillus helveticus NS8 from Mongolian fermented koumiss. BMC Microbiol. 15:196. doi: 10.1186/s12866-015-0525-2
Saarela, M., Virkajarvi, I., Alakomi, H.-L., Mattila-Sandholm, T., Vaari, A., Suomalainen, T., et al. (2005). Influence of fermentation time, cryoprotectant and neutralization of cell concentrate on freeze-drying survival, storage stability, and acid and bile exposure of Bifidobacterium animalis ssp. lactis cells produced without milk-based ingredients. J. Appl. Microbiol. 99, 1330–1339. doi: 10.1111/j.1365-2672.2005.02742.x
Saez-Lara, M. J., Gomez-Llorente, C., Plaza-Diaz, J., and Gil, A. (2015). The role of probiotic lactic acid bacteria and Bifidobacteria in the prevention and treatment of inflammatory bowel disease and other related diseases: a systematic review of randomized human clinical trials. BioMed. Res. Int. 2015, 1–15. doi: 10.1155/2015/505878
Sanchez, B., Champomier-Verges, M.-C., Collado, M. D. C., Anglade, P., Baraige, F., Sanz, Y., et al. (2007). Low-pH adaptation and the acid tolerance response of Bifidobacterium longum biotype longum. Appl. Environ. Microbiol. 73, 6450–6459. doi: 10.1128/AEM.00886-07
Sanchez, B., de los Reyes-Gavilan, C. G., and Margolles, A. (2006). The F 1 F 0 -ATPase of Bifidobacterium animalis is involved in bile tolerance. Environ. Microbiol. 8, 1825–1833. doi: 10.1111/j.1462-2920.2006.01067.x
Sánchez, B., Ruiz, L., Gueimonde, M., Ruas-Madiedo, P., and Margolles, A. (2013). Adaptation of bifidobacteria to the gastrointestinal tract and functional consequences. Pharmacol. Res. 69, 127–136. doi: 10.1016/j.phrs.2012.11.004
Santivarangkna, C., Kulozik, U., and Foerst, P. (2007). Alternative Drying processes for the industrial preservation of lactic acid starter cultures. Biotechnol. Prog. 23, 302–315. doi: 10.1021/bp060268f
Sarkar, A., and Mandal, S. (2016). Bifidobacteria—insight into clinical outcomes and mechanisms of its probiotic action. Microbiol. Res. 192, 159–171. doi: 10.1016/j.micres.2016.07.001
Savijoki, K., Suokko, A., Palva, A., Valmu, L., Kalkkinen, N., and Varmanen, P. (2005). Effect of heat-shock and bile salts on protein synthesis of Bifidobacterium longum revealed by [ 35 S]methionine labelling and two-dimensional gel electrophoresis. FEMS Microbiol. Lett. 248, 207–215. doi: 10.1016/j.femsle.2005.05.032
Schell, M. A., Karmirantzou, M., Snel, B., Vilanova, D., Berger, B., Pessi, G., et al. (2002). The genome sequence of Bifidobacterium longum reflects its adaptation to the human gastrointestinal tract. Proc. Natl. Acad. Sci. U.S.A. 99, 14422–14427. doi: 10.1073/pnas.212527599
Schoug,Å, Fischer, J., Heipieper, H. J., Schnürer, J., and Håkansson, S. (2008). Impact of fermentation pH and temperature on freeze-drying survival and membrane lipid composition of Lactobacillus coryniformis Si3. J. Ind. Microbiol. Biotechnol. 35, 175–181. doi: 10.1007/s10295-007-0281-x
Sengupta, R., Altermann, E., Anderson, R. C., McNabb, W. C., Moughan, P. J., and Roy, N. C. (2013). The role of cell surface architecture of Lactobacilli in host-microbe interactions in the gastrointestinal tract. Mediat. Inflamm. 2013, 1–16. doi: 10.1155/2013/237921
Sheehan, V. M., Sleator, R. D., Fitzgerald, G. F., and Hill, C. (2006). Heterologous expression of BetL, a betaine uptake system, enhances the stress tolerance of Lactobacillus salivarius UCC118. Appl. Environ. Microbiol. 72, 2170–2177. doi: 10.1128/AEM.72.3.2170-2177.2006
Sikorska, H., and Smoragiewicz, W. (2013). Role of probiotics in the prevention and treatment of meticillin-resistant Staphylococcus aureus infections. Int. J. Antimicrob. Agents 42, 475–481. doi: 10.1016/j.ijantimicag.2013.08.003
Silva, J., Carvalho, A. S., Ferreira, R., Vitorino, R., Amado, F., Domingues, P., et al. (2005). Effect of the pH of growth on the survival of Lactobacillus delbrueckii subsp. bulgaricus to stress conditions during spray-drying. J. Appl. Microbiol. 98, 775–782. doi: 10.1111/j.1365-2672.2004.02516.x
Simpson, P. J., Stanton, C., Fitzgerald, G. F., and Ross, R. P. (2005). Intrinsic tolerance of Bifidobacterium species to heat and oxygen and survival following spray drying and storage. J. Appl. Microbiol. 99, 493–501. doi: 10.1111/j.1365-2672.2005.02648.x
Sollohub, K., and Cal, K. (2010). Spray drying technique: II. current applications in pharmaceutical technology. J. Pharm. Sci. 99, 587–597. doi: 10.1002/jps.21963
Stack, H. M., Kearney, N., Stanton, C., Fitzgerald, G. F., and Ross, R. P. (2010). Association of beta-glucan endogenous production with increased stress tolerance of intestinal Lactobacilli. Appl. Environ. Microbiol. 76, 500–507. doi: 10.1128/AEM.01524-09
Steed, H., Macfarlane, G. T., Blackett, K. L., Bahrami, B., Reynolds, N., Walsh, S. V., et al. (2010). Clinical trial: the microbiological and immunological effects of synbiotic consumption - a randomized double-blind placebo-controlled study in active Crohn’s disease: clinical trial: synbiotic therapy in Crohn’s disease. Aliment. Pharmacol. Ther. 32, 872–883. doi: 10.1111/j.1365-2036.2010.04417.x
Streit, F., Delettre, J., Corrieu, G., and Béal, C. (2008). Acid adaptation of Lactobacillus delbrueckii subsp. bulgaricus induces physiological responses at membrane and cytosolic levels that improves cryotolerance. J. Appl. Microbiol. 105, 1071–1080. doi: 10.1111/j.1365-2672.2008.03848.x
Su, M. S., Schlicht, S., and Gänzle, M. G. (2011). Contribution of glutamate decarboxylase in Lactobacillus reuteri to acid resistance and persistence in sourdough fermentation. Microb. Cell Factories 10(Suppl. 1):S8. doi: 10.1186/1475-2859-10-S1-S8
Taranto, M. P., Fernandez Murga, M. L., Lorca, G., and Valdez, G. F. (2003). Bile salts and cholesterol induce changes in the lipid cell membrane of Lactobacillus reuteri. J. Appl. Microbiol. 95, 86–91. doi: 10.1046/j.1365-2672.2003.01962.x
Taverniti, V., Stuknyte, M., Minuzzo, M., Arioli, S., De Noni, I., Scabiosi, C., et al. (2013). S-layer protein mediates the stimulatory effect of Lactobacillus helveticus MIMLh5 on innate immunity. Appl. Environ. Microbiol. 79, 1221–1231. doi: 10.1128/AEM.03056-12
Teixeira, J. S., Seeras, A., Sanchez-Maldonado, A. F., Zhang, C., Su, M. S.-W., and Gänzle, M. G. (2014). Glutamine, glutamate, and arginine-based acid resistance in Lactobacillus reuteri. Food Microbiol. 42, 172–180. doi: 10.1016/j.fm.2014.03.015
Thierry, A., Deutsch, S.-M., Falentin, H., Dalmasso, M., Cousin, F. J., and Jan, G. (2011). New insights into physiology and metabolism of Propionibacterium freudenreichii. Int. J. Food Microbiol. 149, 19–27. doi: 10.1016/j.ijfoodmicro.2011.04.026
Torino, M. I., Taranto, M. P., Sesma, F., and de Valdez, G. F. (2001). Heterofermentative pattern and exopolysaccharide production by Lactobacillus helveticus ATCC 15807 in response to environmental pH. J. Appl. Microbiol. 91, 846–852. doi: 10.1046/j.1365-2672.2001.01450.x
Tsvetkova, N. M., Horvath, I., Torok, Z., Wolkers, W. F., Balogi, Z., Shigapova, N., et al. (2002). Small heat-shock proteins regulate membrane lipid polymorphism. Proc. Natl. Acad. Sci. U.S.A. 99, 13504–13509. doi: 10.1073/pnas.192468399
Valdés-Varela, L., Hernández-Barranco, A. M., Ruas-Madiedo, P., and Gueimonde, M. (2016). Effect of Bifidobacterium upon Clostridium difficile growth and toxicity when co-cultured in different prebiotic substrates. Front. Microbiol. 7:738. doi: 10.3389/fmicb.2016.00738
Ventura, M., Canchaya, C., Zink, R., Fitzgerald, G. F., and van Sinderen, D. (2004). Characterization of the groEL and groES Loci in Bifidobacterium breve UCC 2003: genetic, transcriptional, and phylogenetic analyses. Appl. Environ. Microbiol. 70, 6197–6209. doi: 10.1128/AEM.70.10.6197-6209.2004
Verma, R., Lee, C., Jeun, E.-J., Yi, J., Kim, K. S., Ghosh, A., et al. (2018). Cell surface polysaccharides of Bifidobacterium bifidum induce the generation of Foxp3 + regulatory T cells. Sci. Immunol. 3:eaat6975. doi: 10.1126/sciimmunol.aat6975
Vrancken, G., Rimaux, T., Wouters, D., Leroy, F., and De Vuyst, L. (2009). The arginine deiminase pathway of Lactobacillus fermentum IMDO 130101 responds to growth under stress conditions of both temperature and salt. Food Microbiol. 26, 720–727. doi: 10.1016/j.fm.2009.07.006
Walker, D. C., Girgis, H. S., and Klaenhammer, T. R. (1999). The groESL chaperone operon of Lactobacillus johnsonii. Appl. Environ. Microbiol. 65, 3033–3041.
Walsh, A. M., Crispie, F., Kilcawley, K., O’Sullivan, O., O’Sullivan, M. G., Claesson, M. J., et al. (2016). Microbial succession and flavor production in the fermented dairy beverage Kefir. MSystems 1, e00052-16. doi: 10.1128/mSystems.00052-16
Wang, X., Xiao, J., Jia, Y., Pan, Y., and Wang, Y. (2018). Lactobacillus kefiranofaciens, the sole dominant and stable bacterial species, exhibits distinct morphotypes upon colonization in Tibetan kefir grains. Heliyon 4:e00649. doi: 10.1016/j.heliyon.2018.e00649
Wang, Y., Delettre, J., Guillot, A., Corrieu, G., and Béal, C. (2005). Influence of cooling temperature and duration on cold adaptation of Lactobacillus acidophilus RD758. Cryobiology 50, 294–307. doi: 10.1016/j.cryobiol.2005.03.001
Yadav, A. K., Tyagi, A., Kaushik, J. K., Saklani, A. C., Grover, S., and Batish, V. K. (2013). Role of surface layer collagen binding protein from indigenous Lactobacillus plantarum 91 in adhesion and its anti-adhesion potential against gut pathogen. Microbiol. Res. 168, 639–645. doi: 10.1016/j.micres.2013.05.003
Yoo, D., Bagon, B. B., Valeriano, V. D. V., Oh, J. K., Kim, H., Cho, S., et al. (2017). Complete genome analysis of Lactobacillus fermentum SK152 from kimchi reveals genes associated with its antimicrobial activity. FEMS Microbiol. Lett. 364:fnx185. doi: 10.1093/femsle/fnx185
Young, G. R., Smith, D. L., Embleton, N. D., Berrington, J. E., Schwalbe, E. C., Cummings, S. P., et al. (2017). Reducing Viability bias in analysis of gut microbiota in preterm infants at risk of NEC and sepsis. Front. Cell. Infect. Microbiol. 7:237. doi: 10.3389/fcimb.2017.00237
Yu, Y., Zhu, X., Shen, Y., Yao, H., Wang, P., Ye, K., et al. (2015). Enhancing the vitamin B12 production and growth of Propionibacterium freudenreichii in tofu wastewater via a light-induced vitamin B12 riboswitch. Appl. Microbiol. Biotechnol. 99, 10481–10488. doi: 10.1007/s00253-015-6958-6
Zayed, G., and Roos, Y. H. (2004). Influence of trehalose and moisture content on survival of Lactobacillus salivarius subjected to freeze-drying and storage. Process Biochem. 39, 1081–1086. doi: 10.1016/S0032-9592(03)00222-X
Keywords: stress, probiotic, adaptation, drying, osmo regulation
Citation: Gaucher F, Bonnassie S, Rabah H, Marchand P, Blanc P, Jeantet R and Jan G (2019) Review: Adaptation of Beneficial Propionibacteria, Lactobacilli, and Bifidobacteria Improves Tolerance Toward Technological and Digestive Stresses. Front. Microbiol. 10:841. doi: 10.3389/fmicb.2019.00841
Received: 19 July 2018; Accepted: 02 April 2019;
Published: 24 April 2019.
Edited by:
Riadh Hammami, University of Ottawa, CanadaReviewed by:
Pasquale Russo, University of Foggia, ItalyM. Y. Sreenivasa, University of Mysore, India
Ehab Eissa Kheadr, Alexandria University, Egypt
Copyright © 2019 Gaucher, Bonnassie, Rabah, Marchand, Blanc, Jeantet and Jan. This is an open-access article distributed under the terms of the Creative Commons Attribution License (CC BY). The use, distribution or reproduction in other forums is permitted, provided the original author(s) and the copyright owner(s) are credited and that the original publication in this journal is cited, in accordance with accepted academic practice. No use, distribution or reproduction is permitted which does not comply with these terms.
*Correspondence: Gwénaël Jan, Z3dlbmFlbC5qYW5AaW5yYS5mcg==