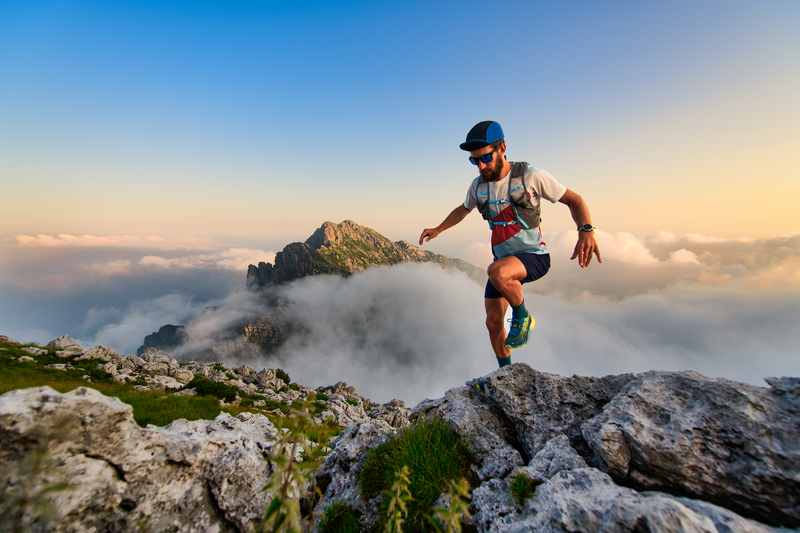
94% of researchers rate our articles as excellent or good
Learn more about the work of our research integrity team to safeguard the quality of each article we publish.
Find out more
ORIGINAL RESEARCH article
Front. Microbiol. , 04 April 2019
Sec. Extreme Microbiology
Volume 10 - 2019 | https://doi.org/10.3389/fmicb.2019.00603
This article is part of the Research Topic Microbial Life Under Stress: Biochemical, Genomic, Transcriptomic, Proteomic, Bioinformatics, Evolutionary Aspects and Biotechnological Applications of Poly-Extremophilic Bacteria View all 27 articles
This study was motivated by surprising gaps in the current knowledge of microbial inorganic carbon (Ci) uptake and assimilation at acidic pH values (pH < 3). Particularly striking is the limited understanding of the differences between Ci uptake mechanisms in acidic versus circumneutral environments where the Ci predominantly occurs either as a dissolved gas (CO2) or as bicarbonate (HCO3-), respectively. In order to gain initial traction on the problem, the relative abundance of transcripts encoding proteins involved in Ci uptake and assimilation was studied in the autotrophic, polyextreme acidophile Acidithiobacillus ferrooxidans whose optimum pH for growth is 2.5 using ferrous iron as an energy source, although they are able to grow at pH 5 when using sulfur as an energy source. The relative abundance of transcripts of five operons (cbb1-5) and one gene cluster (can-sulP) was monitored by RT-qPCR and, in selected cases, at the protein level by Western blotting, when cells were grown under different regimens of CO2 concentration in elemental sulfur. Of particular note was the absence of a classical bicarbonate uptake system in A. ferrooxidans. However, bioinformatic approaches predict that sulP, previously annotated as a sulfate transporter, is a novel type of bicarbonate transporter. A conceptual model of CO2 fixation was constructed from combined bioinformatic and experimental approaches that suggests strategies for providing ecological flexibility under changing concentrations of CO2 and provides a portal to elucidating Ci uptake and regulation in acidic conditions. The results could advance the understanding of industrial bioleaching processes to recover metals such as copper at acidic pH. In addition, they may also shed light on how chemolithoautotrophic acidophiles influence the nutrient and energy balance in naturally occurring low pH environments.
Acidithiobacillus ferrooxidans is a polyextremophile inhabiting very acidic (pH < 3) and often metal laden environments that belongs to the Acidithiobacillia class within the Proteobacteria (Williams and Kelly, 2013). It is an obligate chemolithoautotrophic, mesophilic microorganism that gains energy and reducing power by the aerobic oxidation of hydrogen, inorganic sulfur compounds, and ferrous iron (Bonnefoy and Holmes, 2012; Dopson and Johnson, 2012) and anaerobically via sulfur or formate oxidation coupled to reduction of ferric iron (Pronk et al., 1991; Hedrich and Johnson, 2013; Osorio et al., 2013).
A. ferrooxidans is one of the most abundant microorganisms found at ambient temperatures in industrial bioleaching heaps used for the recovery of, e.g., copper (Soto et al., 2013; Vera et al., 2013; Zhang et al., 2016). It also forms an integral part of natural occurring acidic ecosystems such as the Rio Tinto and deep subsurface in the Iberian pyrite belt (Amils et al., 2014), acidic springs, cave systems plus volcanic soils (reviewed in Johnson, 2012; Hedrich and Schippers, 2016), and acid mine drainage (AMD) (Chen et al., 2015; Teng et al., 2017). A. ferrooxidans is considered a model species for understanding genetic and metabolic functions reviewed in Cardenas et al., 2016) and survival mechanisms at extremely low pH (Chao et al., 2008) and reviewed in Slonczewski et al. (2009). It has also provided useful information for understanding how microorganisms can contribute to the nutrient and energy balance in bioleaching heaps (Valdes et al., 2008; Valdés et al., 2010).
The dominant source of available inorganic carbon (Ci) in circumneutral and slightly alkaline environments such as terrestrial fresh water and oceans is bicarbonate (HCO-3) with lower concentrations of dissolved CO2 (Mangan et al., 2016). The majority of models for prokaryotic Ci uptake and assimilation have been elucidated for organisms, such as cyanobacteria, that inhabit these environments (Burnap et al., 2015; Klanchui et al., 2017). Cyanobacteria fix carbon via the Calvin-Benson-Bassham (CBB) cycle and use a variety of carbon concentration mechanisms (CCMs) to take up CO2 or bicarbonate and provide CO2 to the carbon fixation enzyme, ribulose bisphosphate carboxylase-oxygenase (RubisCO). Five Ci uptake systems have been reported including three bicarbonate transporters: BCT1, SbtA, and BicA that vary in affinity and flux for bicarbonate and two intracellular CO2 “uptake” systems, that convert CO2, passively diffusing into the cell, into bicarbonate (Burnap et al., 2015; Klanchui et al., 2017). The transporters vary in affinity and flux for bicarbonate providing a selective advantage to organisms in environments with a wide dynamic range of HCO3- availability. For example, freshwater β-cyanobacteria that live at about pH 7 not only use the high affinity SbtA transporter and the low affinity, high flux BicA transporter but also the medium affinity BCT1, an inducible bicarbonate transporter under limited Ci conditions (Sandrini et al., 2014, 2015; Klanchui et al., 2017). Alkaline lake β-cyanobacteria tend to have just BicA and it is hypothesized that the high affinity SbtA is not necessary in environments rich in HCO3- (Klanchui et al., 2017).
In contrast, less is known about Ci uptake and assimilation in extremely acidic environments where the dominant source of Ci is the dissolved gas CO2 (Carroll and Mather, 1992; Cardenas et al., 2010; Valdés et al., 2010; Mangan et al., 2016; WikiVividly, 2018). A. ferrooxidans fixes carbon by the CBB cycle (Esparza et al., 2010). Bioinformatic analyses, EMSA assays, and complementation of mutants in the surrogate host Cupriavidus necator (formerly Ralstonia eutropha) have demonstrated the presence of four operons (cbb1-4) of CBB cycle genes in A. ferrooxidans that are involved in Ci uptake and assimilation. Operons cbb1-3 were shown experimentally to be regulated by CbbR, a LysR-family transcription regulator (Esparza et al., 2009, 2010, 2015). In the present study, RNA transcript and protein abundance profiles were determined for genes present in A. ferrooxidans operons cbb1-4 under different CO2 concentrations. In addition, a fifth cbb operon (cbb5) and a gene cluster predicted to encode a bicarbonate uptake transporter and a carbonic anhydrase were detected and were also evaluated for expression under different CO2 concentration regimes. Acquiring this knowledge is important considering the central roles that the CCM and CBB cycle genes play in the determination of CO2 fixation and biomass formation in extremely acidic environments.
A. ferrooxidans ATCC 23270 was cultured in 9K medium (Quatrini et al., 2007) adjusted to pH 3.5 with H2SO4 and containing 5 g/L elemental sulfur at 30°C under aerobic conditions (0.036% CO2). Increased concentrations of CO2 were obtained by sparging with a mixture of CO2 and air by changing the ratio of CO2 in the gas mixture. A. ferrooxidans cultures were grown to mid-log phase (Guacucano et al., 2000) as measured by cell counts using a Neubauer chamber. Cells were rapidly cooled on ice and then centrifuged at 800 × g for 5 min at 4°C to remove solid sulfur particles followed by cell capture by centrifugation at 8,000 × g for 10 min at 4°C. The cell pellet was re-suspended in ice-cold 9K salt solution for further washing. Total RNA was prepared immediately after cell harvesting.
Total RNA was isolated from A. ferrooxidans cells as described previously (Guacucano et al., 2000). The RNA preparations were treated with DNase I (Fermentas) before proceeding with the cDNA synthesis step. One microgram of total cellular RNA was used for each reaction. Real-time quantitative RT-PCR (RT-qPCR) was performed using RevertAid H Minus Reverse Transcriptase (Fermentas). The sequences of the qPCR primers for genes involved in CO2 assimilation are provided in Table 1. Control reactions performed using RNA but lacking reverse transcriptase to assess genomic DNA contamination did not produce any bands after gel electrophoresis (data not shown). RT-qPCR assays were carried out in a 25 μL PCR mixture consisting of 12.5 μl 2 × SYBR Green Supermix (Bio-Rad). The RT- qPCR was performed on iCycler iQ Real-time PCR detection system (Bio-Rad Laboratories, United States) with IQ SYBR green supermix (Bio-Rad) as described previously (Liu et al., 2011). Quantification of the target gene expression was performed using iCycler iQ5TM software using a normalized expression analysis method as described by the manufacturer. Relative quantifications were performed from duplicate biological replicates using expression of recA as a control as described previously (Esparza et al., 2015). PCR primers were designed as described (Thornton and Basu, 2011) and the results were analyzed using IQ Bio-Rad equipment software RT-qPCR and excel software. Statistical variance was analyzed using the Tukey test (Tukey, 1949) and ANOVA (Kotz et al., 2014). The P-value was 0.05.
Antibodies against CbbR were obtained as previously described (Esparza et al., 2010). Antibodies against phosphoribulokinase (CbbP) and RubisCO small sub-unit (CbbS) were provided by Dr. Botho Bowien (Institut für Mikrobiologie und Genetik, Georg-August-Universität Göttingen, Germany). The Western Blotting was performed as previously described (Quatrini et al., 2005) using the Supersignal West Pico chemiluminescent substrate (Pierce).
Experimentally validated and predicted SulP protein sequences were obtained from multiple Bacteria including cyanobacteria (Price et al., 2004), sulfate-reducing microorganisms (Marietou et al., 2018), and other microorganisms (Moraes and Reithmeier, 2012). These included the experimentally validated sulfate transporter Rv1739c from Mycobacterium tuberculosis H37Rv (Marietou et al., 2018) and experimentally validated bicarbonate transporters YchM from Escherichia coli APEC O1 and E. coli O157:H7 str. Sakai (Moraes and Reithmeier, 2012), Rv3273 from M. tuberculosis H37Rv (Felce and Saier, 2004), and BicA from Synechococcus sp. PCC 7002 (Price et al., 2004). Additional SulP protein sequences were added to the analysis (Ward et al., 2004; Boden et al., 2011; Huntley et al., 2011; Svenning et al., 2011; Scott et al., 2019). A multiple sequence alignment was constructed using MAFFT with accurate option L-INS-i as alignment tools (Katoh and Standley, 2013; Nakamura et al., 2018) and ClustalW alignment program (Larkin et al., 2007). The alignments were imported into GBLOCKS (Castresana, 2000) and phylogenetically uninformative or noisy, unreliable sections of the alignments were masked (Rajan, 2013). Resultant alignments were used to construct a maximum likelihood, unrooted, phylogenetic tree with IQtree (Nguyen et al., 2015) using 1000 replicates. Trees were inferred using the LG + I + G model with empirically determined amino acid frequencies according to the ProtTest (Darriba et al., 2011) tool. Trees were visualized and annotated in Figtree1.
Transmembrane regions in protein sequences were predicted with TMHMM (Sonnhammer et al., 1998; Krogh et al., 2001) and TMPRED (Hofmann, 1993). Subcellular localization for protein sequences was predicted using PSORTb (Yu et al., 2010). Gene functional associations were predicted using String (Szklarczyk et al., 2017). RNA Secondary structure was predicted using Mfold (Zuker, 2003).
A list of A. ferrooxidans genes used in this study, their predicted functions and GenBank locus tags is provided in Table 2. The table has been updated from Esparza et al. (2010).
Table 2. Genes, predicted functions, and GenBank locus tags for the A. ferrooxidans CBB cycle and CCM genes used in this study.
In order to evaluate the effect of CO2 on the growth of A. ferrooxidans, cells were cultivated in 9K medium, pH 3.5 and containing 5 g/L elemental sulfur at 30°C (Quatrini et al., 2007) with increasing concentrations of CO2 from 0.036% (air) to 20%. Maximum growth rate occurred in 2.5% CO2 with decreasing growth rates in 5, 0.036, 10 and 20% CO2, respectively (Figure 1). However, maximum cell concentration (cells/mL) was unaffected by increasing CO2.
Figure 1. Growth curves of Acidithiobacillus ferrooxidans grown in 9K medium supplemented with 5 g/L elemental sulfur at 30°C in increasing concentrations of CO2 from 0.036% (vol/vol) (air) to 20% (vol/vol). Ranges are shown for duplicate measurements. μ = growth rate (hr-1).
Having established that CO2 concentration impacts cell growth rate, we wished to examine the effect of CO2 concentration on the expression of genes involved in the CBB and CCM pathways. Levels of RNA transcripts were assayed by RT-qPCR for one or more representative genes of each of the five cbb operons isolated from cells grown under different regimens of CO2 concentration from 0.036% (natural CO2 concentration in air) up to 20% (Figure 2). Transcript numbers of each tested gene are reported with respect to the level of RNA during growth at 0.036% CO2 normalized to one. The relative levels of transcripts of cbbR encoding the CbbR transcriptional regulator increased 3.4 ± 0.6-fold at a concentration of 10% CO2. A further increase to 20% CO2 did not result in any additional changes in RNA expression (Figure 2). In contrast, levels of RNA expression decreased with increasing CO2 concentrations for genes in the cbb1 operon including RubisCO Form IAc, associated carboxysome genes (including can1 encoding a carboxysome-associated 𝜀-type carbonic anhydrase), and the RubisCO activase genes cbbQ1 plus cbbO1. The expression of RNA from the cbb2 operon, encoding RubisCO Form IAq and the RubisCO activase genes cbbQ2 and cbbO2, also decreased with increasing CO2 but the decrease was more abrupt than that for RubisCO Form IAc suggesting that its expression was more sensitive to increasing CO2. RNA transcripts for hyp3 (unknown function) and cbbG (encoding glyceraldehyde-3-phosphate dehydrogenase) in the cbb3 operon were increased 30- and 20-fold, respectively when the CO2 concentration was raised from air to 2.5% CO2 followed by a subsequent decrease in transcript numbers in 5, 10, and 20% CO2, although transcripts in 20% CO2 were still higher than in air. The operon cbb3 encodes enzymes in the Calvin cycle together with phosphoglycolate phosphatase (cbbZ) that is involved in the detoxification of 2-phosphoglycolate produced by the reaction of RubisCO with oxygen (Ogren and Bowes, 1971) and part of the Trp operon that is involved in pyruvate formation and tryptophan biosynthesis. Transcripts for cbbP encoding phosphoribulokinase (PRK) in the cbb4 operon increased about 70-fold when cells were grown in 2.5% CO2 with a further increase in 5% CO2 to approximately 100-fold. Although the fold difference increased further in 10 and 20% CO2, the increases were not statistically significant using the Tukey test (Tukey, 1949) and ANOVA (Kotz et al., 2014). The P-value was 0.05. PRK catalyzes the ATP-dependent phosphorylation of ribulose 5-phosphate (RuP) into ribulose 1,5-bisphosphate (RuBP) which is the substrate for RubisCO.
Figure 2. RNA transcript levels for genes and corresponding cbb operons involved in A. ferrooxidans CO2 assimilation under different CO2 concentrations relative to the transcript levels in air (0.036% CO2) normalized to one. mRNA abundance was determined by RT-qPCR (n = 4) for the following genes: cbbR encoding the transcriptional regulator CbbR (cbb1); cbbS1 small subunit of RubisCO Form 1Ac (cbb1), cbbS2 small subunit of RubisCO form 1Aq (cbb2); hyp (hypothetical) and cbbG glyceraldehyde-3-phosphate dehydrogenase (cbb3); cbbP phosphoribulokinase (cbb4) and cbbM RubisCO form II (cbb5). The genes assayed in each operon are highlighted in orange. The cbbR responsive promotors are indicated with a DNA symbol and a cartoon of the two subunits of CbbR1 (gray ellipses). A full list of genes in the operons is provide in Table 1.
RNA transcript abundance for cbbM (cbb operon 5), encoding RubisCO form II increased about two-fold in 2% CO2 with further increases to about threefold in 5–20% CO2.
RNA transcript abundance, as measured by RT-qPCR, does not always correspond to the level of the corresponding protein (Rocca et al., 2015). In order to evaluate whether protein concentration exhibited similar trends as the RNA levels, proteins encoded by selected cbb operon genes were assayed by Western blotting when cells were grown in increasing concentrations of CO2 (Figure 3). CbbR concentrations increased with increasing CO2 concentrations, mimicking transcript changes. The levels of CbbS1 and/or CbbS2 (the antibody cannot distinguish between the two forms of CbbS) decreased when cells were grown in increasing concentrations of CO2. Levels of CbbP increased until a concentration of 10% CO2 was reached with a subsequent slight decrease with 20% CO2. These data matched the changes in levels of RNA abundance in all three cases. However, absolute levels of protein abundance do not match transcript abundance, perhaps because of additional levels of post-transcriptional and post-translational regulation of the proteins and because Western-blotting is at best semi-quantitative (Gassmann et al., 2009).
Figure 3. (A) Representative Western blots (of duplicates) of CbbR (cbb operon 1), CbbS1/S2 (the antibody used in the assay cannot distinguish between the two proteins) (cbb operon 1 and/or 2), and CbbP (cbb operon 4). (B) Quantification of the intensity of the Western blots relative to expression in 0.036% CO2 normalized to 1. Cells were grown in different concentrations of CO2 as indicated above the Western blots in (A) and in the scale bar in (B). Error bars depict ranges of duplicate values.
High affinity NDH-I3 and low affinity NDH-I4 CO2 uptake systems have been described in cyanobacteria (reviewed in Klanchui et al., 2017). However, bioinformatic examination of the genome of A. ferrooxidans failed to reveal gene candidates for the critical cupA in the NDH-I3 system or cupB in the NDH-I4 system, suggesting that A. ferrooxidans does not use these systems. Instead, we propose that CO2 passively diffuses into A. ferrooxidans, as has been shown in other organisms (Gutknecht et al., 1977).
In order to investigate the possibility that SulP in A. ferrooxidans encodes a bicarbonate transporter, a detailed bioinformatic examination of the gene/protein was undertaken. SulP is predicted to be an inner membrane protein with eleven transmembrane regions with a similar topology to the experimentally verified BicA from M. tuberculosis H37Rv (Supplementary Figure S1). Particularly significant is that sulP is juxtaposed to can2 in A. ferrooxidans. Can2 is strongly predicted to encode a cytoplasmic carbonic anhydrase of the β-class clade B (Valdes et al., 2008). Carbonic anhydrases (EC 4.2.1.1) are metallo-anhydrases that catalyze the reversible hydration of CO2 to HCO3- (Frost and McKenna, 2014). The juxtaposition of sulP and can2 suggests a functional relationship involving the uptake (or export) of HCO3- by SulP and the interconversion of HCO3- and CO2 by Can2 inside the cell. This hypothesis is strongly supported by the discovery, using the String database (Szklarczyk et al., 2017), of multiple examples of conserved microsynteny between sulP and can including gene fusions in many different organisms (Supplementary Figure S2).
Motivated by the mounting evidence that SulP is a bicarbonate transporter, the functional relationship between SulP and experimentally validated sulfate or bicarbonate transporters was explored using phylogenomic approaches. SulP sequences chosen for comparison included an experimentally validated sulfate transporter from M. tuberculosis H37Rv and experimentally validated bicarbonate transporters from E. coli APEC O1, E. coli O157:H7 str. Sakai, M. tuberculosis H37Rv, and Synechococcus sp. PCC 7002 as specified in the Section “Materials and Methods.” Additional SulP protein sequences with predicted sulfate or bicarbonate transport functions were obtained from multiple phylogenetically distinct Bacteria and added to the analysis. A multiple sequence alignment was constructed using MAFFT and ClustalW alignment program. Resultant alignments were used to construct a maximum likelihood, unrooted phylogenetic tree that was visualized and annotated in Figtree (Figure 4).
Figure 4. Maximum likelihood unrooted phylogenetic tree of SulP annotated as a sulfate (orange background), bicarbonate transporter (gray background) or of unknown function (light yellow background). (A–E) Refer to five phylogenetically distinct clades of sulfate or bicarbonate transporters. Bootstrap values between 50–60% (from 1000 resampling) are shown by white circles and bootstrap values >65% (1000 resampling) are represented by black circles. Arrows indicate genes where green = sulP, purple = can and blue = STAS domain. Abbreviations for species names: A. caldus, Acidithiobacillus caldus; A. thiooxidans, Acidithiobacillus thiooxidans; A. ferrivorans, Acidithiobacillus ferrivorans; A. ferrooxidans, Acidithiobacillus ferrooxidans; C. watsonii, Crocosphaera watsonii; D. alkenivorans AK-01, Desulfatibacillum alkenivorans AK-01; D. autotrophicum HRM2, Desulfobacterium autotrophicum HRM2; D. orientis DSM 765, Desulfobacterium orientis DSM 765; D. reducens MI-1, Desulfobacterium reducens MI-1; D. vulgaris DP4, Desulfovibrio vulgaris DP4; E. coli APEC O1, Escherichia coli APEC O1; E. coli O157:H7 str. Sakai, Escherichia coli O157:H7 str. Sakai; H. thermophilus JR2, Hydrogenovibrio thermophilus JR2; L. interrogans Lai, Leptospira interrogans Lai; M. capsulatus, Methylococcus capsulatus; M. methanica, Methylomonas methanica; M. tuberculosis H37Rv, Mycobacterium tuberculosis H37Rv; M. tundripaludum, Methylobacter tundripaludum; Nostoc punctiforme, Nostoc punctiforme PCC 73102; P. aeruginosa PAO1, Pseudomonas aeruginosa PAO1; P. marinus, Prochlorococcus marinus; S. aurantiaca, Stigmatella aurantiaca; Sy. sp. PCC 6803, Synechocystis sp. PCC 6803 substr. PCC-P; Syn. elongatus PCC 7942, Synechococcus elongatus PCC 7942; Syn. PCC 7002, Synechococcus sp. PCC 7002; Syn. WH 8102, Synechococcus sp. WH 8102; T. elongatus BP-1, Thermosynechococcus elongatus BP-1; T. erythraeum, Trichodesmium erythraeum; T. tepidarius, Thermithiobacillus tepidarius DSM 3134; T. variabilis ATCC 29413, Trichormus variabilis ATCC 29413. The scale bar represents the number of substitutions per site. (Figure with bootstrap values, gene names, accession numbers and references can be found in Supplementary Figure S3).
Five phylogenetically distinct clades were detected (labeled A to E in Figure 4). In clade A, sequences cluster with the experimentally verified sulfate transporter Rv1739c from M. tuberculosis H37Rv (Marietou et al., 2018). Clade B contains no sequences with experimentally validated functions and remains of unknown function. Clade C is associated with the experimentally validated bicarbonate transporter Rv3273 from M. tuberculosis H37Rv (Felce and Saier, 2004). SulP from A. ferrooxidans ATCC 23270 clusters in this clade, strongly supporting the contention that it is a bicarbonate and not a sulfate transporter. SulP sequences from A. ferrooxidans ATCC 53993 and Acidithiobacillus ferrivorans SS3 plus CF27 also cluster in this clade suggesting that they are also bicarbonate transporters. Microsynteny examination of clade C indicated that sulP is always juxtaposed to can2 and in some instances they are fused, providing additional support for the idea that the two genes are functionally related. Bicarbonate transporters in other systems use either Na+ or H+ as the counter-ion for the importation of HCO3- (Saier et al., 2016). The counter-ion used by A. ferrooxidans remains unknown. Clade D includes sequences that cluster with the experimentally verified bicarbonate transporter BicA of Synechococcus PCC 7002 (Price et al., 2004). In clade E, sequences cluster with experimentally verified bicarbonate transporters YchM of E. coli APEC O1 and E. coli O157:H7 str. Sakai (Moraes and Reithmeier, 2012). In contrast to clade C, SulP in all other clades is not associated with Can2 rather it is fused to a STAS domain (sulfate transporter/anti-sigma factor antagonist) that is thought to be involved in regulation or targeting (Shibagaki and Grossman, 2006).
Given the multiple lines of bioinformatic evidence suggesting that sulP encodes a bicarbonate transporter and that it is functionally related to the adjacent can2 encoding carbonic anhydrase, transcript abundance of can2 was assayed by RT-qPCR when cells were grown in increasing concentrations of CO2 (Figure 5). RNA transcript abundance in 2% CO2 decreased to less than one-half that determined in 0.036% CO2, with further decreases to 0.1% in 20% CO2. The can2-sulP gene cluster has not been experimentally demonstrated to be an operon, but their phylogenetically conserved juxtaposition and close proximately separated by only nine nucleotides suggest that they are co-transcribed.
Figure 5. RNA transcript abundance (assayed by RT-qPCR) of can2 when cells were grown in different concentrations of CO2 from 0.036% CO2 (air) to 20% CO2. Orange arrow indicates the gene assayed for transcript abundance.
This study advances our understanding of the mechanisms employed by A. ferrooxidans to take-up and concentrate Ci and the incorporation of CO2 into fixed carbon via the CBB cycle. A model is presented that builds upon prior investigations (Appia-Ayme et al., 2006; Esparza et al., 2009, 2010, 2015) and provides a preliminary framework to understand carbon fixation at extremely acidic pH under different regimes of CO2 concentration (Figure 6). Though much remains to validate aspects of the model, this work is an important step toward identifying the components, pathways, and regulation of carbon sequestration in A. ferrooxidans. It generates a more accurate and perceptive starting point to characterize the genetics and physiology of carbon sequestration in other extreme acidophiles. In addition, the model reveals a potentially flexible metabolic repertoire mediating carbon sequestration in different environments that can guide future research. Finally, it serves as a portal for deducing aspects of the CCM and CBB pathways in metagenomes from low pH environments (Guo et al., 2013).
Figure 6. Model of Ci uptake and assimilation in A. ferrooxidans. Predicted genes and complexes involved in Ci uptake and assimilation are color coded where purple = genes and complexes with fewer RNA transcripts in cells grown at 2.5% CO2 versus air (O.036% CO2) and yellow = genes with an increase in transcript abundance in cells grown at 2.5% CO2 versus air (O.036% CO2). 1,3-BP glycerate, 1,3-bisphosphoglycerate; Fructose-1,6-BP, fructose-1,6-bisphosphate; Fructose-6-P, fructose-6-phosphate; G-3-P, glyceraldehyde-3-phosphate; 3-PGA, 3-phosphoglycerate; Ribulose-1,5-BP, ribulose-1,5-bisphosphate; Ribulose-5-P, ribulose-5-phosphate; Erythrose-4-P, Erythrose-4-phosphate; Sedoheptulose-7-P, sedoheptulose-7-phosphate; Sedoheptulose-1,7-BP, sedoheptulose-1,7-bisphosphate; Xylose-5-P, xylose-5-phosphate; PEP, phosphoenol pyruvate; DHAP, dihydroxyacetone phosphate.
The maximum rate of A. ferrooxidans growth in media containing elemental S as an energy source was obtained in the presence of 2.5% CO2 (Figure 1). This tendency can be explained, at least partially, by the expression of the five cbb operons as determined by changes in RNA transcript abundance (Figure 2) supported by protein abundance profiling (Figure 3). Two representative genes of the cbb3 operon, hyp3 and cbbG, obtain maximum transcript abundance in 2.5% CO2 that subsequently diminishes as the CO2 concentration is increased to 20% CO2. These genes are part of an operon coding for enzymes that pass the carbon from 3-PGA (3-phosphoglycerate), generated by RubisCO, through the pentose phosphate and glycolysis pathways to pyruvate and pathways for glycogen metabolism. The cbb3 operon also encodes cbbZ involved in 2-phosphoglycolate detoxification, a by-product of the reaction of RubisCO with O2 (Esparza et al., 2009, 2010, 2015). Assuming that a higher level of transcript abundance results in a concomitant increase in the levels of their respective encoded enzymes, growth in 2.5% CO2 could result in an increase in fixed carbon compounds and increased protection from O2 damage compared to growth in air. This, in turn, could contribute to more rapid growth in 2.5% CO2.
In order to achieve this increase in growth, the CBB cycle needs to provide more 3-PGA as a starting material to feed into the sugar transformation pathway. 3-PGA is the primary product of RubisCO and in 2.5% CO2 there is an increase in the abundance of transcripts for RubisCO form II encoded by cbbM of the cbb5 operon (Figure 2) that could account in part for an increase in 3-PGA production. In other organisms, Form II RubisCO has poor affinity for CO2 and a low discrimination against O2 as an alternative substrate suggesting that the enzyme is adapted to functioning in low-O2 and high-CO2 environments (Dobrinski et al., 2005; Badger and Bek, 2008). The observed increase in transcript abundance for RubisCO Form II in higher concentrations of CO2 (Figure 2) is consistent with this view.
One of the products of the enzymes encoded by the cbb3 operon is ribulose-5-P that is a precursor to ribulose-1,5-P, the substrate for RubisCO (Figure 6). The conversion of ribulose-5-P to ribulose-1,5-P is carried out by phosphoribulokinase (PRK) encoded by cbbP of the cbb4 operon. PRK catalyzes the ATP-dependent phosphorylation of ribulose 5-phosphate (RuP) into ribulose 1,5-bisphosphate (RuBP) that are both intermediates in the CBB Cycle. Together with RubisCO, PRK is unique to this cycle. There is a 65-fold increase in transcript abundance for cbbP in 2.5% CO2 compared to air (Figure 2) that could be responsible for an increase in ribulose-1,5-P. RNA transcript abundance for cbbP continues to rise in 10% CO2 but this is not accompanied by a concomitant increase in growth rate (Figure 1). Clearly, there are other factors limiting the growth rate at concentrations of CO2 above 2.5%. One possible explanation is the observed decrease in transcript abundance of genes in the cbb3 operon (encoding many genes involved in sugar interconversions) in 5–20% CO2 (Figure 2) that would potentially limit growth by diminished enzyme availability for various sugar conversions (Figure 6).
In summary, the model suggests A. ferrooxidans grows fastest in 2.5% CO2 due to an increase in transcript abundance for sugar transformation pathway genes, transcripts for cbbP that feeds RBP into the CBB cycle, and transcripts for genes encoding RubisCO Form II that is postulated to be the RubisCO used at higher CO2 concentrations.
Another important consideration is how A. ferrooxidans CCM genes involved in the uptake and concentration of Ci respond to changes in CO2 concentration. A. ferrooxidans has evolved efficient CCMs to transport and accumulate Ci. First, it encodes the formation of α-carboxysomes, bacterial micro-compartments that provide elevated concentrations of CO2 to the main CO2-fixing enzyme RubisCO and reduce its reaction with oxygen (reviewed in Rae et al., 2013). A. ferrooxidans has multiple forms of RubisCO including two copies of Form I and one copy of Form II (Heinhorst et al., 2002; Levican et al., 2008). Using protein similarity analysis, we now predict the two forms of Form I RubisCO as sub-types IAc and IAq that consist of the large and small subunits of RubisCO as has been observed in other organisms (Badger and Bek, 2008). RubisCO Form II consists only of a large subunit with little sequence or structural similarity with the large subunit of forms IAc and IAq (Tabita et al., 2008; Bohnke and Perner, 2017). Genes encoding RubisCO Form IAc are encoded in the cbb1 operon, co-occur with carboxysome formation genes, and is probably encapsulated within the carboxysome as has been found in other organisms (Tabita et al., 2008). In addition, csoS3 is also present in the cbb1 operon and encodes a β-type carbonic anhydrase (Can1) (Sawaya et al., 2006). CsoS3 is located in the carboxysome shell and is responsible for the conversion of bicarbonate to CO2 and is an important contributor to CCM (So et al., 2004). Thus, cbb1 encodes the major components of the CCM carboxysome that encapsulates RubisCO in A. ferrooxidans.
Under conditions of low CO2 concentrations, carboxysome formation genes are upregulated in other microorganisms (Orus et al., 2001). This has been confirmed in A. ferrooxidans where increased transcript abundance was observed for cbb1 operon genes under anaerobic conditions with low CO2 concentrations (Osorio et al., 2013). On the other hand, genes encoding RubisCO Form IAq, located in the cbb2 operon, are not closely linked in the genome to carboxysome genes. In addition, despite considerable sequence similarity of both large and small subunits of Form IAq and Form IAc, a major difference is the presence in many bacteria including A. ferrooxidans of a six amino acid insertion in the small subunit of Form IAq not found in Form IAc (Supplementary Figure S4). A crystal structure of the small subunit of Form IAc RubisCO from Halothiobacillus neapolitanus [1SVD; the structure of H. neapolitanus RubisCO, (Kerfield, C. A. et al., 2005, unpublished)] provides evidence that this insertion impedes its interaction with carboxysome proteins (Badger and Bek, 2008), suggesting that Form IAq is not associated with carboxysomes in the cell. An examination of its kinetic properties suggests that Form IAq is adapted to environments with medium to high CO2 concentrations with oxygen present whereas Form IAc is more adapted to low CO2 and low to high O2 environments (Badger and Bek, 2008). RNA transcript abundance of both RubisCO Form IAc and Form IAq indicate that their abundance diminishes in CO2 concentrations above that of air. However, as both their expressions are low it is not possible to discern if there are statistically significant differences in the rate of decrease of transcript abundance between the two RubisCO Forms as CO2 concentrations are increased.
In summary, the A. ferrooxidans genome encodes α-carboxysomes that include β-type carbonic anhydrases and a type IAc RubisCO. The genes for these functions are present in operon cbb1 under the control of CbbR. RNA transcript abundance for cbb1 decreases with increasing CO2 concentrations. It is most likely that the carboxysomes and associated functions are used as a CCM mechanism in low CO2 concentrations. In contrast, RubisCO Type IAq (cbb2 operon) and RubisCO Type II (cbb5 operon) are probably not encapsulated in carboxysomes. RNA transcript abundance for RubisCO Type II increases in higher CO2 concentrations and may be the principle RubisCO used at higher concentrations of CO2 (or lower O2 concentrations). The role of RubisCO Type IAq is not clear but may represent a form that is used at slightly higher concentrations of CO2 or during rapid fluxes of CO2 concentration.
In addition to the proposed involvement of a carboxysome β-type carbonic anhydrase, a second potential CCM mechanism is the presence of a cytoplasmic-located β-carbonic anhydrase (Can2) that is genetically linked to a predicted bicarbonate transporter SulP. Carbonic anhydrases catalyze the proton-mediated reversible hydration of CO2 to HCO3- (Smith and Ferry, 2000) equilibrating the reaction between CO2, bicarbonate, and protons and play important roles in ion transport, acid-base regulation, gas exchange, and CO2 fixation in many organisms (Lotlikar et al., 2013; Aggarwal and McKenna, 2015). Although the function of Can2 in A. ferrooxidans remains to be experimentally validated, the model suggests that it is involved in the reversible hydration of CO2 (that has entered the cell by diffusion) to HCO3- as found in other organisms (Smith and Ferry, 2000). The genomic co-localized of can2 with the predicted bicarbonate transporter sulP suggests that they work together, perhaps in pH regulation as has been found in other organisms (Lotlikar et al., 2013; Aggarwal and McKenna, 2015). In this model, the importation of bicarbonate into the cell by SulP would be accompanied by the expulsion of protons. Subsequently, Can2 could convert the bicarbonate to CO2 accompanied by the conversion of cellular protons to water and the diffusion of the CO2 outside the cell as shown in Figure 6. Thus, protons are both exported and consumed and this may be an important mechanism for pH regulation in extremely acidic conditions. Alternatively, and not mutually exclusive, there is the possibility that Can2 works in the reverse direction and converts CO2 to bicarbonate that is subsequently taken into the carboxysome by Can1. This could improve the efficiency of carbon fixation under limiting conditions of external CO2 as has been observed in the facilitation of growth of other microbes at low partial pressures of CO2 (Kusian et al., 2002; Merlin et al., 2003; Mitsuhashi et al., 2004; Burghout et al., 2010). Low partial pressures of CO2 in bioleaching heaps has been observed due to the decreased solubility of CO2 in low pH especially when the temperature of the heap rises (lowering further the solubility of CO2), resulting from chemical and biochemical exothermic reactions including the conversion of pyrite to oxidized sulfur compounds (Valdés et al., 2010). If Can2 is involved in improving uptake of CO2 at low partial pressures of CO2, then it might explain why can2 exhibits a decrease in transcript abundance in increasing concentrations of CO2 (Figure 2).
In summary, A. ferrooxidans is predicted to have a second carbonic anhydrase, encoded by can2, located in the cytoplasm that functions in the reversible hydration of CO2. Juxtaposed is a gene (sulP) predicted to be membrane associated bicarbonate transporter. It is predicted that sulP/can2 constitute an operon. The abundance of transcripts for sulP/can2 decreases with increasing CO2 concentrations. It is hypothesized that SulP/Can2 function as a bicarbonate uptake system but they may also serve as an intracellular proton concentration homeostatic mechanism.
Regulation of Ci uptake and assimilation is very complex and is dependent on transcriptional regulators that act in concert with small molecular effectors that are well known metabolites. In addition, it has recently been discovered that numerous small RNA molecules act as antisense regulators (Burnap et al., 2015). Although there are many studies of the regulation of Ci uptake and assimilation in autotrophs, principally in photoautotrophs (Kusian and Bowien, 1997), there have been only limited insights into their regulation in extremely acidophilic chemolithoautotrophs. It was suggested in Esparza et al. (2010) that the regulation of cbb operons 1–4 of A. ferrooxidans involved the action of the master regulator CbbR, as has been observed in many microorganisms (Badger and Bek, 2008). The evidence included: (i) the presence of a CbbR binding site upstream of cbbR leading to autoregulation of cbbR (Esparza et al., 2015); (ii) the presence of CbbR binding sites upstream of operons cbb1-3 (Esparza et al., 2010); and (iii) the activity of A. ferrooxidans cbbR promoters when cloned into the surrogate host C. necator (formerly R. eutropha) (Esparza et al., 2015), including the detection of promoter activity upstream of cbb4 even in the absence of an experimentally validated CbbR binding site in this operon. The observed transcript profiles of operons cbb1-4 can be explained on the basis of the activity of CbbR. Increased CbbR down-regulates the expression of the cbb2 operon and up-regulates the cbb3 and cbb4 operons (Figure 4). That CbbR can act as both a positive and negative regulator has been observed in other organisms (Viale et al., 1991). However, what controls the up-regulation of CbbR in A. ferrooxidans in response to increasing CO2 concentrations is unknown. One possibility is that it involves the interaction of the regulator RegA, that responds to the redox state of the cell, with CbbR (Dangel and Tabita, 2015). Alternatively, it could involve the binding of possible effectors such as ATP, NADPH, RuBP, and fructose-1,6-bisphosphate to CbbR, many of which are metabolites of the Cbb cycle involved in feedback regulation (Joshi et al., 2012) as discussed further below. An important observation is the increase in transcripts of RubisCO Form II in high CO2 concentrations (Figure 2). In other organisms it has been shown that RubisCO Form II is controlled by the transcriptional regulator CbbRm (Bohnke and Perner, 2017) and CbbRm plus RubisCO Form II expression levels increase at CO2 concentrations above 2%. The molecular mechanisms underlying the regulation of RubisCO Form II are only beginning to be understood (Dubbs et al., 2004; Toyoda et al., 2005; Tsai et al., 2015). It has been suggested that RubisCO Form II evolved at least 2.7 billion years ago, when atmospheric CO2 levels were one to three orders of magnitude higher than today (Raven, 1991; Rye et al., 1995; Tortell, 2000; Kaufman and Xiao, 2003; Dobrinski et al., 2005; Griffiths et al., 2017). At that time, CCMs were perhaps not required and that is consistent with the observation that RubisCO Form II in A. ferrooxidans is not associated with the CCM carboxysome formation genes.
Regulation of expression of the CCM and CBB cycle genes in other organisms is also known to be mediated by small effector molecules (Dubbs et al., 2004; Tamoi et al., 2005). These include CO2 (Shimizu et al., 2015), α-ketoglutarate and the oxidized form of nicotinamide adenine dinucleotide phosphate (NADP+) (Daley et al., 2012), ATP, fructose 1,6-bisphosphate, and NADPH (Joshi et al., 2012) and several compounds of the CBB reductive pentose phosphate pathway several of which are encoded by the operon cbb3 of A. ferrooxidans (Figures 3, 6) (Dubbs et al., 2004). The role of these effectors has not been tested in A. ferrooxidans and it will be a considerable challenge to elucidate the manifold dependencies and interconnections between the diverse cellular processes that together facilitate the regulation of the CCM and CBB pathways in this organism. The use of the surrogate host C. necator provides an opportunity to experimentally test the role of metabolic effectors in A. ferrooxidans (Esparza et al., 2015).
In summary, CbbR has been shown to regulate the expression of cbb operons 1-4. Its increase in expression in higher CO2 concentrations is consistent with previous observations that it can serve as both a negative regulator (cbb operons 1 and 2) and a positive regulator (cbb4 operon). In the case of operon cbb3, an initial increase in expression is observed when the CO2 concentration is increased to 2.5% suggesting that CbbR acts as a positive regulator, but this is followed by subsequent decreases in transcript abundance as CO2 levels are increased beyond 2.5% indicating that other factors are involved in the regulation of cbb3. These factors are unknown but could include interactions with small metabolites and with the redox sensing RegAB system.
Network analyses of the multiple levels of CCM and CBB regulation including the regulation of bicarbonate uptake by a CbbR-like transcription factor (Omata et al., 2001), the interconnection between carbon and nitrogen metabolism (Wheatley et al., 2016) and with oxidative stress as sensed by the redox-sensitive two-component global regulator system RegAB (Romagnoli and Tabita, 2009), and other multilayered connections (Eisenhut et al., 2007; McClure et al., 2016; Westermark and Steuer, 2016) have been carried out principally in photoautotrophs. Less is known about the potential high level regulatory networks involved in Ci uptake and assimilation in extremely acidic chemolithoautotrophs7 (Campodonico et al., 2016). Of particular relevance to the present study, was the discovery that transcripts were more abundant for the glycogen biosynthetic pathway genes (glyB, EC 2.4.18; glyC, EC 2.7.7.27; amy and malQ, EC 2.4.1.25) when A. ferrooxidans was cultivated in sulfur versus ferrous iron and that this coincided with increased expression of CBB genes (Appia-Ayme et al., 2006). Glycogen biosynthesis/degradation has been shown to be interconnected with glycolysis and the pentose phosphate pathway in A. ferrooxidans (Mamani et al., 2016), supporting the idea that there is a direct connection with CBB cycle genes and the biosynthesis of glycogen. It is postulated that more energy is available when sulfur is used as an energy source and we propose that this is used as an opportunity to synthesize glycogen as a stored energy source as has been proposed in other organisms (Goh and Klaenhammer, 2013; Preiss, 2014).
The availability of Ci depends, in part, on the pH of the environment. At high pH values (>pH 9) it occurs principally as carbonate/bicarbonate (HCO3-/CO32-). At circumneutral pH values, it is mainly available as bicarbonate, whereas in very low pH environments (<pH4) Ci occurs principally as a dissolved hydrated CO2 gas (H2CO3). In addition, to the different chemical forms of Ci, their concentrations can vary over a wide range in different environments (Sandrini et al., 2014, 2015; Klanchui et al., 2017).
Whereas Ci uptake has been studied extensively in cyanobacteria, to the best of our knowledge, there are no studies on the uptake of bicarbonate in very low pH environments. In initial studies using BlastP with an acceptance cut-off of 1e-06 to probe the genome of A. ferrooxidans, we were unable to detect any of the known bicarbonate transporters. However, weak sequence similarity of SulP with the bicarbonate transporter BicA was observed and additional phylogenomic and gene microsynteny studies supported the prediction that SulP was a bicarbonate transporter rather than the original prediction that it was a sulfate transporter (Figure 4). A. ferrooxidans grows optimally at pH 2.4 when ferrous iron is used as an energy source and would be expected to rely principally on the free diffusion of the hydrated CO2 gas (H2CO3) through the membrane as their source of Ci. So why does A. ferrooxidans have a predicted bicarbonate transporter?
Although A. ferrooxidans grows at a pH optimum of 2.5 when grown on ferrous iron medium, it can also grow at pH 5 when elemental sulfur is used as an energy source (Mcgoran et al., 1969). At this pH, and in an environment with a temperature of 25°C and a salinity of 5,000 ppm, up to 10% of the dissolved Ci could be in the form of bicarbonate (WikiVividly, 2018) and having a bicarbonate transporter would allow A. ferrooxidans to use this source of Ci. This would permit A. ferrooxidans to exploit a wide range of HCO3- availability, providing potential access to environments with a spectrum of pH values from, e.g., pH 1 to at least pH 5. Thus A. ferrooxidans would be considered a “generalist” rather than a “specialist” (Baronchelli et al., 2013) in a dynamic environment such as a bioleaching heap where initial pHs are around 5–6 at a time when acid addition is consumed by, e.g., silica minerals (Dopson et al., 2008, 2009), and before sulfur compound oxidation to sulfuric acid has lowered the pH to the A. ferrooxidans optimum.
The datasets generated for this study can be found in GenBank NCBI, NC_011761.
DH and EJ conceived the project. ME, EJ, and DH planned the experiments. ME carried out the experiments and CG helped with the bioinformatic analyses. All authors interpreted the results. DH and MD wrote the initial draft of the paper. All authors contributed to manuscript revision and approved the submitted version.
This work was supported by the Programa de Apoyo a Centros con Financiamiento Basal AFB 170004 to Fundación Ciencia & Vida and grants from Fondecyt 1130683 and 1181717. ME received a Deutscher Akademischer Austauschdienst (DAAD).
The authors declare that the research was conducted in the absence of any commercial or financial relationships that could be construed as a potential conflict of interest.
We thank Eva Vergara for bioinformatic support.
The Supplementary Material for this article can be found online at: https://www.frontiersin.org/articles/10.3389/fmicb.2019.00603/full#supplementary-material
AMD, acid mine drainage; CBB, Calvin–Benson–Bassham; CCM, carbon concentration mechanism; Ci, inorganic carbon.
Aggarwal, M., and McKenna, R. (2015). Carbonic anhydrases: nature’s way to balance CO2 concentration. Biochem. Mol. Biol. J. 1:8. doi: 10.21767/22471-28084.100008
Amils, R., Fernandez-Remolar, D., and The IPBSL Team (2014). Rio tinto: a geochemical and mineralogical terrestrial analogue of Mars. Life 4, 511–534. doi: 10.3390/life4030511
Appia-Ayme, C., Quatrini, R., Denis, Y., Denizot, F., Silver, S., Roberto, F., et al. (2006). Microarray and bioinformatic analyses suggest models for carbon metabolism in the autotroph Acidithiobacillus ferrooxidans. Hydrometallurgy 83, 273–280. doi: 10.1016/j.hydromet.2006.03.029
Badger, M. R., and Bek, E. J. (2008). Multiple Rubisco forms in proteobacteria: their functional significance in relation to CO2 acquisition by the CBB cycle. J. Exp. Bot. 59, 1525–1541. doi: 10.1093/jxb/erm297
Baronchelli, A., Chater, N., Christiansen, M. H., and Pastor-Satorras, R. (2013). Evolution in a changing environment. PLoS One 8:e52742. doi: 10.1371/journal.pone.0052742
Boden, R., Cunliffe, M., Scanlan, J., Moussard, H., Kits, K. D., Klotz, M. G., et al. (2011). Complete genome sequence of the aerobic marine methanotroph Methylomonas methanica MC09. J. Bacteriol. 193, 7001–7002. doi: 10.1128/JB.06267-11
Bohnke, S., and Perner, M. (2017). Unraveling RubisCO form I and form II regulation in an uncultured organism from a deep-sea hydrothermal vent via metagenomic and mutagenesis studies. Front. Microbiol. 8:1303. doi: 10.3389/fmicb.2017.01303
Bonnefoy, V., and Holmes, D. S. (2012). Genomic insights into microbial oxidation and iron homeostasis in extremely acidic environments. Environ. Microbiol. 14, 1597–1611. doi: 10.1111/j.1462-2920.2011.02626.x
Burghout, P., Cron, L. E., Gradstedt, H., Quintero, B., Simonetti, E., Bijlsma, J. J., et al. (2010). Carbonic anhydrase is essential for Streptococcus pneumoniae growth in environmental ambient air. J. Bacteriol. 192, 4054–4062. doi: 10.1128/JB.00151-10
Burnap, R., Hagemann, M., and Kaplan, A. (2015). Regulation of CO2 concentrating mechanism in cyanobacteria. Life 5:348. doi: 10.3390/life5010348
Campodonico, M. A., Vaisman, D., Castro, J. F., Razmilic, V., Mercado, F., Andrews, B. A., et al. (2016). Acidithiobacillus ferrooxidans’s comprehensive model driven analysis of the electron transfer metabolism and synthetic strain design for biomining applications. Metab. Eng. Commun. 3, 84–96. doi: 10.1016/j.meteno.2016.03.003
Cardenas, J. P., Quatrini, R., and Holmes, D. S. (2016). “Progress in acidophile genomics,” in Acidophiles: Life in Extremely Acidic Environments, eds R. Quatrini and D. B. Johnson (Poole: Caister Academic Press), 179–197. doi: 10.21775/9781910190333.11
Cardenas, J. P., Valdes, J., Quatrini, R., Duarte, F., and Holmes, D. S. (2010). Lessons from the genomes of extremely acidophilic bacteria and archaea with special emphasis on bioleaching microorganisms. Appl. Microbiol. Biotechnol. 88, 605–620. doi: 10.1007/s00253-010-2795-9
Carroll, J. J., and Mather, A. E. (1992). The system carbon dioxide-water and the Krichevsky-Kasarnovsky equation. J. Solution Chem. 21, 607–621. doi: 10.1007/BF00650756
Castresana, J. (2000). Selection of conserved blocks from multiple alignments for their use in phylogenetic analysis. Mol. Biol. Evol. 17, 540–552. doi: 10.1093/oxfordjournals.molbev.a026334
Chao, J., Wang, W., Xiao, S. M., and Liu, X. D. (2008). Response of Acidithiobacillus ferrooxidans ATCC 23270 gene expression to acid stress. World J. Microbiol. Biotechnol. 24, 2103–2109. doi: 10.1007/s11274-008-9715-5
Chen, L. X., Hu, M., Huang, L. N., Hua, Z. S., Kuang, J. L., Li, S. J., et al. (2015). Comparative metagenomic and metatranscriptomic analyses of microbial communities in acid mine drainage. ISME J. 9, 1579–1592. doi: 10.1038/ismej.2014.245
Daley, S. M., Kappell, A. D., Carrick, M. J., and Burnap, R. L. (2012). Regulation of the cyanobacterial CO2-concentrating mechanism involves internal sensing of NADP+ and α-ketogutarate levels by transcription factor CcmR. PLoS One 7:e41286. doi: 10.1371/journal.pone.0041286
Dangel, A. W., and Tabita, F. R. (2015). CbbR, the master regulator for microbial carbon dioxide fixation. J. Bacteriol. 197, 3488–3498. doi: 10.1128/JB.00442-15
Darriba, D., Taboada, G. L., Doallo, R., and Posada, D. (2011). ProtTest 3: fast selection of best-fit models of protein evolution. Bioinformatics 27, 1164–1165. doi: 10.1093/bioinformatics/btr088
Dobrinski, K. P., Longo, D. L., and Scott, K. M. (2005). The carbon-concentrating mechanism of the hydrothermal vent chemolithoautotroph Thiomicrospira crunogena. J. Bacteriol. 187, 5761–5766. doi: 10.1128/JB.187.16.5761-5766.2005
Dopson, M., Halinen, A.-K., Rahunen, N., Boström, D., Sundkvist, J.-E., Riekkola-Vanhanen, M., et al. (2008). Silicate mineral dissolution during heap bioleaching. Biotechnol. Bioeng. 99, 811–820. doi: 10.1002/bit.21628
Dopson, M., and Johnson, D. B. (2012). Biodiversity, metabolism and applications of acidophilic sulfur- metabolizing micro-organisms. Environ. Microbiol. 14, 2620–2631. doi: 10.1111/j.1462-2920.2012.02749.x
Dopson, M., Lövgren, L., and Boström, D. (2009). Silicate mineral dissolution in the presence of acidophilic microorganisms: implications for heap bioleaching. Hydrometallurgy 96, 288–293. doi: 10.1016/j.hydromet.2008.11.004
Dubbs, P., Dubbs, J. M., and Tabita, F. R. (2004). Effector-mediated interaction of CbbRI and CbbRII regulators with target sequences in Rhodobacter capsulatus. J. Bacteriol. 186, 8026–8035. doi: 10.1128/JB.186.23.8026-8035.2004
Eisenhut, M., Von, Wobeser, E. A., Jonas, L., Schubert, H., Ibelings, B. W., et al. (2007). Long-term response toward inorganic carbon limitation in wild type and glycolate turnover mutants of the cyanobacterium Synechocystis sp. strain PCC 6803. Plant Physiol. 144, 1946–1959.
Esparza, M., Bowien, B., Jedlicki, E., and Holmes, D. S. (2009). Gene organization and CO2-responsive expression of four cbb operons in the biomining bacterium Acidithiobacillus ferrooxidans. Adv. Mater. Res. 7, 207–210. doi: 10.4028/www.scientific.net/AMR.71-73.207
Esparza, M., Cardenas, J. P., Bowien, B., Jedlicki, E., and Holmes, D. S. (2010). Genes and pathways for CO2 fixation in the obligate, chemolithoautotrophic acidophile, Acidithiobacillus ferrooxidans. BMC Microbiol. 10:229. doi: 10.1186/1471-2180-10-229
Esparza, M., Jedlicki, E., Dopson, M., and Holmes, D. S. (2015). Expression and activity of the Calvin–Benson–Bassham cycle transcriptional regulator CbbR from Acidithiobacillus ferrooxidans in Ralstonia eutropha. FEMS Microbiol. Lett. 362:fnv108. doi: 10.1093/femsle/fnv1108
Felce, J., and Saier, M. H. Jr. (2004). Carbonic anhydrases fused to anion transporters of the SulP family: evidence for a novel type of bicarbonate transporter. J. Mol. Microbiol. Biotechnol. 8, 169–176. doi: 10.1159/000085789
Frost, S. C., and McKenna, R. (eds) (2014). Carbonic Anhydrase: Mechanism, Regulation, Links to Disease, and Industrial Applications. Dordrecht: Springer. doi: 10.1007/978-94-007-7359-2
Gassmann, M., Grenacher, B., Rohde, B., and Vogel, J. (2009). Quantifying Western blots: pitfalls of densitometry. Electrophoresis 30, 1845–1855. doi: 10.1002/elps.200800720
Goh, Y. J., and Klaenhammer, T. R. (2013). A functional glycogen biosynthesis pathway in Lactobacillus acidophilus: expression and analysis of the glg operon. Mol. Microbiol. 89, 1187–1200. doi: 10.1111/mmi.12338
Griffiths, H., Meyer, M. T., and Rickaby, R. E. M. (2017). Overcoming adversity through diversity: aquatic carbon concentrating mechanisms. J. Exp. Bot. 68, 3689–3695. doi: 10.1093/jxb/erx278
Guacucano, M., Levican, G., Holmes, D. S., and Jedlicki, E. (2000). An RT-PCR artifact in the characterization of bacterial operons. Electron. J. Biotechnol. 3, 12–13.
Guo, X., Yin, H., Cong, J., Dai, Z., Liang, Y., and Liu, X. (2013). RubisCO gene clusters found in a metagenome microarray from acid mine drainage. Appl. Environ. Microbiol. 79, 2019–2026. doi: 10.1128/AEM.03400-12
Gutknecht, J., Bisson, M. A., and Tosteson, F. C. (1977). Diffusion of carbon dioxide through lipid bilayer membranes: effects of carbonic anhydrase, bicarbonate, and unstirred layers. J. Gen. Physiol. 69, 779–794. doi: 10.1085/jgp.69.6.779
Hedrich, S., and Johnson, D. B. (2013). Aerobic and anaerobic oxidation of hydrogen by acidophilic bacteria. FEMS Microbiol. Lett. 349, 40–45. doi: 10.1111/1574-6968.12290
Hedrich, S., and Schippers, A. (2016). “Distribution of acidophilic microorganisms in natural and man-made acidic enviroments,” in Acidophiles: Life in Extremely Acidic Environments, eds R. Quatrini and D. B. Johnson (Norfolk: Caister Academic Press), 153–175. doi: 10.21775/9781910190333.10
Heinhorst, S., Baker, S. H., Johnson, D. R., Davies, P. S., Cannon, G. C., and Shively, J. M. (2002). Two copies of form I RuBisCO genes in Acidithiobacillus ferrooxidans ATCC 23270. Curr. Microbiol. 45, 115–117. doi: 10.1007/s00284-001-0094-5
Hofmann, S. (1993). TMbase – a database of membrane spanning proteins segments. Biol. Chem. Hoppe Seyler 374, 166.
Huntley, S., Hamann, N., Wegener-Feldbrugge, S., Treuner-Lange, A., Kube, M., Reinhardt, R., et al. (2011). Comparative genomic analysis of fruiting body formation in Myxococcales. Mol. Biol. Evol. 28, 1083–1097. doi: 10.1093/molbev/msq292
Johnson, D. B. (2012). Geomicrobiology of extremely acidic subsurface environments. FEMS Microbiol. Ecol. 81, 2–12. doi: 10.1111/j.1574-6941.2011.01293.x
Joshi, G. S., Zianni, M., Bobst, C. E., and Tabita, F. R. (2012). Further unraveling the regulatory twist by elucidating metabolic coinducer-mediated CbbR-cbbI promoter interactions in Rhodopseudomonas palustris CGA010. J. Bacteriol. 194, 1350–1360. doi: 10.1128/JB.06418-11
Katoh, K., and Standley, D. M. (2013). MAFFT multiple sequence alignment software version 7: improvements in performance and usability. Mol. Biol. Evol. 30, 772–780. doi: 10.1093/molbev/mst010
Kaufman, A. J., and Xiao, S. (2003). High CO2 levels in the Proterozoic atmosphere estimated from analyses of individual microfossils. Nature 425, 279–282. doi: 10.1038/nature01902
Klanchui, A., Cheevadhanarak, S., Prommeenate, P., and Meechai, A. (2017). Exploring components of the CO2-concentrating mechanism in alkaliphilic cyanobacteria through genome-based analysis. Comput. Struct. Biotechnol. J. 15, 340–350. doi: 10.1016/j.csbj.2017.05.001
Kotz, S., Read, C. B., Balakrishnan, N., Vidakovic, B., Johnson, N. L., Kaufmann, J., et al. (2014). “Analysis of variance ANOVA,” in Encyclopedia of Statistical Sciences, eds S. Kotz, C. B. Read, N. Balakrishnan, B. Vidakovic, and N. L. Johnson (Hoboken, NJ: Wiley).
Krogh, A., Larsson, B., Von Heijne, G., and Sonnhammer, E. L. (2001). Predicting transmembrane protein topology with a hidden markov model: application to complete genomes. J. Mol. Biol. 305, 567–580. doi: 10.1006/jmbi.2000.4315
Kusian, B., and Bowien, B. (1997). Organization and regulation of cbb CO2 assimilation genes in autotrophic bacteria. FEMS Microbiol. Rev. 21, 135–155. doi: 10.1111/j.1574-6976.1997.tb00348.x
Kusian, B., Sultemeyer, D., and Bowien, B. (2002). Carbonic anhydrase is essential for growth of Ralstonia eutropha at ambient CO2 concentrations. J. Bacteriol. 184, 5018–5026. doi: 10.1128/JB.184.18.5018-5026.2002
Larkin, M. A., Blackshields, G., Brown, N. P., Chenna, R., Mcgettigan, P. A., Mcwilliam, H., et al. (2007). Clustal W and Clustal X version 2.0. Bioinformatics 23, 2947–2948. doi: 10.1093/bioinformatics/btm404
Levican, G., Ugalde, J. A., Ehrenfeld, N., Maass, A., and Parada, P. (2008). Comparative genomic analysis of carbon and nitrogen assimilation mechanisms in three indigenous bioleaching bacteria: predictions and validations. BMC Genomics 9:581. doi: 10.1186/1471-2164-9-581
Liu, H., Yin, H., Dai, Y., Dai, Z., Liu, Y., Li, Q., et al. (2011). The co-culture of Acidithiobacillus ferrooxidans and Acidiphilium acidophilum enhances the growth, iron oxidation, and CO2 fixation. Arch. Microbiol. 193, 857–866. doi: 10.1007/s00203-011-0723-8
Lotlikar, S. R., Hnatusko, S., Dickenson, N. E., Choudhari, S. P., Picking, W. L., and Patrauchan, M. A. (2013). Three functional beta-carbonic anhydrases in Pseudomonas aeruginosa PAO1: role in survival in ambient air. Microbiology 159, 1748–1759. doi: 10.1099/mic.0.066357-0
Mamani, S., Moinier, D., Denis, Y., Soulere, L., Queneau, Y., Talla, E., et al. (2016). Insights into the quorum sensing regulon of the acidophilic Acidithiobacillus ferrooxidans revealed by transcriptomic in the presence of an acyl homoserine lactone superagonist analog. Front. Microbiol. 7:1365. doi: 10.3389/fmicb.2016.01365
Mangan, N. M., Flamholz, A., Hood, R. D., Milo, R., and Savage, D. F. (2016). pH determines the energetic efficiency of the cyanobacterial CO2 concentrating mechanism. Proc. Natl. Acad. Sci. U.S.A. 113, E5354–E5362. doi: 10.1073/pnas.1525145113
Marietou, A., Røy, H., Jørgensen, B. B., and Kjeldsen, K. U. (2018). Sulfate transporters in dissimilatory sulfate reducing microorganisms: a comparative genomics analysis. Front. Microbiol. 9:309. doi: 10.3389/fmicb.2018.00309
McClure, R. S., Overall, C. C., Mcdermott, J. E., Hill, E. A., Markillie, L. M., Mccue, L. A., et al. (2016). Network analysis of transcriptomics expands regulatory landscapes in Synechococcus sp. PCC 7002. Nucleic Acids Res. 44, 8810–8825.
Mcgoran, C. J. M., Duncan, D. W., and Walden, C. C. (1969). Growth of Thiobacillus ferrooxidans on various substrates. Can. J. Microbiol. 15, 135–138. doi: 10.1139/m69-024
Merlin, C., Masters, M., Mcateer, S., and Coulson, A. (2003). Why is carbonic anhydrase essential to Escherichia coli? J. Bacteriol. 185, 6415–6424. doi: 10.1128/JB.185.21.6415-6424.2003
Mitsuhashi, S., Ohnishi, J., Hayashi, M., and Ikeda, M. (2004). A gene homologous to beta-type carbonic anhydrase is essential for the growth of Corynebacterium glutamicum under atmospheric conditions. Appl. Microbiol. Biotechnol. 63, 592–601. doi: 10.1007/s00253-003-1402-8
Moraes, T. F., and Reithmeier, R. A. F. (2012). Membrane transport metabolons. Biochim. Biophys. Acta 1818, 2687–2706. doi: 10.1016/j.bbamem.2012.06.007
Nakamura, T., Yamada, K. D., Tomii, K., and Katoh, K. (2018). Parallelization of MAFFT for large-scale multiple sequence alignments. Bioinformatics 34, 2490–2492. doi: 10.1093/bioinformatics/bty121
Nguyen, L. T., Schmidt, H. A., Von Haeseler, A., and Minh, B. Q. (2015). IQ-TREE: a fast and effective stochastic algorithm for estimating maximum-likelihood phylogenies. Mol. Biol. Evol. 32, 268–274. doi: 10.1093/molbev/msu300
Ogren, W. L., and Bowes, G. (1971). Ribulose diphosphate carboxylase regulates soybean photorespiration. Nat. New Biol. 230, 159–160. doi: 10.1038/newbio230159a0
Omata, T., Gohta, S., Takahashi, Y., Harano, Y., and Maeda, S. (2001). Involvement of a CbbR homolog in low CO2-induced activation of the bicarbonate transporter operon in cyanobacteria. J. Bacteriol. 183, 1891–1898. doi: 10.1128/JB.183.6.1891-1898.2001
Orus, M. I., Rodriguez-Buey, M. L., Marco, E., and Fernandez-Valiente, E. (2001). Changes in carboxysome structure and grouping and in photosynthetic affinity for inorganic carbon in Anabaena strain PCC 7119 (Cyanophyta) in response to modification of CO2 and Na+ supply. Plant Cell Physiol. 42, 46–53. doi: 10.1093/pcp/pce005
Osorio, H., Mangold, S., Denis, Y., Nancucheo, I., Johnson, D. B., Bonnefoy, V., et al. (2013). Anaerobic sulfur metabolism coupled to dissimilatory iron reduction in the extremophile Acidithiobacillus ferrooxidans. Appl. Environ. Microbiol. 79, 2172–2181. doi: 10.1128/AEM.03057-12
Preiss, J. (2014). Glycogen: biosynthesis and regulation. EcoSal Plus 6. doi: 10.1128/ecosalplus.ESP-0015-2014
Price, G. D., Woodger, F. J., Badger, M. R., Howitt, S. M., and Tucker, L. (2004). Identification of a SulP-type bicarbonate transporter in marine cyanobacteria. Proc. Natl. Acad. Sci. U.S.A. 101, 18228–18233. doi: 10.1073/pnas.0405211101
Pronk, J. T., Liem, K., Bos, P., and Keunen, J. G. (1991). Energy transduction by anaerobic ferric iron respiration in Thiobacillus ferrooxidans. Appl. Environ. Microbiol. 57, 2063–2068.
Quatrini, R., Lefimil, C., Holmes, D. S., and Jedlicki, E. (2005). The ferric iron uptake regulator (Fur) from the extreme acidophile Acidithiobacillus ferrooxidans. Microbiology 151, 2005–2015. doi: 10.1099/mic.0.27581-0
Quatrini, R., Lefimil, C., Veloso, F. A., Pedroso, I., Holmes, D. S., and Jedlicki, E. (2007). Bioinformatic prediction and experimental verification of Fur-regulated genes in the extreme acidophile Acidithiobacillus ferrooxidans. Nucleic Acids Res. 35, 2153–2166. doi: 10.1093/nar/gkm068
Rae, B. D., Long, B. M., Whitehead, L. F., Forster, B., Badger, M. R., and Price, G. D. (2013). Cyanobacterial carboxysomes: microcompartments that facilitate CO2 fixation. J. Mol. Microbiol. Biotechnol. 23, 300–307. doi: 10.1159/000351342
Rajan, V. (2013). A method of alignment masking for refining the phylogenetic signal of multiple sequence alignments. Mol. Biol. Evol. 30, 689–712. doi: 10.1093/molbev/mss264
Raven, J. A. (1991). Implications of inorganic carbon utilization: ecology, evolution, and geochemistry. Can. J. Bot. 69, 908–924. doi: 10.1139/b91-118
Rocca, J. D., Hall, E. K., Lennon, J. T., Evans, S. E., Waldrop, M. P., Cotner, J. B., et al. (2015). Relationships between protein-encoding gene abundance and corresponding process are commonly assumed yet rarely observed. ISME J. 9, 1693–1699. doi: 10.1038/ismej.2014.252
Romagnoli, S., and Tabita, F. R. (2009). “Carbon dioxide metabolism and its regulation in nonsulfur purple photosynthetic bacteria,” in The Purple Phototrophic Bacteria. Advances in Photosynthesis and Respiration, eds C. N. Hunter, F. Daldal, M. Thurnauer, and T. Beatty (Dordrecht: Springer).
Rye, R., Kuo, P. H., and Holland, H. D. (1995). Atmospheric carbon dioxide concentrations before 2.2 billion years ago. Nature 378, 603–605. doi: 10.1038/378603a0
Saier, M. H. Jr., Reddy, V. S., Tsu, B. V., Ahmed, M. S., Li, C., and Moreno-Hagelsieb, G. (2016). The Transporter Classification Database (TCDB): recent advances. Nucleic Acids Res. 44, D372–D379. doi: 10.1093/nar/gkv1103
Sandrini, G., Jakupovic, D., Matthijs, H. C., and Huisman, J. (2015). Strains of the harmful cyanobacterium Microcystis aeruginosa differ in gene expression and activity of inorganic carbon uptake systems at elevated CO2 Levels. Appl. Environ. Microbiol. 81, 7730–7739. doi: 10.1128/AEM.02295-15
Sandrini, G., Matthijs, H. C., Verspagen, J. M., Muyzer, G., and Huisman, J. (2014). Genetic diversity of inorganic carbon uptake systems causes variation in CO2 response of the cyanobacterium Microcystis. ISME J. 8, 589–600. doi: 10.1038/ismej.2013.179
Sawaya, M. R., Cannon, G. C., Heinhorst, S., Tanaka, S., Williams, E. B., Yeates, T. O., et al. (2006). The structure of beta-carbonic anhydrase from the carboxysomal shell reveals a distinct subclass with one active site for the price of two. J. Biol. Chem. 281, 7546–7555. doi: 10.1074/jbc.M510464200
Scott, K. M., Leonard, J. M., Boden, R., Chaput, D., Dennison, C., Haller, E., et al. (2019). Diversity in CO2-concentrating mechanisms among chemolithoautotrophs from the genera Hydrogenovibrio, Thiomicrorhabdus, and Thiomicrospira, ubiquitous in sulfidic habitats worldwide. Appl. Environ. Microbiol. doi: 10.1128/AEM.02096-18 [Epub ahead of print].
Shibagaki, N., and Grossman, A. R. (2006). The role of the STAS domain in the function and biogenesis of a sulfate transporter as probed by random mutagenesis. J. Biol. Chem. 281, 22964–22973. doi: 10.1074/jbc.M603462200
Shimizu, R., Dempo, Y., Nakayama, Y., Nakamura, S., Bamba, T., Fukusaki, E., et al. (2015). New insight into the role of the Calvin Cycle: reutilization of CO2 emitted through sugar degradation. Sci. Rep. 5:11617. doi: 10.1038/srep11617
Slonczewski, J. L., Fujisawa, M., Dopson, M., and Krulwich, T. A. (2009). Cytoplasmic pH measurement and homeostasis in bacteria and archaea. Adv. Microb. Physiol. 55, 1–79. doi: 10.1016/S0065-2911(09)05501-5
Smith, K. S., and Ferry, J. G. (2000). Prokaryotic carbonic anhydrases. FEMS Microbiol. Rev. 24, 335–366. doi: 10.1111/j.1574-6976.2000.tb00546.x
So, A. K., Espie, G. S., Williams, E. B., Shively, J. M., Heinhorst, S., and Cannon, G. C. (2004). A novel evolutionary lineage of carbonic anhydrase (epsilon class) is a component of the carboxysome shell. J. Bacteriol. 186, 623–630. doi: 10.1128/JB.186.3.623-630.2004
Sonnhammer, E. L., Von Heijne, G., and Krogh, A. (1998). A hidden Markov model for predicting transmembrane helices in protein sequences. Proc. Int. Conf. Intell. Syst. Mol. Biol. 6, 175–182.
Soto, P., Acosta, M., Tapia, P., Contador, Y., Velasquez, A., Espoz, C., et al. (2013). From mesophilic to moderate thermophilic populations in an industrial heap bioleaching process. Adv. Mater. Res. 825, 376–379. doi: 10.4028/www.scientific.net/AMR.825.376
Svenning, M. M., Hestnes, A. G., Wartiainen, I., Stein, L. Y., Klotz, M. G., Kalyuzhnaya, M. G., et al. (2011). Genome sequence of the Arctic methanotroph Methylobacter tundripaludum SV96. J. Bacteriol. 193, 6418–6419. doi: 10.1128/JB.05380-11
Szklarczyk, D., Morris, J. H., Cook, H., Kuhn, M., Wyder, S., Simonovic, M., et al. (2017). The STRING database in 2017: quality-controlled protein-protein association networks, made broadly accessible. Nucleic Acids Res. 45, D362–D368. doi: 10.1093/nar/gkw937
Tabita, F. R., Satagopan, S., Hanson, T. E., Kreel, N. E., and Scott, S. S. (2008). Distinct form I, II, III, and IV Rubisco proteins from the three kingdoms of life provide clues about Rubisco evolution and structure/function relationships. J. Exp. Bot. 59, 1515–1524. doi: 10.1093/jxb/erm361
Tamoi, M., Nagaoka, M., Yabuta, Y., and Shigeoka, S. (2005). Carbon metabolism in the Calvin cycle. Plant Biotechnol. 22, 355–360. doi: 10.5511/plantbiotechnology.22.355
Teng, W., Kuang, J., Luo, Z., and Shu, W. (2017). Microbial diversity and community assembly across environmental gradients in acid mine drainage. Minerals 7:106. doi: 10.3390/min7060106
Thornton, B., and Basu, C. (2011). Real-time PCR (qPCR) primer design using free online software. Biochem. Mol. Biol. Educ. 39, 145–154. doi: 10.1002/bmb.20461
Tortell, P. D. (2000). Evolutionary and ecological perspectives on carbon acquisition in phytoplankton. Limnol. Oceanogr. 45, 744–750. doi: 10.4319/lo.2000.45.3.0744
Toyoda, K., Yoshizawa, Y., Arai, H., Ishii, M., and Igarashi, Y. (2005). The role of two CbbRs in the transcriptional regulation of three ribulose-1,5-bisphosphate carboxylase/oxygenase genes in Hydrogenovibrio marinus strain MH-110. Microbiology 151, 3615–3625. doi: 10.1099/mic.0.28056-0
Tsai, Y.-C. C., Lapina, M. C., Bhushan, S., and Mueller-Cajar, O. (2015). Identification and characterization of multiple rubisco activases in chemoautotrophic bacteria. Nat. Commun. 6:8883. doi: 10.1038/ncomms9883
Tukey, J. W. (1949). Comparing individual means in the analysis of variance. Biometrics 5, 99–114. doi: 10.2307/3001913
Valdés, J., Cárdenas, J. P., Quatrini, R., Esparza, M., Osorio, H., Duarte, F., et al. (2010). Comparative genomics begins to unravel the ecophysiology of bioleaching. Hydrometallurgy 104, 471–476. doi: 10.1016/j.hydromet.2010.03.028
Valdes, J., Pedroso, I., Quatrini, R., Dodson, R. J., Tettelin, H., Blake, R., et al. (2008). Acidithiobacillus ferrooxidans metabolism: from genome sequence to industrial applications. BMC Genomics 9:597. doi: 10.1186/1471-2164-9-597
Vera, M., Schippers, A., and Sand, W. (2013). Progress in bioleaching: fundamentals and mechanisms of bacterial metal sulfide oxidation–part A. Appl. Microbiol. Biotechnol. 97, 7529–7541. doi: 10.1007/s00253-013-4954-2
Viale, A. M., Kobayashi, H., Akazawa, T., and Henikoff, S. (1991). rbcR [correction of rcbR], a gene coding for a member of the LysR family of transcriptional regulators, is located upstream of the expressed set of ribulose 1,5-bisphosphate carboxylase/oxygenase genes in the photosynthetic bacterium Chromatium vinosum. J. Bacteriol. 173, 5224–5229. doi: 10.1128/jb.173.16.5224-5229.1991
Ward, N., Larsen, O., Sakwa, J., Bruseth, L., Khouri, H., Durkin, A. S., et al. (2004). Genomic insights into methanotrophy: the complete genome sequence of Methylococcus capsulatus (Bath). PLoS Biol. 2:e303. doi: 10.1371/journal.pbio.0020303
Westermark, S., and Steuer, R. (2016). Toward multiscale models of cyanobacterial growth: a modular approach. Front. Bioeng. Biotechnol. 4:95. doi: 10.3389/fbioe.2016.00095
Wheatley, N. M., Eden, K. D., Ngo, J., Rosinski, J. S., Sawaya, M. R., Cascio, D., et al. (2016). A PII-like protein regulated by bicarbonate: structural and biochemical studies of the carboxysome-associated CPII protein. J. Mol. Biol. 428, 4013–4030. doi: 10.1016/j.jmb.2016.07.015
WikiVividly (2018). Bjerrum Plot. Available at: https://wikivividly.com/wiki/: WikiVividly.
Williams, K. P., and Kelly, D. P. (2013). Proposal for a new class within the phylum Proteobacteria, Acidithiobacillia classis nov., with the type order Acidithiobacillales, and emended description of the class Gammaproteobacteria. Int. J. Syst. Evol. Microbiol. 63, 2901–2906. doi: 10.1099/ijs.0.049270-0
Yu, N. Y., Wagner, J. R., Laird, M. R., Melli, G., Rey, S., Lo, R., et al. (2010). PSORTb 3.0: improved protein subcellular localization prediction with refined localization subcategories and predictive capabilities for all prokaryotes. Bioinformatics 26, 1608–1615. doi: 10.1093/bioinformatics/btq249
Zhang, X., Niu, J., Liang, Y., Liu, X., and Yin, H. (2016). Metagenome-scale analysis yields insights into the structure and function of microbial communities in a copper bioleaching heap. BMC Genetics 17:21. doi: 10.1186/s12863-016-0330-4
Keywords: CO2 fixation, CCM, carbon concentration mechanism, Acidithiobacillus ferrooxidans, acidic environment, low pH environment, bicarbonate uptake, RubisCO
Citation: Esparza M, Jedlicki E, González C, Dopson M and Holmes DS (2019) Effect of CO2 Concentration on Uptake and Assimilation of Inorganic Carbon in the Extreme Acidophile Acidithiobacillus ferrooxidans. Front. Microbiol. 10:603. doi: 10.3389/fmicb.2019.00603
Received: 02 November 2018; Accepted: 11 March 2019;
Published: 04 April 2019.
Edited by:
Gloria Paz Levicán, Universidad de Santiago de Chile, ChileReviewed by:
Sabrina Hedrich, Federal Institute for Geosciences and Natural Resources, GermanyCopyright © 2019 Esparza, Jedlicki, González, Dopson and Holmes. This is an open-access article distributed under the terms of the Creative Commons Attribution License (CC BY). The use, distribution or reproduction in other forums is permitted, provided the original author(s) and the copyright owner(s) are credited and that the original publication in this journal is cited, in accordance with accepted academic practice. No use, distribution or reproduction is permitted which does not comply with these terms.
*Correspondence: David S. Holmes, ZHNob2xtZXMyMDAwQHlhaG9vLmNvbQ==
Disclaimer: All claims expressed in this article are solely those of the authors and do not necessarily represent those of their affiliated organizations, or those of the publisher, the editors and the reviewers. Any product that may be evaluated in this article or claim that may be made by its manufacturer is not guaranteed or endorsed by the publisher.
Research integrity at Frontiers
Learn more about the work of our research integrity team to safeguard the quality of each article we publish.