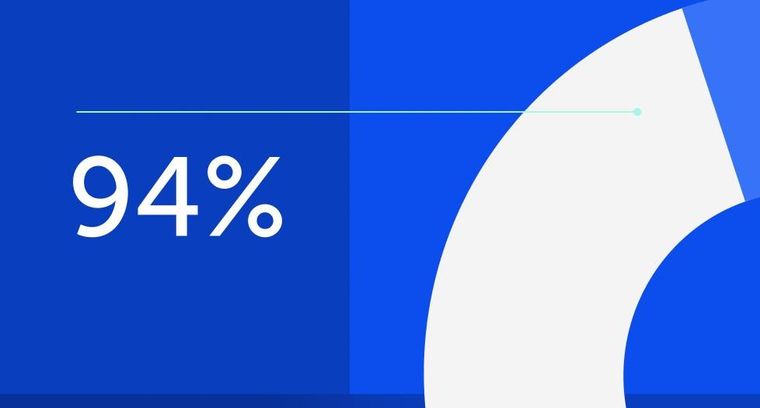
94% of researchers rate our articles as excellent or good
Learn more about the work of our research integrity team to safeguard the quality of each article we publish.
Find out more
REVIEW article
Front. Microbiol., 05 March 2019
Sec. Virology
Volume 10 - 2019 | https://doi.org/10.3389/fmicb.2019.00417
N6-methyladenosine (m6A), as a dynamic posttranscriptional RNA modification, recently gave rise to the field of viral epitranscriptomics. The interaction between virus and host is affected by m6A. Multiple m6A-modified viral RNAs have been observed. The epitranscriptome of m6A in host cells are altered after viral infection. The expression of viral genes, the replication of virus and the generation of progeny virions are influenced by m6A modifications in viral RNAs during virus infection. Meanwhile, the decorations of m6A in host mRNAs can make viral infections more likely to happen or can enhance the resistance of host to virus infection. However, the mechanism of m6A regulation in viral infection and host immune response has not been thoroughly elucidated to date. With the development of sequencing-based biotechnologies, transcriptome-wide mapping of m6A in viruses has been achieved, laying the foundation for expanding its functions and corresponding mechanisms. In this report, we summarize the positive and negative effects of m6A in distinct viral infection. Given the increasingly important roles of m6A in diverse viruses, m6A represents a novel potential target for antiviral therapy.
Among the diverse layers of epigenetic regulation, modifications of DNA and proteins have been explored in depth; however, RNA modification is a relatively new field (He, 2010). More than 100 posttranscriptional covalent modifications have been identified on RNA transcripts from various organisms, including viruses, yeast, and mammals (Meyer et al., 2012; Mamadou et al., 2013; Tan and Gao, 2018). The most prevalent modification of internal messenger RNA in eukaryotes and in nuclear-replicating viruses is the addition of a methyl group to the N6 position of adenosine, known as m6A (Dominissini et al., 2012; Meyer and Jaffrey, 2014). In 1975, m6A was first reported to exist in cellular mRNAs, and it was reported that there are three internal m6A residues on the average ∼2.2 kb cellular transcript (Desrosiers et al., 1975; Kennedy et al., 2017). Furthermore, highly decorated and regulated transcripts might include ten or more m6A groups (Linder et al., 2015). Similar to DNA methylation, the m6A modification is reversibly catalyzed by corresponding enzymes (Li et al., 2018). Despite the discovery of m6A decades ago (Desrosiers et al., 1974; Adams and Cory, 1975; Wei et al., 1975), the related signaling pathways it is involved in and the biological roles it plays were not fully described until recently (Nilsen, 2014; Cao et al., 2016). Meanwhile, with the development of efficient methods of m6A detection and subsequent analysis, increasing biological functions are being elucidated (Meyer et al., 2012; Li et al., 2015). m6A has been reported to control the fate of modified RNAs at multiple steps, including RNA splicing (Dominissini et al., 2012), mRNA stability (Li and Mason, 2014), cap-independent translation (Meyer et al., 2015), and miRNA biogenesis (Alarcon et al., 2015b). m6A-decorated RNAs participate in many biological processes, such as stress responses (Zhou et al., 2015), cellular reprogramming (Chen T. et al., 2015), circadian cycle (Fustin et al., 2013), stem cell differentiation (Yue et al., 2015), fertility (Zhao and He, 2017), and cancer (Pan et al., 2018).
Viruses, as a type of organism, were found to be modified by m6A in their genomic RNAs (Zhou et al., 2010; Courtney et al., 2017). Earlier studies have shown that some viruses, such as simian virus 40 (Lavi and Shatkin, 1975), influenza A virus (Krug et al., 1976), adenovirus (Sommer et al., 1976), avian sarcoma virus (Dimock and Stoltzfus, 1977), and RSV (Kane and Beemon, 1985) have m6A residues in their mRNAs. Narayan and colleagues performed the biochemical analysis of different influenza virus mRNAs, to detect the distribution of m6A and showed that the amount of m6A in different hemagglutinin (HA) mRNAs varied (Narayan et al., 1987). Those authors attempted to explore the functions of m6A in RNA splicing and translation, but the experimental conditions and relevant knowledge of m6A at that time were not enough. The specific sites of m6A were not mapped, and the functions of m6A in IAV remained vague for decades since the restriction with techniques and knowledge of the methyltransferases, demethylases, and m6A reader proteins then. Transcriptome-wide mapping of m6A was available after N6-methyladenosine-sequencing (m6A-seq) was developed by two independent research teams in Dominissini et al. (2012) and Meyer et al. (2012). In the following years, m6A has attracted the attention of scientists to elucidate the role it plays in viral epitranscriptomics (Gokhale and Horner, 2017; Kennedy et al., 2017). Immediately following m6A-seq, new technologies to unravel the m6A epitransctiptome were developed, including PA-m6A-seq, miCLIP, m6A-LAIC-seq, microarray, and SELECT (Chen K. et al., 2015; Li et al., 2015; Linder et al., 2015; Molinie et al., 2016; Xiao et al., 2018). The advantages and disadvantages of these techniques were well summarized in a review by Tan and Gao (2018). Based on these sequencing data, some bioinformatics tools were developed to help researchers investigate the potential functions and mechanisms of m6A modification. At present, most of the m6A decoration profiles have been available in some databases. A brief description of these databases related to m6A research are summarized in Table 1. Moreover, some computational m6A site predictors have been constructed, such as SRAMP (Zhou et al., 2016), m6Apred (Chen W. et al., 2015) and RNA-MethylPred (Jia et al., 2016). Although few studies have been done using these bioinformatics tools on the role of m6A in viral infection currently, they would be helpful for m6A prediction prior to experimental study. With the help of all these advanced technologies, a number virus types were subjected to transcriptome-wide mapping of m6A. Gokhale et al. (2016) mapped the m6A sites within the viral RNA genomes of the flaviviridae family of viruses, including HCV, ZIKV, DENV, YFV, and WNV. These researchers verified that m6A negatively regulated HCV infection by using m6A-abrogating mutations in HCV E1 (Gokhale et al., 2016). In addition, other viruses, such as influenza A virus (IAV) (Courtney et al., 2017), Kaposi’s Sarcoma-Associated Herpesvirus (KSHV) (Ye et al., 2017), Human Immunodeficiency Virus-1 (HIV-1) (Tirumuru et al., 2016), Simian Virus 40 (SV40) (Tsai et al., 2018), Hepatitis B Virus (HBV) (Imam et al., 2018), and Enterovirus 71 (EV71) (Hao et al., 2019) have been subjected to transcriptome-wide mapping of m6A and reported for their different roles in the life cycles of the viruses.
Our review summarizes the recent advances in related literature in this relatively new emerging field and discusses the different functions and relevant mechanisms of m6A in the biological processes of different types of viral infections.
The associated mechanisms that regulate m6A involve proteins working as writers, erasers, readers, and anti-readers (Edupuganti et al., 2017; Li et al., 2018). M6A decoration is not randomly distributed in RNA transcripts (Batista, 2017), which was found to occur on the consensus RNA motif of RRACH (R = A or G;H = A, U, or C), and preferentially center on specific transcript landmarks such as near 3′ untranslated regions (3′ UTRs) and stop codons or in long exons (Yang et al., 2018). m6A is added cotranscriptionally to nuclear pre-mRNAs by a multicomponent protein complex consisting of catalytic subunit Methyltransferase Like 3 (METTL3) (Yao et al., 2018), RNA-binding platform METTL14 (Kobayashi et al., 2018), cofactors Wilms tumor 1-associated protein (WTAP) and KIAA1429 (Schwartz et al., 2014; Scholler et al., 2018), two novel subunits of the methyltransferase complex RBM15 (Patil et al., 2016), and zinc finger CCCH domain-containing protein 13 (ZC3H13) (Guo et al., 2018; Knuckles et al., 2018; Wen et al., 2018). As the core methyltransferase subunit, METTL3 is a strongly conserved protein (Bujnicki et al., 2002; Yang et al., 2018) and has been demonstrated to selectively methylate the GAC or AAC motifs in synthetic single-stranded RNA in vitro (Rottman et al., 1994; Kan et al., 2017). METTL14 is also highly conserved in mammals and can form a stable protein heterodimer with METTL3 (Liu et al., 2014; Ping et al., 2014; Wang Y. et al., 2014). Wang et al. studied the crystal structure of Mettl3-Mettl14 complex and formulated that Mettl14 has a degenerate active site and is unavailable for catalysis (Wang et al., 2016). Mettl14 has a structural role that could offer an RNA-binding scaffold, allosterically activating and enhancing Mettl3’s catalytic function (Sledz and Jinek, 2016). As a regulatory subunit of the m6A methyltransferase complex, WTAP allows METTL3/METTL14 to interact with messenger RNAs in the nucleus to improve m6A modification efficiency (Liu et al., 2014; Ping et al., 2014). WTAP is required for the localization of the METTL3-METTL14 complex into nuclear speckles that are enriched with various precursor messenger RNA (pre-mRNA) processing factors (Ping et al., 2014). The depletion of KIAA1429 in human A549 cells results in a fourfold reduction of m6A abundance (Schwartz et al., 2014), suggesting a significant regulatory effect in the writer complex. Recently, RBM15 and its paralog RBM15B were shown to be members of the m6A methyltransferase complex that recruit the METTL3/14 protein complex to specific sites in RNA for the selective methylation (Patil et al., 2016). More recently, three research teams demonstrated that ZC3H13 was another member of the m6A writer complex and modulated m6A methylation (Guo et al., 2018; Knuckles et al., 2018; Wen et al., 2018). METTL16 is cognate with METTL3, was reported to control the cellular SAM level and catalyze the m6A group onto the U6 small nuclear RNA (Pendleton et al., 2017). More subunits of the m6A methyltransferase complex might be explored to achieve accurate posttranscriptional RNA regulation through selectively recognizing candidate m6A sites.
The removal of m6A from the decorated mRNA is catalyzed by the demethylase FTO or a-ketoglutarate-dependent dioxygenase AlkB homolog 5 (ALKBH5) (Jia et al., 2011; Zheng et al., 2013). Jia et al. (2011) found that FTO could efficiently demethylate m6A in RNA in vitro. They used siRNA mediated knockdown of FTO and then detected an increased level of m6A in mRNA, whereas the overexpression of FTO resulted in a decreased level of m6A in human HeLa cells. In 2013, another m6A demethylase, ALKBH5 was identified and it exhibited m6A demethylation efficiency comparable to that of FTO (Zheng et al., 2013). A recent report showed that ALKBH5-mediated m6A elimination in the nucleus of spermatocytes and round spermatids was elementary for correct splicing and the production of longer 3′-UTR mRNAs (Tang et al., 2018).
The function of m6A modification on target mRNAs is thought to be mediated by “reader” proteins (Riquelme-Barrios et al., 2018). To date, several m6A reader proteins have been identified in mammalian cellular extracts using affinity chromatography combined with mass spectrometry (Dominissini et al., 2012). Among the “reader” proteins, the YT521-B homology (YTH) family of proteins have been very well studied (Meyer and Jaffrey, 2017; Patil et al., 2018). There are three YTHDF (YTH domain family) members localized in the cytoplasm, including YTHDF1, YTHDF2, and YTHDF3 (Dominissini et al., 2012; Wang X. et al., 2014; Wang X. et al., 2015; Shi et al., 2017), and two YTHDC (YTH domain containing) proteins, YTHDC1 located in the nucleus (Xu et al., 2014) and YTHDC2 located in the cytoplasm (Morohashi et al., 2011). YTHDF proteins contain a conserved YTH RNA-binding domain that is inclined to bind the m6A-targeted RNAs and a N/P/Q-rich region that is correlated with different RNA-protein complexes (Fu et al., 2014). YTHDF1 has been demonstrated to interact with the translation initiation machinery and enhance the translational efficiency of its mRNA targets (Wang X. et al., 2015). The function of YTHDF2 was the induction of mRNA degradation (Wang X. et al., 2014). It has been shown that YTHDF2 accelerates the degradation of m6A-containing RNAs by directly recruiting the CCR4-NOT deadenylase complex (Du et al., 2016). YTHDF3 has been shown to promote the function of both YTHDF1 and YTHDF2. When it cooperates with YTHDF1, YTHDF3 can favor mRNA translation by the interactions with some ribosomal proteins (Li et al., 2017; Shi et al., 2017). Moreover, when it is associated with YTHDF2, YTHDF3 could participate in mRNA decay through direct relations with YTHDF2 (Shi et al., 2017). The nuclear m6A reader YTHDC1 was demonstrated to promote exon inclusion by assisting the splicing factor SRSF3 during its recruitment, while blocking the binding of SRSF10 (Kasowitz et al., 2018). Another study in HeLa cells showed that the association of YTHDC1 with SRSF3 and NXF1 could promote the nuclear export of m6A-target mRNAs (Roundtree et al., 2017b). YTHDC2, was identified to favor translational efficiency while decreasing the abundance of m6A-containing mRNAs (Hsu et al., 2017). YTHDC2 is a relatively large protein molecule (∼160 kDa) and contains many helicase domains and two ankyrin repeats. These special structural features might allow YTHDC2 to possess multiple functions, including regulating effects on RNA binding and RNA structure, and binding with or the recruitment of other interacting proteins (Tanabe et al., 2016; Hsu et al., 2017). A study reported that YTHDC2 lacked the m6A binding activity in HEK cells (Patil et al., 2016, 2018). This finding suggests that YTHDC2 might have indirect effects in regulating m6A-modified RNAs through interaction with other factors. In addition to the YTH family members, there are other proteins that have been identified to recognize and bind to m6A. The eukaryotic initiation factor 3 (eIF3) complex, interacts with m6A-containing 5′UTR through a multisubunit interface to directly recruit the 40S preinitiation complex to the 5′UTR of target-mRNAs to stimulate translation initiation (Meyer et al., 2015). hnRNPA2/B1 was shown to bind m6A-modified RNAs to regulate splicing and microRNA maturation (Alarcon et al., 2015a). Fragile X Mental Retardation Protein (FMRP) was also demonstrated to bind m6A-containing transcripts in a sequence context-dependent manner (Edupuganti et al., 2017). Recently, insulin growth factor 2 binding proteins, IGF2BP 1, 2, and 3, were shown to promote stability and storage of mRNAs through binding target m6A mRNAs under normal and stress conditions (Huang et al., 2018).
Furthermore, researchers have found a new m6A-regulated protein, referred to as m6A-repelled proteins or anti-readers, during the study of proteomics that interact with m6A. These researchers found that m6A disrupts RNA binding by the stress granule proteins G3BP1/2, CAPRIN1, USP10 and RBM42 (Arguello et al., 2017). A study has confirmed that the presence of m6A in mRNA could decrease mRNA stability by interfering with the binding of G3BP1 and G3BP2 (Edupuganti et al., 2017). This phenomenon reveals an additional function of m6A in RNA metabolism. We provide a picture (Figure 1) as an overview of the associated machinery and molecular functions of m6A.
Figure 1. Related mechanisms and functions of m6A modification in mRNAs. The m6A modification is regulated by the “writers,” “erasers,” “readers” and “anti-readers.” Writers are composed of METTL3, METTL14, WTAP, KIAA1429, ZC3H13, RBM15, and METTL16, which have been reported to induce m6A RNA methylation. Erasers are m6A demethylases including FTO and ALKBH5. Readers are proteins that bind to m6A modified mRNAs and play corresponding roles. Those proteins that have been identified as readers to date include YTHDF1, YTHDF2, YTHDF3, YTHDC1, YTHDC2, eIF3, IGF2BP1, IGF2BP2, IGF2BP3, FMRP, and hnRNPA2/B1. The functions of m6A are related to almost all stages in deciding the fate of mRNAs including pre-mRNA splicing, pri-miRNA processing, mRNA export, mRNA stability, translation modulation and mRNA degradation. Anti-readers are proteins that preferentially bind to mRNAs in the absence of m6A, such as G3BP1/2, CAPRIN1, USP10, and RBM42.
The specific functions and mechanisms of m6A in RNA viruses are not well-known, although m6A has long been known to be present in some RNA viruses, such as IAV (Krug et al., 1976), RSV (Kane and Beemon, 1985), B77 avian sarcoma virus (Stoltzfus and Dimock, 1976; Dimock and Stoltzfus, 1977) and feline leukemia virus (Thomason et al., 1976). In recent years, technological advances have made m6A a focus of research in elucidating the role of this RNA modification in viral epitranscriptomics. Herein we summarize and describe the role of m6A in RNA viruses including IAV, HIV-1, HCV, ZIKV, VSV, and EV71.
As the first virus found to express mRNAs bearing internal m6A groups, Influenza A virus (IAV) was mapped for the sites of m6A by Courtney et al. in both the IAV mRNA and vRNA strands, and it was demonstrated that m6A modification increases viral RNA expression in cis (Courtney et al., 2017). Those authors used two methods to inhibit m6A modification in A549 cells infected by IAV. One method used a non-toxic dose of DAA treatment (an inhibitor of m6A addition), and the other used knockout of METTL3 through gene editing with CRISPR/Cas. The results showed that both methods could reduce the expression of the IAV proteins NS1 and M2. However, when the m6A reader YTHDF2 was ectopically overexpressed, increased IAV replication and infectious particle production were found. Moreover, they used synonymous mutations to remove m6A on both strands of the hemagglutinin (HA) segment, and found that IAV HA m6A mutants revealed reduced pathogenicity in mice. The findings confirmed that the addition of m6A residues in IAV transcripts could enhance viral gene expression.
Prior to this review, four reports have studied the involvement of m6A in HIV-1 infection. Three excellent reviews comprehensively compared and summarized the functions and mechanisms of m6A modification in the HIV-1 life cycle (Gonzales-van Horn and Sarnow, 2017; Riquelme-Barrios et al., 2018; Tan and Gao, 2018). Despite some agreed-upon conclusions, there are unconformities in the locations, effects, and mechanisms of m6A in HIV-1 RNA in these studies. Herein, a table was created to allow readers to understand functions of m6A more clearly and intuitively in the four different articles (Table 2).
RNA-based regulation of HCV plays an essential role in its infection (Wang X. et al., 2012), for example the liver-specific microRNA 122 has been proved to facilitate replication of the viral RNA (Jopling et al., 2005; Cuypers et al., 2016). Recently, Gokhale et al. carried out m6A analyses in cells infected with HCV and demonstrated that the HCV RNA genome was modified by m6A (Gokhale et al., 2016). The abundance of HCV NS5A protein was significantly increased through siRNA mediated simultaneous depletion of m6A methyltransferases METTL3 and METTL14 in Huh7 cells infected with HCV. In contrast, HCV NS5A levels were decreased when the m6A demethylase FTO was depleted. Considering that CD81 is an essential entry factor but has no effects on other steps of HCV’S life cycle (Zhang et al., 2004), Huh7.5 CD81 knockout (KO) cells were used to test whether the m6A machinery affects viral RNA replication or viral particle production. The results indicated that the m6A machinery modulated HCV particle production but did not regulate HCV translation or RNA replication. Furthermore, the authors found that YTHDF proteins relocalized at viral assembly sites to retain HCV RNA with m6A, leading to reduced HCV particle production. To further confirm this conclusion, mutations in the E1 gene of HCV to inactivate m6A modification were constructed marked as HCV-E1mut. Like the above findings, these mutations increased viral particle assembly. In brief, this article revealed that m6A, as a conserved regulatory mark across the HCV genome, negatively regulates HCV infection.
Like HCV, ZIKV is also a member of the Flaviviridae family. To date, there has been only one research article that reports the relevance of ZIKV to m6A modification. Twelve discrete m6A peaks were identified spanning the full length of ZIKV RNA, among which half were present in the region encoding the NS5 protein and the 3′ UTR region (Lichinchi et al., 2016b). Like the role of m6A in regulating the HCV life cycle, the knockdown of host methyltransferases METTL3 and METTL14 increased ZIKV production while the silencing of demethylases ALKBH5 and FTO suppressed ZIKV production. The difference was that the m6A machinery decreased HCV production by affecting the assembly of virus, yet m6A modification inhibited ZIKV production by impacting replication of ZIKV. Furthermore, YTHDF family proteins bound to ZIKV RNA and decreased the viral titer. Remarkably, YTHDF2 showed the strongest effect compared with YTHDF1 and YTHDF3. In total, these results identified that the m6A methylome was a new mechanism by which ZIKV interacted with the host cells and inhibited viral infection.
Because of its relatively simple structure and high replication capacity, VSV has been widely used in the study of innate immunity. After VSV infection, pattern-recognition receptors trigger activation of the type I interferon signaling pathway (Schlee and Hartmann, 2016). Since m6A is involved in regulating the life cycles of many viruses, whether m6A modification participates in the regulation of VSV infection is a question. The Cao group demonstrated in 2017 that knockdown of the m6A eraser ALKBH5 noticeably increased the production of type I interferons triggered by VSV infection (Zheng et al., 2017). This finding suggested that m6A modifications might play a negative role in the life cycle of VSV. To investigate the detailed mechanisms related to m6A in regulating the antiviral effects, the authors performed numerous biological experiments. They found that nuclear DDX member DDX46 recruited ALKBH5 via DDX46’s DEAD helicase domain to erase the m6A modification of three important antiviral transcripts MAVS, TRAF3, and TRAF6 and therefore caused their retention in the nucleus and reduced their translation into protein; this result further caused decreased production of type I interferons. In effect, elimination of the m6A modification suppressed the exportation of mRNA from the nucleus and induced retention of the mRNA encoding proteins in the nucleus such as those involved in circadian rhythm (Fustin et al., 2013; Zheng et al., 2013); this phenomenon was well verified in this article. This work might promote research progress in m6A-modification-based regulation of gene expression in innate immunity.
As a single-stranded RNA virus which belongs to the genus Enterovirus within the family Picornaviridae, Enterovirus 71 (EV71) is one of the main pathogens that causes hand-foot-and-mouth disease (HFMD) (Wang and Li, 2018). Lately, a study reported that EV71 RNA contained m6A residues and the expression and localization of the m6A methyltransferase and demethylase were altered during viral infection (Hao et al., 2019). The genomic copy numbers of EV71 RNA were significantly decreased by silencing METTL3 gene and increased by FTO gene depletion. When two m6A sites were mutated in the EV71 RNA, the virus titer was significantly decreased. Besides, the authors found that METTL3 interacted with viral RdRp 3D protein and overexpression of METTL3 induced enhanced sumoylation and ubiquitination of 3D. As it has been reported that sumoylation and ubiquitination levels can enhance self-stability of 3D and facilitate EV71 replication (Liu et al., 2016), this suggested us that the replication of EV71 were also influenced through METTL3-mediated sumoylation and ubiquitination of viral 3D protein. Collectively, these results suggest that m6A modifications in EV71 RNA played a positive role in viral replication.
DNA viruses use the host machinery to replicate in the nucleus, are likely to usurp m6A machinery to regulate their lifecycle, and have been reported to have m6A modifications in viral mRNAs such as Kaposi sarcoma–associated herpesvirus (KSHV), Simian virus 40 (SV40) and Hepatitis B virus (HBV) (Ye et al., 2017; Hesser et al., 2018; Imam et al., 2018; Tsai et al., 2018).
Kaposi’s sarcoma-associated herpesvirus (KSHV) is a carcinogenic virus associated with a variety of malignant tumors including Kaposi’s sarcoma (KS), primary effusion lymphoma (PEL), and multicentric Castleman’s disease (MCD) (Chang et al., 1994; Cesarman et al., 1995; Soulier et al., 1995). Like all herpesviruses, KSHV has two phases of the lifecycle, latent infection and lytic replication (Zhao et al., 2015; Liu et al., 2017). During KSHV latent infection, most of the viral genome is suppressed through DNA methylation, repressive histone modifications and other regulatory mechanisms which negatively regulate gene expression (Pantry and Medveczky, 2009; Gunther and Grundhoff, 2010; Lu et al., 2010; Toth et al., 2010; Purushothaman et al., 2015). Changes in the host cell microenvironment could reactivate the virus from latency to the lytic cycle, wherein the inhibitory epigenetic marks are replaced by active ones to allow for transcription of viral lytic genes (Ye, 2017; Uppal et al., 2018). Three recent studies revealed that the KSHV life cycle was affected by RNA N6-adenosine methylation epigenetic modification (Ye et al., 2017; Hesser et al., 2018; Tan et al., 2018). In the first report, the authors found that most KSHV transcripts undergo m6A modifications by experiments of MeRIP combined with qRT-PCR (Ye et al., 2017). Knockdown (KD) or functional inhibition of FTO using a reagent, named meclofenamic acid (MA), could enhance the expression of lytic gene ORF50 and ORF57, while KD of METTL3 or the blocking of m6A could abolish the expression of the two lytic genes and virion production. The splicing of pre-mRNA of ORF50, a key KSHV lytic switch gene, was inhibited when m6A deposition was blocked. Further research suggested that m6A positively regulates ORF50 (RTA) RNA splicing through binding of the YTH domain containing 1 (YTHDC1) to identified m6A sites in RTA pre-mRNA and the cooperation with serine/arginine-rich splicing factor 3 (SRSF3) and SRSF10. RTA itself could induce m6A decoration and enhance its own pre-mRNA splicing. In short, m6A marks in the KSHV genome promoted lytic replication. In the second report, the KSHV-positive renal carcinoma cell line iSLK.219 during lytic reactivation went through transcriptome-wide m6A-sequencing, and the results revealed that the m6A modification was present across most viral transcripts. Depletion of the m6A writer METTL3 and the reader YTHDF2 significantly impaired virion production in iSLK.219 and iSLK.BAC16 cells, suggesting that the m6A pathway functioned in a pro-viral manner. In contrast, ORF50 protein expression was increased upon depletion of METTL3 in KSHV infected B cells, reflecting the anti-viral impacts of m6A in the KSHV life cycle (Hesser et al., 2018). The second report emphasized the result that the m6A pathway might play different roles in promoting or inhibiting viral gene expression depending on the cell-type analyzed. The last report found that KSHV transcripts contain abundant and highly conserved m6A modifications among different cell types and infection systems. The m6A reader protein YTHDF2 inhibited KSHV lytic replication by facilitating the degradation of viral lytic transcripts which is consistent with the second report. The lytic replication period of KSHV induced dynamic reprogramming of the epitranscriptome, regulating relevant pathways that control lytic replication (Tan et al., 2018). This report provided insights into the mechanism of KSHV-induced disease by helping us understand the changes of viral and cellular m6A modifications during KSHV latent and lytic infection. The three articles together suggest that m6A modifications in KSHV might play a positive role through different mechanisms or play different roles owing to different cell types during lytic replication.
As a member of the polyomavirus family, the gene expression of simian virus 40 (SV40) is regulated in an early phase, encoding the viral regulatory proteins, and a late phase, encoding the viral structural proteins. In 1979, SV40 was reported to contain some m6A groups in transcripts in the “late” region of the virus (Canaani et al., 1979). However, the specific location of these m6A groups was not identified and their functional roles have remained unclear. Recently, a report demonstrated that the overexpression of YTHDF2 induced faster viral replication, and larger viral plaques in BSC40 cells infected with SV40, whereas mutational inactivation of the endogenous YTHDF2 gene, or the m6A writer METTL3, had the contrary effect, suggesting a positive influence for m6A modification in the regulation of the SV40 life cycle (Tsai et al., 2018). The authors also mapped the sites of m6A residues on SV40 transcripts and identified two m6A sites on the viral early transcripts and eleven m6A sites within the SV40 late region. The authors observed that the mutant virus replicated more slowly than wild type SV40 when they inactivated most of the m6A addition sites on the SV40 late mRNAs using synonymous mutations. Together, these results suggest that the addition of m6A residues to the late SV40 transcripts played a positive role in viral gene expression and replication.
Hepatitis B virus infection is the leading cause of chronic hepatitis (Fu et al., 2016) and plays a role in the development of cirrhosis (Zhao et al., 2011) and hepatocellular carcinoma (Chen J. et al., 2009; Chen L. et al., 2009; Levrero et al., 2009). Imam et al. (2018) reported that HBV transcripts detected from both liver tissues of chronic HBV patients and HBV-expressing cells contain m6A. They observed that m6A located in HBV 3′UTRs reduced the stability of these RNAs, ultimately affecting the expression of their corresponding proteins. YTHDF proteins were found to bind HBV transcripts, and the depletion of them increased HBV protein expression, likely by changing the stability of the HBV transcript. Similar effects were observed when the m6A site within the 3′ epsilon loop of all HBV transcripts was inactivated. However, the authors found that m6A at the 5′epsilon loop, which is present only in pregenomic RNA (pgRNA), positively regulates pgRNA reverse transcription. Therefore, m6A regulates HBV RNAs in more than one way, hinging on its position in the RNA. The roles of m6A in different viral infections are summarized in Table 3.
The addition of m6A in viral mRNAs has both pro-viral and antiviral functions in distinct viral life cycles (He et al., 2011). The m6A modification enhanced the translation of viral late transcripts in SV40 while decreasing infectious HCV particle production (Gokhale et al., 2016; Tsai et al., 2018). Different reports studying the m6A effects in the same virus indicated that there exist discrepancies in the distribution of m6A sites and roles of m6A in the viral life cycle such as the abovementioned studies focusing on HIV-1, KSHV and HBV. The reasons for the contradictory results remain unclear in this field. One reason is the suggestion that m6A machinery functions in a cell type specific manner to either promote or inhibit KSHV gene expression (Hesser et al., 2018). The use of different cell types to study the m6A modification is one of the reasons for the different results. Some other reasons might contribute to different results obtained. First, the m6A decoration itself is dynamic in controlling mRNA fate and its ability to impact virtually every stage of host gene expression (Gonzales-van Horn and Sarnow, 2017; Roundtree et al., 2017a). Therefore, different experimental results are likely to result from different time points of sampling or different viral infection times. Second, the intracellular localizations of m6A modified by viral RNAs might influence the availability of host proteins, which might further result in different functions of m6A in different viruses. Third, the distinct m6A distribution sites of HIV-1 might be attributed to the sequencing-based methodologies used by each report which had different resolutions. For example, neither the m6A-seq nor the PA-m6A-seq can discriminate between m6A and m6Am (Tan and Gao, 2018), therefore leading to comprehensive distribution of the two types of modification when using the abovementioned m6A transcriptome-wide profiling techniques. Finally, the binding sites of the three m6A reader proteins YTHDF1, YTHDF2, and YTHDF3 identified by CLIP-seq analysis were different in the reports about HIV-1 (Ao et al., 2016; Riquelme-Barrios et al., 2018). Certain binding sites for YTHDF1, 2, and 3 do not line up with predicted m6A sites (Riquelme-Barrios et al., 2018), suggesting that cytoplasmic readers would also bind the HIV-1 gRNAs in an m6A-independent manner or that the viral transcripts possess additional m6A sites that have not yet been mapped. In general, the different approaches, detection strategies, cell lines and other reagents used in m6A-related studies might contribute to the distinct results obtained, which deserve to be clarified in the future.
Given that the presence of m6A participates in the life cycles of multiple viruses, drugs that target this pathway could have the potential to be used to fight a range of viral illnesses. A drug, 3-deazaadenosine (DAA), which inhibits m6A addition through depleting intracellular levels of the methyl donor SAM (Bader et al., 1978; Fustin et al., 2013), is a potent inhibitor of IAV replication at doses that do not exert any apparent cytotoxic effects (Fischer et al., 1990). DAA has been reported to suppress a wide variety of viruses, not only in vitro culture but also in vivo in mice and in rats (Bader et al., 1978; Wyde et al., 1990; Bray et al., 2000; Kennedy et al., 2016; Courtney et al., 2017). In addition, a reagent, known as meclofenamic acid (MA), which can inhibit FTO demethylation by competition on m6A-containing substrate binding, has been demonstrated to enhance the expression of KSHV lytic genes (Ye et al., 2017). It is not difficult to conclude that developing new small molecule inhibitors or drugs targeting m6A “writers” or “erasers” might prove to be of great significance for antiviral therapies.
All authors listed have made a substantial, direct and intellectual contribution to the work, and approved it for publication. WD analyzed the literatures and studies and wrote the manuscript. JL designed this review and revised the manuscript. The rest of the authors assisted with the process of writing the manuscript.
This work was supported by the National Key Research and Development Program of China (2017YFC1200204), the National Natural Science Foundations of China (31670171 and 81728011).
The authors declare that the research was conducted in the absence of any commercial or financial relationships that could be construed as a potential conflict of interest.
ALKBH5, AlkB Homolog 5, RNA Demethylase; CAPRIN1, cytoplasmic activation- and proliferation-associated protein 1; CLIP-seq, crosslinking-immunprecipitation and high-throughput sequencing; DENV, Dengue virus; eIF3, Eukaryotic Translation Initiation Factor 3; FMRP, Fragile X Mental Retardation Protein 1; FTO, fat mass and obesity-associated protein; G3BP1/2, GTPase Activating Protein (SH3 Domain) Binding Protein 1; HCV, hepatitis C virus; hnRNPA2/B1, heterogeneous nuclear ribonucleoprotein A2/B1; IGF2BP1, Insulin Like Growth Factor 2 MRNA Binding Protein 1; KIAA1429, Vir Like m6A methyltransferase associated protein; LC-MS, liquid chromatography-mass spectrometry; m6A, N6-methyladenosine; m6A-LAIC-seq, m6A level and isoform characterization sequencing; m6Am, 2-O-dimethyladenosine; m6A-seq, N6-methyladenosine-sequencing; MAVS, mitochondrial antiviral signaling protein; MeRIP-seq, methylated RNA immunoprecipitation sequening; METTL14, Methyltransferase Like 14; METTL16, Methyltransferase Like 16; METTL3, Methyltransferase Like 3; miCLIP, m6A individual nucleotide resolution cross-linking and immunoprecipitation; NXF1, nuclear RNA export factor 1; PA-m6A-seq, photo-cross-linking-assisted m6A sequencing; PAR-CLIP, photoactivatable ribonucleoside-enhanced crosslinking and immunoprecipitation; RBM15, RNA Binding Motif Protein 15; RBM42, RNA Binding Motif Protein 42; RSV, Rous sarcoma virus; SAM, S-adenosylmethionine; SRSF10, Serine and Arginine Rich Splicing Factor 10; SRSF3, Splicing Factor, Arginine/Serine-Rich 3; TRAF3, TNF Receptor Associated Factor 3; TRAF6, TNF Receptor Associated Factor 6; USP10, Ubiquitin-Specific-Processing Protease 10; VSV, vesicular stomatitis virus; WNV, West Nile virus; WTAP, WT1 associated protein; YFV, yellow fever virus; YTHDC1, YTH Domain-Containing Protein 1; YTHDC2, YTH Domain-Containing Protein 2; YTHDF1, YTH N(6)-Methyladenosine RNA Binding Protein 1; YTHDF2, YTH N(6)-Methyladenosine RNA Binding Protein 2; YTHDF3, YTH N(6)-Methyladenosine RNA Binding Protein 3; ZC3H13, Zinc Finger CCCH Domain-Containing Protein 13; ZIKV, Zika virus.
Adams, J. M., and Cory, S. (1975). Modified nucleosides and bizarre 5’-termini in mouse myeloma mRNA. Nature 255, 28–33.
Alarcon, C. R., Goodarzi, H., Lee, H., Liu, X., Tavazoie, S., and Tavazoie, S. F. (2015a). HNRNPA2B1 is a mediator of m(6)A-dependent nuclear RNA processing events. Cell 162, 1299–1308. doi: 10.1016/j.cell.2015.08.011
Alarcon, C. R., Lee, H., Goodarzi, H., Halberg, N., and Tavazoie, S. F. (2015b). N6-methyladenosine marks primary microRNAs for processing. Nature 519, 482–485. doi: 10.1038/nature14281
Ao, Z., Zhu, R., Tan, X., Liu, L., Chen, L., Liu, S., et al. (2016). Activation of HIV-1 expression in latently infected CD4+ T cells by the small molecule PKC412. Virol. J. 13:177. doi: 10.1186/s12985-016-0637-9
Arguello, A. E., DeLiberto, A. N., and Kleiner, R. E. (2017). RNA chemical proteomics reveals the N(6)-methyladenosine (m(6)A)-regulated protein-RNA interactome. J. Am. Chem. Soc. 139, 17249–17252. doi: 10.1021/jacs.7b09213
Bader, J. P., Brown, N. R., Chiang, P. K., and Cantoni, G. L. (1978). 3-Deazaadenosine, an inhibitor of adenosylhomocysteine hydrolase, inhibits reproduction of Rous sarcoma virus and transformation of chick embryo cells. Virology 89, 494–505.
Batista, P. J. (2017). The RNA modification N(6)-methyladenosine and its implications in human disease. Genomics Proteomics Bioinformatics 15, 154–163. doi: 10.1016/j.gpb.2017.03.002
Bray, M., Driscoll, J., and Huggins, J. W. (2000). Treatment of lethal Ebola virus infection in mice with a single dose of an S-adenosyl-L-homocysteine hydrolase inhibitor. Antiviral Res. 45, 135–147.
Bujnicki, J. M., Feder, M., Radlinska, M., and Blumenthal, R. M. (2002). Structure prediction and phylogenetic analysis of a functionally diverse family of proteins homologous to the MT-A70 subunit of the human mRNA: m(6)A methyltransferase. J. Mol. Evol. 55, 431–444. doi: 10.1007/s00239-002-2339-8
Canaani, D., Kahana, C., Lavi, S., and Groner, Y. (1979). Identification and mapping of N6-methyladenosine containing sequences in simian virus 40 RNA. Nucleic Acids Res. 6, 2879–2899.
Cao, G., Li, H. B., Yin, Z., and Flavell, R. A. (2016). Recent advances in dynamic m6A RNA modification. Open Biol. 6:160003. doi: 10.1098/rsob.160003
Cesarman, E., Chang, Y., Moore, P. S., Said, J. W., and Knowles, D. M. (1995). Kaposi’s sarcoma-associated herpesvirus-like DNA sequences in AIDS-related body-cavity-based lymphomas. N. Engl. J. Med. 332, 1186–1191. doi: 10.1056/NEJM199505043321802
Chang, Y., Cesarman, E., Pessin, M. S., Lee, F., Culpepper, J., Knowles, D. M., et al. (1994). Identification of herpesvirus-like DNA sequences in AIDS-associated Kaposi’s sarcoma. Science 266, 1865–1869.
Chen, J., Han, J. H., Liu, C., Yu, R. H., Li, F. Z., Li, Q. F., et al. (2009). Short-term entecavir therapy of chronic severe hepatitis B. Hepatobiliary Pancreat. Dis. Int. 8, 261–266.
Chen, K., Lu, Z., Wang, X., Fu, Y., Luo, G. Z., Liu, N., et al. (2015). High-resolution N(6) -methyladenosine (m(6) A) map using photo-crosslinking-assisted m(6) A sequencing. Angew. Chem. Int. Ed. Engl. 54, 1587–1590. doi: 10.1002/anie.201410647
Chen, L., Wu, C., Fan, X., Gao, J., Yin, H., Wang, T., et al. (2009). Replication and infectivity of hepatitis B virus in HBV-related glomerulonephritis. Int. J. Infect. Dis. 13, 394–398. doi: 10.1016/j.ijid.2008.08.014
Chen, T., Hao, Y. J., Zhang, Y., Li, M. M., Wang, M., Han, W., et al. (2015). m(6)A RNA methylation is regulated by microRNAs and promotes reprogramming to pluripotency. Cell Stem Cell 16, 289–301. doi: 10.1016/j.stem.2015.01.016
Chen, W., Tran, H., Liang, Z., Lin, H., and Zhang, L. (2015). Identification and analysis of the N(6)-methyladenosine in the Saccharomyces cerevisiae transcriptome. Sci. Rep. 5:13859. doi: 10.1038/srep13859
Courtney, D. G., Kennedy, E. M., Dumm, R. E., Bogerd, H. P., Tsai, K., Heaton, N. S., et al. (2017). Epitranscriptomic enhancement of influenza a virus gene expression and replication. Cell Host Microbe 22, 377–386.e5. doi: 10.1016/j.chom.2017.08.004
Cuypers, L., Ceccherini-Silberstein, F., Van Laethem, K., Li, G., Vandamme, A. M., and Rockstroh, J. K. (2016). Impact of HCV genotype on treatment regimens and drug resistance: a snapshot in time. Rev. Med. Virol. 26, 408–434. doi: 10.1002/rmv.1895
Desrosiers, R., Friderici, K., and Rottman, F. (1974). Identification of methylated nucleosides in messenger RNA from Novikoff hepatoma cells. Proc. Natl. Acad. Sci. U.S.A. 71, 3971–3975.
Desrosiers, R. C., Friderici, K. H., and Rottman, F. M. (1975). Characterization of Novikoff hepatoma mRNA methylation and heterogeneity in the methylated 5’ terminus. Biochemistry 14, 4367–4374.
Dimock, K., and Stoltzfus, C. M. (1977). Sequence specificity of internal methylation in B77 avian sarcoma virus RNA subunits. Biochemistry 16, 471–478.
Dominissini, D., Moshitch-Moshkovitz, S., Schwartz, S., Salmon-Divon, M., Ungar, L., Osenberg, S., et al. (2012). Topology of the human and mouse m6A RNA methylomes revealed by m6A-seq. Nature 485, 201–206. doi: 10.1038/nature11112
Du, H., Zhao, Y., He, J., Zhang, Y., Xi, H., Liu, M., et al. (2016). YTHDF2 destabilizes m(6)A-containing RNA through direct recruitment of the CCR4-NOT deadenylase complex. Nat. Commun. 7:12626. doi: 10.1038/ncomms12626
Edupuganti, R. R., Geiger, S., Lindeboom, R. G. H., Shi, H., Hsu, P. J., Lu, Z., et al. (2017). N(6)-methyladenosine (m(6)A) recruits and repels proteins to regulate mRNA homeostasis. Nat. Struct. Mol. Biol. 24, 870–878. doi: 10.1038/nsmb.3462
Fischer, A. A., Muller, K., and Scholtissek, C. (1990). Specific inhibition of the synthesis of influenza virus late proteins and stimulation of early, M2, and NS2 protein synthesis by 3-deazaadenosine. Virology 177, 523–531.
Fu, X., Tan, D., Dou, X., Chen, J., and Wu, J. (2016). A multi-center clinical study comparing Sansure Magb and CAP/CTM HBV tests in the quantitative detection of HBV DNA. J. Infect. Dev. Ctries. 10, 755–761. doi: 10.3855/jidc.7112
Fu, Y., Dominissini, D., Rechavi, G., and He, C. (2014). Gene expression regulation mediated through reversible m(6)A RNA methylation. Nat. Rev. Genet. 15, 293–306. doi: 10.1038/nrg3724
Fustin, J. M., Doi, M., Yamaguchi, Y., Hida, H., Nishimura, S., Yoshida, M., et al. (2013). RNA-methylation-dependent RNA processing controls the speed of the circadian clock. Cell 155, 793–806. doi: 10.1016/j.cell.2013.10.026
Gokhale, N. S., and Horner, S. M. (2017). RNA modifications go viral. PLoS Pathog. 13:e1006188. doi: 10.1371/journal.ppat.1006188
Gokhale, N. S., McIntyre, A. B. R., McFadden, M. J., Roder, A. E., Kennedy, E. M., Gandara, J. A., et al. (2016). N6-methyladenosine in flaviviridae viral RNA genomes regulates infection. Cell Host Microbe 20, 654–665. doi: 10.1016/j.chom.2016.09.015
Gonzales-van Horn, S. R., and Sarnow, P. (2017). Making the mark: the role of adenosine modifications in the life cycle of RNA viruses. Cell Host Microbe 21, 661–669. doi: 10.1016/j.chom.2017.05.008
Gunther, T., and Grundhoff, A. (2010). The epigenetic landscape of latent Kaposi sarcoma-associated herpesvirus genomes. PLoS Pathog. 6:e1000935. doi: 10.1371/journal.ppat.1000935
Guo, J., Tang, H. W., Li, J., and Yan, D. (2018). Xio is a component of the Drosophila sex determination pathway and RNA N(6)-methyladenosine methyltransferase complex. Proc. Natl. Acad. Sci. U.S.A. 115, 3674–3679. doi: 10.1073/pnas.1720945115
Hao, H., Hao, S., Chen, H., Chen, Z., Zhang, Y., Wang, J., et al. (2019). N6-methyladenosine modification and METTL3 modulate Enterovirus 71 replication. Nucleic Acids Res. 47, 362–374. doi: 10.1093/nar/gky1007
He, C. (2010). Grand challenge commentary: RNA epigenetics? Nat. Chem. Biol. 6, 863–865. doi: 10.1038/nchembio.482
He, M., Zheng, Y. H., Zhou, H. Y., Mamadou, D., Chen, Z., Chen, X., et al. (2011). Prospective observation for seven-year’s highly active antiretroviral therapy in Chinese HIV-1 infected patients. Curr. HIV Res. 9, 160–165.
Hesser, C. R., Karijolich, J., Dominissini, D., He, C., and Glaunsinger, B. A. (2018). N6-methyladenosine modification and the YTHDF2 reader protein play cell type specific roles in lytic viral gene expression during Kaposi’s sarcoma-associated herpesvirus infection. PLoS Pathog. 14:e1006995. doi: 10.1371/journal.ppat.1006995
Hsu, P. J., Zhu, Y., Ma, H., Guo, Y., Shi, X., Liu, Y., et al. (2017). Ythdc2 is an N(6)-methyladenosine binding protein that regulates mammalian spermatogenesis. Cell Res. 27, 1115–1127. doi: 10.1038/cr.2017.99
Huang, H., Weng, H., Sun, W., Qin, X., Shi, H., Wu, H., et al. (2018). Recognition of RNA N(6)-methyladenosine by IGF2BP proteins enhances mRNA stability and translation. Nat. Cell Biol. 20, 285–295. doi: 10.1038/s41556-018-0045-z
Imam, H., Khan, M., Gokhale, N. S., McIntyre, A. B. R., Kim, G. W., Jang, J. Y., et al. (2018). N6-methyladenosine modification of hepatitis B virus RNA differentially regulates the viral life cycle. Proc. Natl. Acad. Sci. U.S.A. 115, 8829–8834. doi: 10.1073/pnas.1808319115
Jia, C. Z., Zhang, J. J., and Gu, W. Z. (2016). RNA-MethylPred: a high-accuracy predictor to identify N6-methyladenosine in RNA. Anal. Biochem. 510, 72–75. doi: 10.1016/j.ab.2016.06.012
Jia, G., Fu, Y., Zhao, X., Dai, Q., Zheng, G., Yang, Y., et al. (2011). N6-methyladenosine in nuclear RNA is a major substrate of the obesity-associated FTO. Nat. Chem. Biol. 7, 885–887. doi: 10.1038/nchembio.687
Jopling, C. L., Yi, M., Lancaster, A. M., Lemon, S. M., and Sarnow, P. (2005). Modulation of hepatitis C virus RNA abundance by a liver-specific MicroRNA. Science 309, 1577–1581. doi: 10.1126/science.1113329
Kan, L., Grozhik, A. V., Vedanayagam, J., Patil, D. P., Pang, N., Lim, K. S., et al. (2017). The m(6)A pathway facilitates sex determination in Drosophila. Nat. Commun. 8:15737. doi: 10.1038/ncomms15737
Kane, S. E., and Beemon, K. (1985). Precise localization of m6A in Rous sarcoma virus RNA reveals clustering of methylation sites: implications for RNA processing. Mol. Cell Biol. 5, 2298–2306.
Kasowitz, S. D., Ma, J., Anderson, S. J., Leu, N. A., Xu, Y., Gregory, B. D., et al. (2018). Nuclear m6A reader YTHDC1 regulates alternative polyadenylation and splicing during mouse oocyte development. PLoS Genet. 14:e1007412. doi: 10.1371/journal.pgen.1007412
Kennedy, E. M., Bogerd, H. P., Kornepati, A. V., Kang, D., Ghoshal, D., Marshall, J. B., et al. (2016). Posttranscriptional m(6)A editing of HIV-1 mRNAs enhances viral gene expression. Cell Host Microbe 19, 675–685. doi: 10.1016/j.chom.2016.04.002
Kennedy, E. M., Courtney, D. G., Tsai, K., and Cullen, B. R. (2017). Viral epitranscriptomics. J. Virol. 91:e02263-16. doi: 10.1128/JVI.02263-16
Knuckles, P., Lence, T., Haussmann, I. U., Jacob, D., Kreim, N., Carl, S. H., et al. (2018). Zc3h13/Flacc is required for adenosine methylation by bridging the mRNA-binding factor Rbm15/Spenito to the m(6)A machinery component Wtap/Fl(2)d. Genes Dev. 32, 415–429. doi: 10.1101/gad.309146.117
Kobayashi, M., Ohsugi, M., Sasako, T., Awazawa, M., Umehara, T., Iwane, A., et al. (2018). The RNA methyltransferase complex of WTAP, METTL3, and METTL14 regulates mitotic clonal expansion in adipogenesis. Mol. Cell Biol. 38:e00116-18. doi: 10.1128/MCB.00116-18
Krug, R. M., Morgan, M. A., and Shatkin, A. J. (1976). Influenza viral mRNA contains internal N6-methyladenosine and 5’-terminal 7-methylguanosine in cap structures. J. Virol. 20, 45–53.
Lavi, S., and Shatkin, A. J. (1975). Methylated simian virus 40-specific RNA from nuclei and cytoplasm of infected BSC-1 cells. Proc. Natl. Acad. Sci. U.S.A. 72, 2012–2016.
Levrero, M., Pollicino, T., Petersen, J., Belloni, L., Raimondo, G., and Dandri, M. (2009). Control of cccDNA function in hepatitis B virus infection. J. Hepatol. 51, 581–592. doi: 10.1016/j.jhep.2009.05.022
Li, A., Chen, Y. S., Ping, X. L., Yang, X., Xiao, W., Yang, Y., et al. (2017). Cytoplasmic m(6)A reader YTHDF3 promotes mRNA translation. Cell Res. 27, 444–447. doi: 10.1038/cr.2017.10
Li, S., and Mason, C. E. (2014). The pivotal regulatory landscape of RNA modifications. Annu. Rev. Genomics Hum. Genet. 15, 127–150. doi: 10.1146/annurev-genom-090413-025405
Li, Y., Wang, Y., Zhang, Z., Zamudio, A. V., and Zhao, J. C. (2015). Genome-wide detection of high abundance N6-methyladenosine sites by microarray. RNA 21, 1511–1518. doi: 10.1261/rna.051474.115
Li, Z., Qian, P., Shao, W., Shi, H., He, X. C., Gogol, M., et al. (2018). Suppression of m(6)A reader YTHDF2 promotes hematopoietic stem cell expansion. Cell Res. 28, 904–917. doi: 10.1038/s41422-018-0072-0
Lichinchi, G., Gao, S., Saletore, Y., Gonzalez, G. M., Bansal, V., Wang, Y., et al. (2016a). Dynamics of the human and viral m(6)A RNA methylomes during HIV-1 infection of T cells. Nat. Microbiol. 1:16011. doi: 10.1038/nmicrobiol.2016.11
Lichinchi, G., Zhao, B. S., Wu, Y., Lu, Z., Qin, Y., He, C., et al. (2016b). Dynamics of human and viral RNA methylation during zika virus infection. Cell Host Microbe 20, 666–673. doi: 10.1016/j.chom.2016.10.002
Linder, B., Grozhik, A. V., Olarerin-George, A. O., Meydan, C., Mason, C. E., and Jaffrey, S. R. (2015). Single-nucleotide-resolution mapping of m6A and m6Am throughout the transcriptome. Nat. Methods 12, 767–772. doi: 10.1038/nmeth.3453
Liu, H., Wang, H., Wei, Z., Zhang, S., Hua, G., Zhang, S. W., et al. (2018). MeT-DB V2.0: elucidating context-specific functions of N6-methyl-adenosine methyltranscriptome. Nucleic Acids Res. 46, D281–D287. doi: 10.1093/nar/gkx1080
Liu, J., Yue, Y., Han, D., Wang, X., Fu, Y., Zhang, L., et al. (2014). A METTL3-METTL14 complex mediates mammalian nuclear RNA N6-adenosine methylation. Nat. Chem. Biol. 10, 93–95. doi: 10.1038/nchembio.1432
Liu, L., Zhou, Q., Xie, Y., Zuo, L., Zhu, F., and Lu, J. (2017). Extracellular vesicles: novel vehicles in herpesvirus infection. Virol. Sin. 32, 349–356. doi: 10.1007/s12250-017-4073-9
Liu, Y., Zheng, Z., Shu, B., Meng, J., Zhang, Y., Zheng, C., et al. (2016). SUMO modification stabilizes Enterovirus 71 polymerase 3D to facilitate viral replication. J. Virol. 90, 10472–10485. doi: 10.1128/JVI.01756-16
Lu, F., Stedman, W., Yousef, M., Renne, R., and Lieberman, P. M. (2010). Epigenetic regulation of Kaposi’s sarcoma-associated herpesvirus latency by virus-encoded microRNAs that target Rta and the cellular Rbl2-DNMT pathway. J. Virol. 84, 2697–2706. doi: 10.1128/JVI.01997-09
Lu, W., Tirumuru, N., St Gelais, C., Koneru, P. C., Liu, C., Kvaratskhelia, M., et al. (2018). N(6)-methyladenosine-binding proteins suppress HIV-1 infectivity and viral production. J. Biol. Chem. 293, 12992–13005. doi: 10.1074/jbc.RA118.004215
Mamadou, D., Yu-Huang, Z., Xia, C., Bo, H., Hua-Ying, Z., Yan, H., et al. (2013). HIV DNA and immune alteration during successful HAART. Curr. HIV Res. 11, 255–262.
Meyer, K. D., and Jaffrey, S. R. (2014). The dynamic epitranscriptome: N6-methyladenosine and gene expression control. Nat. Rev. Mol. Cell Biol. 15, 313–326. doi: 10.1038/nrm3785
Meyer, K. D., and Jaffrey, S. R. (2017). Rethinking m(6)A readers, writers, and erasers. Annu. Rev. Cell Dev. Biol. 33, 319–342. doi: 10.1146/annurev-cellbio-100616-060758
Meyer, K. D., Patil, D. P., Zhou, J., Zinoviev, A., Skabkin, M. A., Elemento, O., et al. (2015). 5’ UTR m(6)A promotes cap-independent translation. Cell 163, 999–1010. doi: 10.1016/j.cell.2015.10.012
Meyer, K. D., Saletore, Y., Zumbo, P., Elemento, O., Mason, C. E., and Jaffrey, S. R. (2012). Comprehensive analysis of mRNA methylation reveals enrichment in 3’ UTRs and near stop codons. Cell 149, 1635–1646. doi: 10.1016/j.cell.2012.05.003
Molinie, B., Wang, J., Lim, K. S., Hillebrand, R., Lu, Z. X., Van Wittenberghe, N., et al. (2016). m(6)A-LAIC-seq reveals the census and complexity of the m(6)A epitranscriptome. Nat. Methods 13, 692–698. doi: 10.1038/nmeth.3898
Morohashi, K., Sahara, H., Watashi, K., Iwabata, K., Sunoki, T., Kuramochi, K., et al. (2011). Cyclosporin A associated helicase-like protein facilitates the association of hepatitis C virus RNA polymerase with its cellular cyclophilin B. PLoS One 6:e18285. doi: 10.1371/journal.pone.0018285
Narayan, P., Ayers, D. F., Rottman, F. M., Maroney, P. A., and Nilsen, T. W. (1987). Unequal distribution of N6-methyladenosine in influenza virus mRNAs. Mol. Cell Biol. 7, 1572–1575.
Nilsen, T. W. (2014). Molecular biology. Internal mRNA methylation finally finds functions. Science 343, 1207–1208. doi: 10.1126/science.1249340
Pan, Y., Ma, P., Liu, Y., Li, W., and Shu, Y. (2018). Multiple functions of m(6)A RNA methylation in cancer. J. Hematol. Oncol. 11:48. doi: 10.1186/s13045-018-0590-8
Pantry, S. N., and Medveczky, P. G. (2009). Epigenetic regulation of Kaposi’s sarcoma-associated herpesvirus replication. Semin. Cancer Biol. 19, 153–157. doi: 10.1016/j.semcancer.2009.02.010
Patil, D. P., Chen, C. K., Pickering, B. F., Chow, A., Jackson, C., Guttman, M., et al. (2016). m(6)A RNA methylation promotes XIST-mediated transcriptional repression. Nature 537, 369–373. doi: 10.1038/nature19342
Patil, D. P., Pickering, B. F., and Jaffrey, S. R. (2018). Reading m(6)A in the transcriptome: m(6)A-binding proteins. Trends Cell Biol. 28, 113–127. doi: 10.1016/j.tcb.2017.10.001
Pendleton, K. E., Chen, B., Liu, K., Hunter, O. V., Xie, Y., Tu, B. P., et al. (2017). The U6 snRNA m(6)A methyltransferase METTL16 regulates SAM synthetase intron retention. Cell 169, 824–835.e14. doi: 10.1016/j.cell.2017.05.003
Ping, X. L., Sun, B. F., Wang, L., Xiao, W., Yang, X., Wang, W. J., et al. (2014). Mammalian WTAP is a regulatory subunit of the RNA N6-methyladenosine methyltransferase. Cell Res. 24, 177–189. doi: 10.1038/cr.2014.3
Purushothaman, P., Uppal, T., and Verma, S. C. (2015). Molecular biology of KSHV lytic reactivation. Viruses 7, 116–153. doi: 10.3390/v7010116
Riquelme-Barrios, S., Pereira-Montecinos, C., Valiente-Echeverria, F., and Soto-Rifo, R. (2018). Emerging roles of N(6)-methyladenosine on HIV-1 RNA metabolism and viral replication. Front. Microbiol. 9:576. doi: 10.3389/fmicb.2018.00576
Rottman, F. M., Bokar, J. A., Narayan, P., Shambaugh, M. E., and Ludwiczak, R. (1994). N6-adenosine methylation in mRNA: substrate specificity and enzyme complexity. Biochimie 76, 1109–1114.
Roundtree, I. A., Evans, M. E., Pan, T., and He, C. (2017a). Dynamic RNA modifications in gene expression regulation. Cell 169, 1187–1200. doi: 10.1016/j.cell.2017.05.045
Roundtree, I. A., Luo, G. Z., Zhang, Z., Wang, X., Zhou, T., Cui, Y., et al. (2017b). YTHDC1 mediates nuclear export of N(6)-methyladenosine methylated mRNAs. eLife 6:e31311. doi: 10.7554/eLife.31311
Schlee, M., and Hartmann, G. (2016). Discriminating self from non-self in nucleic acid sensing. Nat. Rev. Immunol. 16, 566–580. doi: 10.1038/nri.2016.78
Scholler, E., Weichmann, F., Treiber, T., Ringle, S., Treiber, N., Flatley, A., et al. (2018). Interactions, localization, and phosphorylation of the m(6)A generating METTL3-METTL14-WTAP complex. RNA 24, 499–512. doi: 10.1261/rna.064063.117
Schwartz, S., Mumbach, M. R., Jovanovic, M., Wang, T., Maciag, K., Bushkin, G. G., et al. (2014). Perturbation of m6A writers reveals two distinct classes of mRNA methylation at internal and 5’ sites. Cell Rep. 8, 284–296. doi: 10.1016/j.celrep.2014.05.048
Shi, H., Wang, X., Lu, Z., Zhao, B. S., Ma, H., Hsu, P. J., et al. (2017). YTHDF3 facilitates translation and decay of N(6)-methyladenosine-modified RNA. Cell Res. 27, 315–328. doi: 10.1038/cr.2017.15
Sledz, P., and Jinek, M. (2016). Structural insights into the molecular mechanism of the m(6)A writer complex. eLife 5:e18434. doi: 10.7554/eLife.18434
Sommer, S., Salditt-Georgieff, M., Bachenheimer, S., Darnell, J. E., Furuichi, Y., Morgan, M., et al. (1976). The methylation of adenovirus-specific nuclear and cytoplasmic RNA. Nucleic Acids Res. 3, 749–765.
Soulier, J., Grollet, L., Oksenhendler, E., Cacoub, P., Cazals-Hatem, D., Babinet, P., et al. (1995). Kaposi’s sarcoma-associated herpesvirus-like DNA sequences in multicentric Castleman’s disease. Blood 86, 1276–1280.
Stoltzfus, C. M., and Dimock, K. (1976). Evidence of methylation of B77 avian sarcoma virus genome RNA subunits. J. Virol. 18, 586–595.
Tan, B., and Gao, S. J. (2018). RNA epitranscriptomics: regulation of infection of RNA and DNA viruses by N(6) -methyladenosine (m(6) A). Rev. Med. Virol. 28:e1983. doi: 10.1002/rmv.1983
Tan, B., Liu, H., Zhang, S., da Silva, S. R., Zhang, L., Meng, J., et al. (2018). Viral and cellular N(6)-methyladenosine and N(6),2’-O-dimethyladenosine epitranscriptomes in the KSHV life cycle. Nat. Microbiol. 3, 108–120. doi: 10.1038/s41564-017-0056-8
Tanabe, A., Tanikawa, K., Tsunetomi, M., Takai, K., Ikeda, H., Konno, J., et al. (2016). RNA helicase YTHDC2 promotes cancer metastasis via the enhancement of the efficiency by which HIF-1alpha mRNA is translated. Cancer Lett. 376, 34–42. doi: 10.1016/j.canlet.2016.02.022
Tang, C., Klukovich, R., Peng, H., Wang, Z., Yu, T., Zhang, Y., et al. (2018). ALKBH5-dependent m6A demethylation controls splicing and stability of long 3’-UTR mRNAs in male germ cells. Proc. Natl. Acad. Sci. U.S.A. 115, E325–E333. doi: 10.1073/pnas.1717794115
Thomason, A. R., Brian, D. A., Velicer, L. F., and Rottman, F. M. (1976). Methylation of high-molecular-weight subunit RNA of feline leukemia virus. J. Virol. 20, 123–132.
Tirumuru, N., Zhao, B. S., Lu, W., Lu, Z., He, C., and Wu, L. (2016). N(6)-methyladenosine of HIV-1 RNA regulates viral infection and HIV-1 Gag protein expression. eLife 5:e15528. doi: 10.7554/eLife.15528
Toth, Z., Maglinte, D. T., Lee, S. H., Lee, H. R., Wong, L. Y., Brulois, K. F., et al. (2010). Epigenetic analysis of KSHV latent and lytic genomes. PLoS Pathog. 6:e1001013. doi: 10.1371/journal.ppat.1001013
Tsai, K., Courtney, D. G., and Cullen, B. R. (2018). Addition of m6A to SV40 late mRNAs enhances viral structural gene expression and replication. PLoS Pathog. 14:e1006919. doi: 10.1371/journal.ppat.1006919
Uppal, T., Sarkar, R., Dhelaria, R., and Verma, S. C. (2018). Role of pattern recognition receptors in KSHV infection. Cancers 10:E85. doi: 10.3390/cancers10030085
Wang, H., and Li, Y. (2018). Recent progress on functional genomics research of Enterovirus 71. Virol. Sin. doi: 10.1007/s12250-018-0071-9 [Epub ahead of print].
Wang, P., Doxtader, K. A., and Nam, Y. (2016). Structural basis for cooperative function of Mettl3 and Mettl14 methyltransferases. Mol. Cell 63, 306–317. doi: 10.1016/j.molcel.2016.05.041
Wang, X., Lu, Z., Gomez, A., Hon, G. C., Yue, Y., Han, D., et al. (2014). N6-methyladenosine-dependent regulation of messenger RNA stability. Nature 505, 117–120. doi: 10.1038/nature12730
Wang, X., Tan, L., Li, Y., Zhang, Y., Zhou, D., Liu, T., et al. (2012). HCV and HIV infection among heroin addicts in methadone maintenance treatment (MMT) and not in MMT in Changsha and Wuhan, China. PLoS One 7:e45632. doi: 10.1371/journal.pone.0045632
Wang, X., Zhao, B. S., Roundtree, I. A., Lu, Z., Han, D., Ma, H., et al. (2015). N(6)-methyladenosine modulates messenger RNA translation efficiency. Cell 161, 1388–1399. doi: 10.1016/j.cell.2015.05.014
Wang, Y., Li, Y., Toth, J. I., Petroski, M. D., Zhang, Z., and Zhao, J. C. (2014). N6-methyladenosine modification destabilizes developmental regulators in embryonic stem cells. Nat. Cell Biol. 16, 191–198. doi: 10.1038/ncb2902
Wei, C. M., Gershowitz, A., and Moss, B. (1975). Methylated nucleotides block 5’ terminus of HeLa cell messenger RNA. Cell 4, 379–386.
Wen, J., Lv, R., Ma, H., Shen, H., He, C., Wang, J., et al. (2018). Zc3h13 regulates nuclear RNA m(6)A methylation and mouse embryonic stem cell self-renewal. Mol. Cell 69, 1028–1038.e6. doi: 10.1016/j.molcel.2018.02.015
Wyde, P. R., Ambrose, M. W., Meyer, H. L., Zolinski, C. L., and Gilbert, B. E. (1990). Evaluation of the toxicity and antiviral activity of carbocyclic 3-deazaadenosine against respiratory syncytial and parainfluenza type 3 viruses in tissue culture and in cotton rats. Antiviral Res. 14, 215–225.
Xiang, S., Liu, K., Yan, Z., Zhang, Y., and Sun, Z. (2016). RNAMethPre: a web server for the prediction and query of mRNA m6A sites. PLoS One 11:e0162707. doi: 10.1371/journal.pone.0162707
Xiao, Y., Wang, Y., Tang, Q., Wei, L., Zhang, X., and Jia, G. (2018). An elongation- and ligation-based qPCR amplification method for the radiolabeling-free detection of locus-specific N(6) -methyladenosine modification. Angew. Chem. Int. Ed. Engl. 57, 15995–16000. doi: 10.1002/anie.201807942
Xu, C., Wang, X., Liu, K., Roundtree, I. A., Tempel, W., Li, Y., et al. (2014). Structural basis for selective binding of m6A RNA by the YTHDC1 YTH domain. Nat. Chem. Biol. 10, 927–929. doi: 10.1038/nchembio.1654
Xuan, J. J., Sun, W. J., Lin, P. H., Zhou, K. R., Liu, S., Zheng, L. L., et al. (2018). RMBase v2.0: deciphering the map of RNA modifications from epitranscriptome sequencing data. Nucleic Acids Res. 46, D327–D334. doi: 10.1093/nar/gkx934
Yang, Y., Hsu, P. J., Chen, Y. S., and Yang, Y. G. (2018). Dynamic transcriptomic m(6)A decoration: writers, erasers, readers and functions in RNA metabolism. Cell Res. 28, 616–624. doi: 10.1038/s41422-018-0040-8
Yao, Q. J., Sang, L., Lin, M., Yin, X., Dong, W., Gong, Y., et al. (2018). Mettl3-Mettl14 methyltransferase complex regulates the quiescence of adult hematopoietic stem cells. Cell Res. 28, 952–954. doi: 10.1038/s41422-018-0062-2
Ye, F. (2017). RNA N(6)-adenosine methylation (m(6)A) steers epitranscriptomic control of herpesvirus replication. Inflamm. Cell Signal. 4:e1604.
Ye, F., Chen, E. R., and Nilsen, T. W. (2017). Kaposi’s sarcoma-associated herpesvirus utilizes and manipulates RNA N(6)-adenosine methylation to promote lytic replication. J. Virol. 91:e00466-17. doi: 10.1128/JVI.00466-17
Yue, Y., Liu, J., and He, C. (2015). NA N6-methyladenosine methylation in post-transcriptional gene expression regulation. Genes Dev. 29, 1343–1355. doi: 10.1101/gad.262766.115
Zhang, J., Randall, G., Higginbottom, A., Monk, P., Rice, C. M., and McKeating, J. A. (2004). CD81 is required for hepatitis C virus glycoprotein-mediated viral infection. J. Virol. 78, 1448–1455.
Zhao, B. S., and He, C. (2017). “Gamete On” for m(6)A: YTHDF2 exerts essential functions in female fertility. Mol. Cell 67, 903–905. doi: 10.1016/j.molcel.2017.09.004
Zhao, Q., Liang, D., Sun, R., Jia, B., Xia, T., Xiao, H., et al. (2015). Kaposi’s sarcoma-associated herpesvirus-encoded replication and transcription activator impairs innate immunity via ubiquitin-mediated degradation of myeloid differentiation factor 88. J. Virol. 89, 415–427. doi: 10.1128/JVI.02591-14
Zhao, S. S., Tang, L. H., Dai, X. H., Wang, W., Zhou, R. R., Chen, L. Z., et al. (2011). Comparison of the efficacy of tenofovir and adefovir in the treatment of chronic hepatitis B: a systematic review. Virol. J. 8:111. doi: 10.1186/1743-422X-8-111
Zheng, G., Dahl, J. A., Niu, Y., Fedorcsak, P., Huang, C. M., Li, C. J., et al. (2013). ALKBH5 is a mammalian RNA demethylase that impacts RNA metabolism and mouse fertility. Mol. Cell 49, 18–29. doi: 10.1016/j.molcel.2012.10.015
Zheng, Q., Hou, J., Zhou, Y., Li, Z., and Cao, X. (2017). The RNA helicase DDX46 inhibits innate immunity by entrapping m(6)A-demethylated antiviral transcripts in the nucleus. Nat. Immunol. 18, 1094–1103. doi: 10.1038/ni.3830
Zheng, Y., Nie, P., Peng, D., He, Z., Liu, M., Xie, Y., et al. (2018). m6AVar: a database of functional variants involved in m6A modification. Nucleic Acids Res. 46, D139–D145. doi: 10.1093/nar/gkx895
Zhou, H. Y., Zheng, Y. H., He, Y., Chen, Z., Liu, M., Yin, W., et al. (2010). Evaluation of a 6-year highly active antiretroviral therapy in Chinese HIV-1-infected patients. Intervirology 53, 240–246. doi: 10.1159/000302762
Zhou, J., Wan, J., Gao, X., Zhang, X., Jaffrey, S. R., and Qian, S. B. (2015). Dynamic m(6)A mRNA methylation directs translational control of heat shock response. Nature 526, 591–594. doi: 10.1038/nature15377
Keywords: m6A, virus, infection, immune, viral life cycle
Citation: Dang W, Xie Y, Cao P, Xin S, Wang J, Li S, Li Y and Lu J (2019) N6-Methyladenosine and Viral Infection. Front. Microbiol. 10:417. doi: 10.3389/fmicb.2019.00417
Received: 30 November 2018; Accepted: 18 February 2019;
Published: 05 March 2019.
Edited by:
Herman W. Favoreel, Ghent University, BelgiumReviewed by:
Hao Lin, University of Electronic Science and Technology of China, ChinaCopyright © 2019 Dang, Xie, Cao, Xin, Wang, Li, Li and Lu. This is an open-access article distributed under the terms of the Creative Commons Attribution License (CC BY). The use, distribution or reproduction in other forums is permitted, provided the original author(s) and the copyright owner(s) are credited and that the original publication in this journal is cited, in accordance with accepted academic practice. No use, distribution or reproduction is permitted which does not comply with these terms.
*Correspondence: Jianhong Lu, amlhbmhsdUBjc3UuZWR1LmNu
Disclaimer: All claims expressed in this article are solely those of the authors and do not necessarily represent those of their affiliated organizations, or those of the publisher, the editors and the reviewers. Any product that may be evaluated in this article or claim that may be made by its manufacturer is not guaranteed or endorsed by the publisher.
Research integrity at Frontiers
Learn more about the work of our research integrity team to safeguard the quality of each article we publish.