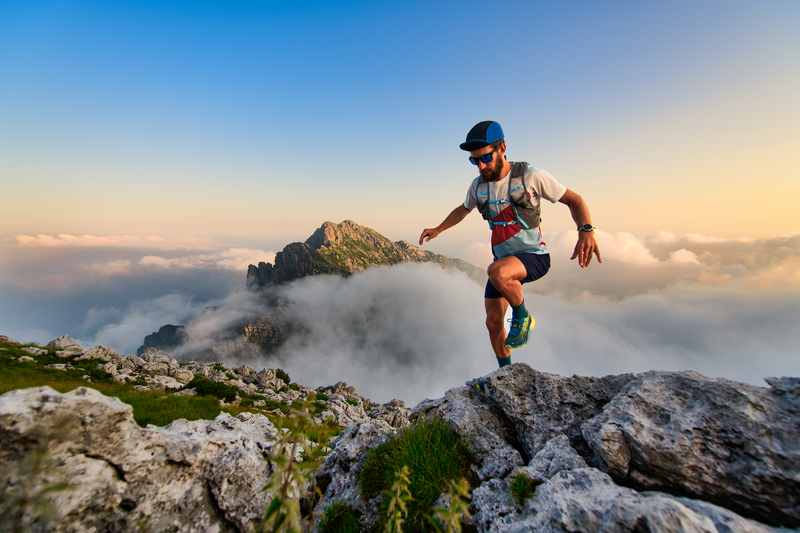
94% of researchers rate our articles as excellent or good
Learn more about the work of our research integrity team to safeguard the quality of each article we publish.
Find out more
ORIGINAL RESEARCH article
Front. Microbiol. , 05 March 2019
Sec. Microbiotechnology
Volume 10 - 2019 | https://doi.org/10.3389/fmicb.2019.00399
This article is part of the Research Topic Bacterial Bidirectional Extracellular Electron Transfer View all 29 articles
In the present study, we found that our isolate Shewanella decolorationis NTOU1 is able to degrade acetate under anaerobic condition with concomitant implementation of extracellular electron transfer (EET). With +0.63 V (vs. SHE) poised on the anode, in a 72-h experiment digesting acetate, only 2 mM acetate was consumed, which provides 6% of the electron equivalents derived from the initial substrate mass to support biomass (5%) and current generation (1%). To clarify the effects on EET of the addition of electron-shuttles, riboflavin, anthraquinone-2,6-disulfonate (AQDS), hexaammineruthenium, and hexacyanoferrate were selected to be spiked into the electrochemical cell in four individual experiments. It was found that the mediators with proton-associated characteristics (i.e., riboflavin and AQDS) would not enhance current generation, but the metal-complex mediators (i.e., hexaammineruthenium, and hexacyanoferrate) significantly enhanced current generation as the concentration increased. According to the results of electrochemical analyses, the i-V graphs represent that the catalytic current induced by the primitive electron shuttles started at the onset potential of −0.27 V and continued increasing until +0.73 V. In the riboflavin-addition experiment, the catalytic current initiated at the same potential but rapid saturated beyond −0.07 V; this indicated that the addition of riboflavin affects mediator secretion by S. decolorationis NTOU1. It was also found that the current was eliminated after adding 48 mM N-acetyl-L-methionine (i.e., the cytochrome inhibitor) when using acetate as a substrate, indicating the importance of outer-membrane cytochrome.
Bioelectrochemical systems (BESs) can now be derived as technologies or applications that utilize the electrochemical interaction of microbes and electrodes, which is usually powered by oxidizing organic-matter oxidation by means of the redox reactions of microorganisms (or the other biological moieties like enzyme and cell organelle) occurring on the anode (Schroder et al., 2015). To enhance the performances of the anode, the most promising way is to facilitate extracellular electron transfer (EET) either by selectively inoculating EET capable microorganisms, or adding fixed [e.g., tungsten carbide (Rosenbaum et al., 2006) and α-Fe2O3 (Nakamura et al., 2009)] or diffusive electron shuttles in proximity to the electrodes to chemically assist the EET. Diffusive electron shuttles used as mediators need some essential properties, including high diffusion coefficients, rapid electron transfer, sustainability in repeated redox turnover, and non-cytotoxicity (Bullen et al., 2006). The soluble electron shuttles usually work as transferring across the porin to the cell interior (Ikeda, 2012) in order to bring the electrons out from the internal redox protein (e.g., nicotinamide adenine dinucleotide dehydrogenase, NDH, Li et al., 2017) to the outer electron acceptors, or directly exchange electrons with the outer membrane cytochromes (OMCs, Coursolle et al., 2010). Moreover, recent studies indicated that electron shuttles (i.e., flavin mononucleotide and riboflavin) might interact with OMC by direct bonding, creating the shortest physical distance to favor the electron flow (Okamoto et al., 2013). In addition, in our recent study, we reported that tricarboxylic-acid (TCA)-cycle activities stopped due to excessive mediator addition. This result indicates that Shewanella spp. cannot obtain the necessary adenosine triphosphate (ATPs) via the oxidative phosphorylation at high mediator concentrations (Li et al., 2018).
Diffusive electron shuttles were frequently found in the wastewater or groundwater of an aquifer; this will be the electrolyte for MFC development in the future. For example, a large amount of riboflavin is found in the wastewater of food or pharmaceutical industries (Qian et al., 2009; Sun et al., 2013). Dyes like safranine and methylene blue may be found in the wastewater discharged by the industries which need to color their products (Gupta et al., 2011). These have been used as an effective electron shuttle in the MFC study (Choi et al., 2003; Miroliaei et al., 2015). The humic substances (typically studied using anthraquinone-2,6-disulfonate (AQDS) as a model substitution, Newman and Kolter, 2000) and ferrocyanide (formed in a nuclear-waste-processing site, Plymale et al., 2018) possess redox capabilities and exist in the ground water. Therefore, regarding scaling-up BES for practical applications, it is of utmost importance—essential, in fact—to know just how these external mediators affect EET.
Microorganisms require different metabolic pathways to deal with different substrates. Without competitive electron acceptors like sulfate and nitrate on the anode, for example, glucose may be fermented into acetate and butyrate that absorb two-thirds of substrate electrons, with a resultant low electron recovery (Rabaey and Verstraete, 2005). When lactate is used as the substrate, the metabolism diverging from acetyl-CoA can either enter the TCA cycle or can be reversibly transferred into acetate production to generate ATP (i.e., substrate-level phosphorylation). As for acetate, the only metabolism to extract energy from it is by implementing the TCA cycle for completed oxidation. While the Shewanella spp. have been intensively applied in many BES studies so far (Li et al., 2010; Zhang et al., 2017; Li et al., 2018), to our best knowledge, no reported study has yet to utilize acetate as an electron donor to drive EET on the Shewanella anodes, although it was reported that Shewanella spp. are able to use acetate to reduce some electron acceptors, such as nitrate, which can be uptaken without the need of EET (Yoon et al., 2013). Surprisingly, in our preliminary study, we found that our isolate Shewanella decolorationis NTOU1 was able to use acetate for EET without oxygen. To clarify the interaction between the central metabolism and phosphorylation during EET of Shewanella sp., it is interesting to test the current generation in an electrochemical cell using different substrates that reveals different pathways of metabolism and phosphorylation.
The objective of the present study is to clarify the effect of mediator addition on EET by S. decolorationis NTOU1, especially when acetate is the sole electron donor on the anode. First, the concept of electron-balance calculation was employed to understand the electron distribution when S. decolorationis NTOU1 was exploiting different substrates (i.e., lactate and acetate) for current generation. To study the effect of electron-shuttle addition, four different mediators were used to treat the S. decolorationis NTOU1 anode (connected to a potentiostat) via a serial spiking that generates an array of mediator concentrations in one experimental run: riboflavin; AQDS; hexaammineruthenium (III) chloride (Ru(NH3)63+); and potassium hexacyanoferrate (Fe(CN)63−). By analyzing the i-V graph, it could be clarified whether the externally added mediators could be a useful addition, or if they may inhibit the primitive EET mechanisms of S. decolorationis NTOU1. Finally, the experiment involving the addition of a cytochrome inhibitor (i.e., N-acetyl-L-methionine, AcMet) addition can clarify the importance of OMCs to EET.
Detailed information on S. decolorationis NTOU1 is given elsewhere (Li et al., 2010). The strains of S. oneidensis MR-1 and S. putrefaciens ATCC8071 were purchased from Bioresource Collection and Research Center, Hsinchu, Taiwan. Prior to the electrochemical experiments, both strains were pre-cultured using the Luria-Bertani medium for 24 h at 30°C, harvested by centrifugation (4,629 g, 5 min, 26°C), and then washed three times with a neutral pH phosphate buffer (0.1 M, pH 7) prior to inoculation, to avoid bringing in any unexpected mediators left in the cultural media (Masuda et al., 2010).
In this study, two-chamber bioelectrochemical cells consisting of a working-electrode (anode) chamber and a counter-electrode (cathode) chamber were used to evaluate the efficiency of current generation. The inner walls of 200-mL anode chambers were lined with 24 cm2 of carbon felt which was secured using a Teflon spacer. For the alternative electron donor experiment described in section 3.1, 0.75-mm-thick carbon felt was used (B0050, Toray, Japan). In the other experiments, the thick carbon felt was manually sectioned into a thickness of 3 mm (1-cm-thick, Gansu Haoshi Carbon Fiber Co., Ltd., China) for the following usage. The amount of initial inoculated biomass was about 60 mg-cell L−1 in each bioelectrochemical cell as harvested from 10 mL of the pre-culture broth; t = 0 h in all Figures indicates the moment S. decolorationis NTOU1 was inoculated. In all experiments, the working electrode potential was controlled at +0.63 V (vs. SHE) using a potentiostat (HA-151A, Hokuto Denko, Tokyo, Japan). The response current was recorded using a data acquisition system (GL240, Graphtec, Yokohama, Japan). At the start of each experiment, ca. 45 mM organic acids (i.e., lactate and acetate) were added as electron donors. Other details, including the reactor configuration, anolyte and catholyte composition, and temperature- and redox-condition maintenances are given in our previous works (Li et al., 2018). All the experiments in the present study were conducted at 35°C and pH 7. To know whether S. decolorationis NTOU1 is able to exploit mediators to boost EET/acetate degradation on a polarized electrode, four kinds of redox compounds, riboflavin, AQDS, Ru(NH3)63+, and Fe(CN)63−, were spiked into the electrochemical cell in separate experiments. These mediators were dissolved in the phosphate-buffer saline (i.e., PBS, consisted of 100 mM phosphate and 80 mM potassium chloride, adjusted to pH = 7) to generate the 0.2 and 0.4 mM dense solutions for the spiking processes, and an array of concentrations were consequently generated as listed in the Figure 3.
The organic acids in the anode medium were determined using a high-performance liquid chromatography (HPLC) equipped with a gradient pump (5160, Hitachi, Tokyo, Japan), a UV-Vis detector (5420, Hitachi, Tokyo, Japan), an autosampler (5280, Hitachi, Tokyo, Japan), and an ICSep COREGEL-87H3 column (7.8 mm × 300 mm) placed in an oven (Super CO-150, Enshine, Taipei, Taiwan) at 45°C. Sulfuric acid (8 mM) was used as a mobile phase at a flow rate of 0.5 mL min−1, and all the organic acids were detected at a wavelength of 210 nm. To better understand the steady-state catalytic current performance at different potentials, serial short-term chronoamperometry was carried out by manually adjusting the potential outputs on the Hokuto-Denko potentiostat. Each data point shown in Figure 4 was processed by waiting for 1 h at each potential and averaging the current data recorded in the last 40 min. All the potentials stated in this paper refer to the Standard Hydrogen Electrode.
The specimens (i.e., microbial cells attached on the graphite fibers) were fixed in a solution of 2.5% glutaraldehyde and phosphate buffer saline (100 mM phosphate and 80 mM KCl, pH 7.8, PBS) for 16 h at 4°C. Specimens were rinsed three times in the PBS to remove residual glutaraldehyde, for 10 min each time. Dehydration was carried out with a series of gradually increasing ethanol concentrations: 50, 75, 85, 95, and 100%. Finally, the dehydrated specimens were dried using the critical point drier (HCP-H2, Hitachi, Tokyo, Japan) and then coated with gold by ion sputtering (E101, Hitachi, Tokyo, Japan). Desiccated samples were observed using a TM3000 SEM (Hitachi, Tokyo, Japan).
In the experiments described in Sections 3-2 and 3-4, the mediators and metabolic inhibitor were added to the electrochemical cells to clarify their effect on current generation and substrate degradation, namely, riboflavin (Sigma Chemical Co., United States), anthraquinone-2,6-disulfonate (AQDS, Tokyo Chemical Industry Co., Ltd., Japan), Ru(NH3)63+ (Sigma Chemical Co., United States), Fe(CN)63− (Kanto Chemical Co., Inc., Japan), and AcMet (Sigma Chemical Co., United States). In addition to the mediators, the AcMet was also dissolved in the aforementioned PBS to generate a 1.2 M dense solution for the spiking processes, and an array of AcMet concentrations were consequently generated as listed in the Figure 5.
When carrying out EET on an anode, Shewanella cells catalyze the substrate oxidation and electrode reduction, which also play a role as products (i.e., cell synthesis) of the entire reaction. In our recent study (Li et al., 2018), we developed a mathematic calculation describing cell growth, lactate consumption, as well as pyruvate and acetate production, which is based on the known central metabolic pathways involved in S. oneidensis MR-1 (Scott and Nealson, 1994). According to our previous findings, it is clarified that the intermediate (i.e., pyruvate and acetate) productions can indicate whether the lactate has been partially or completely oxidized, which are the major sources generating nicotinamide-adenine-dinucleotide equivalents to contribute current generation. To continuously track cell growth during the batch tests, the use of the mass-balance calculation, instead of destructive methods like protein assay, was proposed. By analyzing the organic-acid data detected using HPLC, the following electron-equivalent balance was developed to calculate biomass production:
where QCell is the metabolic charge used for biomass growth (not actually measured in this study); ΔnLa (<0), ΔnPy (>0), and ΔnAc (>0) are the changes in the mole numbers of lactate, pyruvate, and acetate, respectively; QEL is the total charge of the electricity production calculated by integrating the electric current over time (i.e., ∫idt); and F is the Faraday constant. The coefficients 12 (for lactate), 10 (for pyruvate), and 8 (for acetate) indicate the electron equivalents of biomass, lactate, pyruvate, and acetate, respectively. As for the experimental condition using acetate as the electron donor, Eq. (1) can be, respectively, rearranged as:
Some assumptions need to be made prior to validating Eq. (2): (i) the metabolism flows in the direction of acetate first converting to acetyl-CoA, then entering the TCA cycle; (ii) all biomass formation was assumed to proceed via the tricarboxylic acid (TCA) cycle; (iii) no unexpected intermediate was produced (e.g., TCA cycle intermediates) during the metabolism. To express the electron distribution shown in Figure 1, the electron-conversion (i.e., −12FΔnLa, 10FΔnPy, 8FΔnAc, QCell, and QEL) proportion is calculated over the initial electron pool (i.e., 12FnLa and 8FnAc at t = 0 h), in terms of percentage.
Figure 1. Profiles of electron distribution illustrating organic-acid metabolism for 72 h in the electrochemical cells using ca. 35 mM of (A) lactate and (B) acetate as electron donors. X in the y axis denotes the electron quantities as fractions derived from the total charge in the initial-substrate mass (i.e., 12FnLa and 8FnAc in (A) and (B), respectively).
During the batch experiments, no significant changes in pH were found over time. The electron distributions of different substrate utilization and intermediate production are shown in Figure 1. In the lactate-degrading experiment, 41% of the substrate was consumed after 72 h. Within the end product composition, 20, 6, and 16% of electrons were distributed to acetate, charge, and biomass, respectively. In the acetate-degrading experiment, only 6% of the substrate was consumed. It is noteworthy that at 28 h (Figure 1B), 6 and 0.2% of the electron were distributed to the biomass and charge production, respectively, but at 72 h, the proportions of them became 5 and 1%. The changing proportions indicated that the current generation from acetate was not perfectly growth associated, and presumably some cellular materials were lysed to generate current due to the decreasing of biomass-charge proportion (i.e., from 6 to 5%). To investigate the morphologies of the S. decolorationis NTOU1 grown on the carbon felt, the specimens for SEM observations were prepared after 31 h of electrochemical culturing. The SEM micrographs in the Figure 2A shows the clear structure of graphite fibers piling together in the carbon felt, and the Figure 2B shows the microbial cells colonizing on the graphite fibers with visible bacterial-nanowire-like morphologies, indicating that S. decolorationis NTOU1 was successfully cultivated on the electrode surfaces.
Figure 2. SEM images of S. decolorationis NTOU1 colonizing on the carbon-felt electrodes. (A) the clear structures of piled graphite fibers; (B) developed biofilms of S. decolorationis NTOU1 fed with acetate in the electrochemical cell under the poised potential of +630 mV.
As shown in Figure 3A, with a series of riboflavin spiking (from 0.5 to 22 μM) the response current was not affected, but instead put out a steady current of ca. 0.44 mA after 1 h. After 22 μM riboflavin was entirely spiked into the electrochemical cell, a 100 μM Fe(CN)63− was spiked at t = 9.5 h, consequently resulting in a drastic current increase to 1.7 mA. From 0 to 9.5 h, acetate decreased from 44 to 41 mM, but after Fe(CN)63− spiking, the acetate concentration remained at 41 mM from 9.5 to 11.2 h without significant change. Similarly, no significant current change was found in the AQDS spiking experiment (Figure 3B). The acetate degrading rate was not affected before 8 μM AQDS spiking was done (i.e., at t = 5.3 h), but an unexpected acetate-concentration drop (i.e., from 39 to 37 mM) happened instantly after 12 μM AQDS spiking from t = 6.8 to 7.7 h. In Figures 3C,D, the response current immediately jumped on each spiking, resulting in staircase-like increments. After the spiking was complete, the final concentrations of Ru(NH3)63+ and Fe(CN)63− both accumulated to 22 μM, and consequently their anodic currents reached 0.8 and 1.5 mA, respectively. With regard to the substrate concentration, the acetate degradation was found to stop at the last spike (i.e., 18 μM Ru(NH3)63+ and 22 μM Fe(CN)63−, respectively), although the currents at the same moment were found to be higher than prior performance in the same experiments.
Figure 3. Time course profiles of the electric current (sold line) and the acetate concentration (open triangle) obtained with different mediator spikings: (A) riboflavin, (B) AQDS, (C) Ru(NH3)63+, and (D) Fe(CN)63− spiked during the chronoamperometric processes. The arrows in (A) indicate the 0.5, 1, 2, 4, 8, 12, and 22 μM riboflavin, and 100 μM Fe(CN)63− in turn in the electrochemical cell; the arrows in (B) and (D), respectively, indicate the AQDS and Fe(CN)63− concentrations of 0.5, 1, 2, 4, 8, 12, and 22 μM in turn; the arrows in (C) indicate the Ru(NH3)63+ concentrations of 0.5, 1, 2, 4, 8, and 18 μM in turn.
To plot the i-V graph, chronoamperometry (with +0.63 V poised) commenced by implementing a mediator-free running for ca. 20 h after inoculating S. decolorationis NTOU1. After 20 h, 10 μM riboflavin or Fe(CN)63− was spiked into each electrochemical cell, and the running was extended for an additional 2 h. After the aforementioned processes were complete, the potentials were manually adjusted to different levels and the responded current was recorded and averaged as described in the Section 2.3. To implement a mediator-control experiment, all the procedures were repeated, but this time mediator spiking was omitted. By reading the steady-state current increment in the same direction of the increasing poised potential, the catalytic current taking place at an onset of −0.27 V was found in all the experimental conditions (Figure 4). In the riboflavin-spiking experiment, the steady-state current was saturating at 0.07 V, but with regard to the mediator-control experiment, the steady-state current continued increasing from −0.27 to 0.73 V with no obvious saturation observed. In the Fe(CN)63−-spiking experiment, a secondary catalytic current started at 0.13 V was found, in addition to the one starting at −0.27 V.
Figure 4. The steady-state catalytic currents of the acetate degradation recorded at different applied potentials. Open circle, S. decoloration NTOU1 digesting acetate without any mediator addition; open square, with 10 μM riboflavin addition; open triangle, with 10 μM ferricyanide addition; cross, only the acetate anolyte but without any mediator addition and S. decoloration NTOU1 inculation.
To evaluate the role of c-type cytochromes in current generation, AcMet was spiked in an order of different concentrations, binding to the c-type cytochromes and rendering them non-functional (Nakamura et al., 2009). First, 6 mM of AcMet was added after 3.3 h of operation at +0.63 V; subsequently, each addition of AcMet was conducted every 1 or 2 h. In the lactate experiment (Figure 5A), the response current decreased after the first spiking. As the AcMet concentration increased from 6 to 48 mM, the rate of current decrease did not change significantly. After 48 mM AcMet were entirely added, 6 μM riboflavin was subsequently added into the electrochemical cell, which immediately rescued the current to ca. 0.73 mA following a mild reduction to ca 0.50 mA at 20 h. In the acetate experiment (Figure 5B), as the AcMet concentration increased from 6 to 48 mM, the current significantly decreased after each spiking. With the same procedure of adding 6 μM riboflavin, although the current could be immediately rescued from 0.07 to 0.12 mA at 10 h, the response current continued decreasing until total elimination at 20 h.
Figure 5. The effects of metabolic inhibitors on the current genration of S. decolorationis NTOU1 utilizing (A) lactate and (B) acetate. The arrows in both figures indicate the 6, 12, 24, 36, 48 mM AcMet, and 6 μM riboflavin in turn in the electrochemical cell.
According to our preliminary work, we set a current-generating competition between S. decolorationis NTOU1 and S. putrefaciens ATCC8071 using thicker carbon felts (SG-224K, Osaka Gas, Osaka, Japan, cut into 1.4 × 5.5 × 18 cm3) and 35 mM lactate as a substrate, but the same cell configuration and electrolyte ingredients mentioned in Section 2-2; and the current generation results are shown in the Supplementary Figure S1. The major advantages of using S. decolorationis NTOU1 over S. putrefaciens ATCC8071 are a higher reaction rate and superior adaptability: after inoculating the same quantities of microbial cells, the experiment with S. decolorationis NTOU1 presented an immediate current generation, whereas there was a 40-h lag phase found when using S. putrefaciens ATCC8071. Regarding the performance of the current generation, the charge production of S. putrefaciens ATCC8021 (724 C) are lower than that of S. decolorationis NTOU1 (770 C). Combining the other performances including faster lactate degradation rate and greater coulomb efficiency (see the descriptions in the Supplementary Materials), it is indicated that S. decolorationis NTOU1 demonstrates superior performance to S. putrefaciens ATCC8071 when lactate was provided as an electron donor.
With our results suggesting that lactate is the preferable substrate (41% electron consumption) for EET, deliberately using acetate here becomes an alternative method to render S. decolorationis NTOU1 a “weak electricigen” that should be studied to understand what drives EET in response to electron-donor- or acceptor-limiting condition (Doyle and Marsili, 2018). In a separated experiment, we inoculated S. oneidensis MR-1 in the electrochemical cell and subsequently analyzed its current generation and acetate consumption. According to our results (Supplementary Figure S2), S. oneidensis MR-1 can hardly utilize acetate in 48 h, but a small amount of current could still be generated in the absence of acetate addition, indicating that EET-capable microorganisms may somehow utilizing their own cellular materials to implement EET (Freguia et al., 2007). To understand how many coulombs of charge could be produced via cell lysis of S. decolorationis NTOU1, the control experiments with/without acetate addition were implemented and the results are shown in the Supplementary Figure S3. By comparing the current-generating results, the charge production in the experiment of with acetate addition (i.e., 6 C in 16.8 h) was obviously higher than the one without acetate addition (i.e., 16 C in 16.8 h), indicating that a significant number of charge could be produced via acetate degradation. For acetate, only 6% of the substrate was removed, indicating that acetate was slightly utilized by Shewanella under anaerobic condition in the electrochemical cell. Although the acetate-degrading quantity is significant, this result agrees with those reported in other studies, which found similar acetate-accumulation (Park and Zeikus, 2002; Marsili et al., 2008). Considering the metabolic pathway, one mole of ATP is generated when one mole of acetyl-CoA is converted to one mole of acetate; vice versa, one mole of ATP is consumed when one mole of acetate is converted to acetyl-CoA with the aid of phosphotransacetylase, acetate kinase, and acetyl-CoA synthase (Kumari et al., 2000). When acetate is used as the sole substrate, unlike the lactate-utilizing conditions, there is no substrate-level phosphorylation pathway that may instantly generate sufficient ATPs to boost the catabolism of acetyl-CoA. Notably, an insufficient ATP level might impair the efficiency of current generation due to numerous ATPs being required to generate redox compounds such as riboflavin (Bacher et al., 2000; Dauner et al., 2002).
Fe(CN6)3− possesses a higher formal potential (+0.36 V) than riboflavin (−0.3 V), and as such can better receive electrons from OMC or NDH of Shewanella (Li et al., 2018), thus driving a faster kinetics so as to generate a stronger current. Furthermore, the riboflavin is a proton-associated redox compound (though metal complexes such as Fe(CN6)3− are not) that might absorb the protons available in the periplasm during EET, leading to less ATP production (i.e., turning down the oxidative phosphorylation) and unfavorable acetate degradation. To better clarify the effects of mediator potential and proton-absorbing characteristics, AQDS and Ru(NH3)63+ were selected for further examination. Although the formal-potential difference between AQDS (−0.16 V at pH = 7, 25 C, Li et al., 2018) and Ru(NH3)63+ (+0.12 V, Loew et al., 2017) is much smaller than the one between riboflavin and Fe(CN6)3−, AQDS also holds the characteristic of proton absorption during redox reactions. Therefore, it cannot enhance the current generation for the same reason as when riboflavin is used as a mediator. In addition, it was unexpectedly found that the acetate consumption rate slowed down even when the current got stronger with the Fe(CN6)3− (Figure 3A), Ru(NH3)63+ (Figure 3C), and Fe(CN6)3− (Figure 3C) concentrations reading 100, 18, and 22 μM, respectively. It is presumably due to an unknown mechanism inhibiting acetate metabolism by metal-complex mediators; consequently, the cellular material of S. decolorationis NTOU1 was used for current generation.
While cyclic voltammetry (CV) is a potentiodynamic method which ramps potential linearly versus time in a quiescent solution, the advantage of carrying out the serial short-term chronoamperometry (mentioned in the Section 2.3) is that the non-faradaic current is much smaller than that observed in CV and that the catalytic current can be easily measured under the steadily mixing conditions. Since it is critical to exhibit the sigmoidal i-V curves that directly indicate which mediator is exactly carrying out turnover (i.e., activated microbial cells keep reducing mediators oxidized by the anodic electrode), we would suggest that in the case of utilizing less preferable electron donors (e.g., acetate in the present study), serial short-term chronoamperometry is a rather promising method that could better analyze the electrochemical characteristics of EET-capable microorganisms. In our past study, it was reported that S. decolorationis NTOU1 is able to secrete riboflavin and menaquinone on cell surfaces that are able to drive the catalytic current under the anaerobic condition (Li et al., 2010). The results shown in Figures 3A, 4 consistently indicate that with the 10 μM riboflavin addition, the current would not be enhanced at +0.63 V. Moreover, unlike the mediator-control experiment (i.e., open-circle symbols in the Figure 4), the steady-state current in the riboflavin-addition experiment (i.e., open-square symbols) did not ramp up from −0.07 to +0.73 V. This result indicates that the riboflavin addition might possibly inhibit some primitive mediator (e.g., menaquinone, −0.75 V, Hernandez and Newman, 2001) secretion by either turning down some specific genes (e.g., menC decoding menaquinone-synthesizing proteins, Newman and Kolter, 2000), or impairing mediator diffusion to the cell exterior. When the lactate is used as the substrate, it is found that S. decolorationis NTOU1 is less sensitive to AcMet (Figure 5A). This result agrees with a recent study using potassium cyanide as an OMC inhibitor of Shewanella strain Hac319 (Takeuchi et al., 2018). The EET activities rescued by diffusive-riboflavin addition (Figure 5) indicate that in addition to the model of flavin-bound OMC (aka flavocytochrome, Edwards et al., 2015), diffusive riboflavin can still assist EET after a large fraction of OMCs been deactivated. In the present study, the interactions between primitive mediators and OMCs are not yet clear. However, when acetate is used as the substrate, the significant current response to AcMet indicates the importance of OMC acting as the last step of oxidative phosphorylation, the only pathway to phosphorylate ADP to ATP during acetate metabolism.
To clarify the status of EET when S. decolorationis NTOU1 simultaneously exploits acetate and externally added mediators, this study is comprised four individual experiments which concurrently provide strong evidences by comparing different substrate utilization, mediator potentials and chemical characteristics. The combination of electrochemical and instrumental analyses used in this work leads to the following conclusions:
1. Shewanella decolorationis NTOU1 is holding significant acetate-exploiting capabilities for EET, although acetate is considered as a less preferable substrate for S. decolorationis NTOU1 (only 6% electron consumed after a 72-h incubation).
2. Considering the cross-comparison results based on the formal potentials and chemical characteristics of the four externally added mediators used in this study, it is apparent that the proton-associated mediators (i.e., riboflavin and AQDS) do not significantly assist current generation, but the metal-complex mediators (i.e., Fe(CN)63− and Ru(NH3)63+) do, when acetate is used as a substrate.
3. According to the electrochemical analyses, the results show that the riboflavin addition affects primitive mediator production, but Fe(CN)63− does not. The inhibitor adding experiment also reveals the crucial role of OMCs when S. decolorationis NTOU1 exploits acetate for EET.
S-LL conceived and designed the experiments. S-LL, Y-JW, and Y-CC performed the experiments. S-LL and C-PY wrote the manuscript. S-ML and C-PY analyzed the data. S-ML provided the pure strains of Shewanella spp.
The authors acknowledge the financial support provided by the Ministry of Science and Technology, Taiwan, and National Taiwan University Higher Education Sprout Project, Ministry of Education Taiwan, under grants MOST 106-2221-E-002-023-MY3 and 106R7821, respectively.
The authors declare that the research was conducted in the absence of any commercial or financial relationships that could be construed as a potential conflict of interest.
The Supplementary Material for this article can be found online at: https://www.frontiersin.org/articles/10.3389/fmicb.2019.00399/full#supplementary-material
Bacher, A., Eberhardt, S., Fischer, M., Kis, K., and Richter, G. (2000). Biosynthesis of vitamin B-2 (riboflavin). Annu. Rev. Nutr. 20, 153–167. doi: 10.1146/annurev.nutr.20.1.153
Bullen, R. A., Arnot, T. C., Lakeman, J. B., and Walsh, F. C. (2006). Biofuel cells and their development. Biosens. Bioelectron. 21, 2015–2045. doi: 10.1016/j.bios.2006.01.030
Choi, Y., Kim, N., Kim, S., and Jung, S. (2003). Dynamic behaviors of redox mediators within the hydrophobic layers as an important factor for effective microbial fuel cell operation. Bull. Korean Chem. Soc. 24, 437–440. doi: 10.5012/bkcs.2003.24.4.437
Coursolle, D., Baron, D. B., Bond, D. R., and Gralnick, J. A. (2010). The Mtr respiratory pathway is essential for reducing flavins and electrodes in Shewanella oneidensis. J. Bacteriol. 192, 467–474. doi: 10.1128/JB.00925-09
Dauner, M., Sonderegger, M., Hochuli, M., Szyperski, T., Wuthrich, K., Hohmann, H. P., et al. (2002). Intracellular carbon fluxes in riboflavin-producing Bacillus subtilis during growth on two-carbon substrate mixtures. Appl. Environ. Microbiol. 68, 1760–1771. doi: 10.1128/AEM.68.4.1760-1771.2002
Doyle, L. E., and Marsili, E. (2018). Weak electricigens: a new avenue for bioelectrochemical research. Bioresour. Technol. 258, 354–364. doi: 10.1016/j.biortech.2018.02.073
Edwards, M. J., White, G. F., Norman, M., Tome-Fernandez, A., Ainsworth, E., Shi, L., et al. (2015). Redox linked flavin sites in extracellular decaheme proteins involved in microbe-mineral electron transfer. Sci. Rep. 5:11677. doi: 10.1038/srep11677
Freguia, S., Rabaey, K., Yuan, Z. G., and Keller, J. (2007). Electron and carbon balances in microbial fuel cells reveal temporary bacterial storage behavior during electricity generation. Environ. Sci. Technol. 41, 2915–2921. doi: 10.1021/es062611i
Gupta, V. K., Jain, R., Saleh, T. A., Nayak, A., Malathi, S., and Agarwal, S. (2011). Equilibrium and thermodynamic studies on the removal and recovery of safranine-T dye from industrial effluents. Sep. Purif. Technol. 46, 839–846. doi: 10.1080/01496395.2010.535591
Hernandez, M. E., and Newman, D. K. (2001). Extracellular electron transfer. Cell Mol. Life Sci. 58, 1562–1571. doi: 10.1007/PL00000796
Ikeda, T. (2012). Bioelectrochemical studies based on enzyme-electrocatalysis. Electrochim. Acta 82, 158–164. doi: 10.1016/j.electacta.2012.01.114
Kumari, S., Beatty, C. M., Browning, D. F., Busby, S. J. W., Simel, E. J., Hovel-Miner, G. A., et al. (2000). Regulation of acetyl coenzyme A synthetase in Escherichia coli. J. Bacteriol. 182, 4173–4179. doi: 10.1128/JB.182.15.4173-4179.2000
Li, S. L., Bai, M. D., Hsiao, C. J., Cheng, S. S., and Nealson, K. H. (2017). A metabolic-activity-detecting approach to life detection: restoring a chemostat from stop-feeding using a rapid bioactivity assay. Bioelectrochemistry 118, 147–153. doi: 10.1016/j.bioelechem.2017.08.001
Li, S.-L., Freguia, S., Liu, S.-M., Cheng, S.-S., Tsujimura, S., Shirai, O., et al. (2010). Effects of oxygen on Shewanella decolorationis NTOU1 electron transfer to carbon-felt electrodes. Biosens. Bioelectron. 25, 2651–2656. doi: 10.1016/j.bios.2010.04.038
Li, S.-L., Yen, J.-H., Kano, K., Liu, S.-M., Cheng, S.-S., and Chen, H.-Y. (2018). Using metabolic charge production in the tricarboxylic acid cycle (QTCA) to evaluate the extracellular-electron-transfer performances of Shewanella spp. Bioelectrochemistry 124, 119–126. doi: 10.1016/j.bioelechem.2018.07.001
Loew, N., Tsugawa, W., Nagae, D., Kojima, K., and Sode, K. (2017). Mediator preference of two different FAD-dependent glucose dehydrogenases employed in disposable enzyme glucose sensors. Sensors 17:2636. doi: 10.3390/s17112636
Marsili, E., Baron, D. B., Shikhare, I. D., Coursolle, D., Gralnick, J. A., and Bond, D. R. (2008). Shewanella secretes flavins that mediate extracellular electron transfer. Proc. Natl. Acad. Sci. U.S.A. 105, 3968–3973. doi: 10.1073/pnas.0710525105
Masuda, M., Freguia, S., Wang, Y.-F., Tsujimura, S., and Kano, K. (2010). Flavins contained in yeast extract are exploited for anodic electron transfer by Lactococcus lactis. Bioelectrochemistry 78, 173–175. doi: 10.1016/j.bioelechem.2009.08.004
Miroliaei, M. R., Samimi, A., Mohebbi-Kalhori, D., Khorram, M., and Qasemi, A. (2015). Competition between E. coli and Shewanella sp. for electricity generation in air cathode MFC in presence of methylene blue as artificial mediator. Environ. Prog. Sustain. Energy 34, 1097–1105. doi: 10.1002/ep.12111
Nakamura, R., Kai, F., Okamoto, A., Newton, G. J., and Hashimoto, K. (2009). Self-constructed electrically conductive bacterial networks. Angew. Chem. Int. Ed. Engl. 121, 516–519. doi: 10.1002/ange.200804750
Newman, D. K., and Kolter, R. (2000). A role for excreted quinones in extracellular electron transfer. Nature 405, 94–97. doi: 10.1038/35011098
Okamoto, A., Hashimoto, K., Nealson, K. H., and Nakamura, R. (2013). Rate enhancement of bacterial extracellular electron transport involves bound flavin semiquinones. Proc. Natl. Acad. Sci. U.S.A. 110, 7856–7861. doi: 10.1073/pnas.1220823110
Park, D. H., and Zeikus, J. G. (2002). Impact of electrode composition on electricity generation in a single-compartment fuel cell using Shewanella putrefaciens. Appl. Microbiol. Biotechnol. 59, 58–61. doi: 10.1007/s00253-002-0972-1
Plymale, A., Wells, J., Graham, E., Qafoku, O., Brooks, S., and Lee, B. (2018). Bacterial productivity in a ferrocyanide-cContaminated aquifer at a nuclear waste site. Water 10:1072. doi: 10.3390/w10081072
Qian, S., Wu, Z., Zheng, H., and Geng, Y. (2009). Study on riboflavin recovery from wastewater by a batch foam separation process. Sep. Purif. Technol. 44, 2681–2694. doi: 10.1080/01496390902885940
Rabaey, K., and Verstraete, W. (2005). Microbial fuel cells: novel biotechnology for energy generation. Trends Biotechnol. 23, 291–298. doi: 10.1016/j.tibtech.2005.04.008
Rosenbaum, M., Zhao, F., Schroder, U., and Scholz, F. (2006). Interfacing electrocatalysis and biocatalysis with tungsten carbide: a high-performance, noble-metal-free microbial fuel cell. Angew. Chem. Int. Ed. Engl. 45, 6658–6661. doi: 10.1002/anie.200602021
Schroder, U., Harnisch, F., and Angenent, L. T. (2015). Microbial electrochemistry and technology: terminology and classification. Energy Environ. Sci. 8, 513–519. doi: 10.1039/c4ee03359k
Scott, J. H., and Nealson, K. H. (1994). A biochemical study of the intermediary carbon metabolism of Shewanella putrefaciens. J. Bacteriol. 176, 3408–3411. doi: 10.1128/jb.176.11.3408-3411.1994
Sun, X., Wang, C., Li, Z., Wang, W., Tong, Y., and Wei, J. (2013). Microalgal cultivation in wastewater from the fermentation effluent in Riboflavin (B2) manufacturing for biodiesel production. Bioresour. Technol. 143, 499–504. doi: 10.1016/j.biortech.2013.06.044
Takeuchi, R., Sugimoto, Y., Kitazumi, Y., Shirai, O., Ogawa, J., and Kano, K. (2018). Electrochemical study on the extracellular electron transfer pathway from Shewanella strain Hac319 to electrodes. Anal. Sci. 34, 1177–1182. doi: 10.2116/analsci.18P237
Yoon, S., Sanford, R. A., and Löffler, F. E. (2013). Shewanella spp. use acetate as an electron donor for denitrification but not ferric iron or fumarate reduction. Appl. Environ. Microbiol. 79, 2818–2822. doi: 10.1128/AEM.03872-12
Keywords: extracellular electron transfer, electron-balance calculation, Shewanella decolorationis NTOU1, electron shuttle mediator, outer membrane cytochrome
Citation: Li S-L, Wang Y-J, Chen Y-C, Liu S-M and Yu C-P (2019) Chemical Characteristics of Electron Shuttles Affect Extracellular Electron Transfer: Shewanella decolorationis NTOU1 Simultaneously Exploiting Acetate and Mediators. Front. Microbiol. 10:399. doi: 10.3389/fmicb.2019.00399
Received: 02 October 2018; Accepted: 15 February 2019;
Published: 05 March 2019.
Edited by:
Fanghua Liu, Yantai Institute of Coastal Zone Research (CAS), ChinaReviewed by:
Shafeer Kalathil, University of Cambridge, United KingdomCopyright © 2019 Li, Wang, Chen, Liu and Yu. This is an open-access article distributed under the terms of the Creative Commons Attribution License (CC BY). The use, distribution or reproduction in other forums is permitted, provided the original author(s) and the copyright owner(s) are credited and that the original publication in this journal is cited, in accordance with accepted academic practice. No use, distribution or reproduction is permitted which does not comply with these terms.
*Correspondence: Chang-Ping Yu, Y3B5dUBudHUuZWR1LnR3
Disclaimer: All claims expressed in this article are solely those of the authors and do not necessarily represent those of their affiliated organizations, or those of the publisher, the editors and the reviewers. Any product that may be evaluated in this article or claim that may be made by its manufacturer is not guaranteed or endorsed by the publisher.
Research integrity at Frontiers
Learn more about the work of our research integrity team to safeguard the quality of each article we publish.