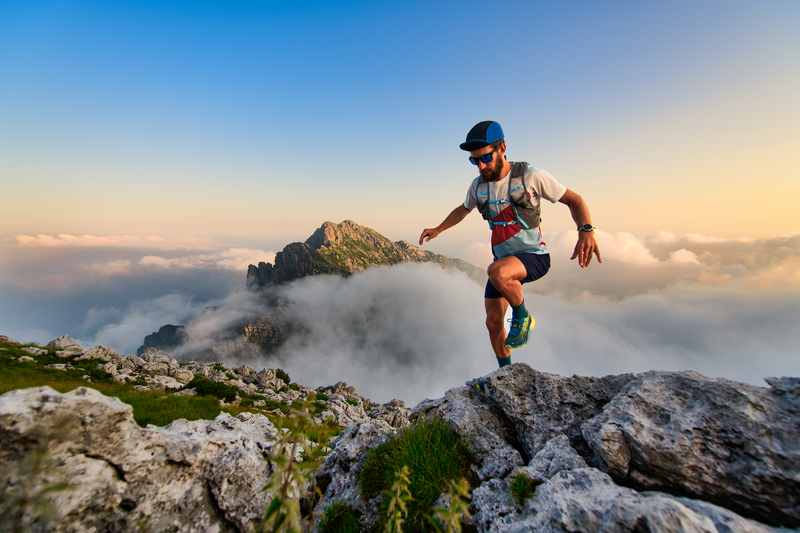
94% of researchers rate our articles as excellent or good
Learn more about the work of our research integrity team to safeguard the quality of each article we publish.
Find out more
ORIGINAL RESEARCH article
Front. Microbiol. , 04 March 2019
Sec. Aquatic Microbiology
Volume 10 - 2019 | https://doi.org/10.3389/fmicb.2019.00365
Bacterial-bioluminescence regulation is often associated with quorum sensing. Indeed, many studies have been made on this subject and indicate that the expression of the light-emission-involved genes is density dependent. However, most of these studies have concerned two model species, Aliivibrio fischeri and Vibrio campbellii. Very few works have been done on bioluminescence regulation for the other bacterial genera. Yet, according to the large variety of habitats of luminous marine bacteria, it would not be surprising to find different light-regulation systems. In this study, we used Photobacterium phosphoreum ANT-2200, a piezophilic bioluminescent strain isolated from Mediterranean deep-sea waters (2200-m depth). To answer the question of whether or not the bioluminescence of P. phosphoreum ANT-2200 is under quorum-sensing control, we focused on the correlation between growth and light emission through physiological, genomic and, transcriptomic approaches. Unlike A. fischeri and V. campbellii, the light of P. phosphoreum ANT-2200 immediately increases from its initial level. Interestingly, the emitted light increases at much higher rate at the low cell density than it does for higher cell-density values. The expression level of the light-emission-involved genes stays constant all along the exponential growth phase. We also showed that, even when more light is produced, when the strain is cultivated at high hydrostatic pressure, no change in the transcription level of these genes can be detected. Through different experiments and approaches, our results clearly indicate that, under the tested conditions, the genes, directly involved in the bioluminescence in P. phosphoreum ANT-2200, are not controlled at a transcriptomic level. Quite obviously, these results demonstrate that the light emission of the strain is not density dependent, which means not under quorum-sensing control. Through this study, we point out that bacterial-bioluminescence regulation should not, from now on, be always linked with the quorum-sensing control.
Quorum sensing (in short designed as QS) is the regulation of gene expression in response to fluctuations in cell-population density as defined by Miller and Bassler (2001). The QS is often described as a way to communicate for bacteria. Actually, it is more the capability of the bacterial population to synchronize an individual behavior using small hormone-like chemical molecules called autoinducers. QS has been discovered by studying bacterial bioluminescence even if it was not, by then, defined as QS yet (Kempner and Hanson, 1968; Nealson et al., 1970). Indeed, using cultures, freshly inoculated with the bioluminescent bacterium Aliivibrio fischeri (previously identified as Vibrio or Photobacterium fischeri), Kempner and Hanson (1968) observed that the exponential growth phase starts straight on, i.e., without any latency phase. On the contrary, the light emission does not evolve until half of the logarithmic phase where it, then, increases quickly. This phenomenon of shift, between growth and luminescence, has already been reported 20 years earlier (Farghaly, 1950), but the nature of this uncoupling was not hypothesized at that time. It was later shown that, in fact, the luminescence was initiated by the accumulation of the autoinducer, which is synthesized by the bacteria and excreted into the medium (Nealson et al., 1970; Rosson and Nealson, 1981). It results that the light seems to be emitted only from a certain cell density allowing an important amount of these autoinducers to be produced. Since these studies, the whole genetic mechanism for A. fischeri has been well described as summarized thereafter. The LuxI-LuxR QS system directly regulates the expression of the lux operon, required for the light production in A. fischeri (Figure 1). LuxI, the autoinducer synthase, produces the N-(3-oxohexanoyl) homoserine lactone (3OC6-HSL), which belongs to the acyl-homoserine-lactone (AHL) family (Eberhard et al., 1981; Engebrecht and Silverman, 1984). LuxR is the cytoplasmic autoinducer receptor/DNA binding transcriptional activator (Engebrecht et al., 1983). When the 3OC6-HSL reaches a critical, threshold concentration, it binds to LuxR and this complex activates the transcription of the lux operon (Stevens and Greenberg, 1997). Two additional QS systems, AinS-AinR and LuxS-LuxP/Q, indirectly control luminescence by modulating luxR transcription (Verma and Miyashiro, 2013). Firstly referenced to as “autoinduction” (Nealson et al., 1970), the term quorum sensing was coined later by Fuqua et al. (1994). It is now common knowledge among microbiologists that QS systems, analogous to the one described above, regulate gene expression in a great variety of gram-negative Bacteria (Hastings and Greenberg, 1999), as well as in gram-positive Bacteria (Miller and Bassler, 2001) and more recently in Archaea (Zhang et al., 2012). QS is also known to regulate the bacterial-pathogen behavior, including, for instance, virulence-gene expression, biofilm formation, swarming, antibiotic production, and antibiotic resistance. Recently, there have been extraordinary advances in our understanding of the genetic, genomic, biochemistry, and signal diversity of QS (Whiteley et al., 2017). For more details, see reviews about QS (Whitehead et al., 2001; Waters and Bassler, 2005; Li and Nair, 2012; Rajput et al., 2015).
Figure 1. Activation of the lux operon by LuxR and LuxI in Aliivibrio fischeri. (A) At low cell density, the autoinducers (3OC6-HSL – red dots), produced by LuxI, diffuse through the cell membrane into the growth medium. (B) As the cell growth continues, the autoinducers in the medium start to accumulate in a confined environment. A very low intensity of light can be detected. (C) When enough autoinducers have accumulated in the medium, they can re-enter the cell where they directly bind the LuxR protein to activate luxICDABEG expression. (D) High levels of autoinducers activate the luminescent system of A. fischeri. A high intensity of light can be detected. Based on Li and Nair (2012) (authorization of use license number 4454260086749).
Despite the long-standing interest in bioluminescent bacteria and the regulation of their light, the vast majority of the studies have concerned only two model organisms: A. fischeri and Vibrio campbellii (previously identified as Beneckea or Vibrio harveyi). However, luminous bacteria cannot be confined to these two species. Currently, about 25 species of luminous bacteria have been discovered across five genera in three families of the Gammaproteobacteria: Shewanellaceae (Shewanella), Enterobacteriaceae (Photorhabdus), and Vibrionaceae (Aliivibrio, Photobacterium, and Vibrio) (Dunlap and Urbanczyk, 2013). In marine environments, luminous bacteria are widely distributed. They can be isolated from seawater, sediments or suspended, and sinking particles, but bioluminescent bacteria are also well known as symbionts with animals (Nealson and Hastings, 1979; Andrews et al., 1984; Dunlap and Urbanczyk, 2013). Quite obviously, according to the large variety of habitats, it would not be surprising to find, in other luminous bacteria, light-regulation systems different than the two most studied model organisms, A. fischeri and V. campbellii. Due to its historic discovery with luminous bacteria, QS control is too often associated to all bioluminescent bacteria. Yet, some previous works on Photobacterium have suggested different kind of regulation (Katznelson and Ulitzur, 1977; Rosson and Nealson, 1981). To date, only rare studies have dealt with the bacterial-light regulation in Photobacterium species, as for instance the work of Dunn et al. (2015) on Photobacterium leiognathi.
In this study, we have chosen to use Photobacterium phosphoreum ANT-2200, a luminous piezomesophilic bacterium (Martini et al., 2013), which entire genome has been sequenced (Zhang et al., 2014). We focused on the relationship between growth and light emission through physiological, genomic and, transcriptomic approaches at atmospheric pressure. Since its growth and light emission have been well described previously at high hydrostatic pressure (Martini et al., 2013), we also tested lux genes expression at this condition.
Photobacterium phosphoreum ANT-2200 (16S rDNA GenBank accession number EU881910) was isolated from seawater collected in the Northwestern Mediterranean Sea at the ANTARES neutrino telescope site (42°54′N/06°06′E) at 2200-m depth (13°C). See Al Ali et al. (2010) for details. For the first experiment, conducted at atmospheric pressure, the strain was cultured in a mineral-salt medium (ONR7a modified), close to the environmental composition and requiring the preparation of three different solutions as described hereafter. Solution 1: 22.79 g NaCl, 3.98 g Na2SO4, 0.72 g KCl, 0.8 g NH4Cl, 0.2 g Na2HPO4.2H2O, 83 mg NaBr, 2.6 mg NaF, 31 mg NaHCO3, 27 mg H3BO3, 2 mL glycerol, and 10 mL Balch oligo-element (Balch et al., 1979) in 700 mL of distilled water (pH 7.5). Solution 2: 11 g MgCl2.6H2O, 1.46 g CaCl2.2H2O, and 24 mg SrCl2.6H2O in 300 mL of distilled water. Solution 3: 0.025 g FeSO4.7H2O in 10 mL of distilled water. The three solutions were autoclaved separately for 20 min at 121°C. Solution 1, solution 2 and 1 mL of the solution 3 were mixed after autoclave and 20 mL of Balch vitamins were added (Balch et al., 1979). Cultures were grown in 100-mL flasks containing 50 mL ONR7a modified medium, in a 19°C-temperature-controlled room with orbital shaking at 150 rpm.
Bacterial growth was estimated by measuring the optical density at 600 nm in a Milton Roy Spectronic 401 spectrophotometer. The light emission was measured in a 96-well plate, with 150 μL of culture, in a luminometer Luminoskan Ascent (Lab Systems, Ascent Software Version 2.6), with the following parameters: PMT sensibility: 800 V, shaking: 10 s, reading time: 1 s. The light intensity is evaluated in relative light unit (RLU). Samples were taken regularly all along the growth. Each filtered volume was adjusted depending on the increase of the cell density. For samples 1, 2, 3, 4, and 5, 200, 100, 35, 20, and 7 mL were filtered on 0.2 μm cellulose-nitrate filters (Whatman), respectively. The experiment was done in duplicate. Less than 1% and less than 5% difference between both experiments were measured for the OD and the bioluminescence, respectively.
For these experiments, due to the specificities of culturing under high-pressure conditions, and in order to reproduce the exact same experiment than Martini et al. (2013), a medium, richer than the ONR7a, was used. Procedures for culturing were performed as described by Martini et al. (2013). Briefly, cultures were incubated at 0.1 and 22 MPa of pressure and at 13°C. For the 22-MPa condition, cultures were placed into high-pressure bottles (HPBs). Each HPB contains three identical 5-mL plastic syringes and one 145-mL glass flask equipped with a rubber-septum cap to transmit the pressure. Each of the items contained a mix of 3/4 culture and 1/4 oxygen-saturated FluorinertTM FC-72 (3 M). FluorinertTM FC-72 was used as the oxygen supplier to ensure the growth and the luminescence of the bacterial strain in closed conditions (see Martini et al., 2013). The syringes were used for growth estimation by measuring the optical density (OD600 nm) and the flask was used for the RT-qPCR experiments. In order to avoid decompression-recompression of the samples, each HPB corresponded to one incubation time. Three samples were chosen as described in results. Each filtered volume was adjusted depending on the increase of the cell density. For samples T1, T2, and T3, 50, 35, and 25 mL were filtered on 0.2 μm cellulose-nitrate filters (Whatman), respectively.
RNA extraction was performed with the High Pure RNA Isolation Kit (Roche Applied Science, United States) according to the supplier’s recommendations. The extracted RNAs were quantified at 260 nm using the BioSpec-Nano spectrophotometer (Shimadzu). The cDNAs were synthesized from the total extracted RNAs using random primers and the GoScript Reverse Transcription System (Promega) according to the supplier’s recommendations. For each sample, the amount of RNAs, added to the reaction mixture, was adjusted so that the amount of RNAs was identical for each sample. Thus, despite the difference in sampled volumes and cell density, the same quantity of RNAs from the five samples was used for the reverse transcription. The quantity of the various target cDNAs was quantified by qPCR (qPCR Master Mix, Promega) according to the supplier’s recommendations. Experiences were made using the CFX96 Bio-Rad and the associated software. Various plasmids were constructed by cloning the target genes in the pGEM-T-easy vector (Promega) according to the supplier’s recommendations (Supplementary Table S1). They were used for constituting the standard curves for qPCR allowing the quantification of the synthetized cDNAs. Each extracted RNA were analyzed through six technical replicates (three independent reverse transcriptions and two qPCR analyses per cDNAs).
Figure 2 represents the lux operon and its organization in the genome of three luminous marine bacteria: A. fischeri, V. campbellii, and P. phosphoreum ANT-2200. The genes luxCDABEG are common to all of them and code for the luciferase (AB) and for the fatty-acid-reductase polypeptides (CDEG) (Meighen, 1993). Photobacterium strains present some additional genes. For example, in P. phosphoreum ANT-2200, and in the majority of other bioluminescent Photobacterium species, the luxF gene is present between luxB and luxE (Mancini et al., 1988; Soly et al., 1988). Not found, to date, in any Aliivibrio, Vibrio or other bioluminescent bacteria, the luxF gene appears to be confined to the genus Photobacterium (Mancini et al., 1989; Ast and Dunlap, 2004). The luxF gene encodes a protein which is suggested to increase the emitted luminous intensity by binding to a bioluminescence inhibitor, the myrFMN (Bergner et al., 2015). Furthermore, Photobacterium species possess also the rib genes, involved in the riboflavin synthetase, which are located directly downstream luxG (Lee et al., 1994; Lin et al., 2001). The rib genes belong to the lux operon since they are co-transcribed from a promoter located upstream luxC (Sung and Lee, 2004). Such an organization is not surprising since riboflavin is the direct precursor of riboflavin 5′-mono-phosphate (FMN), the oxidized form of the substrate (FMNH2) used by bacteria in the bioluminescence reaction. This clearly underlines the importance of the rib genes and their links to the lux genes and the light reaction. The classic rib-gene organization in Photobacterium is rib E-B-H-A. However, it is worth noting that the absence of ribE is described for some P. phosphoreum strains (Urbanczyk et al., 2011; Dunlap, 2014) and not in some others (Lee et al., 1994; Sung and Lee, 2004; Zhang et al., 2014). Interestingly, in V. campbellii, only one gene, ribB, coding for a key step of the riboflavin-complex synthetase, can be found (Swartzman, 1990; Dunlap, 2014).
Figure 2. Comparison of the organization of the light-emission-involved genes in different luminous species. A. fischeri possesses the two regulatory genes (luxI/luxR), involved in the quorum sensing, in the vicinity of the lux operon. Whereas, at the same location, there are absent in V. campbellii and Photobacterium spp. P. phosphoreum ANT-2200, and P. leiognathi KNH6 organizations are identical.
One of the main differences in the bioluminescence-gene organization concerns the regulatory genes. Some species, such as A. fischeri, present the regulatory genes, luxI and luxR, upstream the luxCDABEG cluster. In this case, the luxI gene is transcribed with luxC and therefore belongs to the lux operon. On the contrary, luxR is located further upstream the lux operon and is transcribed in the opposite direction. Both genes are involved in the quorum-sensing-regulation mechanism. Remarkably, in all the luminous Photobacterium species, luxI and luxR are absent upstream luxC (Dunlap, 2014). In P. phosphoreum ANT-2200, instead of these genes, other ones precede the lux operon: lumP, also called luxL, and lumQ. They form the lumazine operon, which runs in the opposite direction of the lux genes. The same organization was found in P. leiognathi (Lin et al., 1995). The importance of LumP in P. phosphoreum was established prior to its localization, and was shown to be involved in the wavelength, as well as in the intensity of the light emission (Gast and Lee, 1978; Prasher et al., 1990). On the contrary, the function of LumQ remains unknown (Dunlap, 2014), although it is thought to code for a DNA-binding protein (Lin et al., 1995). Nevertheless, the only absence of the regulatory genes in the vicinity of the lux operon does not mean an absence of QS. Indeed, as observed in Photobacterium species, both genes are absent in V. campbellii at this location. However, in this organism, a lag time before the increase of the light emission has been described (Greenberg et al., 1979). It also has been observed that a high concentration of autoinducers permits the activation of the lux-operon transcription. The involved mechanism is now well described and is quite different than A. fischeri one (Bassler et al., 1997; Whitehead et al., 2001; Defoirdt et al., 2008).
In view of these various examples, the only organization of the genes in P. phosphoreum ANT-2200 is not enough to confirm an independent-cell-density light control. In this goal, we designed a follow-up of the luxrib-gene expressions and bioluminescence kinetics throughout the growth.
The growth and luminescence of P. phosphoreum ANT-2200 were measured over time in a mineral-salt medium (ONR7a) with glycerol as the only source of carbon and results are presented in Figure 3A. There is no lag time between the light emission and the growth as already observed for P. leiognathi. These results are in contrast with A. fischeri and V. campbellii results generally obtained, in which light emission appears when a certain quantity of cells is reached (Kempner and Hanson, 1968; Nealson et al., 1970; Katznelson and Ulitzur, 1977; Nealson, 1977; Engebrecht et al., 1983; Makemson, 1986; Meighen, 1999; Nackerdien et al., 2008). A light emission starting to increase at the same time than the growth means that the microorganism emits light even at really low cell density, which is not coherent with a density-dependent light control (Nealson, 1977).
Figure 3. Growth, bioluminescence, and luxrib-gene-expression kinetics of P. phosphoreum ANT-2200 throughout the growth at atmospheric pressure. (A) Growth ( black) and bioluminescence (• blue) kinetics of P. phosphoreum ANT-2200 on ONR7a modified medium. Orange dots represent the sampling times S1 to S5. (B) Light emission of P. phosphoreum ANT-2200 according to the cell density. (C) Light emission of A. fischeri according to the cell density (Nealson, 1977). Authorization of use license number 4454241308645. (D) Expression level of the genes luxC, luxA, luxF, and ribE for sampling times S1–S5.
In order to compare the light-emission kinetics of P. phosphoreum ANT-2200 with these of luminous bacteria under quorum sensing, we plotted the emitted light according to the cell density (Figure 3B), as Nealson (1977) did for A. fischeri (Figure 3C). For P. phosphoreum ANT-2200, the pattern completely contrasts to the one obtained for A. fischeri. Indeed, for the later, the light decreases from its initial level until the bacterial population reaches the required density – here 0.1 OD600 nm – and then, considerably increases. On the contrary, for P. phosphoreum ANT-2200, the light, according to the cell density, strongly increases directly from its initial level. It is worth noting that, not only, it starts immediately, but also, it increases at a much higher rate at the low cell density – between OD600 nm values of 0.003–0.01 – than it does for superior cell-density values – from 0.01 to 0.2. If it has been already suggested that Photobacterium spp. do not wait any quorum to emit light, this is, to our knowledge, the first report of higher specific luminescence for low rather than high cell density.
While the luminescence increases rapidly from the very beginning of the growth, we also noticed a slowdown period, from 5 to 14 h (Figure 3A), before increasing again to reach the maximum intensity at the end of the exponential growth phase after 25 h. Such slowdown period of light emission has already been described for P. leiognathi (Katznelson and Ulitzur, 1977; Dunn et al., 2015) when the cells were grown in rich-organic medium. Based on a previous work (Eberhard, 1972), they hypothesized the presence of an inhibitor contained into the rich-organic medium. In our study and using a minimal medium, the slowdown period of light emission could be explained by a molecule that accumulates in the medium of culture after a few hours before not being available anymore. Only bioluminescence would then be affected, since no slowdown phase is observed on the growth curve during this same period (Figure 3A). Amongst the potential molecules, one could imagine that myrFMN, by-product of the bioluminescence reaction, would compete with the substrate – as described by Wei et al. (2001) – before being scavenged by LuxF (Bergner et al., 2015), therefore allowing the light to increase again.
The phenotype of P. phosphoreum ANT-2200 presents so many differences with those of QS-controlled bioluminescent bacteria that it seems obvious to complete the follow-up of growth and light emission of P. phosphoreum ANT-2200 by a genetic approach.
A quantitative reverse-transcription PCR (RT-qPCR) was applied to monitor, all along the growth, the mRNA-expression level of the luxrib genes, the light-emission-involved genes in P. phosphoreum ANT-2200. Cells in different conditions, or under the influence of specific compounds, differ by their gene expression patterns and thus by their mRNA pools. One of the most important technique for the accurate quantification of gene expression is the fluorescent RT-qPCR (Muller et al., 2002). Indeed, RT-qPCR has become a routine and robust approach for measuring the expression of genes of interest (VanGuilder et al., 2008). In this objective, regular samples have been taken throughout the growth (orange dots in Figure 3A). Four genes were chosen for this experiment: luxC, the first gene of the operon, luxA, which codes for the α-subunit of the luciferase, luxF, specific to the Photobacterium genus and ribE, first gene of the rib cluster. In order to be sure to accurately compare the expression levels of each gene between the samples, they were normalized with the rpoD-gene expression. rpoD is an house-keeping gene and is often used to resolve phylogenetic analyses in Photobacterium clade (Ast et al., 2007; Bjornsdottir-Butler et al., 2016). The genes luxC, luxA, luxF, and ribE display a constant expression for every sample (Figure 3D) showing that they are not regulated during the growth in this condition. Furthermore, the first sample (S1) was performed at a very low optical density – 0.007 OD600 nm – and yet, the mRNA amounts of all the targeted genes were high enough to be detected. Constant mRNA levels, even at low density, go against the hypothesis saying that a minimum cell density is required to activate the lux-operon transcription, which is coherent with the physiological observations.
Our results, obtained with P. phosphoreum ANT-2200, combined with recent studies with P. leiognathi (Dunn et al., 2015), confirm the absence of QS regulation for the bioluminescence in this genus. However, in some conditions, light intensity may vary. Therefore, despite the absence of regulation by the cell density, could the expression of the luxrib operon be regulated by other factors?
A previous study (see Figure 7 in Martini et al., 2013) showed that P. phosphoreum ANT-2200 produces three-times more light at 22 MPa – the in situ pressure condition – than at 0.1 MPa – the atmospheric pressure. The authors demonstrated that not only the bioluminescence was higher, under pressure, but also, that it occurs later on during the growth, comparatively to the results obtained at atmospheric pressure. In order to estimate if this variation can be related to a transcriptional regulation, we performed a RT-qPCR to compare the expression of the luxrib genes at 22 MPa vs. 0.1 MPa. Three sampling times have been chosen according to the light emission differences between the cultures. One corresponds to the initial time (T1) when the light emission is the same for both cultures. The two others correspond to the times when the bioluminescence-emission peak is reached at 0.1 and 22 MPa of pressure (T2 and T3). Despite a luminescence production three-times higher under 22 MPa, the expression levels of the genes luxC, luxA, luxF, and ribE do not show higher value than at 0.1 MPa (Figure 4). Therefore, the higher light production at 22 MPa cannot be explained by a transcriptional regulation of the luxrib genes. Other hypotheses could explain such a phenomenon. For example, the pressure role could still happen at a transcriptional level by regulating the expression of genes coding for proteins indirectly involved in the light emission. On the other hand, the pressure could also impact the structure and/or the function of proteins (Mozhaev et al., 1996), whether they are directly involved in the light emission or just associated to the quality of this light.
Figure 4. Comparison luxrib-gene-expression at atmospheric vs. high pressure of P. phosphoreum ANT-2200. Expression level of the genes luxC, luxA, luxF, and ribE at atmospheric (ATM, 0.1 MPa, blue) and at high hydrostatic pressure (HP, 22 MPa, red), for sampling times T1–T3. T1 corresponds to the initial time when the light emission is the same for both conditions (approximately 4 h). T2 and T3 correspond to the times when a bioluminescence-emission peak is reached at ATM (approximately 13 h) and HP (approximately 17 h) conditions, respectively. Reference bioluminescence and growth curves are available in Martini et al. (2013).
Bioluminescence is considered to be a major factor in ecological interactions since 76% of the observed individuals in the water column have the capability of emitting light (Martini and Haddock, 2017). Knowledge of control mechanisms is important to the understanding of the selective advantage of luminescence and the ecology of the bacteria. With the discovering of the quorum sensing and the requirement of a minimum cell density to activate the bacterial light system, it was mentioned that free-living bacteria may have no ecological significance (Hastings and Greenberg, 1999). However, this hypothesis could be conceivable only if we considered that all bioluminescent bacteria are under quorum-sensing control.
Often described as the brightest species (Meighen, 1999; Cheng et al., 2010) and associated to midwaters and deep-sea habitats (Ruby and Morin, 1978; Urbanczyk et al., 2011), P. phosphoreum is found free-living or attached-to-particles in the seawater (Andrews et al., 1984) but never in a specialized light organ (Herring, 1982; Urbanczyk et al., 2011). As a matter of fact, P. phosphoreum ANT-2200 was found free in the deep waters of the Mediterranean Sea during a high level of bioluminescence (in relationship with a strong convection event) detected by the underwater neutrino telescope ANTARES (Tamburini et al., 2013). This result is coherent with the description of this species as the dominant bioluminescent microorganism in the Mediterranean Sea (Gentile et al., 2009). Moreover, the genus of Photobacterium has also been found as active even during a stable hydrological period suggesting an ecological benefit of bioluminescence (Martini et al., 2016). This finding agrees with one hypothesis on the ecological function of free-living bioluminescent bacteria: promoting their propagation and dispersal. According to this hypothesis, luminous bacteria growing on food particles visually mark their presence for higher trophic organisms, such as fish or zooplankton. These glowing particles have been shown to be preferentially ingested (Zarubin et al., 2012). The consumption of the particles by the predator would provide the bacteria a more suitable environment regarding the growth conditions and the nutrient accessibility (Nealson and Hastings, 1979). Therefore, attached bacteria are transferred to the nutritious guts of these macroorganisms, where they survive digestion and gain effective means for growth and dispersal. Thus, bioluminescence would be highly beneficial for marine bacteria, especially in food-deprived environments like the deep sea. This hypothesis can only be true if the light can be produced without QS control since, for free-living microorganisms, the conditions allowing the accumulation of the pheromone-like molecules can never be reached. To be able to produce light at very low concentration (therefore without QS control) will give P. phosphoreum this advantage.
Through different experiments and approaches, our results clearly indicate that, under the tested conditions, the genes, directly involved in the bioluminescence in P. phosphoreum ANT-2200, are not controlled at a transcriptomic level. Quite obviously, this absence of regulation shows the absence of a cell-density control mechanism coherent with its free-living behavior. Because numerous studies about QS are linked to bioluminescence and due to its historic discovery with luminous bacteria, QS control is too often associated to all bioluminescent bacteria. We showed that this is clearly not the case with all light-emitting microorganisms, and that such automatic association should now be avoided.
All datasets generated for this study are included in the manuscript and/or the Supplementary Files.
LT performed most of the experiments. CT and LC participated in the experimental design. CB and LC participated to experimental work on RT-qPCR. The high-pressure experiments were carried out by LT, MG, and CT. LT, GS, CT, and LC interpreted the results and wrote the manuscript. All authors reviewed the final version of the manuscript.
LT was supported by a doctoral grant “Région PACA/TANGRAM.” We gratefully acknowledge support from IRD, CNRS (Project EC2CO “HEMERA”), and Aix Marseille University. The project leading to this publication has received funding from European FEDER Fund under project 1166-39417.
The authors declare that the research was conducted in the absence of any commercial or financial relationships that could be construed as a potential conflict of interest.
The authors wished to thank Sophie Guasco for helping with some experiments. The laboratories, where all the experiments were performed, are in compliance with ISO9001-2015.
The Supplementary Material for this article can be found online at: https://www.frontiersin.org/articles/10.3389/fmicb.2019.00365/full#supplementary-material
TABLE S1 | Primer sequences and features of the target genes in P. phosphoreum ANT2200, used in RT-qPCR.
Al Ali, B., Garel, M., Cuny, P., Miquel, J. C., Toubal, T., Robert, A., et al. (2010). Luminous bacteria in the deep-sea waters near the ANTARES underwater neutrino telescope (Mediterranean Sea). Chem. Ecol. 26, 57–72. doi: 10.1080/02757540903513766
Andrews, C. C., Karl, D. M., Small, L. F., and Fowler, S. W. (1984). Metabolic-activity and bioluminescence of oceanic fecal pelletsand sediment trap particles. Nature 307, 539–541. doi: 10.1038/307539a0
Ast, J. C., Cleenwerck, I., Engelbeen, K., Urbanczyk, H., Thompsom, F. L., De Vos, P., et al. (2007). Photobacterium kishitanii sp. nov., a luminous marine bacterium symbiotic with deep-sea fishes. Int. J. Syst. Evol. Microbiol. 57, 2073–2078. doi: 10.1099/ijs.0.65153-0
Ast, J. C., and Dunlap, P. V. (2004). Phylogenetic analysis of the lux operon distinguishes two evolutionarily distinct clades of Photobacterium leiognathi. Arch. Microbiol. 181, 352–361. doi: 10.1007/s00203-004-0663-7
Balch, W. E., Fox, G. E., Magrum, L. J., Woese, C. R., and Wolfe, R. S. (1979). Methanogens: reevaluation of a unique biological group. Microbiol. Rev. 43, 260–296. doi: 10.1016/j.watres.2010.10.010
Bassler, B. L., Greenberg, E. P., and Stevens, A. M. (1997). Cross-species induction of luminescence in the quorum- sensing bacterium Vibrio harveyi. J. Bacteriol. 179, 4043–4045. doi: 10.1128/jb.179.12.4043-4045.1997
Bergner, T., Tabib, C. R., Winkler, A., Stipsits, S., Kayer, H., Lee, J., et al. (2015). Structural and biochemical properties of LuxF from Photobacterium leiognathi. Biochim. Biophys. Acta Proteins Proteomics 1854, 1466–1475. doi: 10.1016/j.bbapap.2015.07.008
Bjornsdottir-Butler, K., McCarthy, S. A., Dunlap, P. V., and Benner, R. A. (2016). Photobacterium angustum and Photobacterium kishitanii, psychrotrophic highlevel histamine-producing bacteria indigenous to tuna. Appl. Environ. Microbiol. 82, 2167–2176. doi: 10.1128/AEM.02833-15
Cheng, C. Y., Kuo, J. T., Lin, Y. C., Liao, Y. R., and Chung, Y. C. (2010). Comparisons of Vibrio fischeri, Photobacterium phosphoreum, and recombinant luminescent using Escherichia coli as BOD measurement. J. Env. Sci. Heal. A Tox. Hazard Subst. Env. Eng. 45, 233–238. doi: 10.1080/10934520903430020
Defoirdt, T., Boon, N., Sorgeloos, P., Verstraete, W., and Bossier, P. (2008). Quorum sensing and quorum quenching in Vibrio harveyi: lessons learned from in vivo work. ISME J. 2, 19–26. doi: 10.1038/ismej.2007.92
Dunlap, P. V. (2014). Biochemistry and genetics of bacterial bioluminescence. Biolumin. Fundam. Appl. Biotechnol. 1, 111–151. doi: 10.1007/978-3-662-43385-0
Dunlap, P. V., and Urbanczyk, H. (2013). “Luminous bacteria,” in The Prokaryotes, eds E. F. DeLong, S. Lory, E. Stackebrandt, and F. Thompson (Berlin: Springer), 495–528. doi: 10.1007/978-3-642-30141-4_75
Dunn, A. K., Rader, B. A., Stabb, E. V., and Mandel, M. J. (2015). Regulation of bioluminescence in Photobacterium leiognathi strain KNH6. J. Bacteriol. 197:JB.00524-15. doi: 10.1128/JB.00524-15
Eberhard, A. (1972). Inhibition and activation of bacterial luciferase synthesis. J. Bacteriol. 109, 1101–1105.
Eberhard, A., Burlingame, A. L., Eberhard, C., Kenyon, G. L., Nealson, K. H., and Oppenheimer, N. J. (1981). Structural identification of autoinducer of Photobacterium fischeri luciferase. Biochemistry 20, 2444–2449. doi: 10.1021/bi00512a013
Engebrecht, J., Nealson, K., and Silverman, M. (1983). Bacterial bioluminescence: isolation and genetic analysis of functions from Vibrio fischeri. Cell 32, 773–781. doi: 10.1016/0092-8674(83)90063-6
Engebrecht, J., and Silverman, M. (1984). Identification of genes and gene products necessary for bacterial bioluminescence. Proc. Natl. Acad. Sci. U.S.A. 81, 4154–4158. doi: 10.1073/pnas.81.13.4154
Farghaly, A. H. (1950). Factors influencing the growth and light production of luminous bacteria. J. Cell. Comp. Physiol. 36, 165–183. doi: 10.1002/jcp.1030360205
Fuqua, W. C., Winans, S. C., and Greenberg, E. P. (1994). Quorum sensing in bacteria: the LuxR-LuxI family of cell density- responsive transcriptional regulators. J. Bacteriol. 176, 269–275. doi: 10.1128/jb.176.2.269-275.1994
Gast, R., and Lee, J. (1978). Isolation of the in vivo emitter in bacterial bioluminescence. Proc. Natl. Acad. Sci. U.S.A 75, 833–837. doi: 10.1073/pnas.75.2.833
Gentile, G., De Luca, M., Denaro, R., La Cono, V., Smedile, F., Scarfi, S., et al. (2009). PCR-based detection of bioluminescent microbial populations in Tyrrhenian Sea. Deep. Res. Part II Top. Stud. Oceanogr. 56, 763–767. doi: 10.1016/j.dsr2.2008.07.023
Greenberg, E. P., Hastings, J. W., and Ulitzur, S. (1979). Induction of luciferase synthesis in Beneckea harveyi by other marine bacteria. Arch. Microbiol. 120, 87–91. doi: 10.1007/BF00409093
Hastings, J. W., and Greenberg, E. P. (1999). Quorum sensing: the explanation of a curious phenomenon reveals a common characteristic of bacteria. Society 181, 2667–2669.
Herring, P. J. (1982). Aspects of bioluminescence of fishes. Ocean. Mar. Biol. Annu. Rev. 20, 415–470.
Katznelson, R., and Ulitzur, S. (1977). Control of luciferase synthesis in a newly isolated strain of Photobacterium leiognathi. Arch. Microbiol. 115, 347–351. doi: 10.1007/BF00446462
Kempner, E. S., and Hanson, F. E. (1968). Aspects of light production by Photobacterium fischeri. J. Bacteriol. 95, 975–979.
Lee, C. Y., O’Kane, D. J., and Meighen, E. A. (1994). Riboflavin synthesis genes are linked with the lux operon of Photobacterium phosphoreum. J. Bacteriol. 176, 2100–2104. doi: 10.1128/jb.176.7.2100-2104.1994
Li, Z., and Nair, S. K. (2012). Quorum sensing: how bacteria can coordinate activity and synchronize their response to external signals? Protein Sci. 21, 1403–1417. doi: 10.1002/pro.2132
Lin, J. W., Chao, Y. F., and Weng, S. F. (2001). Riboflavin synthesis genes ribE, ribB, ribH, ribA reside in the lux operon of Photobacterium leiognathi. Biochem. Biophys. Res. Commun. 284, 587–595. doi: 10.1006/bbrc.2001.5013
Lin, J. W., Yu, K. Y., Chao, Y. F., and Weng, S. F. (1995). The lumQ gene is linked to the lumP gene and the lux operon in Photobacterium leiognathi. Biochem. Biophys. Res. Commun. 217, 684–695. doi: 10.1006/bbrc.1995.2828
Makemson, J. C. (1986). Luciferase dependent oxygen consumption by bioluminescent vibrios. J. Bacteriol. 165, 461–466. doi: 10.1128/jb.165.2.461-466.1986
Mancini, J. A., Boylan, M., Soly, R., Ferri, S., Szittner, R., and Meighen, E. A. (1989). Organization of the lux genes of Photobacterium phosphoreum. J. Bioluminesc. Chemiluminesc. 3, 201–205. doi: 10.1002/bio.1170030407
Mancini, J. A., Boylan, M., Soly, R. R., Graham, A. F., and Meighen, E. A. (1988). Cloning and expression of the Photobacterium phosphoreum luminescence system demonstrates a unique lux gene organization. J. Biol. Chem. 263, 14308–14314.
Martini, S., Al Ali, B., Garel, M., Nerini, D., Grossi, V., Pacton, M., et al. (2013). Effects of hydrostatic pressure on growth and luminescence of a piezomesophilic luminous bacteria Photobacterium phosphoreum ANT-2200. PLoS One 8:e66580. doi: 10.1371/journal.pone.0066580
Martini, S., and Haddock, S. H. D. (2017). Quantification of bioluminescence from the surface to the deep sea demonstrates its predominance as an ecological trait. Sci. Rep. 7:45750. doi: 10.1038/srep45750
Martini, S., Michotey, V., Casalot, L., Bonin, P., Guasco, S., Garel, M., et al. (2016). Bacteria as part of bioluminescence emission at the deep ANTARES station (North-Western Mediterranean Sea) during a one-year survey. Deep Sea Res. Part I Oceanogr. Res. Pap. 116, 33–40. doi: 10.1016/j.dsr.2016.07.014
Meighen, E. A. (1993). Bacterial bioluminescence: organization, regulation, and application of the lux genes. FASEB J. 7, 1016–1022. doi: 10.1096/fasebj.7.11.8370470
Meighen, E. A. (1999). Autoinduction of light emission in different species of bioluminescent bacteria. Luminescence 14, 3–9. doi: 10.1002/(SICI)1522-7243(199901/02)14:1<3::AID-BIO507>3.0.CO;2-4
Miller, M. B., and Bassler, B. L. (2001). Quorum sensing in bacteria. Annu. Rev. Microbiol. 55, 165–199. doi: 10.1146/annurev.micro.55.1.165
Mozhaev, V. V., Heremans, K., Frank, J., Masson, P., and Balny, C. (1996). High pressure effects on protein structure and function. Proteins Struct. Funct. Genet. 24, 81–91. doi: 10.1002/(SICI)1097-0134(199601)24:1<81::AID-PROT6>3.0.CO;2-R
Muller, P. Y., Janovjak, H., Miserez, A. R., and Dobbie, Z. (2002). Processing of gene expression data genered by quantitative real-time RT-PCR. Biotechniques 32, 1372–1378. doi: 10.1007/s10551-011-0963-1
Nackerdien, Z. E., Keynan, A., Bassler, B. L., Lederberg, J., and Thaler, D. S. (2008). Quorum sensing influences Vibrio harveyi growth rates in a manner not fully accounted for by the marker effect of bioluminescence. PLoS One 3:e1671. doi: 10.1371/journal.pone.0001671
Nealson, K. H. (1977). Autoinduction of Bacterial Luciferase occurance, mechanisms and significance. Arch. Microbiol 112, 73–79. doi: 10.1007/BF00446657
Nealson, K. H., and Hastings, J. W. (1979). Bacterial bioluminescence: its control and ecological significance. Microbiol. Rev. 43, 496–518.
Nealson, K. H., Platt, T., and Hastings, J. W. (1970). Cellular control of the synthesis and activity of the bacterial luminescent system. J. Bacteriol. 104, 313–322.
Prasher, D. C., O’Kane, D., Lee, J., and Woodward, B. (1990). The lumazine protein gene in Photobacterium phosphoreum is linked to the lux operon. Nucleic Acids Res. 18, 6450. doi: 10.1093/nar/18.21.6450
Rajput, A., Kaur, K., and Kumar, M. (2015). SigMol: repertoire of quorum sensing signaling molecules in prokaryotes. Nucleic Acids Res. 44, D634–D639. doi: 10.1093/nar/gkv1076
Rosson, R. A., and Nealson, K. H. (1981). Autoinduction of bacterial bioluminescence in a carbon limited chemostat. Arch. Microbiol. 129, 299–304. doi: 10.1007/BF00414701
Ruby, E. G., and Morin, J. G. (1978). Specificity of symbiosis between deep-sea fishes and psychrotrophic luminous bacteria. Deep Res. 25, 161–167. doi: 10.1016/0146-6291(78)90003-6
Soly, R. R., Mancini, J. A., Ferri, S. R., Boylan, M., and Meighen, E. A. (1988). A new lux gene in bioluminescent bacteria codes for a protein homologous to the bacterial luciferase subunits. Biochem. Biophys. Res. Commun. 155, 351–358. doi: 10.1016/S0006-291X(88)81092-1
Stevens, A. M., and Greenberg, E. P. (1997). Quorum sensing in Vibrio fischeri: essential elements for activation of the luminescence quorum sensing in Vibrio fischeri: essential elements for activation of the luminescence genes. J. Bacteriol. 179, 557–562. doi: 10.1111/j.1462-2920.2008.01755.x
Sung, N. D., and Lee, C. Y. (2004). Coregulation of lux genes and riboflavin genes in bioluminescent bacteria of Photobacterium phosphoreum. J. Microbiol. 42, 194–199.
Swartzman, E. (1990). Delineation of the transcriptional boundaries of the zux operon of Vibrio harueyi demonstrates the presence of two new zux genes. J. Biol. Chem. 265, 3513–3517.
Tamburini, C., Canals, M., Durrieu de Madron, X., Houpert, L., Lefèvre, D., Martini, S., et al. (2013). Deep-sea bioluminescence blooms after dense water formation at the ocean surface. PLoS One 8:e67523. doi: 10.1371/journal.pone.0067523
Urbanczyk, H., Ast, J. C., and Dunlap, P. V. (2011). Phylogeny, genomics, and symbiosis of photobacterium. FEMS Microbiol. Rev. 35, 324–342. doi: 10.1111/j.1574-6976.2010.00250.x
VanGuilder, H. D., Vrana, K. E., and Freeman, W. M. (2008). Twenty-five years of quantitative PCR for gene expression analysis. Biotechniques 44, 619–626. doi: 10.2144/000112776
Verma, S. C., and Miyashiro, T. (2013). Quorum sensing in the squid-vibrio symbiosis. Int. J. Mol. Sci. 14, 16386–16401. doi: 10.3390/ijms140816386
Waters, C. M., and Bassler, B. L. (2005). QUORUM SENSING: cell-to-cell communication in bacteria. Annu. Rev. Cell Dev. Biol. 21, 319–346. doi: 10.1146/annurev.cellbio.21.012704.131001
Wei, C. J., Lei, B., and Tu, S. C. (2001). Characterization of the binding of Photobacterium phosphoreum P-flavin by Vibrio harveyi luciferase. Arch. Biochem. Biophys. 396, 199–206. doi: 10.1006/abbi.2001.2612
Whitehead, N. A., Barnard, A. M. L., Slater, H., Simpson, N. J. L., and Salmond, G. P. C. (2001). Quorum-sensing in gram-negative bacteria. FEMS Microbiol. Rev. 25, 365–404. doi: 10.1111/j.1574-6976.2001.tb00583.x
Whiteley, M., Diggle, S. P., and Greenberg, E. P. (2017). Progress in and promise of bacterial quorum sensing research. Nature 551, 313–320. doi: 10.1038/nature24624
Zarubin, M., Belkin, S., Ionescu, M., and Genin, A. (2012). From the cover: bacterial bioluminescence as a lure for marine zooplankton and fish. Proc. Natl. Acad. Sci. 109, 853–857. doi: 10.1073/pnas.1116683109
Zhang, G., Zhang, F., Ding, G., Li, J., Guo, X., Zhu, J., et al. (2012). Acyl homoserine lactone-based quorum sensing in a methanogenic archaeon. ISME J. 6, 1336–1344. doi: 10.1038/ismej.2011.203
Keywords: bacterial bioluminescence, quorum sensing, Photobacterium phosphoreum, lux genes, high pressure
Citation: Tanet L, Tamburini C, Baumas C, Garel M, Simon G and Casalot L (2019) Bacterial Bioluminescence: Light Emission in Photobacterium phosphoreum Is Not Under Quorum-Sensing Control. Front. Microbiol. 10:365. doi: 10.3389/fmicb.2019.00365
Received: 19 October 2018; Accepted: 12 February 2019;
Published: 04 March 2019.
Edited by:
James Cotner, University of Minnesota Twin Cities, United StatesReviewed by:
Jürgen Tomasch, Helmholtz Association of German Research Centres (HZ), GermanyCopyright © 2019 Tanet, Tamburini, Baumas, Garel, Simon and Casalot. This is an open-access article distributed under the terms of the Creative Commons Attribution License (CC BY). The use, distribution or reproduction in other forums is permitted, provided the original author(s) and the copyright owner(s) are credited and that the original publication in this journal is cited, in accordance with accepted academic practice. No use, distribution or reproduction is permitted which does not comply with these terms.
*Correspondence: Laurie Casalot, bGF1cmllLmNhc2Fsb3RAbWlvLm9zdXB5dGhlYXMuZnI=; bGF1cmllLmNhc2Fsb3RAdW5pdi1hbXUuZnI=
Disclaimer: All claims expressed in this article are solely those of the authors and do not necessarily represent those of their affiliated organizations, or those of the publisher, the editors and the reviewers. Any product that may be evaluated in this article or claim that may be made by its manufacturer is not guaranteed or endorsed by the publisher.
Research integrity at Frontiers
Learn more about the work of our research integrity team to safeguard the quality of each article we publish.