- Fujian Provincial Key Laboratory of Soil Environmental Health and Regulation, College of Resources and Environment, Fujian Agriculture and Forestry University, Fuzhou, China
Anaerobes respire extracellular electron acceptors by extracellular electron transfer (EET). It is widely recognized that flavins can act as electron shuttles to facilitate this process. Flavin synthesis genes are widely distributed in Geobacter species. However, the functions of flavins in the EET of Geobacter species are unclear. Here, we demonstrate that G. uraniireducens can secrete abundant riboflavin (up to 270 nM) to facilitate EET. When an electrode was used as the electron acceptor, the quick recovery of anodizing current after anolyte replacement and the electrochemical behavior of the G. uraniireducens biofilm characterized by differential pulse voltammetry suggest that the self-secreted riboflavin promoted EET by serving as bound redox cofactors for cytochromes. On the contrary, when Fe(III) oxide was the electron acceptor, free riboflavin acted as electron shuttle to mediate the reduction of Fe(III) oxide. The results demonstrate the flexibility of flavins in EET, suggesting that the properties of electron acceptors can affect the binding mode of extracellular flavins, and broaden the knowledge of the EET of Geobacter species.
Introduction
Anaerobic organisms can respire extracellular electron acceptors to conserve energy for growth by extracellular electron transfer (EET). This process affects the cycling of minerals, promotes the degradation of organic and radioactive wastes, helps current generation in the microbial fuel cell and contributes to the electron exchange between some syntrophic species (Nealson and Saffarini, 1994; Lovley, 2006, 2011; Nevin et al., 2010; Liu et al., 2018). Three EET methods have been reported (Newman and Kolter, 2000; Shi et al., 2016; Kumar et al., 2017): (i) cytochromes mediate direct electron transport after intimate contact between microbes and terminal electron acceptors; (ii) conductive pili mediate long-range electron transfer for the reduction of remote electron acceptors; and (iii) electron shuttles mediate extracellular electron transport. Notably, the specific methods used are species dependent.
Species from the genus of Shewanella and Geobacter are widely used for research on EET. Shewanella species can excrete abundant free flavins which are supposed to act as electron shuttles for the reduction of extracellular electron acceptors (Marsili et al., 2008). Recent studies further indicated that physiological concentrations of flavins mainly bind to outer membrane cytochromes with a high dissociation constant and can be cofactors mediating extracellular electron transport in Shewanella species (Okamoto et al., 2013, 2014a). In contrast, Geobacter species have abundant c-type cytochromes and are generally thought to transfer electrons to terminal electron acceptors by direct contact (Bond and Lovley, 2003; Reguera et al., 2005). Surprisingly, flavin synthesis and excretion genes are widely distributed in Geobacter species, which indicates that flavins may widely participate in the EET of Geobacter species. Recent studies also indicated that Geobacter sulfurreducens can uptake self-secreted flavins as bound cofactors for EET (Okamoto et al., 2014c; Michelson et al., 2017). However, the detailed functions of flavins in the EET of Geobacter species have not been documented and the possibility that free flavin molecules act as electron shuttles in the EET of Geobacter species has not been reported.
Geobacter uraniireducens is deficient in current generation but is a good iron oxide reducer, even when it is not in direct contact with iron oxide (Rotaru et al., 2015). In most Geobacter species, conductive pili are necessary for the efficient reduction of extracellular electron acceptors (Morita et al., 2011; Malvankar and Lovley, 2014; Rotaru et al., 2014). However, the pili of G. uraniireducens are nonconductive (Tan et al., 2016). Considering there are integral flavin synthesis genes coordinating 2539702 to 2543699 in the genome of G. uraniireducens (accession no. NC_009483.1), we suppose that self-secreted flavins participate in the EET of G. uraniireducens. In this study, abundant self-secreted flavins from G. uraniireducens were identified using fluorescence spectrophotometry and liquid chromatography-electrospray ionization ion-trap mass spectrometry. The possibility of flavins acting as free electron shuttles in Fe(III) oxide reduction was verified. Differential pulse voltammetry was used to reveal the redox properties of self-secreted flavins and to identify the role of flavins in anode respiration for current generation.
Materials and Methods
Bacterial Strains and Growth Conditions
G. uraniireducens strain Rf4 and G. sulfurreducens strain PCA were inoculated from frozen stocks in our lab and were cultured at 30°C under strict anaerobic conditions in NBAF medium as previously reported (Coppi et al., 2001).
Fluorescence Spectrophotometry
To identify and quantify the secreted flavins, cell cultures from G. uraniireducens Rf4 and G. sulfurreducens PCA were collected and filtered through 0.22-μm membrane filters. Fluorescence spectra of the filtrates were recorded using the Agilent Cary Eclipse Fluorescence Spectrometer (Agilent Technologies, California, United States) as previously described (Okamoto et al., 2014c). Emission spectra were measured at an excitation of 440 nm, and excitation spectra were monitored at 520 nm. A standard curve from a gradient concentration of riboflavin (0, 100, 300, and 500 nM) was calculated to quantify the amount of free flavins in the culture medium.
Liquid Chromatography-Electrospray Iron-Trap Mass Spectrometry
Liquid chromatography (Agilent 1100, United States)-electrospray ion-trap mass spectrometry (Agilent MSD-Trap-XCT, United States) (LC/ESI-MS) was performed to detect the secretion of flavins by G. uraniireducens. A total of 10 μL of filtered culture was subjected to LC/ESI-MS analysis at a flow rate of 1 mL min−1. Flavins were separated by a reversed-phase C18 column (Eclipse Plus C18, 4.6 mm × 250 mm, 5 μm) and detected with the UV-Vis detector setting at 468/525 nm (excitation/emission). An acetic acid/methanol gradient was used to elute flavins as previously reported(You et al., 2018), with the mobile phase comprising 650 mM glacial acetic acid (phase A) and methanol (phase B). Riboflavin and flavin mononucleotides were purchased from Sigma and served as reference standards.
Fe (III) Oxide Reduction
Ferrihydrite beads (3∼5 mm diameter) with a molecular mass cutoff of 12 kDa were prepared as described previously (Nevin and Lovley, 2000). There was ca. 10% of ferrihydrite, which can be reduced by microorganisms directly, exposed on their surfaces. To prepare the Fe(III) oxide growth medium, free ferrihydrite or ferrihydrite beads were added with a final concentration of Fe(III) of approximately 50 mM. Acetate (10 mM) was supplied as an electron donor. The same amount of mid-exponential phase cells growing in NBAF medium were collected and washed with PBS and then were used as inocula. The production of Fe(II) was measured at different time points with the Ferrozine assay as previous reported (Lovley and Phillips, 1988).
Flavin Reduction Assay
Flavins are fluorescent in an oxidized state (Coursolle et al., 2010). To test the reduction of riboflavin in vivo by G. uraniireducens, 3 mL culture was collected, washed once with fresh medium, and then injected into an anaerobic cuvette containing 300 nM of riboflavin. The reduction of riboflavin was monitored by a fluorescence spectrophotometer at different time points as described above. Subsequently, the cuvette was exposed to the atmosphere, vortexed for approximately 10 s to reoxidize the riboflavin, and then resealed and tested for fluorescence again.
Reduction of Ferrihydrite by Riboflavin
Riboflavin was reduced as previously described using hydrogen as the reductant in the presence of palladium (Von Canstein et al., 2008). 0.1 mM of reduced riboflavin was added into the medium containing 5 mM ferrihydrite and incubated for 24 h in the dark at room temperature. The reduction of Fe(III) was determined after testing the production of Fe(II) by the ferrozine assay (Lovley and Phillips, 1988).
SDS-PAGE and Heme-Staining
Extracellular proteins in anode biofilm matrix were collected as previously described (Sun et al., 2012). Extracellular proteins were separated from ferrihydrite culture medium as described previously (Smith et al., 2014). Briefly, 200 mL of ferrihydrite culture were shacked heavily and then centrifuged at 10000 × g for 20 min. Supernatants were further pass through a 0.22-mm filter to remove residue cells and ferrihydrite. Extracellular proteins were concentrated using Amicon Centrifugal filter (3 kDa cutoff, Merk millipore) and quantify with the Micro BCA protein assay kit (Thermo Fisher Scientific). Proteins were mixed with 5 × non-reducing loading dye and then 5 μg proteins were loaded on a 12.5% Tris-tricine polyacrylamide gel. The cytochromes were heme stained in the gel with N,N,N′,N′-tetramethylbenzidine (Liu et al., 2014).
Fuel Cell Construction and Electrochemical Measurements
A single-chamber, three-electrode system with a liquid volume of 20 mL and a headspace volume of 10 mL was constructed for the electrochemical measurement as previously described (Okamoto et al., 2013). An indium tin-oxide glass (4 cm × 5 cm) was used as a working electrode and placed at the bottom of the reactor. Hg| Hg2Cl2 (sat. KCl) and a graphite plate (1.5 cm × 1 cm × 0.5 cm) were used as the reference and counter electrode, respectively. The electrolyte was freshwater medium (Bond and Lovley, 2003) supplied with 10 mM acetate. A total of 2 mL of NBAF cultures growing to the mid-exponential phase were collected and washed before cell inoculation.
Current production was monitored with a 1000C electrochemical workstation (CH instruments, United States) with a work electrode polarized at +0.3 V (vs. SCE). Differential pulse voltammetry (DPV) was conducted from –800 mv to 200 mV (vs. SCE). The specific parameters were: Ei = −0.8 V, Ef = 0.2 V, pulse height 50 mV, pulse width 250 ms, step height 2 mV, step time 500 ms, scan rate 1 mV s−1.
Results and Discussion
Geobacter uraniireducens Secretes Abundant Riboflavin
Fluorescence spectrophotometry was used to identify the redox molecules in the G. uraniireducens culture. The fluorescence spectra (Figures 1A,B) of the G. uraniireducens culture have a characteristic emission peak at 520 nm under excitation at both 370 nm and 440 nm, identical to the fluorescence spectra of flavins (Supplementary Figure S1). The secretion of flavins was further confirmed by LC/ESI-MS, which showed the characteristics of riboflavin (Figure 1C). There are three forms of flavins inside cells, namely, flavin adenine dinucleotide (FAD), flavin mononucleotide (FMN) and riboflavin (RF). FMN and RF can be involved in EET, but FAD is only released when cells are dead (Von Canstein et al., 2008). The data indicated that RF was the dominant flavin excreted by G. uraniireducens (Figure 1C and Supplementary Figure S2). Notably, G. uraniireducens excreted a much higher concentration of free riboflavin than G. sulfurreducens (with maximums of 270 nM and 70 nM for G. uraniireducens and G. sulfurreducens at the stationary phase, respectively). In addition, the riboflavin concentrations of both cells were increased with the growth of cells (Figure 1D and Supplementary Figure S3).
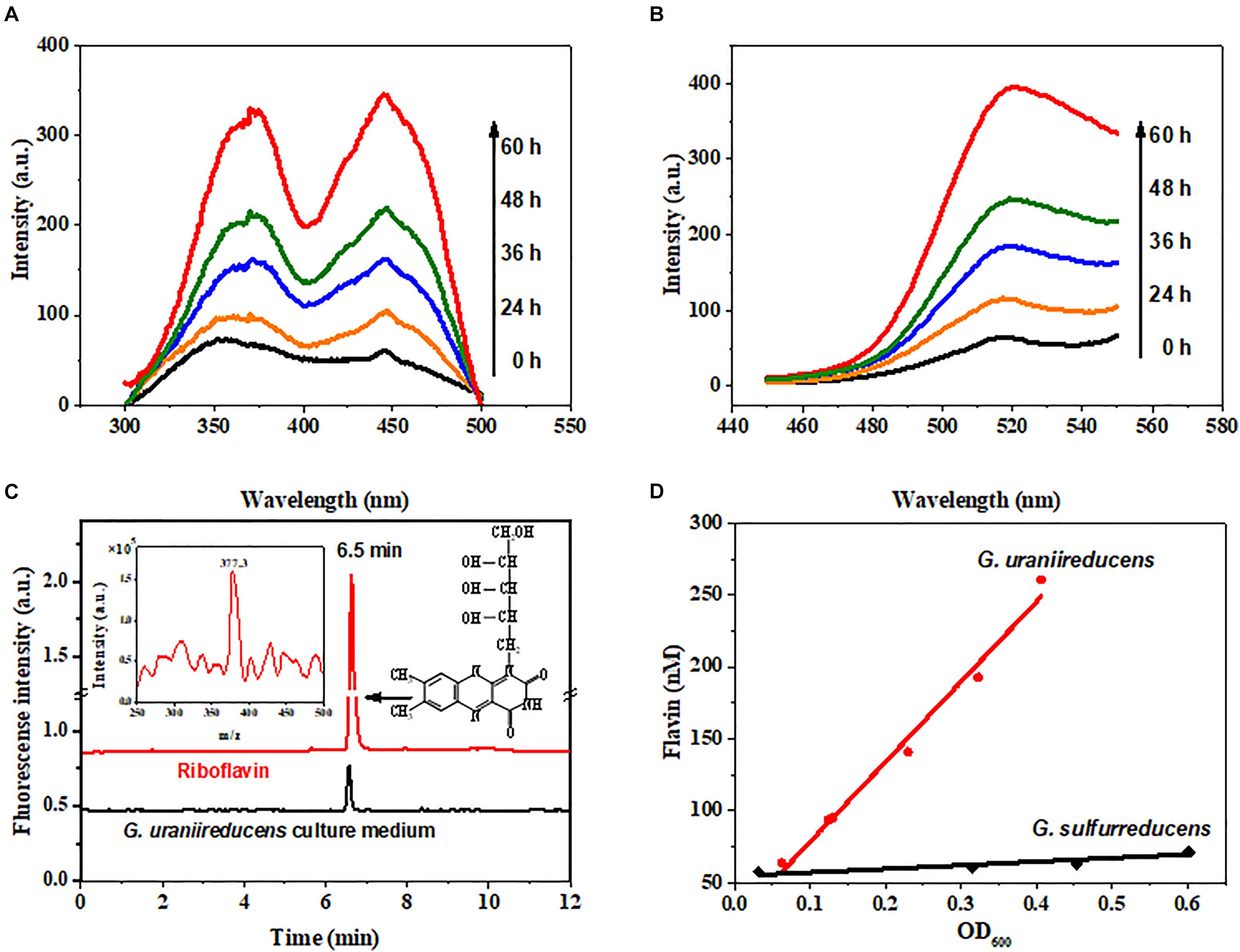
FIGURE 1. Identification of flavins secreted by G. uraniireducens. (A) Excitation and (B) emission spectra (arbitrary units, a.u.) of the cell-free culture medium collected from the G. uraniireducens culture after 0, 24, 36, 48, and 60 h of inoculation. The excitation wavelength was 440 nm and the emission wavelength was 520 nm. (C) LC/ESI-MS identification of riboflavin from the cell-free culture medium of G. uraniireducens collected at the stationary phase. The fluorescence detector was set at 468/525 nm (excitation/emission). The LC peak at 6.5 min corresponds to the compound of riboflavin with a m/z of 377.3, and 0.1 μM riboflavin was used as the reference standard. (D) Quantification of accumulated flavins as a function of the cell densities of G. uraniireducens and G. sulfurreducens.
Free Riboflavin Act as Electron Shuttles to Facilitate Fe(III) Oxide Reduction
Geobacter uraniireducens can reduce Fe(III) oxide much faster than G. sulfurreducens (Figure 2A). Previous studies have implied that conductive pili are necessary for the efficient EET of Geobacter species to reduce Fe(III) oxide (Reguera et al., 2005; Liu et al., 2014). However, the pili of G. uraniireducens are nonconductive. Additional methods of electron transfer are therefore necessary to contribute to the high Fe(III) oxide reduction of G. uraniireducens.
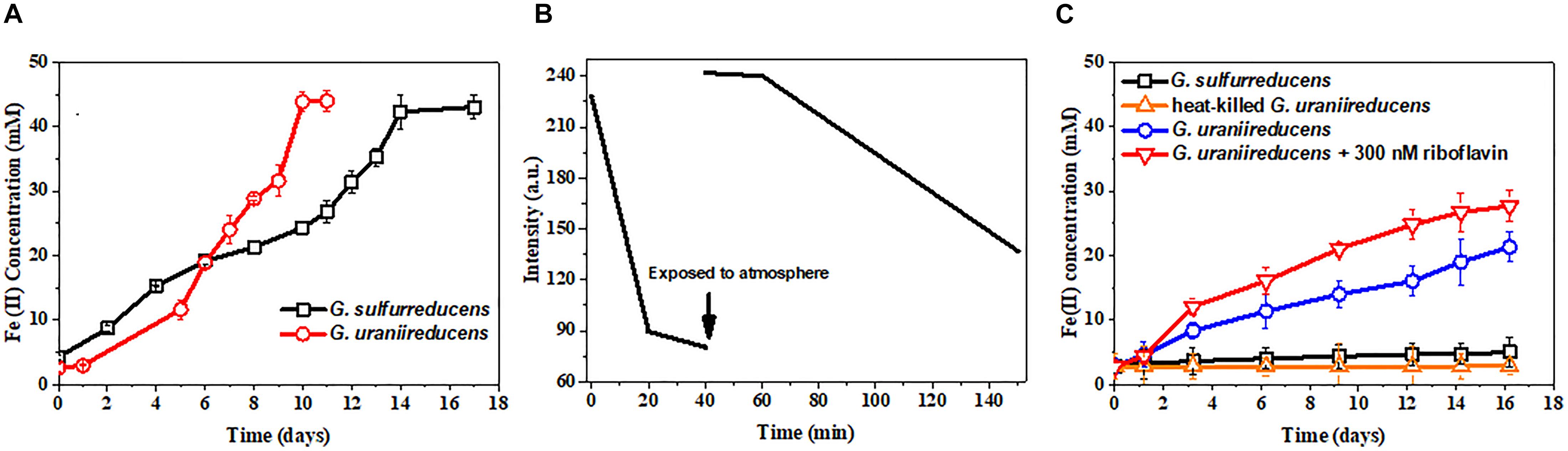
FIGURE 2. Free riboflavin facilitates the extracellular electron transfer of G. uraniireducens. (A) Fe(III) oxides reduction by G. uraniireducens and G. sulfurreducens. (B) Riboflavin reduction by G. uraniireducens. The arrow indicates the time point at which the culture was exposed to the atmosphere and vortex. The redox state of riboflavin was monitored by fluorescence emission at 520 nm with excitation at 440 nm. (C) Ferrihydrite bead reduction. The same number of cells were used as inocula. Data are averages for triplicate cultures.
Fluorescence spectrophotometry was also performed on the Fe(III) oxide-grown culture of G. uraniireducens. Not surprisingly, riboflavin could be detected in the Fe(III) oxide culture medium of G. uraniireducens (Supplementary Figure S4), which is in contrast to the riboflavin-free Fe(III) oxide culture of G. sulfurreducens (data not shown) and indicates an EET involving free riboflavin. The redox state of riboflavin can be detected by fluorescence spectrometry as only oxidized riboflavin fluoresces (Ghisla et al., 1974). The possibility that oxidized riboflavin can accept electrons from G. uraniireducens was examined with fluorescence spectrophotometry. Oxidized riboflavin was incubated with G. uraniireducens. The fluorescence intensity of the riboflavin decreased over time, indicating the continuous reduction of riboflavin by G. uraniireducens and the bio-reduced riboflavin could be re-oxidized after exposure to atmospheric oxygen (Figure 2B). Furthermore, 0.18 mM of Fe(II) was generated when 0.1 mM of reduced riboflavin was added into 5 mM ferrihydrite medium indicating that riboflavin can carry electrons for the reduction of Fe(III) oxide. To determine whether the free riboflavin excreted by G. uraniireducens accelerated insoluble Fe(III) reduction, ferrihydrite trapped in hydrogel beads was used as an electron acceptor. The insulated hydrogel shell prevented the direct contact between G. uraniireducens cells and ferrihydrite. As shown in Figure 2C, G. uraniireducens readily reduced the ferrihydrite beads, generating Fe(II) with a concentration of approximately 20 mM after 16 days. In contrast, G. sulfurreducens reduced less than 5 mM of Fe(III) via the direct reduction of the Fe(III) oxide exposed on the surface of the ferrihydrite beads. The addition of riboflavin further accelerated the reduction of Fe(III) oxide by G. uraniireducens, increasing this value from 0.78 mM⋅day−1 to 1.54 mM⋅day−1.
Riboflavin Bound as Cofactors to Cytochromes Promote Current Production
The anode reduction of G. uraniireducens was tested in a three-electrode system (Figure 3A). The current was generated continuously. When the plateau arrived, the electrolyte was replaced with fresh medium. The current was immediately recovered to 80% of the original value (Figure 3A). When mediators are involved in EET for current generation, the replacement of the electrolyte with fresh medium disrupts the current production (Marsili et al., 2008). The immediate current recovery after the medium exchange indicated that EET mediated by electron shuttles did not dominate the EET of G. uraniireducens when an anode was the electron acceptor. Notably, most of the G. uraniireducens were attached on the anode and formed a compact electroactive biofilm (Figure 3B), indicating the possibility of anode reduction with direct contact.
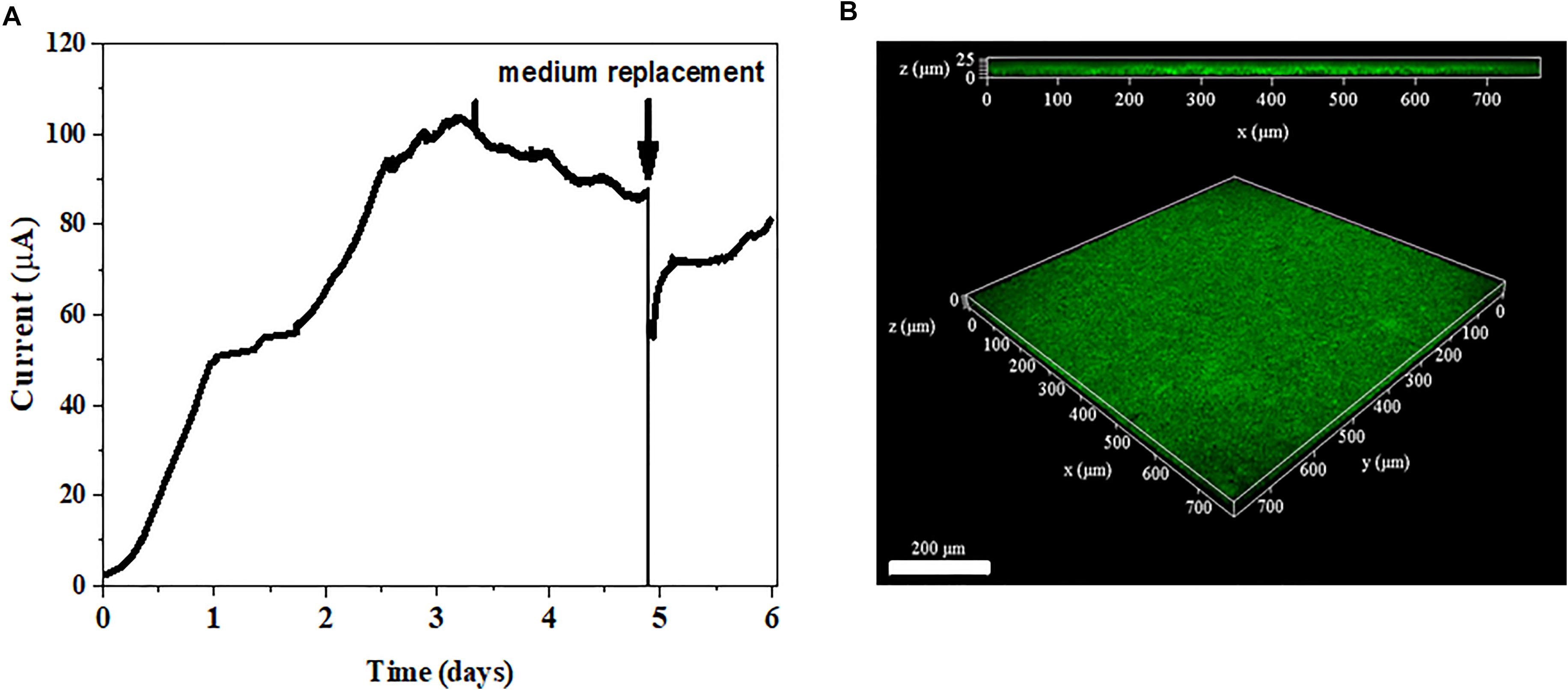
FIGURE 3. Current generation and anode biofilm formation. (A) Current production of G. uraniireducens. The arrow indicates the replacement of the electrolyte. (B) Confocal scanning fluorescence microscope image of G. uraniireducens biofilm growing on the anode. Cells were stained with LIVE/DEAD stain. The scale bar represents 200 μm.
The electrochemical behavior of the G. uraniireducens biofilm was further characterized. Differential pulse voltammetry (DPV) was conducted at different time points during the process of biofilm formation. Three peaks were detected. Notably, only the redox peak current at −176 mV increased with time (Figure 4A) and was positively correlated with the current produced (Figure 4B). In particular, the addition of riboflavin increased the redox peak current at the same potential (Figure 4C). These data indicate that the redox peak at −176 mV can be assigned to the redox cycling of riboflavin and that the excretion of riboflavin can promote the delivery of electrons produced by G. uraniireducens to the anode. However, the redox peak potential of free riboflavin is approximately −235 mV (Figure 4D), which is negative than that of riboflavin in G. uraniireducens biofilm. This discrepancy has also been reported in G. sulfurreducens and the reason can be contributed to the binding of riboflavin to cytochromes positively shifts the redox peak potential (Tan et al., 2016). Therefore, riboflavin acted as cofactors of cytochromes to promote current production in G. uraniireducens when an anode was the electron acceptor.
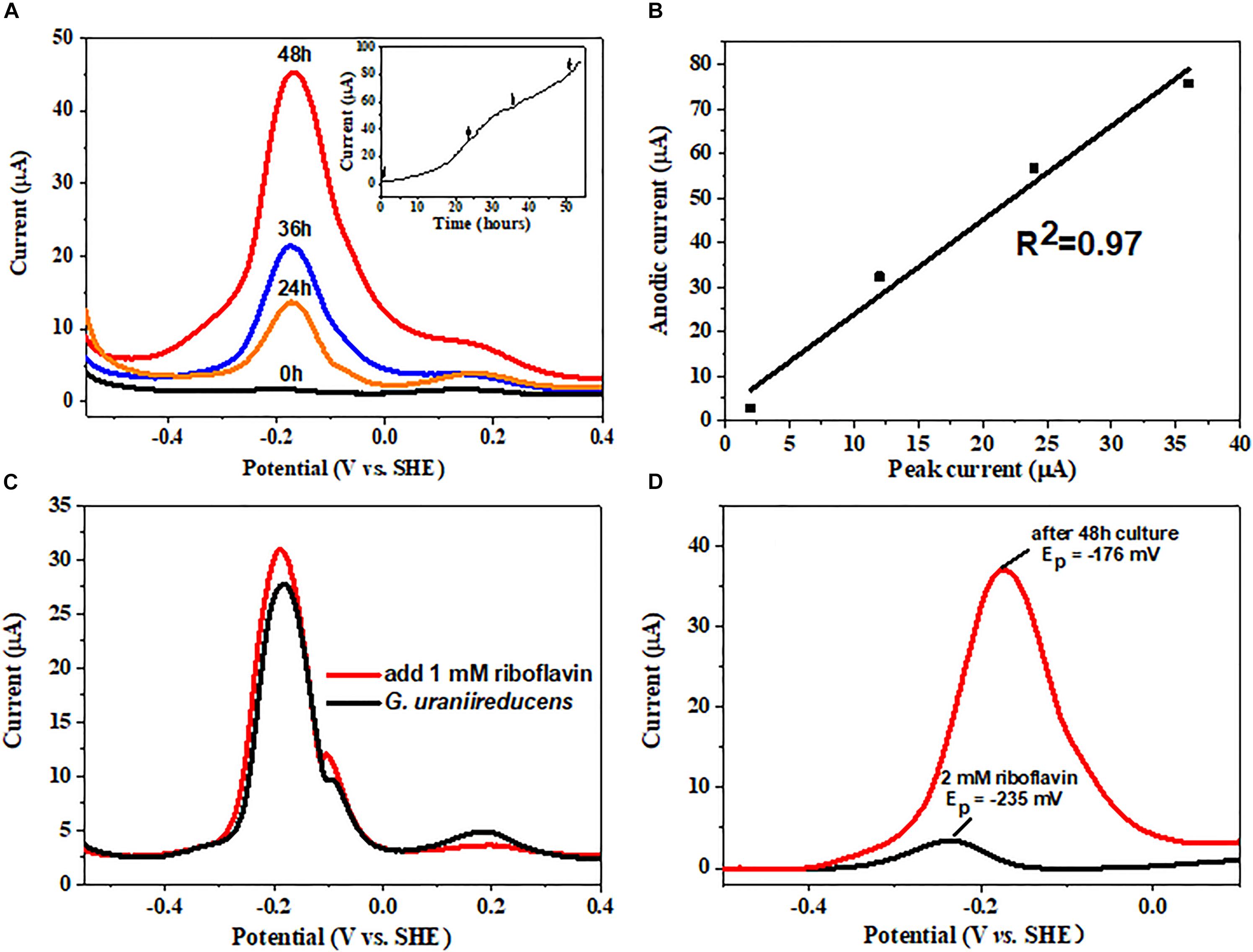
FIGURE 4. Differential pulse voltammetry (DPV) analysis of the G. uraniireducens anode biofilm. (A) Differential pulse voltammogram of the G. uraniireducens anode 0, 24, 36, and 48 h after inoculation. The inserted figure shows the anodic current profile, with arrows representing the time for DPV analysis. (B) Plot of redox peak current at a potential of –176 mV against the corresponding anodic current. (C) DPV for biofilms of G. uraniireducens before and after the addition of 1 μM riboflavin. (D) Base-line subtracted differential pulse voltammogram for 2 μM riboflavin solution and for G. uraniireducens anode biofilm after 48 h growth.
Different Outer Membrane Cytochrome Profiles
Riboflavin facilitates the electron transfer between extracellular c-type cytochromes and electron acceptors. Previous studies have indicated that the binding affinities of flavins to different cytochromes may differ (Okamoto et al., 2014b). When flavins have high binding affinities to cytochromes, they tend to form cofactors; otherwise, they tend to be free. The outer membrane proteins were extracted from G. uraniireducens anode biofilm and ferrihydrite culture medium, and were analyzed to identify c-type cytochrome profiles. Both the heme-stained SDS-PAGE gel (Figure 5) and mass spectrometry analysis (Supplementary Table S1) indicated a different c-type cytochrome distribution between G. uraniireducens growing on anode and in ferrihydrite medium. Especially, the c-type cytochromes in G. uraniireducens anode biofilm showed much more variety (Supplementary Table S1).
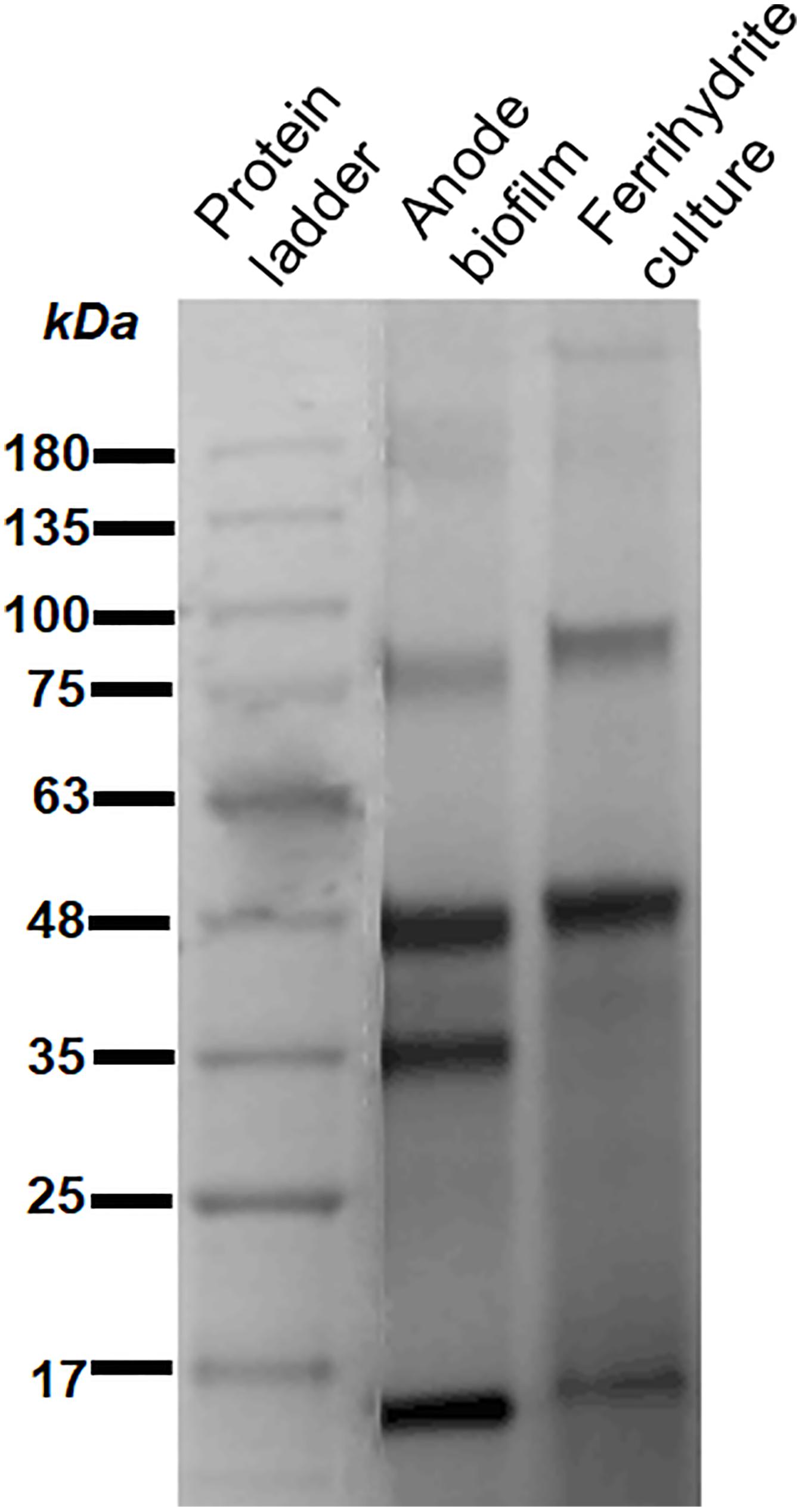
FIGURE 5. Heme-stained SDS-PAGE gel of outer membrane c-type cytochromes extracted from the anode biofilm and ferrihydrite culture medium of G. uraniireducens. The protein marker is Biostep Prestained Protein Marker (Tanon, Shanghai, China).
Implication
The results demonstrate that G. uraniireducens can excrete abundant riboflavin, which mediates EET to reduce extracellular electron acceptors, suggesting that the existing mode of extracellular riboflavin is dependent on the status of terminal electron acceptors. When Fe(III) oxides acted as the electron acceptor, G. uraniireducens used free riboflavin to facilitate EET. In contrast, when the electrode was an electron acceptor, the excreted riboflavin bound to cytochromes acting as cofactors to accelerate EET.
Our studies also indicate that G. uraniireducens expresses different outer membrane c-type cytochromes when reduces different electron acceptors, and these cytochromes probably have different binding affinity toward riboflavin that cytochromes in anode biofilm show higher binding affinity toward riboflavin than the cytochromes on ferrihydrite reduction cells. However, the different existing modes of extracellular riboflavin can help G. uraniireducens to survive in different environments. The free flavins mediate two-electron transfer between cytochromes and electron acceptors, while the bound flavin cofactor facilitates a one-electron redox reaction, which is much more efficient (Okamoto et al., 2013). Fe(III) oxides are consumable, and not all are accessible to Geobacter species. Expression of conductive pili can alleviate the stress. However, the pili of G. uraniireducens are nonconductive. The excretion of free riboflavin in G. uraniireducens facilitates the transfer of electrons to remote or nonaccessible Fe(III) oxides. In contrast, the electrode is a permanent electron acceptor, and the EET in the electroactive biofilm is facilitated by cytochromes. The G. uraniireducens biofilm is compact. Flavins binding on cytochromes can greatly accelerate the electron exchange among cytochromes and at the interface between the cytochromes and electrode.
In summary, this study reveals that the existing modes of extracellular flavins in EET are flexible. The direct extracellular electron transfer in Geobacter species is well-known. However, the flavin-mediated EET in Geobacter species is not generally recognized. This study not only broadens the knowledge of EET in Geobacter species but also provides a new perspective to understand EET in natural systems.
Author Contributions
XL and SZ designed the experiments. LH ran all the tests with the help of JT. MC contributed to the scientific discussion. LH and XL wrote the original manuscript with revisions from all authors.
Funding
This research was supported by the National Natural Science Foundation of China, grant nos. 31600089, 41671264 and 91751109, and the China Postdoctoral Science Foundation, grant no. 2017M622040.
Conflict of Interest Statement
The authors declare that the research was conducted in the absence of any commercial or financial relationships that could be construed as a potential conflict of interest.
Acknowledgments
We would like to thank Prof. Derek Lovley of the University of Massachusetts, Amherst, for providing the Geobacter uraniireducens strain Rf4.
Supplementary Material
The Supplementary Material for this article can be found online at: https://www.frontiersin.org/articles/10.3389/fmicb.2018.02886/full#supplementary-material
References
Bond, D. R., and Lovley, D. R. (2003). Electricity production by Geobacter sulfurreducens attached to electrodes. Appl. Environ. Microbiol. 69, 1548–1555. doi: 10.1128/AEM.69.3.1548-1555.2003
Coppi, M. V., Leang, C., Sandler, S. J., and Lovley, D. R. (2001). Development of a genetic system for Geobacter sulfurreducens. Appl. Environ. Microbiol. 67, 3180–3187. doi: 10.1128/AEM.67.7.3180-3187.2001
Coursolle, D., Baron, D. B., Bond, D. R., and Gralnick, J. A. (2010). The mtr respiratory pathway is essential for reducing flavins and electrodes in Shewanella oneidensis. J. Bacteriol. 192, 467–474. doi: 10.1128/JB.00925-09
Ghisla, S., Massey, V., Lhoste, J. M., and Mayhew, S. G. (1974). Fluorescence and optical characteristics of reduced flavines and flavoproteins. Biochemistry 13, 589–597. doi: 10.1021/bi00700a029
Kumar, A., Hsu, L. H. H., Kavanagh, P., Barrière, F., Lens, P. N., Lapinsonnière, L., et al. (2017). The ins and outs of microorganism-electrode electron transfer reactions. Nat. Rev. Chem. 1:24. doi: 10.1038/s41570-017-0024
Liu, X., Tremblay, P. L., Malvankar, N. S., Nevin, K. P., Lovley, D. R., and Vargas, M. (2014). A Geobacter sulfurreducens strain expressing Pseudomonas aeruginosa type IV pili localizes omcs on pili but is deficient in Fe(III) oxide reduction and current production. Appl. Environ. Microbiol. 80, 1219–1224. doi: 10.1128/AEM.02938-13
Liu, X., Zhuo, S., Rensing, C., and Zhou, S. (2018). Syntrophic growth with direct interspecies electron transfer between pili-free Geobacter species. ISME J. 12, 2142–2151. doi: 10.1038/s41396-018-0193-y
Lovley, D. R. (2006). Bug juice: harvesting electricity with microorganisms. Nat. Rev. Microbiol. 4:497. doi: 10.1038/nrmicro1442
Lovley, D. R. (2011). Live wires: direct extracellular electron exchange for bioenergy and the bioremediation of energy-related contamination. Energ. Environ. Sci. 4, 4896–4906. doi: 10.1039/c1ee02229f
Lovley, D. R., and Phillips, E. J. (1988). Novel mode of microbial energy metabolism: organic carbon oxidation coupled to dissimilatory reduction of iron or manganese. Appl. Environ. Microbiol. 54, 1472–1480.
Malvankar, N. S., and Lovley, D. R. (2014). Microbial nanowires for bioenergy applications. Curr. Opin. Biotech. 27, 88–95. doi: 10.1016/j.copbio.2013.12.003
Marsili, E., Baron, D. B., Shikhare, I. D., Coursolle, D., Gralnick, J. A., and Bond, D. R. (2008). Shewanella secretes flavins that mediate extracellular electron transfer. Proc. Natl. Acad. Sci. U.S.A. 105, 3968–3973. doi: 10.1073/pnas.0710525105
Michelson, K., Sanford, R. A., Valocchi, A. J., and Werth, C. J. (2017). Nanowires of Geobacter sulfurreducens require redox cofactors to reduce metals in pore spaces too small for cell passage. Environ. Sci. Technol. 51, 11660–11668. doi: 10.1021/acs.est.7b02531
Morita, M., Malvankar, N. S., Franks, A. E., Summers, Z. M., Giloteaux, L., Rotaru, A. E., et al. (2011). Potential for direct interspecies electron transfer in methanogenic wastewater digester aggregates. mBio 2, e00159–e01511. doi: 10.1128/mBio.00159-11
Nealson, K. H., and Saffarini, D. (1994). Iron and manganese in anaerobic respiration: environmental significance, physiology, and regulation. Annu. Rev. Microbiol. 48, 311–343. doi: 10.1146/annurev.mi.48.100194.001523
Nevin, K. P., and Lovley, D. R. (2000). Lack of production of electron-shuttling compounds or solubilization of Fe(III) during reduction of insoluble Fe(III) oxide by Geobacter metallireducens. Appl. Environ. Microbiol. 66, 2248–2251. doi: 10.1128/AEM.66.5.2248-2251.2000
Nevin, K. P., Woodard, T. L., Franks, A. E., Summers, Z. M., and Lovley, D. R. (2010). Microbial electrosynthesis: feeding microbes electricity to convert carbon dioxide and water to multicarbon extracellular organic compounds. mBio 1:e00103-10. doi: 10.1128/mBio.00103-10
Newman, D. K., and Kolter, R. (2000). A role for excreted quinones in extracellular electron transfer. Nature 405:94. doi: 10.1038/35011098
Okamoto, A., Hashimoto, K., Nealson, K. H., and Nakamura, R. (2013). Rate enhancement of bacterial extracellular electron transport involves bound flavin semiquinones. Proc. Natl. Acad. Sci. U.S.A. 110, 7856–7861. doi: 10.1073/pnas.1220823110
Okamoto, A., Kalathil, S., Deng, X., Hashimoto, K., Nakamura, R., and Nealson, K. H. (2014a). Cell-secreted flavins bound to membrane cytochromes dictate electron transfer reactions to surfaces with diverse charge and pH. Sci. Rep. 4:5628. doi: 10.1038/srep05628
Okamoto, A., Nakamura, R., Nealson, K. H., and Hashimoto, K. (2014b). Bound flavin model suggests similar electron-transfer mechanisms in Shewanella and Geobacter. ChemElectroChem 1, 1808–1812. doi: 10.1002/celc.201402151
Okamoto, A., Saito, K., Inoue, K., Nealson, K. H., Hashimoto, K., and Nakamura, R. (2014c). Uptake of self-secreted flavins as bound cofactors for extracellular electron transfer in Geobacter species. Energ. Environ. Sci. 7, 1357–1361. doi: 10.1039/C3EE43674H
Reguera, G., Mccarthy, K. D., Mehta, T., Nicoll, J. S., Tuominen, M. T., and Lovley, D. R. (2005). Extracellular electron transfer via microbial nanowires. Nature 435:1098. doi: 10.1038/nature03661
Rotaru, A. E., Shrestha, P. M., Liu, F., Shrestha, M., Shrestha, D., Embree, M., et al. (2014). A new model for electron flow during anaerobic digestion: direct interspecies electron transfer to methanosaeta for the reduction of carbon dioxide to methane. Energ. Environ. Sci. 7, 408–415. doi: 10.1039/C3EE42189A
Rotaru, A. E., Woodard, T. L., Nevin, K. P., and Lovley, D. R. (2015). Link between capacity for current production and syntrophic growth in Geobacter species. Front. Microbiol. 6:744. doi: 10.3389/fmicb.2015.00744
Shi, L., Dong, H., Reguera, G., Beyenal, H., Lu, A., Liu, J., et al. (2016). Extracellular electron transfer mechanisms between microorganisms and minerals. Appl. Environ. Microbiol. 14:651. doi: 10.1038/nrmicro.2016.93
Smith, J. A., Tremblay, P. L., Shrestha, P. M., Snoeyenbos-West, O. L., Franks, A. E., Nevin, K. P., et al. (2014). Going wireless: Fe(III) oxide reduction without pili by Geobacter sulfurreducens strain JS-1. Appl. Environ. Microbiol. 80, 4331–4340. doi: 10.1128/AEM.01122-14
Sun, M., Li, W. W., Yu, H. Q., and Harada, H. (2012). A novel integrated approach to quantitatively evaluate the efficiency of extracellular polymeric substances (EPS) extraction process. Appl. Microbiol. Biotechnol. 96, 1577–1585. doi: 10.1007/s00253-012-4478-1
Tan, Y., Adhikari, R. Y., Malvankar, N. S., Ward, J. E., Nevin, K. P., Woodard, T. L., et al. (2016). The low conductivity of Geobacter uraniireducens pili suggests a diversity of extracellular electron transfer mechanisms in the genus Geobacter. Front. Microbiol. 7:980. doi: 10.3389/fmicb.2016.00980
Von Canstein, H., Ogawa, J., Shimizu, S., and Lloyd, J. R. (2008). Secretion of flavins by Shewanella species and their role in extracellular electron transfer. Appl. Environ. Microbiol. 74, 615–623. doi: 10.1128/AEM.01387-07
Keywords: Geobacter uraniiireducens, flavins, electron shuttles, extracellular electron transfer, cytochromes bound cofactor
Citation: Huang L, Tang J, Chen M, Liu X and Zhou S (2018) Two Modes of Riboflavin-Mediated Extracellular Electron Transfer in Geobacter uraniireducens. Front. Microbiol. 9:2886. doi: 10.3389/fmicb.2018.02886
Received: 02 July 2018; Accepted: 12 November 2018;
Published: 27 November 2018.
Edited by:
Fanghua Liu, Yantai Institute of Coastal Zone Research (CAS), ChinaReviewed by:
Biswarup Mukhopadhyay, Virginia Tech, United StatesWolfgang Buckel, University of Marburg, Germany
Copyright © 2018 Huang, Tang, Chen, Liu and Zhou. This is an open-access article distributed under the terms of the Creative Commons Attribution License (CC BY). The use, distribution or reproduction in other forums is permitted, provided the original author(s) and the copyright owner(s) are credited and that the original publication in this journal is cited, in accordance with accepted academic practice. No use, distribution or reproduction is permitted which does not comply with these terms.
*Correspondence: Xing Liu, eGluZ2xpdUBmYWZ1LmVkdS5jbg==