- 1Key Laboratory of Eco-Environments in Three Gorges Reservoir Region (Ministry of Education), School of Life Sciences, Southwest University, Chongqing, China
- 2Solar Energy Research Institute, School of Energy and Environment Science, Yunnan Normal University, Kunming, China
Fungal endophytes live widely inside plant tissues and some have been revealed to provide benefits to their host and ecological environment. Considering the fact that endophytes are engaged in remarkably stable long-term interactions with the host for their whole life cycle, it’s conceivable that both partners have substantial influence on each other’s metabolic processes. Here, we investigated the fermented products of an endophytic fungus Umbelopsis dimorpha SWUKD3.1410 grown on host–plant Kadsura angustifolia and wheat bran, respectively, to assess the impact of SWUKD3.1410 on the secondary metabolites of K. angustifolia. Twenty compounds (1–20) were isolated and identified as 11 schitriterpenoids (1–9, 17–18), two lignans (10, 20), two sesquiterpenoids (11–12), one trinorsesquiterpenoid (13), one monoterpene (14), one sterol (19), and two simple aromatic compounds (15–16) by the extensive 1D-, 2D-NMR and HR-ESI-MS data analysis. Except for nigranoic acid (1), compounds 2–19 have been firstly found from K. angustifolia. Of them, metabolites 2, 11, and 14 were identified to be new. Obtained results indicated that U. dimorpha SWUKD3.1410 could not only produce the same/similar components as its host does, and modify the host–plant components, but also enhance the production of these highly oxygenated schitriterpenoids/schinortriterpenoids in plants. This study suggested an interesting prospective for setting up alternative processing techniques to improve the quality of crude drugs derived from K. angustifolia and increase their values.
Introduction
Endophytic fungi or endophytes are diverse polyphyletic groups of microorganisms that colonize intracellularly or intercellularly the living tissues of host plant without triggering any visible external sign of infection (Tan and Zou, 2001; Kaul et al., 2012). Some co-existing endophytic fungi are considered to be plant mutualists: they receive nutrition and protection from the host plant to proliferate while the host plant may benefit from enhanced competitive abilities and increased tolerances to pathogens, herbivores, and various abiotic stresses (Schulz and Boyle, 2005; Kusari et al., 2012). Undoubtedly, the key to understanding the endophytic functions is to elucidate the mechanisms involved in plant–endophyte interactions (Herre et al., 2007). Nevertheless, the mechanisms of fungus–plant interaction have not been fully discerned owing to the complexity and diversity of the partners related (Rodriguez et al., 2009; de Siqueira et al., 2017). It is essential to employ simplified systems in investigating interactions of fungi and plant hosts (Schulz and Boyle, 2005). The productions of secondary metabolites, an interesting aspect of the fungus–plant interactions, have been demonstrated to play a critical role in their interactions, especially for defense, signaling purposes, and regulation of the symbiosis (Schulz and Boyle, 2005). Some reports have demonstrated that endophytic fungi can significantly affect the formation of metabolic products in plants, such as inducing host metabolism and transforming host components, and vice versa (Qawasmeh et al., 2012; Tian et al., 2014; Ludwig-Müller, 2015). Considering the fact that endophytes are continuously interacting with their hosts, it’s logical to suggest that both partners have a vital impact on each other’s metabolic processes (Tian et al., 2014).
It has been proposed that endophytic fungi have developed versatile biosynthetic capabilities (including synthesis, transformation, and degradation of host plant metabolites) through the long period of coevolution and genetic recombination with their hosts (Borges et al., 2009). These special symbiotic relationships with the host plant forced the endophytic fungi to dispose several toxic compounds produced by their hosts as defense against other organisms. The existence of biodegradation and biotransformation processes of the toxic substances by the help of certain specific enzymes has enabled them to survive (Borges et al., 2009). Werner et al. (1997) revealed that several endophytes from the roots of Aphelandra plant could well-metabolize aphelandrine, a macrocylic polyamine alkaloid isolated in the roots of different species of the genus Aphelandra, which was the first report on the biotransformation of endophytic fungi. After that, similar findings that endophytic fungi were capable of transforming some natural products and metabolizing drugs have been reported continuously (Gniłka et al., 2015; Smith et al., 2015). Specifically, compared to traditional method, the microbial transformation of raw herb powder has been reported to produce a higher yield of active components (Liu et al., 2010; Zhu et al., 2010; Ding et al., 2014). It has also been found that endophytic fungi may be adept in modifying chemical structures with a high degree of stereo-specificity (Borges et al., 2009). Some reactions are very similar to mammalian phase I metabolism (Borges et al., 2009). Therefore, more recently, endophytes have gained attention as potential sources of novel biocatalysts in the chemical transformation of natural products and drugs (Pimentel et al., 2010).
The family Schisandraceae contains the two genera, Schisandra and Kadsura. There are about 50 species in total belonging to this family, which are mainly distributed in Southeast Asia and North America (Xiao et al., 2010b). Many species of this familya have been used for generations in China as folk medicine to treat premature ejaculation, hepatitis, chronic dysentery, cough, and insomnia (Xiao et al., 2010b; Liang et al., 2012). Since the first discovery of the 3,4-seco-cycloartene nigranoic acid from S. nigra in 1972 (Sun et al., 1996), Schisandraceae plants have been a hot topic within the medicinal chemistry and drug discovery communities because a plethora of schtriterpenoids/schnortriterpenoids with 47 skeletons has been reported over the years with far more than 1000 terpene-type compounds been estimated to be produced by the plants of Schisandraceae family (Xiao et al., 2008; Xia et al., 2014; Shi et al., 2015; Zhou et al., 2016). These fascinating molecules have been reported to possess various beneficial bioactivities such as antihepatitis, antitumor and anti-HIV, and have attracted wide attention of phytochemists and pharmacologists (Xiao et al., 2008). As expected, the chemical synthesis of these specialized metabolites is quite difficult and commercially unfeasible owing to the complicated stereo-chemical rings with multiple chiral centers. Structurally, they are generally supposed to derive from the common cycloartane skeleton by ring rearrangement, oxidative cleavage, loss of carbons, and other reactions (Xia et al., 2014; Shi et al., 2015). However, many attempts to obtain the highly oxygenated triterpenoids failed in previous microbial biotransformation made directly on the substrates of cycloartane-type triterpenes, such as nigranoic acid (Dong et al., 2007a,b,Sun et al., 2013) and cycloartenol (Kuban et al., 2010, 2013). Recently, we noticed a report that cultivation of endophytes without their host plant might result in the loss of the desired compound synthesis (Ludwig-Müller, 2015). Accordingly, we assumed that partial or complete biosynthesis pathways for highly oxygenated schitriterpenoids/schinortriterpenoids can be attributed to the host environment, which may trigger the expression of silent biosynthetic pathways, or result in a change in expression of the enzymes related to the cyclization and oxidation of squalene. Our previous report indicated that Kadsura angustifolia (Lem.) Smith could be a community model for studies of their metabolic relationships between the endophytic fungi and host plant due to its relative single chemical composition, in which the isolated yield of nigranoic acid in the root and stem of K. angustifolia collected at Xichou of Yunnan province can reach up to 3.8% of the dry weight of the separate origin (Sun et al., 2011; Huang et al., 2015). More than 426 isolates were identified by morphological and molecular analysis (Huang et al., 2015). Among them, Umbelopsis dimorpha SWUKD3.1410 appeared to be relatively specific to K. angustifolia and showed remarkable biotransformative activities (Huang et al., 2015). Thus, as reported by Tian et al. (2014), in order to evaluate the impact of endophytic fungal inoculants on the production of secondary metabolites of host–plant K. angustifolia, the strain SWUKD3.1410 was selected to grow on different substrates. Furthermore, the fermentative cultures of K. angustifolia and wheat bran were chemically investigated, which led to the isolation and structural elucidation of 20 compounds (1–20) (Figure 1), including 11 schitriterpenoids (1–9, 17–18). Among them, metabolites 2–20 have never been found before from K. angustifolia, and compounds 2, 11, and 14 were identified to be new. Taken together with the reported components of K. angustifolia (Chen et al., 1998; Gao et al., 2008; Sun et al., 2011) and wheat bran (Posner, 2009; Zhu et al., 2013, 2015; Prinsen et al., 2014; Geng et al., 2015), we found the U. dimorpha SWUKD3.1410 cannot only yield the same and similar compounds as those present in its host–plant K. angustifolia, but also can significantly modify the host plant components and enhance the production of those highly oxygenated schitriterpenoids/schinortriterpenoids. This work will provide new sight for researchers to make better use of the beneficial symbiosis and expand the way for obtaining bioactive natural products from certain medicinal plants.
Materials and Methods
General Experimental Procedures
NMR spectrums (1H-NMR, 13C-NMR, DEPT, HMQC, HMBC, 1H-1HCOSY, NOESY) were recorded on a Bruker Avance 600 spectrometer (Fällanden, Bruker BioSpin, Switzerland). The chemical shifts (δ) were reported in ppm, and the coupling constants in hertz (Hz). Standard pulse sequences were used for DEPT, NOESY, HMQC, and HMBC experiments. Ultraviolet (UV) spectra were measured with a Shimadzu double-beam 210A spectrophometer (Shimadzu). Optical rotations were measured on a Jasco DIP-370 polarimeter (JASCO Corporation, Japan). Infrared (IR) spectra were obtained in KBr pellets with a Bio-Rad FTS-135 spectrophotometer (Bio-Rad). MSs were taken on a VG Auto Spec-3000 mass spectrometer (VG Instruments, East Sussex, England). HR-MSs (ESI-TOF) data were recorded with an API QSTAR Pulsar 1 spectrometer.
Chemicals
All solvents used for product isolation were of analytical grade or higher. Silica gel (200–300 mesh, Qingdao Marine Chemical Factory, Qingdao, China) and Sephadex LH-20 (Amersham Pharmacia, Uppsala, Sweden) were used for column chromatography. Pre-coated silica gel GF254 plates (Qingdao Marine Chemical Factory, Qingdao) were used for TLC. Fractions were monitored by TLC, and spots were visualized by heating silica gel plates sprayed with 5% H2SO4 in ethanol.
Strains and Morphology
The endophytic fungus SWUKD3.1410 used in this study was isolated from fresh, healthy branches of K. angustifolia collected in November (dry season) 2012 at Xichou (N23°26′18.10″, E104°40′19.92″) of Yunnan province of China, lying approximately 55.8 km apart (Huang et al., 2015). The strain was preliminarily identified based on morphological characterization after 7 days in a PDA (potato 200 g, glucose 20 g, agar 18 g, and water 1000 mL) medium. To stimulate sporulation, the strain was cultured in the water-agar plates and placed in darkness at 28°C for up to 20 days. For morphological studies, slide cultures were prepared and stained with lactophenol cotton blue dye. Using a Canon EOS 5D Mark II digital camera, morphology of colonies (texture, color, and presence of pigmentation) grown on PDA media was photographed as described in Simoes et al. (2013). The microscopic characteristics (morphology of vegetative spores’ structures) were observed with an Axio Lab. A1 microscope (Carl Zeiss, Germany) equipped with an Axiocam ICc 5 digital camera (Carl Zeiss Vision, Germany). Standard taxonomic manuals were used to identify the fungal genus and species (Mahoney et al., 2004; Wang et al., 2013).
DNA Extraction, PCR Amplification, Sequencing, and Phylogenetic Analysis
The endophytic fungus SWUKD3.1410 was inoculated onto potato dextrose broth (PDB) and cultured at 28°C for 3 days. Then, the rejuvenated fungus was re-inoculated into 250 mL Erlenmeyer flasks containing 70 mL broth and incubated at 180 rpm at 28°C for 7 days. Finally, the culture was harvested by centrifugation at 12,000 rpm for 10 min. Genomic DNA was extracted from 0.5 to 1 g chilled mycelia in liquid nitrogen using the CTAB method (Zhang et al., 2006). The fungal ITS fragments (ITS1-5.8S-ITS2 rDNA) were amplified using the universal primers ITS1 and ITS4 (White et al., 1990). The PCR reaction mixtures (25 μL) contained 1 μl of genomic DNA, 2.5 μl of PCR buffer, 2 μl of MgCl2, 2 μl of forward and reverse primers, 2 μl of deoxyribonucleotide triphosphate (dNTP), 0.25 μl of rTaq polymerase (TaKaRa Biotechnology, Ltd., China) and 13.25 μl of double distilled water. The PCR reaction was composed of an initial denaturing step at 94°C for 3 min, followed by 32 amplification cycles at 94°C for 30 s, 56°C for 30 s, 72°C for 90 s, and a final extension at 72°C for 10 min. The PCR products were analyzed by agarose gel electrophoresis and purified using a DNA gel extraction kit (Omega Biotechnology, Ltd., China). The purified PCR product was sequenced using the same primers (BGI-Beijing, Beijing, China).
The endophytic fungi were classified by comparing the ITS sequences of endophytic fungi with the data available in NCBI using BLAST search. The resulting sequences were aligned with the Clustal X software (Larkin et al., 2007), with gaps treated as missing data. Phylogenetic tree was built by the neighbor-joining method using Mega6.0 software. The bootstrap was 1000 replications to assess the reliability of the inferred tree (Tamura et al., 2011).
Plant Material and Solid-State Fermentation of the Fungal Strain SWUKD3.1410
The stems and roots of K. angustifolia were collected in Xichou, Yunnan, China, in October 2014. The sample was identified by Prof. H. P. Deng (Southwest University), and a voucher specimen has been deposited in the herbarium of Southwest University. Fresh K. angustifolia plant material was cut to a size of 1 cm and air-dried.
In order to assess whether the high temperature sterilization process will affect the chemical components of K. angustifolia plant material and wheat bran, 6 g wet K. angustifolia plant material and wheat bran were put into 50 mL Erlenmeyer flasks respectively, and then they were routinely sterilized in autoclave (121°C, 30 min). After sterilization was finished, each flask was directly dried in oven at 55°C for 48 h, and then 30 ml acetone was added into the flask. The dried extracts were soaked at room temperature for 4 days, followed by evaporating all the extracts in a rotary evaporator under reduced pressure. Acetone extracts of 6 g non-sterilized dry K. angustifolia plant material and wheat bran were served as controls respectively. The process was similar as above mentioned. And the corresponding extracts were used for further TLC and HPLC analysis.
Umbelopsis dimorpha SWUKD3.1410 was activated on the surface of PDA at 28°C for 5 days and the mycelium-containing agar was then cut into 5-mm disks and inoculated in 50 mL Erlenmeyer flasks, containing 6 g of sterilized wet solid medium (a mixture of the stem and root segments of K. angustifolia) (6 g), which was adjusted to 60–70% (w/v) by distilled water containing 1% (w/v) CaCO3. The cultivation was performed in darkness at 28°C without shaking for 25 days. Meanwhile, the other three different medium, including wheat bran (6 g), the leached residue of K. angustifolia (K. angustifolia plant materials which were extracted with acetone) (6 g), as well as a mixture of wheat bran (2 g) and the stem and root segments of K. angustifolia (4 g), were used to culture U. dimorpha SWUKD3.1410 under the same conditions, respectively. After fermentation was finished, each flask was directly dried in oven at 55°C for 48 h, and then 30 ml acetone was added into the flask. The dried extracts were soaked at room temperature for 4 days, followed by evaporating all the extracts in a rotary evaporator under reduced pressure. And the corresponding residues left were used for further TLC and HPLC analysis. Substrate consisting of pure nigranoic acid, acetone extracts of sterilized K. angustifolia plant materials and wheat bran served as control, respectively.
The preparative-scale biotransformation and fermentation of the fungus was performed in 500 mL Erlenmeyer flasks containing wet K. angustifolia (100 g) or wet wheat bran (100 g). The process was similar as above mentioned. In brief, 20 5-mm disks were inoculated into the sterilized solid medium, and fermented in darkness at 28°C without shaking for 50 days. The fermented materials including all the mycelia and solid medium were collected together and dried at 60°C. All dried materials were extracted with acetone for 4 days for three times until no spots in the extracts were detected by TLC analysis. All the extracts were then put together and evaporated under reduced pressure in a rotary evaporator. After removing the solvent, 110 and 60 g dark brown residues were obtained from fermentation cultures of 5 kg K. angustifolia plant materials and 5 kg bran, respectively.
TLC and HPLC Analysis of Fermented Samples
The acetone extract of each fermented sample in preliminary experiments (10 μg/spot), was spotted on the start line of a 0.5 cm (5 cm × 10 cm) silica gel GF254 fluorescent TLC plates and developed in a solvent system (chloroform/ethyl acetate/glacial acetic acid at 6:4:0.1 v/v/v). In addition, acetone solutions of pure nigranoic acid and non-fermented K. angustifolia plant materials were also used as controls in TLC analysis. After air-dry, the plates were examined with a hand-held UV lamp at 254 nm. Then, the plates were sprayed with a chromogenic agent, concentrated sulfuric acid/absolute ethanol (5:95 v/v), and heated for 5 min at 150°C.
Additionally, each methanol solution of the above-mentioned fermented sample extracts (5 μl of a 1:100 enriched extract), as well as methanol solutions of pure nigranoic acid (5 μl of 0.1 mg/ml), sterilized K. angustifolia plant materials and sterilized wheat bran control (5 μl of a 1:100 enriched extract), were further analyzed on an Agilent 1100 series HPLC equipped with a Agilent ZORBAX Eclipse XDB-C18 column (4.6 mm × 250 mm, 5 μm). The mobile phase consisted of acetonitrile and methanol with 0.05% (vol/vol) phosphoric acid at a constant flow rate of 1 ml/min. The initial mobile phase consisted of MeCN-H2O at 20:80% (v/v), and this was maintained for 10 min, after which the gradient was change to MeCN-0.05% phosphoric acid at 45:55% (v/v) over a period of 10 min. This gradient was then returned to 85:15% (v/v) for 10 min, and then held for 10 min postrun reconditioning. The column temperature was held constant at 30°C, and the metabolites were monitored using a detection wavelength of 217 nm.
Extraction and Purification of Secondary Metabolites of Endophytic Fungus SWUKD3.1410 Grown on K. angustifolia
The residue (about 110 g) was subjected to dry column on silica gel (200–300 mesh, 4.0 cm × 90 cm, with 400 g of silica gel), and eluted with gradient mixtures of petroleum ether-AcOEt (1:0-0:1) to afford five main fractions (Frs. A-E). Fraction A (3.2 g) was further separated on Sephadex LH-20 column eluted with MeOH to obtain 51 fractions (Frs. A1-55). The combined Frs. A21-27 (400 mg) was further purified by a silica gel column chromatography (200–300 mesh, 1.2 cm × 32 cm, 16 g silica gel), and eluted with gradient mixtures of CHCl3-AcOEt (9:1-8:2) to yield compounds 10 (13 mg) and 3 (9 mg). The combined Frs. A29-37 (260 mg) was further supplied to a column chromatography on silica gel (200–300 mesh, 1.2 cm × 32 cm, 12 g silica gel), and eluted with gradient mixtures of petroleum ether-AcOEt (7:3-6:4) to give compound 14 (4 mg). The combined Frs. A40–51 (90 mg) was purified by Sephadex LH-20 and further separated by preparative TLC to give compounds 16 (2 mg) and 15 (3 mg). Fraction B (2.1 g) was further purified to Sephadex LH-20 column eluted with acetone to afford 62 fractions (Frs. B1-62). The combined Frs. B16-23 (200 mg) was further chromatographed on silica gel column (200–300 mesh, 1.2 cm × 32 cm, with 12 g of silica gel), and eluted with gradient mixtures of CHCl3-AcOEt (6:4-5:5) to give compounds 1 (50 mg), 5 (17 mg), 7 (3 mg), and 6 (21 mg). In the same manner, Fr. C (800 mg) was purified to give compounds 1 (25 mg), 8 (2.7 mg), and 12 (15 mg). Fraction D (3.1 g) was chromatographed on Sephadex LH-20 column eluted with MeOH to afforded 78 fractions (Frs. D1-67). The combined Frs. D8-31 (650 mg) were repeatedly separated by a silica gel column chromatography (200–300 mesh, 1.7 cm × 38 cm, 30 g silica gel), and eluted with gradient mixtures of CHCl3-AcOEt (5:5-3:7) to yield compounds 1 (65 mg), 2 (8 mg), 9 (2 mg), and 13 (7 mg). Fraction E (2.8 g) were further separated by a silica gel column chromatography (200–300 mesh, 2.6 cm × 38 cm, 80 g silica gel), and eluted with mixtures of CHCl3- AcOEt (3:7) to yield compounds 4 (13 mg) and 11 (3 mg).
Extraction and Purification of Secondary Metabolites of Fungus SWUKD3.1410 Grown on Wheat Bran
The residue (about 60 g) was subjected to dry column on silica gel (200–300 mesh, 4.0 cm × 90 cm, with 400 g of silica gel), and eluted with gradient mixtures of petroleum ether-AcOEt (1:0-0:1), to afford six fractions (Frs. A-F). Fraction B (2 g) was further supplied to a column chromatography on silica gel (200–300 mesh, 2.0 cm × 40 cm, with 53 g of silica gel), and eluted with gradient mixtures of petroleum ether-AcOEt (8:2-6:4) to give compounds 17 (25 mg) and 19 (4 mg). Fraction C (1.7 g) were chromatographed on silica gel (200–300 mesh, 2.0 cm × 40 cm, with 53 g of silica gel), and eluted with gradient mixtures of petroleum ether-AcOEt (6:4-5:5) to give compounds 18 (7 mg) and 1 (80 mg). Fraction D (1.1 g) was further supplied to a Sephadex LH-20 column eluted with methanol to afford compounds 20 (5.3 mg) and 4 (5 mg).
Structural Determination of the New Secondary Metabolites
23α-hydroxynigranoic acid (2): acicular crystal: : +15.3° (c 0.18, MeOH); UV (MeOH) λ_max (logε): 205.00 (4.20), 403.00 (2.48) and 549.5 (2.27) nm; IR (KBr): νmax = 3425, 3068, 3038, 2928, 2872, 1695, 1570, 1451, 1404, 1303, 1283, 1252, 1155, 1125, 1055, 979, 891, 858, 826, 777, 720, 688, 606, 570, 544, 479, 454, 430 cm-1; 1H and 13C-NMR (CD3OD, 600 MHz) data (Table 1); HR-ESI-MS m/z: 509.3228 ([M+Na]+, calc. for C30H46O5Na, 509.3237).
6,8-Dihydroxy-schensianol A (11): colorless oil: : +22.2° (c 0.12, MeOH); UV (MeOH) λ_max (logε): 202.00 (3.79), 251.60 (2.51) and 298.40 (1.77) nm; IR (KBr): νmax = 3406, 3396, 3387, 3088, 2974, 2933, 2876, 2641, 1847, 1714, 1642, 1548, 1453, 1410, 1381, 1378, 1342, 1309, 1291, 1267, 1207, 1170, 1074, 1056, 997, 944, 922, 817, 788, 757, 740, 691, 602, 681 cm-1;1H and 13C-NMR (CD3COCD3, 600 MHz) data (Table 1); HR-ESI-MS m/z: 311.1832 ([M+Na]+, calc. for C15H28O5 Na, 311.1829).
(R∗, E)-2- (3-(2-hydroxypropan-2-yl) cyclopentylidene) acetic acid (14): white amorphous powder: +1.3° (c 0.06, CHCl3); UV (CHCl3) λ_max (logε): 206.50 (2.72), 217.50 (2.74) and 239.50 (3.14) nm; IR (KBr): νmax = 3302, 3127, 2975, 2956, 2930, 2880, 2667, 2623, 2525, 2168, 2034, 1936, 1906, 1689, 1647, 1466, 1427, 1381, 1310, 1270, 1221, 1175, 1143, 1110, 1088, 1072, 1034, 1002, 965, 921, 867, 826, 770, 734, 694, 618, 572, 543, 499, 475, 445, 416 cm-1;1H and 13C-NMR (CDCl3, 600 MHz) data (Table 1); HR-ESI-MS m/z: 207.0994 ([M+Na]+, calc. for C10H16O3Na, 207.0992).
Results
Identification of the Fungus SWUKD3.1410
Macroscopic examination of the isolate revealed that the fungus was filamentous, with velvety white mycelia on the surface of colony (Figures 2A,B). Colonies on PDA after 5 days reaching 45 mm diameter at 28°C, 1–4 mm high, flat, or high in the center and low at the edge, often with white sectors, velvety. The colonies on water-agar plates after 10 days tended to be sporangiophores with single-spored sporangia (Figure 2C), which is consistent with the morphological description of U. dimorpha in the published literature (Mahoney et al., 2004; Wang et al., 2013). The 5.8S rDNA gene sequence of strain SWUKD3.1410 was determined and classified in the genus Umbelopsis on the basis of its phylogenetic affiliation. Figure 3 shows phylogeny tree of the isolated fungus based on neighbor-joining analysis compared to other similar fungi stains. Based on molecular taxonomy and morphology investigation of the strain SWUKD3.1410, this fungus was identified as U. dimorpha. And the nucleotides sequences were submitted to GenBank and provided a GenBank Accession No. KM013438.
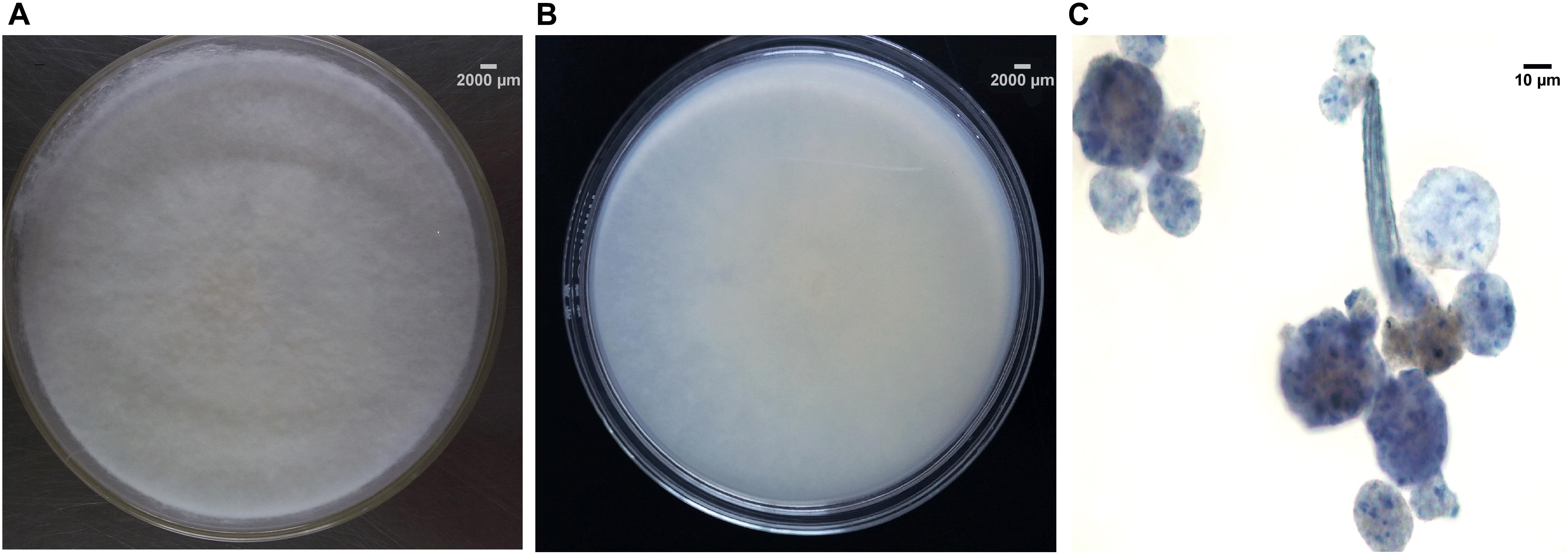
FIGURE 2. Morphological characteristics and microscopic morphology of SWUKD3.1410. (A) Front view of Umbelopsis dimorpha SWUKD3.1410 grown on PDA media 3 days; (B) Back view of U. dimorpha SWUKD3.1410 grown on PDA media 3 days; (C) Microscopic structures (multi-spored and single-spored sporangia) of U. dimorpha SWUKD3.1410 grown on water-agar media 10 days.
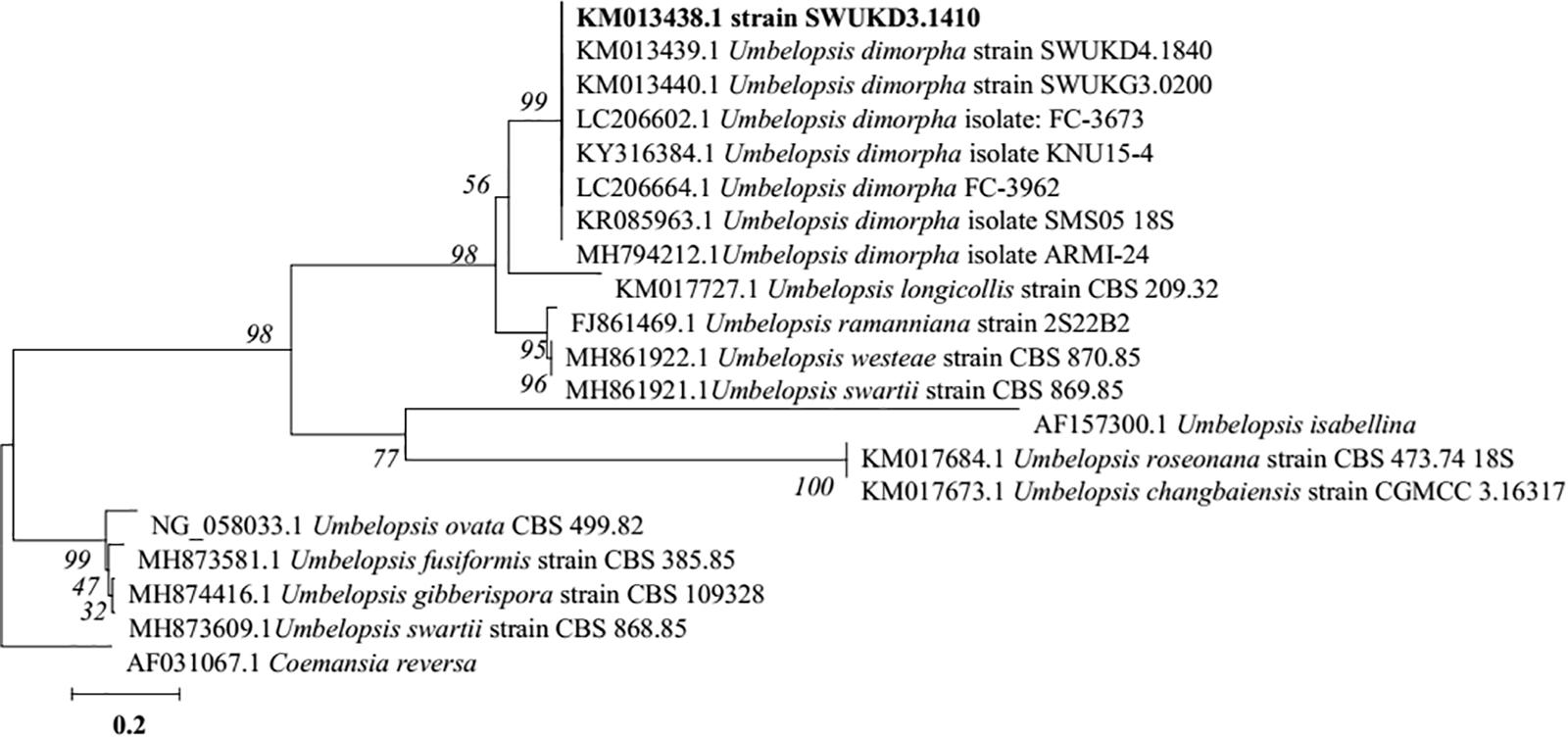
FIGURE 3. Neighbor-joining tree based on ITS rDNA sequence of the fungus WUKD3.1410 and its closest ITS rDNA matches in the GenBank.
TLC and HPLC Analysis of Fermented Samples
The acetone extracts of sterilized/non-sterilized K. angustifolia plant materials and wheat bran were respectively analyzed by TLC and HPLC methods. As seen in the TLC plates (Supplementary Figure S1A) and the chromatographic profiles of HPLC (Supplementary Figure S1B), the high temperature sterilization process has almost no effect on the chemical components of the K. angustifolia plant materials and wheat bran, especially nigranoic acid, the main component of K. angustifolia.
The fermentative extracts of U. dimorpha SWUKD3.1410 respectively grown on K. angustifolia plant materials and wheat bran were analyzed by TLC and HPLC methods. K. angustifolia plant materials, wheat bran, nigranoic acid and the culture of U. dimorpha SWUKD3.1410 grown on the mixed materials consisting of wheat bran and K. angustifolia plant materials (1:2) were respectively served as controls. As seen in the TLC plates (Figure 4A), the chromatographic profile from K. angustifolia plant materials was similar to that from U. dimorpha SWUKD3.1410 grown on wheat bran while the chromatographic profile from U. dimorpha SWUKD3.1410 grown on K. angustifolia plant materials was almost identical to that grown on the mixed materials. Conversely, the TLC chromatographic profiles from fermented K. angustifolia by U. dimorpha SWUKD3.1410 were significantly different from those from K. angustifolia plant materials and U. dimorpha SWUKD3.1410 grown on wheat bran. Two fermented K. angustifolia extracts were revealed to yield more polar compounds than K. angustifolia plant materials and single cultures of U. dimorpha SWUKD3.1410. The result was proved again by the chromatographic profiles of HPLC analysis (Figure 4B). As for the leached residue of K. angustifolia, it was not analyzed because U. dimorpha SWUKD3.1410 could not colonize.
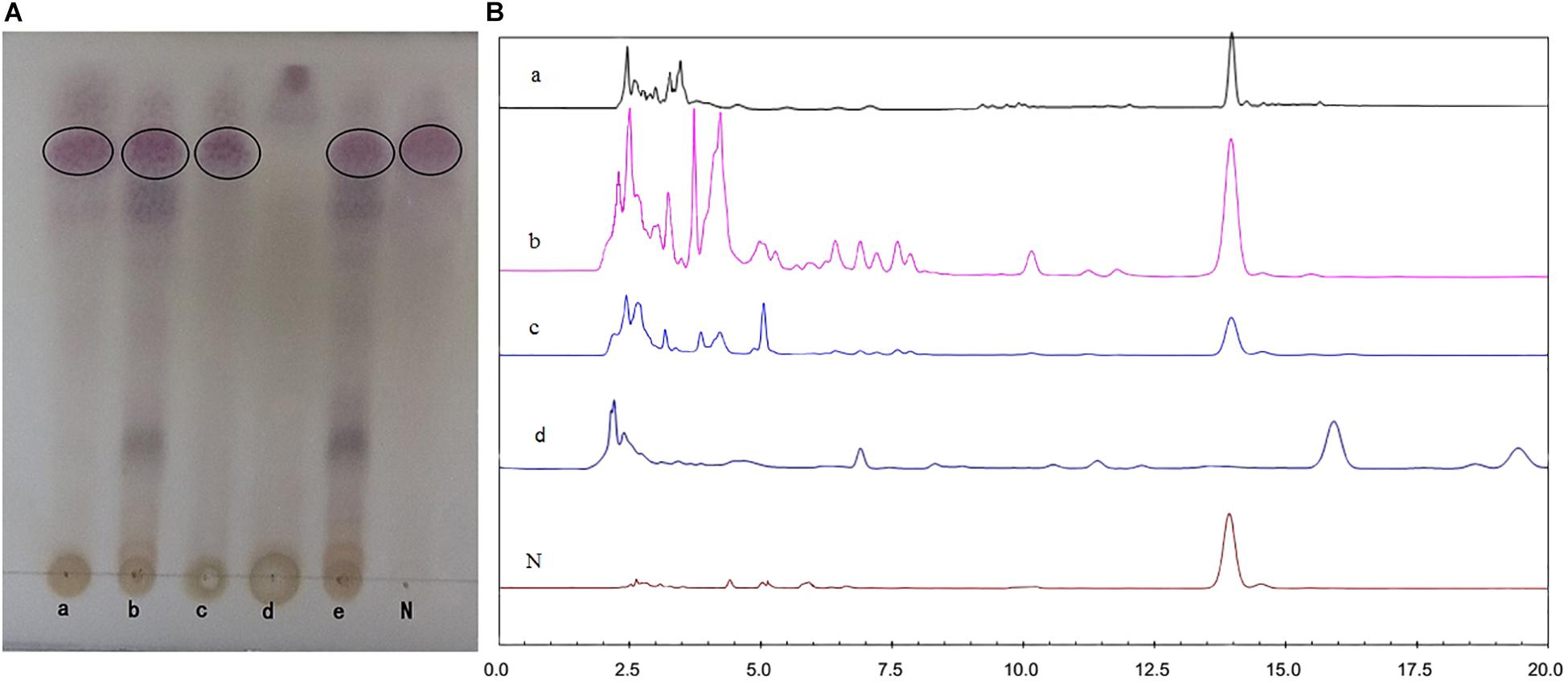
FIGURE 4. Profiles of chromatograms of extracts from treated different samples. (A) Profiles of chromatograms obtained after TLC of the acetone extracts (5 μl of a 1:100 enriched extract) of treated different samples. (B) Profiles of HPLC analysis of methanol extracts of treated different samples (5 μl of a 1:100 enriched extract, detection at UV 217 nm). (a) Sterilized Kadsura angustifolia control; (b) U. dimorpha SWUKD3.1410 grown on K. angustifolia; (c) U. dimorpha SWUKD3.1410 grown on bran; (d) sterilized wheat bran control; (e) U. dimorpha SWUKD3.1410 grown on a mixture of K. angustifolia and bran (1:2); N, nigranoic acid (5 μl of 0.1 mg/ml).
Structural Identification of Compounds From the Fermented K. angustifolia Plant Materials
From the acetone extract of the fermented K. angustifolia plant materials by U. dimorpha SWUKD3.1410, 16 secondary metabolites were obtained. Based on the 1D, 2D-NMR and MS data, their structures were identified as nigranoic acid (1) (Dong et al., 2007a)[26], 23α-hydroxynigranoic acid (2), abiesatrine J (3) (Yang et al., 2010), schisanlactone B (4) (Liu et al., 1983), henrischinin A (5) (Xue et al., 2011), henrischinin B (6) (Xue et al., 2011), lancifodilactone D (7) (Li et al., 2004), schirubridilactone D (8) (Xiao et al., 2010b), wuweizidilactone D (9) (Huang et al., 2007), gomisin D (10) (Chen et al., 2005), 6,8-dihydroxy-schensianol A (11), schensianol A (12) (Zheng et al., 2009), crocinervolide (13) (Ortega et al., 1989), (R∗, E)-2- (3-(2-hydroxypropan-2-yl) cyclopentylidene) acetic acid (14), 4-hydroxy-3-methoxybenzoic acid (15) (Sivakumar et al., 2010), 4-hydroxybenzoic acid (16) (Sivakumar et al., 2010), respectively. Among them, compounds 2, 11, 14 have not been identified before.
Compound 2 was obtained as acicular crystal and its molecular formula was assigned as C30H46O5 by HR-ESI-MS at m/z 509.3228 [M+Na]+ (calcd for C30H46O5Na 509.3237) (Supplementary Figure S2). The IR spectrum showed absorptions at 3425, 1695 and 1570 cm-1, indicating the presence of hydroxyl, carbonyl and double-bond functionalities. The 1H-NMR spectrum showed five methyls [one secondary methyl at δH 0.92 (d, J = 6.2 Hz), two tertiary methyls at δH 0.90 (s) and 0.96 (s), and two olefinic methyls at δH 1.71 (s) and δH 1.66 (s)], an oxymethine at δH 4.33 (1H, m), one olefinic methine at δH 6.53 (d, J = 7.9 Hz) and one pair of typical cycloartane methylene protons [δH 0.69 and 0.38 (each 1H, d, J = 3.8 Hz)] (Table 1 and Supplementary Figure S3). The 13C-NMR and HMQC spectra of 2 resolved 30 carbon signals, corresponding to two carbonyl groups, four double-bonded carbons, four quaternary carbons, five methines (one is oxygenated), 10 methylenes, and five methyls (Table 1 and Supplementary Figures S4, S5). These features closely resembled those of nigranoic acid (1) except for the absence of one CH2 and the presence of one additional CH-OH group (δH 4.33 and δC 64.9), suggesting the OH-substituted nigranoic acid backbone for 2. This hydroxyl group was located at C-23 because of the 1H-1H COSY correlation (Figure 5) of H-23 (δH 4.33, m) with H-24 (δH 6.53, d, J = 7.9 Hz) and HMBC correlations from the oxymethine proton signal at δH 4.33 (H-23) to C-22 (δC 43.4), C-24 (δC 145.3) and C-25 (δC 126.7), and the olefinic proton at δH 6.53 (H-24) to C-23 (δC 64.9) and C-22 (Figure 5 and Supplementary Figure S6). The NOESY correlations of H-23 with CH3-21 (δH 0.92, d, J = 6.2 Hz) and H-24, and correlations of CH3-21 with H-17 (δH 1.54, m), H-20 (δH 1.68, m), and Hα-22 (δH 1.55, m) suggested that the H-23 was β-oriented (Figure 5 and Supplementary Figure S7). This was further confirmed by the absence of NOESY correlation of H-23 with Hα-22. The NOESY correlation of H-24 with CH3-26 (δH 1.71, s), determined the double bond at C-24/C-25 to be Z configuration. The similarity of chemical shifts and other observed NOESY correlations suggested other chiral centers possess the same relative stereochemistry as those of nigranoic acid (1) (Dong et al., 2007a). Consequently, the structure of 2 (Figure 1) was identified as 23α-hydroxynigranoic acid.
Compound 11 was isolated as colorless oil and assigned to have a molecular formula of C15H28O5 by the HR-ESI-MS at m/z 311.1832 ([M+Na]+, calcd for C15H28O5Na 311.1829), indicating two indices of hydrogen deficiency (Supplementary Figure S8). Analysis of 1H-NMR, 13C-NMR, and HMQC data for 11 revealed the presence of two olefinic carbons (including three olefinic protons), along with four methyl groups, three methylene carbons, three oxygenated methine carbons, and three oxygenated quaternary carbons (Table 1 and Supplementary Figures S9–S11). These data accounted for all but four exchangeable protons [δH 3.57 (s), 3.74 (d, J = 4.7 Hz), 3.78 (s) and 4.27 (s)] and required 11 to be monocyclic. Analysis of COSY results (Figure 6 and Supplementary Figure S12) led to the identification of three isolated proton spin-systems corresponding to the C-1-C-2, C-4-C-5-C-6-6-OH, and OH-8-C-8-C-9-C-10 subunits of structure 11. The former two units and C-15 (δC 28.6) were joined at one oxygenated quaternary carbon (C-3, δC 72.9), as evidenced by the HMBC correlations (Figure 6 and Supplementary Figure S13) of CH3-15 (δH 1.21, s) with C-2 (δC 147.2), C-3 (δC 72.9), and C-4 (δC 40.7), and OH-3 (δH 3.78, s) with C-15 and C-4, while the latter two units and C-14 (δC 17.4) were established to attach to another oxygenated quaternary carbon at C-7 (δC 88.7) deduced from the HMBC correlations from CH3-14 (δH 1.09, s) to C-6 (δC 78.6), C-7, and C-8 (δC 73.2), and from OH-8 (δH 3.74, d, J = 4.7 Hz) to C-7. Furthermore, the key HMBC correlations from CH3-12 (δH 1.18, s) to C-10 (δC 83.2) and C-11 (δC 71.4), CH3-13 (δH 1.05, s) to C-10 and C-11, and OH-11 (δH 3.57, s) to C-10, C-11, C-12 (δC 27.4) and C-13 (δC 26.5) supported a 2-hydroxy propyl unit at C-10. Finally, these data, together with the chemical shifts of C-7 and C-10, indicated that C-7 is connected to C-10 via an oxygen atom, thereby constructing the dihydrofuran moiety of 11, accounting for the monocyclic structure of 11 required by the molecular formula. Based on the above evidence, the planer structure of 11 was established.
The relative stereochemistry of compound 11 was established by analysis of 1H-1H coupling constants and NOESY data and by comparison its 1H-NMR data with those of the co-existing known metabolite schensianol A (12). Specifically, the fact that the oxymethine proton on C-6 exhibited a larger (JH-6,Hα-5 = 10.2 Hz) coupling constant with Hβ-5 (δH 1.63, m) but no coupling constant with Hα-5 (δH 1.44, m) indicated the hydroxyl group attached to C-6 in a pseudoequatorial orientation. This was also further confirmed by the observed NOESY correlations of H-6 (δH 3.38, d, J = 10.2 Hz) with CH3-14 (δH 1.09, s), Hα-4 (δH 1.52, m) and Hβ-5 (Figure 6 and Supplementary Figure S14). Additionally, the NOESY correlations of H-8 (δH 4.32, m) with CH3-14, Hα-9 (δH 2.24, m) and Hβ-9 (δH 1.79, m), as well as OH-8 with Hβ-9 and CH3-14 indicated a β-orientation for the 8-OH. Accordingly, the structure of 11 was, therefore, deduced to 6,8-dihydroxy-schensianol A, as shown in Figure 1.
Compound 14 was isolated as white powder and assigned to have a molecular formula of C10H16O3 by the HR-ESI-MS at m/z 207.0994 ([M+Na]+, calcd 207.0992), indicating three degrees of unsaturation (Supplementary Figure S15). The IR spectrum showed absorptions at 3302, 1689 and 1647 cm-1, suggesting the presence of hydroxyl, carbonyl and double-bond functionalities. The 1H-NMR spectrum in CD3Cl solvent showed the presence of two methyls [δH 1.21 (s), and δH 1.22 (s)], and one olefinic methine at δH 7.04 (s) (Table 1 and Supplementary Figure S16). Analysis of 13C-NMR and HSQC data revealed the presence of two methyl groups, three methylenes, one sp3 methine, one oxygenated quaternary carbon, two olefinic carbons (one is protonated), and one carbonyl carbon (δC 170.1) (Table 1 and Supplementary Figures S17, S18). These data accounted for all but two exchangeable protons, and indicated that 14 must contain one carbonyl group and one hydroxyl group. Besides, analysis of COSY results led to the identification of one isolated proton spin-system corresponding to the C-4-C-7 subunit of structure 14 (Figure 7 and Supplementary Figure S19). The HMBC correlations of CH3-9 (δH 1.21, s) with C-5 (δC 44.1) and C-8 (δC 72.3), and CH3-10 (δH 1.22, s) with C-5 and C-8, revealed that C-9 (δC 27.2), C-10 (δC 27.4) and the C-5 of C-4-C-7 unit were joined at C-8 (Figure 7 and Supplementary Figure S20). The cross-peaks of H-2 (δH 7.04, s) with C-4 (δC 26.5, t) and C-7 (δC 25.0), H-4 (δH 2.03, m) with C-3 (δC 129.9) and C-5, and H-7 (δH 2.52, m) with C-3 and C-6 (δC 23.3) led to the direct connection of the quaternary olefinic carbon C-3 to C-4 and C-7, which yielded a cyclopentane ring. The above mentioned HMBC correlations also indicated that the olefinic proton carbon C-2 (δC 140.5) was connected to the carbonyl C-1 (δC 170.1). On the basic of these data, the planer structure of 14 was established. The proton at C-5 was determined to be β-oriented by the observed ROESY correlations of H-5 (δH 1.54, m) with CH3-9, CH3-10, Hβ-4 (δH 2.34, m), Hβ-6 (δH 1.24, m), and Hβ-7 (δH 2.17, m) (Figure 7 and Supplementary Figure S21). Additionally, C-2/C-3 double bond was undisputedly determined as having E-geometry, judging from the ROESY correlations of H-2 with Hα-4 (δH 2.03, m) and Hβ-4. All these evidences established the structure of 14 as (R∗, E)-2- (3-(2-hydroxypropan-2-yl) cyclopentylidene) acetic acid (Figure 1).
Secondary Metabolites of U. dimorpha SWUKD3.1410 Grow on Wheat Bran
From the acetone extract of the endophytic fungus U. dimorpha SWUKD3.1410 grow on wheat bran, six secondary metabolites were obtained. Based on the 1D, 2D-NMR and MS data, their structures were identified as nigranoic acid (1) (Dong et al., 2007a), schisanlactone B (4) (Liu et al., 1983), schisandronic acid (17) (Li et al., 2003), 3-methyl ester-nigranoic acid (18) (Sy and Geoffrey, 1998), sitostanone (19) (Luo et al., 2009), gomisin C (20) (Jiang et al., 2005), respectively. Their structures were shown in Figure 1. As far as we know, this is the first study to report nigranoic acid-producing fungi.
Discussion
Through the long period of co-evolution, some endophytes and their plant hosts have established a special interaction relationship, which can significantly influence the biosynthesis of host metabolism. Previously, the interactions between endophytic fungi and plant hosts have mainly focused on the effects of fungal colonization on secondary metabolism of living host–plant. Almost exclusively, these studies have demonstrated that the fungus–plant interactions relates to the production of secondary metabolites which play a critical role in their interactions, especially for defense, signaling purposes, and regulation of the symbiosis (Schulz and Boyle, 2005). Recently, an alternative approach to study the influence of an endophytic fungus on the host–plant metabolic profiles has been achieved by the analysis of the fungal impacts on the compositions of the host–plant secondary metabolites and their feedback effects on the fungal growth (Tian et al., 2014). Additionally, many studies on microbial transformation have demonstrated that endophytes play an important role in producing novel compounds and modifying their structures and bioactivities (Zeng et al., 2013; Gniłka et al., 2015; Smith et al., 2015). In this paper, in order to obtain highly oxygenated schitriterpenoids/schinortriterpenoids and assess the impact of SWUKD3.1410 on the secondary metabolites of host–plant K. angustifolia, U. dimorpha SWUKD3.1410 was respectively grown on dead K. angustifolia plant materials and wheat bran, and their corresponding fermented products were analyzed.
When cultivated in bran, the unexpected results were obtained that U. dimorpha SWUKD3.1410 also produced nigranoic acid (1), which was the major active component of the host plant K. angustifolia (Huang et al., 2015). Compound 1 has been revealed to possess various bioactivities, including cytotoxicity toward hela and leukemia cell lines, and inhibition of HIV reverse transcriptase and polymerase expression (Sun et al., 1996; Shi et al., 2015). Here, relatively high amounts of 1 were found in the wheat bran cultures of U. dimorpha SWUKD3.1410, producing at levels of over 0.37% of the crude extracts. Besides 1, three additional triterpenes (4, 17, 18), one sterol sitostanone (19) along with one dibenzocyclooctadiene lignan gomisin C (20) were found in the cultures of U. dimorpha SWUKD3.1410 as well, which were similar to the characteristic components of host plant K. angustifolia (Gao et al., 2008; Sun et al., 2011). Taken together with the reported chemical components of wheat bran (Posner, 2009; Zhu et al., 2013, 2015; Prinsen et al., 2014; Geng et al., 2015), we considered that these metabolites (1, 4, and 17–20) should be synthesized by U. dimorpha SWUKD3.1410 rather than from the medium. Additionally, the fact that the U. dimorpha SWUKD3.1410 yielded the same and similar compounds as its host–plant K. angustifolia (Sun et al., 2011) demonstrated that partial or complete biosynthesis pathways of K. angustifolia can be attributed to the endophytic fungus U. dimorpha SWUKD3.1410. The ability of endophytic fungi to produce host–plant secondary metabolites has been postulated as a horizontal gene transfer between endophytic fungi and host plant for a long time. That is, fungus may obtain the biosynthetic gene as plasmid or extra chromosomal element from its host plant or vice versa (Sachin et al., 2013). However, it has not yet been confirmed although there has been one report on horizontal gene transfer between plant and its symbiotic bacteria (Taghavi et al., 2005). Additionally, some researches have demonstrated that the pathway of endophytic fungi biosynthesizing the same plant metabolite was completely different from that of plant hosts (Boemke and Tudzynski, 2009). For example, the hyp-1 gene responsible for the biosynthesizing hypericin by Thielavia subthermophila was found to be absent in its host plant Hypericum perforatum (Kusari et al., 2009). Occasionally, it has been strongly suggested that the interactions between plant hosts and endophytes contribute to the co-production of bioactive secondary metabolites (Heinig et al., 2013). In light of these, we assumed that the biosynthesis of nigranoic acid both by K. angustifolia and U. dimorpha SWUKD3.1410 may be related to its ecological adaptability to environmental conditions, and such includes the ready exchange of genetic information that enables the endophytic fungus to mimic the chemical environment of its host and, ultimately, to acquire a lot of evolutionary superiorities because no other U. dimorpha strains have been detected to synthesize schitriterpene-type metabolites (Tan and Zou, 2001; Wang et al., 2017). Further comparative study on the genomes between U. dimorpha SWUKD3.1410 and other strains may shed light on the molecular mechanisms which trigger same and/or similar chemical reactions of Schisandraceae plant with U. dimorpha SWUKD3.1410.
From K. angustifolia plant materials fermented by a symbiont endophytic fungus, U. dimorpha SWUKD3.1410, 16 secondary metabolites (1–16) were isolated and identified, including three new metabolites (2, 11, and 14). Of them, metabolites 1–6 belong to schitriterpenoids while 7–9 are schinortriterpenoids. Specifically, abiesatrine J (3) was a rare C-24/C-25 double bond isomer of nigranoic acid (1) and was only found in two plants, Abies georgei Orr (Yang et al., 2010) and Hopea odorata Roxb (Satiraphan et al., 2015). Henrischinins A-B (5–6) were previously isolated from Schisandra henryi (Xue et al., 2011) and S. chinensis (Song et al., 2013), and reported to have moderate to weak activity against HSV-2 and adenovirus (Boemke and Tudzynski, 2009) and HL-60 cell lines (Xue et al., 2011). Biogenetically, the rare 3-oxo-2-oxabicyclo[3,2,1]octane moiety of 5–6 probably arose from schisanlactone B (4) after a Michael addition as proposed by Xia and coworkers (Xia et al., 2014). As a key intermediate, 4 was repeatedly found in S. propinqua (Chen et al., 2001), Schisandra henryi (Chen et al., 2003), and K. heteroclita (Wang et al., 2006) and reported to have moderate cytotoxic activity against Leukemia cells and HL-60 cells in vitro (Heinig et al., 2013). As for schinortriterpenoids 7–9, what the most worth mentioning was that they were obtained many times from the genus Schisandra but never from genus Kadsura so far (Huang et al., 2008; Lei et al., 2009; Xiao et al., 2010a; He et al., 2012). From the viewpoint of chemical structures, schitriterpenoids 2–6 and schinortriterpenoids 7–9 were considered to probably arise from nigranoic acid (1), the main component of K. angustifolia plant, because they have never been detected in our previous chemical investigation on the same samplings (Sun et al., 2011), although we found that the U. dimorpha SWUKD3.1410 yielded the same and similar compounds as its host–plant K. angustifolia. Furthermore, the plausible biosynthetic pathways of these products have been well-proposed in the previous reviews on triterpenoids from the Schisandraceae family (Xiao et al., 2008; Shi et al., 2015). Finally, it should be noted that our study will be interesting if 7–9 were transformed products of nigranoic acid (1), because this is the first discovery of highly oxygenated nortriterpenoids by microbial technology. Compared with our reported biotransformation on the substrate nigranoic acid by the same fungus (Wang et al., 2017), the present investigation to detect and isolate highly oxygenated schitriterpenoids/schinortriterpenoids from K. angustifolia fermented by its associated endophytic fungus, U. dimorpha SWUKD3.1410 was successful, indicating perhaps the necessity of specific host plant environment for microbial conversion.
With regard to compound 10, it was elucidated as a known lignan, gomisin D, which possessed a unique structure with an additional 11-membered ring resulting from a cyclization of a tigloyl moiety with a phenolic hydroxyl group and no double bond in the cyclooctadiene ring. Previously, 10 was isolated from S. chinensis (Mocan et al., 2016) and S. grandiflora (Poornima et al., 2016) and suggested that not its amount but rather its chemical structure was responsible for the highest scavenging activity in the DPPH cuvette assay and the best ability to inhibit tyrosine nitration in 14 tested lignins (Mocan et al., 2016). In another paper, gomisin D (10) showed potent inhibition of the AChE activity with the IC50 value as 7.84 ± 0.62 μM, implying that it may be the active component in the plants that is useful in treatment of Alzheimer’s disease (Hung et al., 2007). In addition, this investigation also led to the discovery of one monoterpene (14), one trinorsesquiterpenoid (13), two sesquiterpenoids (11–12), and two simple aroma compounds (15–16). To our knowledge, only a few sesquiterpenoids and monoterpenoids have been obtained from several species of Kadsura, such as K. heteroclita (Roxb.) (Li and Luo, 2002), K. longipedunculata (Pu et al., 2008), K. interior (Dong et al., 2013). However, the terpenes (11–14) found in the fermented K. angustifolia materials were completely different from them. From the literature report, these terpenoids and phenolics are essential to the plant’s healthy growth because they may provide the plant defense against fungi, bacteria, helminths, and insects in its natural environment (Chadwick et al., 2013).
As hypothesized by Demain: if a fungus can produce metabolites in vitro they must have a function in nature. The multienzyme reaction cascade required for the synthesis of secondary metabolites would not be retained by fungi without some beneficial effect for survival (Demain, 1980). Accumulating evidence has demonstrated that endophytic colonization may improve the host’s ecological adaptability under the environment stress by producing antimicrobial metabolites against phytopathogens and predators (Tan and Zou, 2001; Schulz and Boyle, 2005). As previously found for pathogenic fungi, the plants whose roots are usually colonized by endophytes often grew faster than non-infected ones (Pegg and Ayres, 1984). The phenomenon was supposed to be due to the synthesis of plant hormones and other growth-promoting substances by fungi (Petrini, 1991; Tudzynski, 1997; Tudzynski and Sharon, 2002). Recently, several reports have described the influence of fungal endophytes on the secondary metabolites of host–plant. It was notably the case that the induction of phenols was observed after infection with an endophytic fungus Colletotrichum tropicale from the Clavicipitaceae family, which was found to discourage leaf-cutting ants (Estrada et al., 2013). In another case, Paraconiothyrium variabile, a leaf endophytic fungus isolated from the leaves of Cephalotaxus harringtonia, was unraveled to induce a special delycosylation of the host plant flavonoids for its own growth in the host–plant (Tian et al., 2014). Taken together, we assumed that metabolites 1–20 found here in the endophytic fungus U. dimorpha SWUKD3.1410 may play a fundamental role within the host, and (or) have ecological significance, even if this required more research to confirm.
In a conventional view, it is widely considered that the quality and quantity of crude drugs originated from medicinal plants is highly correlated to the genetic background, ecological habitats, and soil nutrients of the concerned plants. Recently, it is gradually recognized that endophytic microbes have served a very important function in enhancing the quality and quantity of medicinal plants through a specific fungus–host interaction (Jia et al., 2016). Endophytic fungi have been found to have fundamental impacts on the biogeographic distribution of host plant species. Especially for those plants that have to rely on certain endophytic fungi to provide nutrients to complete their life history, endophytic fungi are more likely to be an important limiting factor (Jia et al., 2016). For several medicinal plants, it has been indicated that they are active due to their microbial endophytes (Li et al., 2012; Miller et al., 2012). Additionally, among the various so-called “plant metabolites,” especially those exclusive to their host plants, many have been detected in cultivated endophytic fungi (Kusari et al., 2012), as shown in our study. Occasionally, certain plant metabolites can’t be synthesized without the involvement of fungi (Kusari et al., 2011a,b). On the other hand, the colonization of endophytic fungi is not random due to the chemotaxis which is specific chemicals produced by the host plants (Carroll and Petrini, 1983). Generally, the distribution of certain endophytic fungal populations was only restricted to particular genetic background of a species and particular host plant species (or families). Under certain special conditions, the distribution of endophytic fungi from the same regions is highly similar, while species and their population structure of endophytic fungi even in the same host plant species from different regions usually have very low similarity (Jiang et al., 2010). In light of these, we assumed that genuine medicinal materials with the highest quality and best effects to a certain disease had a special relationship with endophytic fungi of medicinal plants. Additionally, it should be noted that the cultivation of endophytes without their host may lead to the loss of the desired compound synthesis, since the plant host can change or influence the metabolic pattern of secondary metabolites in endophytic fungi (Ludwig-Müller, 2015). Thus, more understanding on the beneficial symbiosis can help to enhance the synthesis and accumulation of bioactive metabolites of the host medicinal plants for higher quality of crude drugs.
Author Contributions
DQ and LW conceived and designed the experiments. DQ, LW, and JW performed the experiments. DQ and JD analyzed the data. MH, HS, XY, and XD contributed reagents, materials, and analysis tools. DQ and LW wrote the paper.
Funding
This work was financially supported by the National Natural Science Foundation of China (Grant Nos. 31270091 and 20762015) and the Natural Science Foundation of Chongqing (Grant No. cstc2018jcyjA0864).
Conflict of Interest Statement
The authors declare that the research was conducted in the absence of any commercial or financial relationships that could be construed as a potential conflict of interest.
Acknowledgments
The authors thank professor Xiao-Yong Liu and his research group for assistance with identifying the fungal genus and species. Special thanks are due to corresponding authors’ colleague Professor Ru Li for acting as a pre-submission reviewer and improving the language quality.
Supplementary Material
The Supplementary Material for this article can be found online at: https://www.frontiersin.org/articles/10.3389/fmicb.2018.02845/full#supplementary-material
References
Boemke, C., and Tudzynski, B. (2009). Diversity, regulation, and evolution of the gibberellin biosynthetic pathway in fungi compared to plants and bacteria. Phytochemistry 70, 1876–1893. doi: 10.1016/j.phytochem.2009.05.020
Borges, W. S., Borges, K. B., Bonato, P. S., Said, S., and Pupo, M. T. (2009). Endophytic fungi: natural products, enzymes and biotransformation reactions. Curr. Org. Chem. 13, 1137–1163. doi: 10.2174/138527209788921783
Carroll, G., and Petrini, O. (1983). Patterns of substrate utilization by some fungal endophytes from coniferous foliage. Mycologia 75, 53–63. doi: 10.2307/3792923
Chadwick, M., Trewin, H., Gawthrop, F., and Wagstaff, C. (2013). Sesquiterpenoids lactones: benefits to plants and people. Int. J. Mol. Sci. 14, 12780–12785. doi: 10.3390/ijms140612780
Chen, H. Y., Zhou, C. X., Yao, W., Wang, L. W., Dou, H., and Dong, X. W. (2005). NMR study of two lignans isolated from Schisandra chinensis. Chin. J. Magn. Reson. 22, 43–54.
Chen, Y. G., Qin, G. W., and Xie, Y. Y. (2001). A novel triterpenoid lactone schiprolactone A from Schisandra propinqua (Wall) Hook. f. et. Thoms. Chin. J. Chem. 19, 304–307. doi: 10.1002/cjoc.20010190319
Chen, Y. G., Wang, P., Lin, Z. W., Sun, H. D., Qin, G. W., and Xie, Y. Y. (1998). Dibenzocyclooctadiene lignans from Kadsura angustifolia. Phytochemistry 48, 1059–1062. doi: 10.1016/S0031-9422(97)00996-5
Chen, Y. G., Wu, Z. C., Lv, Y. P., Gui, S. H., Wen, J., Liao, X. R., et al. (2003). Triterpenoids from Schisandra henryi with cytotoxic effect on Leukemia and Hela cells in vitro. Arch. Pharm. Res. 26, 912–916. doi: 10.1007/BF02980199
de Siqueira, K. A., Brissow, E. R., Santos dos, J. L., White, J. F., Santos, F. R., Almeida, et al. (2017). Endophytism and bioactivity of endophytic fungi isolated from Combretum lanceolatum Pohl ex Eichler. Symbiosis 71, 211–222. doi: 10.1007/s13199-016-0427-6
Ding, C. H., Du, X. W., Xu, Y., Xu, X. M., Mou, J. C., Yu, D., et al. (2014). Screening for differentially expressed genes in endophytic fungus strain 39 during co-culture with herbal extract of its host Dioscorea nipponica Makino. Curr. Microbial. 69, 517–524. doi: 10.1007/s00284-014-0615-7
Dong, J. Y., Chen, Y. G., Song, H. C., He, Y. P., Li, L., Zhong, Y. P., et al. (2007a). Hydroxylation of nigranoic acid to 6β-hydroxynigranoic acid by Caryospora carllicarpa YMF1.01026. Chin. Chem. Lett. 18, 165–167. doi: 10.1016/j.cclet.2006.12.020
Dong, J. Y., Chen, Y. G., Song, H. C., Zhu, Y. H., Zhou, Y. P., Li, L., et al. (2007b). Hydroxylation of the triterpenoid nigranoic acid by the fungus Gliocladium roseum YMF1.00133. Chem. Biodivers. 4, 112–117. doi: 10.1002/cbdv.200790015
Dong, K., Pu, J. X., Du, X., Li, X. N., and Sun, H. D. (2013). Two new guaianolide-type sesquiterpenoids from Kadsura interior. Chin. Chem. Lett. 21, 111–113. doi: 10.1016/j.cclet.2013.01.024
Estrada, C., Wcislo, W. T., and Bael, S. A. (2013). Symbiotic fungi alter plant chemistry that discourages leaf-cutting ants. New Phytol. 198, 241–251. doi: 10.1111/nph.12140
Gao, X. M., Pu, J. X., Huang, S. X., Yang, L. M., Huang, H., Xiao, W. L., et al. (2008). Lignans from Kadsura angustifolia. J. Nat. Prod. 71, 558–563. doi: 10.1021/np0705108
Geng, P., Harnly, J. M., and Chen, P. (2015). Differentiation of whole grain from refined wheat (T. aestivum) flour using lipid profile of wheat bran, germ, and endosperm with UHPLC-HRAM mass spectrometry. J. Agric. Food Chem. 63, 6189–6211. doi: 10.1021/acs.jafc.5b01599
Gniłka, R., Majchrzak, W., and Wawrzeńczyk, C. (2015). Microbial transformations of (-)-α- and (+)-β-thujone by fungi of Absidia species. Phytochem. Lett. 13, 41–46. doi: 10.1016/j.phytol.2015.05.006
He, F., Li, X. Y., Yang, G. Y., Li, X. N., Luo, X., Zou, J., et al. (2012). Nortriterpene constituents from Schisandra sphenanthera. Tetrahedron 68, 440–446. doi: 10.1016/j.tet.2011.11.026
Heinig, U., Scholz, S., and Jennewein, S. (2013). Getting to the bottom of Taxol biosynthesis by fungi. Fungal Divers. 60, 161–170. doi: 10.1007/s13225-013-0228-7
Herre, E. A., Mejia, L. C., Kyllo, D. A., Rojas, E., Maynard, Z., Butler, A., et al. (2007). Ecological implications of anti-pathogen effects of tropical fungal endophytes and mycorrhizae. Ecology 88, 550–558. doi: 10.1890/05-1606
Huang, Q., An, H. M., Song, H. C., Mao, H. Q., Shen, W. Y., and Dong, J. Y. (2015). Diversity and biotransformative potential of endophytic fungi associated with the medicinal plant Kadsura angustifolia. Res. Microbiol. 166, 45–55. doi: 10.1016/j.resmic.2014.12.004
Huang, S. X., Han, Q. B., Lei, C., Pu, J. X., Xiao, W. L., Yu, J. L., et al. (2008). Isolation and characterization of miscellaneous terpenoids of Schisandra chinensis. Tetrahedron 64, 4260–4267. doi: 10.1016/j.tet.2008.02.085
Huang, S. X., Yang, L. B., Xiao, W. L., Lei, C., Liu, J. P., Lu, Y., et al. (2007). Wuweizidilactones A-F: novel highly oxygenated nortriterpenoids with unusual skeletons isolated from Schisandra chinensis. Chemistry 13, 4816–4822. doi: 10.1002/chem.200700346
Hung, T. M., Na, M. K., Min, B. S., Ngoc, T. M., Lee, I. S., Zhang, X. F., et al. (2007). Acetylcholinesterase inhibitory effect of lignans isolated from Schizandra chinensis. Arch. Pharm. Res. 30, 685–690. doi: 10.1007/BF02977628
Jia, M., Chen, L., Xin, H. L., Zheng, C. J., Rahman, K., Han, T., et al. (2016). A friendly relationship between endophytic fungi and medicinal plants: a systematic review. Front. Microbiol. 7:906. doi: 10.3389/fmicb.2016.00906
Jiang, S., Duan, J. A., Tao, J. H., Yan, H., and Zheng, J. B. (2010). Ecological distribution and elicitor activities of endophytic fungi in Changium smyrnioides. Chin. Tradit. Herb. Drugs 1, 121–125.
Jiang, S. J., Wang, Y. H., and Chen, D. F. (2005). Sphenanlignan, a new lignan from the seeds of Schisandra sphenanthera. Chin. J. Nat. Med. 3, 78–82.
Kaul, S., Gupta, S., Ahmed, M., and Dhar, M. K. (2012). Endophytic fungi from medicinal plants: a treasure hunt for bioactive metabolites. Phytochem. Rev. 11, 487–505. doi: 10.1007/s11101-012-9260-6
Kuban, M., Öngen, G., and Bedir, E. (2010). Biotransformation of cycloastragenol by Cunninghamella blakesleeana NRRL 1369 Resulting in a novel framework. Org. Lett. 12, 4252–4255. doi: 10.1021/ol101642a
Kuban, M., Öngen, G., Khan, I. A., and Bedir, E. (2013). Microbial transformation of cycloastragenol. Phytochemistry 88, 99–104. doi: 10.1016/j.phytochem.2012.12.007
Kusari, S., Hertweck, C., and Spiteller, M. (2012). Chemical ecology of endophytic fungi: origins of secondary metabolites. Chem. Biol. 19, 792–798. doi: 10.1016/j.chembiol.2012.06.004
Kusari, S., Kosuth, J., Cellarova, E., and Spiteller, M. (2011a). Survival-strategies of endophytic Fusarium solani against indigenous camptothecin biosynthesis. Fungal Ecol. 4, 219–223. doi: 10.1016/j.funeco.2010.11.002
Kusari, S., Zühlke, S., and Spiteller, M. (2011b). Effect of artificial reconstitution of the interaction between the plant Camptotheca acuminata and the fungal endophyte Fusarium solani on camptothecin biosynthesis. J. Nat. Prod. 74, 764–775. doi: 10.1021/np1008398
Kusari, S., Zuhlke, S., Kosuth, J., Cellarova, E., and Spiteller, M. (2009). Light-independent metabolomics of endophytic Thielavia subthermophila provides insight into microbial hypericin biosynthesis. J. Nat. Prod. 72, 1825–1835. doi: 10.1021/np9002977
Larkin, M. A., Blackshields, G., Brown, N. P., Chenna, R., McGettigan, P. A., McWilliam, H., et al. (2007). Clustal W and Clustal X version 2.0. Bioinformatics 23, 2947–2948. doi: 10.1093/bioinformatics/btm404
Lei, C., Pu, J. X., and Huang, S. X. (2009). A class of 18(13⇒14)-abeo-schiartane skeleton nortriterpenoids from Schisandra propinqua var. propinqua. Tetrahedron 65, 164–170. doi: 10.1016/j.tet.2008.10.079
Li, J., Zhao, G. Z., Huang, H. Y., Qin, S., Zhu, W. Y., Zhao, L. X., et al. (2012). Isolation and characterization of culturable endophytic actinobacteria associated with Artemisia annua L. Antonie Van Leeuwenhoek 101, 515–527. doi: 10.1007/s10482-011-9661-3
Li, R. T., Han, Q. B., Zhao, A. H., and Sun, H. D. (2003). Micranoic acids A and B: two new octanortriterpenoids from Schisandra micrantha. Chem. Pharm. Bull. 51, 1174–1176. doi: 10.1248/cpb.51.1174
Li, R. T., Xiang, W., Li, S. H., Lin, Z. W., and Sun, H. D. (2004). Lancifodilactones B-E, new nortriterpenes from Schisandra lancifolia. J. Nat. Prod. 67, 94–97. doi: 10.1021/np030339+
Li, X. G., and Luo, H. M. (2002). Study on chemical constituents of the essential oil from Kadusra heteroclita (Roxb.) Craib. Chin. J. Med. Chem. 12, 89–90.
Liang, C. Q., Shi, Y. M., Luo, R. H., Li, X. Y., Gao, Z. H., Li, X. N., et al. (2012). Kadcoccitones A and B, two new 6/6/5/5-fused tetracyclic triterpenoids from Kadsura coccinea. Org. Lett. 14, 6362–6365. doi: 10.1021/ol303168y
Liu, J. S., Huang, M. F., Arnold, G. F., Arnold, E., Clardy, J., and Ayer, W. A. (1983). Schisanlactone B, a new triterpenoid from a Schisandra sp. Tetrahedron Lett. 24, 2355–2358. doi: 10.1016/S0040-4039(00)81923-1
Liu, L., Dong, Y. S., Qi, S. S., Wang, H., and Xiu, Z. L. (2010). Biotransformation of steriodal saponins in Dioscorea zingiberensis C. H. Wright to diosgenin by Trichoderma harzianum. Appl. Microbiol. Biotechnol. 85, 933–940. doi: 10.1007/s00253-009-2098-1
Ludwig-Müller, J. (2015). Plants and endophytes: equal partners in secondary metabolite production? Biotechnol. Lett. 37, 1325–1334. doi: 10.1007/s10529-015-1814-4
Luo, J. R., Ma, Q. Y., Zhao, Y. X., Yi, T. M., Li, C. S., and Zhou, J. (2009). Palaeophytochemical components from the miocene-fossil wood of Pinus griffithii. J. Chin. Chem. Soc. 56, 600–605. doi: 10.1002/jccs.200900089
Mahoney, D., Gams, W., Meyer, W., and Starink-Willemse, M. (2004). Umbelopsis dimorpha sp. nov., a link between U. vinacea and U. versiformis. Mycol. Res. 108, 107–111. doi: 10.1017/S0953756203008876
Miller, K. I., Qing, C., Sze, D. M. Y., Roufogalis, B. D., and Neilan, B. (2012). Culturable endophytes of medicinal plants and the genetic basis for their bioactivity. Microb. Ecol. 64, 431–449. doi: 10.1007/s00248-012-0044-8
Mocan, A., Schafberg, M., Cri?an, G., and Rohn, S. (2016). Determination of lignans and phenolic components of Schisandra chinensis (Turcz.) Baill using HPLC-ESI-ToF-MS and HPLC-online TEAC: contribution of individual components to overall antioxidant activity and comparison with traditional antioxidant assays. J. Funct. Foods 24, 579–594. doi: 10.1016/j.jff.2016.05.007
Ortega, A. J., López, D. C., and Maldonado, E. (1989). A tris-norsesquiterpene lactone and other sesquiterpenes from Calea crocinervosa. Phytochemistry 28, 2735–2736. doi: 10.1016/S0031-9422(00)98078-6
Petrini, O. (1991). “Fungal endophytes of tree leaves,” in Microbial Ecology of Leaves, eds J. Andrews and S. Hirano (New York, NY: Springer Verlag), 179–197. doi: 10.1007/978-1-4612-3168-4_9
Pimentel, M. R., Molina, G., Dionísio, A. P., Maróstica Junior, M. R., and Pastore, G. M. (2010). The use of endophytes to obtain bioactive compounds and their application in biotransformation process. Biotechnol. Res. Int. 2011, 1–11. doi: 10.4061/2011/576286
Poornima, B., Kumar, D. A., Siva, B., Venkanna, A., Vadaparthi, P. R., Kumar, K., et al. (2016). Advanced glycation end-products inhibitors isolated from Schisandra grandiflora. Nat. Prod. Res. 30, 493–496. doi: 10.1080/14786419.2015.1024117
Posner, E. (2009). “Wheat flour milling,” in Wheat Chemistry and Technology, 4th Edn, eds K. Khan and R. Shewry (St. Paul, MN: AACC International), 119–152. doi: 10.1094/9781891127557.005
Prinsen, P., Gutierrez, A., Faulds, C. B., and Del Rio, J. C. (2014). Comprehensive study of valuable lipophilic phytochemicals in wheat bran. J. Agric. Food Chem. 62, 1664–1673. doi: 10.1021/jf404772b
Pu, J. X., Gao, X. M., Lei, C., Xiao, W. L., Wang, R. R., Yang, L. B., et al. (2008). Three new compounds from Kadsura longipedunculata. Chem. Pharm. Bull. 56, 1143–1146. doi: 10.1248/cpb.56.1143
Qawasmeh, A., Obied, H. K., Raman, A., and Wheatley, W. (2012). Influence of fungal endophyte infection on phenolic content and antioxidant activity in grasses: interaction between Lolium perenne and different strains of Neotyphodium lolii. J. Agric. Food Chem. 60, 3381–3388. doi: 10.1021/jf204105k
Rodriguez, R. J., White, J. F. Jr., Arnold, A. E., and Redman, R. S. (2009). Fungal endophytes: diversity and functional roles. New Phytol. 182, 314–330. doi: 10.1111/j.1469-8137.2009.02773.x
Sachin, N., Manjunatha, B. L., Kumara, P. M., Ravikanth, G., Shweta, S., and Suryanarayanan, T. S. (2013). Do endophytic fungi possess pathway genes for plant secondary metabolites. Curr. Sci. 104, 178–182.
Satiraphan, M., Thai, Q. D., Sotanaphun, U., Sittisombut, C., Michel, S., and Cachet, X. (2015). A new 3,4-seco-cycloartane from the leaves of Hopea odorata Roxb. Nat. Prod. Res. 29, 1820–1827. doi: 10.1080/14786419.2015.1007975
Schulz, B., and Boyle, C. (2005). The endophytic continuum. Mycol. Res. 109, 661–686. doi: 10.1017/S095375620500273X
Shi, Y. M., Xiao, W. L., Pu, J. X., and Sun, H. D. (2015). Triterpenoids from the Schisandraceae family: an update. Nat. Prod. Rep. 32, 367–410. doi: 10.1039/C4NP00117F
Simoes, M. F., Pereira, L., Santos, C., and Lima, N. (2013). “Polyphasic identification and preservation of fungal diversity: concepts and applications,” in Management of Microbial Resources in the Environment, eds A. Malik, E. Grohmann, and M. Alves (Dordrecht: Springer), 91–117. doi: 10.1007/978-94-007-5931-2_5
Sivakumar, S., Reddy, M. L., Cowley, A. H., and Vasudevan, K. V. (2010). Synthesis and crystal structures of lanthanide 4-benzyloxy benzoates: influence of electron-withdrawing and electron-donating groups on luminescent properties. Dalton Trans. 39, 776–786. doi: 10.1039/B917256D
Smith, C., Wahab, A. T., Khan, M. S., Ahmad, M. S., Farran, D., Iqbal Choudhary, M., et al. (2015). Microbial transformation of oxandrolone with Macrophomina phaseolina and Cunninghamella blakesleeana. Steroids 102, 39–45. doi: 10.1016/j.steroids.2015.06.008
Song, Q. Y., Jiang, K., Zhao, Q. Q., Gao, K., Jin, X. J., and Yao, X. J. (2013). Eleven new highly oxygenated triterpenoids from the leaves and stems of Schisandra chinensis. Org. Biomol. Chem. 11, 1251–1258. doi: 10.1039/C2OB27115J
Sun, H. D., Qiu, S. X., Lin, L. Z., Wang, Z. Y., Lin, Z. W., Pengsuparp, T., et al. (1996). Nigranoic acid, a triterpenoid from Schisandra sphaerandra that inhibits HIV-1 reverse transcriptase. J. Nat. Prod. 59, 525–527. doi: 10.1021/np960149h
Sun, R., Song, H. C., Wang, C. R., Shen, K. Z., Xu, Y. B., Gao, Y. X., et al. (2011). Compounds from Kadsura angustifolia with anti-HIV activity. Bioorg. Med. Chem. Lett. 21, 961–965. doi: 10.1016/j.bmcl.2010.12.055
Sun, R., Song, H. C., Yang, Y. H., Yang, P., Yang, D. Y., Shen, K. Z., et al. (2013). Microbiological transformation of the triterpene nigranoic acid by the freshwater fungus Dictyosporium heptasporum. J. Asian Nat. Prod. Res. 15, 433–440. doi: 10.1080/10286020.2013.778833
Sy, L. K., and Geoffrey, D. B. (1998). A seco-cycloartane from Illicium verum. Phytochemistry 48, 1169–1171. doi: 10.1016/S0031-9422(98)00150-2
Taghavi, S., Barac, T., Greenberg, B., Borremans, B., Vangronsveld, J., and van der Lelie, D. (2005). Horizontal gene transfer to endogenous endophytic bacteria from poplar improves phytoremediation of toluene. Appl. Environ. Microbiol. 71, 8500–8505. doi: 10.1128/AEM.71.12.8500-8505.2005
Tamura, K., Peterson, D., Peterson, N., Stecher, G., Nei, M., and Kumar, S. (2011). MEGA5: molecular evolutionary genetics analysis using maximum likelihood, evolutionary distance, and maximum parsimony methods. Mol. Biol. Evol. 8, 2731–2739. doi: 10.1093/molbev/msr121
Tan, R. X., and Zou, W. X. (2001). Endophytes: a rich source of functional metabolites. Nat. Prod. Rep. 18, 448–459. doi: 10.1039/B100918O
Tian, Y., Amand, S., Buisson, D., Kunz, C., Hachette, F., Dupont, J., et al. (2014). The fungal leaf endophyte Paraconiothyrium variabile specifically metabolizes the host plant metabolome for its own benefit. Phytochemistry 108, 95–101. doi: 10.1016/j.phytochem.2014.09.021
Tudzynski, B. (1997). “Fungal phytohormones in pathogenic and mutualistic associations,” in The Mycota, eds G. C. Carroll and P. Tudzynski (Berlin: Springer Verlag), 167–184. doi: 10.1007/978-3-662-10370-8_10
Tudzynski, B., and Sharon, A. (2002). “Biosynthesis, biological role and application of fungal phytohormones,” in The Mycota, Industrial Applications, ed. H.-D. Osie cwacz (Berlin: Springer Verlag), 183–212. doi: 10.1007/978-3-662-10378-4_9
Wang, L., Qin, D., Zhang, K., Huang, Q., Liu, S., Han, M. J., et al. (2017). Metabolites from the co-culture of nigranoic acid and Umbelopsis dimorpha SWUKD3. 1410, an endophytic fungus from Kadsura angustifolia. Nat. Prod. Res. 31, 1414–1421. doi: 10.1080/14786419.2016.1255891
Wang, W., Liu, J. Z., Han, J., Xu, Z. G., Liu, R. X., Liu, P., et al. (2006). New Triterpenoids from Kadsura heteroclite and their cytotoxic activity. Planta Med. 72, 450–457. doi: 10.1055/s-2005-916263
Wang, Y. L., Liu, X. Y., and Zheng, R. Y. (2013). Four new species records of Umbelopsis (Mucoromycotina) from China. J. Mycol. 2013, 1–6. doi: 10.1155/2013/970216
Werner, C., Petrini, O., and Hesse, M. (1997). Degradation of the polyamine alkaloid aphelandrine by endophytic fungi isolated from Aphelandra tetragona. FEMS. Microbiol. Lett. 155, 147–153. doi: 10.1111/j.1574-6968.1997.tb13871.x
White, T. J., Bruns, T., Lee, S., and Taylor, J. (1990). “Amplification and direct sequencing of fungal ribosomal RNA genes for phylogenetics,” in PCR Protocols: a Guide to Methods and Application, eds M. A. Innis, D. H. Gelfand, J. J. Sninsky, and T. J. White (San Diego, CA: Academic Press Inc), 315–322.
Xia, Y. G., Yang, B. Y., and Kuang, H. X. (2014). Schisandraceae triterpenoids: a review. Phytochem. Rev. 14, 155–187. doi: 10.1007/s11101-014-9343-7
Xiao, W. L., Li, R. T., Huang, S. X., Pu, J. X., and Sun, H. D. (2008). Triterpenoids from the Schisandraceae family. Nat. Prod. Rep. 25, 871–891. doi: 10.1039/B719905H
Xiao, W. L., Wu, Y. L., Shang, S. Z., He, F., Luo, X., Yang, G. Y., et al. (2010a). Four new nortriterpenoids from Schisandra lancifolia. Helv. Chim. Acta 93, 1975–1981. doi: 10.1002/hlca.201000004
Xiao, W. L., Yang, S. Y., Yang, L. M., Yang, G. Y., Wang, R. R., Zhang, H. B., et al. (2010b). Chemical constituents from the leaves and stems of Schisandra rubriflora. J. Nat. Prod. 73, 221–225. doi: 10.1021/np900849y
Xue, Y. B., Yang, J. H., Li, X. N., Du, X., Pu, J. X., Xiao, W. L., et al. (2011). Henrischinins A-C: three new triterpenoids from Schisandra henryi. Org. Lett. 13, 1564–1567. doi: 10.1021/ol200283y
Yang, X. W., Li, S. M., Wu, L., Li, Y. L., Feng, L., Shen, Y. H., et al. (2010). Abiesatrines A-J: anti-inflammatory and antitumor triterpenoids from Abies georgei Orr. Org. Biomol. Chem. 8, 2609–2616. doi: 10.1039/C001885F
Zeng, W. L., Li, W. K., Han, H., Tao, Y. Y., Li, Y., Wang, Z. T., et al. (2013). Microbial biotransformation of gentiopicroside by the endophytic fungus Penicillium crustosum 2T01Y01. Appl. Envioron. Microbiol. 80, 184–192. doi: 10.1128/AEM.02309-13
Zhang, D., Yang, Y., Castlebury, L. A., and Cerniglia, C. E. (2006). A method for the large scale isolation of high transformation efficiency fungal genomic DNA. FEMS. Microbiol. Lett. 145, 261–265. doi: 10.1111/j.1574-6968.1996.tb08587.x
Zheng, X. K., Guo, J. H., Feng, W. S., and Li, H. W. (2009). Three new sesquiterpenes from Euonymus schensianus Maxim. Chin. Chem. Lett. 20, 952–954. doi: 10.1016/j.cclet.2009.03.041
Zhou, M., Liu, Y., Song, J., Peng, X. G., Cheng, Q., Cao, H., et al. (2016). Schincalide A, a schinortriterpenoid with a tricyclo [5.2. 1.01, 6] decane-bridged system from the stems and leaves of Schisandra incarnate. Org. Lett. 18, 4558–4561. doi: 10.1016/j.cclet.2009.03.041
Zhu, Y., Soroka, D. N., and Sang, S. (2013). Structure elucidation and chemical profile of sphingolipids in wheat bran and their cytotoxic effects against human colon cancer cells. J. Agric. Food Chem. 61, 866–874. doi: 10.1021/jf3047863
Zhu, Y., Soroka, D. N., and Sang, S. (2015). Oxyphytosterols as active ingredients in wheat bran suppress human colon cancer cell growth: identification, chemical synthesis, and biological evaluation. J. Agric. Food Chem. 63, 2264–2276. doi: 10.1021/jf506361r
Keywords: Kadsura angustifolia, Umbelopsis dimorpha, highly oxygenated schitriterpenoids/schinortriterpenoids, microbial fermentation, fungus–plant interaction
Citation: Qin D, Wang L, Han M, Wang J, Song H, Yan X, Duan X and Dong J (2018) Effects of an Endophytic Fungus Umbelopsis dimorpha on the Secondary Metabolites of Host–Plant Kadsura angustifolia. Front. Microbiol. 9:2845. doi: 10.3389/fmicb.2018.02845
Received: 21 March 2018; Accepted: 05 November 2018;
Published: 22 November 2018.
Edited by:
Guido Lingua, Università degli Studi del Piemonte Orientale, ItalyReviewed by:
Anja Schüffler, Institut für Biotechnologie und Wirkstoff-Forschung (IBWF), GermanyValeria Prigione, Università degli Studi di Torino, Italy
Copyright © 2018 Qin, Wang, Han, Wang, Song, Yan, Duan and Dong. This is an open-access article distributed under the terms of the Creative Commons Attribution License (CC BY). The use, distribution or reproduction in other forums is permitted, provided the original author(s) and the copyright owner(s) are credited and that the original publication in this journal is cited, in accordance with accepted academic practice. No use, distribution or reproduction is permitted which does not comply with these terms.
*Correspondence: Jinyan Dong, ZG9uanlhYUBzd3UuZWR1LmNu
†These authors have contributed equally to this work and should be considered as co-first authors