- 1Laboratory of Plant Improvement and Valorization of Agroresources, National School of Engineering of Sfax, Sfax, Tunisia
- 2Institute for Insect Biotechnology, Justus-Liebig-University Giessen, Giessen, Germany
- 3Faculty of Sciences of Gabès, University of Gabès, Gabès, Tunisia
- 4Institute for Pharmaceutical Biology, University of Bonn, Bonn, Germany
- 5Department of Bioresources of the Fraunhofer Institute for Molecular Biology and Applied Ecology, Giessen, Germany
Arid regions show relatively fewer species in comparison to better-watered biomes, but the competition for the few nutrients is very distinct. Here, in total 373 bacterial strains were isolated from rhizospheric soils obtained from three different sampling sites in Tunisia. Their potential for the production of antimicrobial compounds was evaluated. Bacterial strains, showing antibacterial activity against pathogenic bacteria, were isolated from all three sites, one strain from the Bou-Hedma national park, 15 strains from Chott-Djerid, and 13 strains from Matmata, respectively. The dominant genus was Bacillus, with 27 out of 29 strains. Most interestingly, 93% of the isolates showed activity against Gram-positive and Gram-negative test bacteria. Strain Bacillus sp. M21, harboring high inhibitory potential, even against clinical isolates of Gram-negative bacteria, was analyzed in detail to enable purification and identification of the bioactive compound responsible for its bioactivity. Subsequent HPLC-MS and NMR analyses resulted in the identification of 1-acetyl-β-carboline as active component. Furthermore, fungicides of the bacillomycin and fengycin group, which in addition show antibiotic effects, were identified. This work highlights the high potential of the arid-adapted strains for the biosynthesis of specialized metabolites and suggest further investigation of extreme environments, since they constitute a promising bioresource of biologically active compounds.
Introduction
The discovery of antibiotics to treat infectious diseases has revolutionized the field of medicine in the mid-twentieth century (Wohlleben et al., 2016). However, due to the misuse of antibiotics or extensive use for clinical and veterinary purposes, many of the relevant pathogens became resistant to antibiotic therapy (Ventola, 2015). These pathogens have accumulated a large number of resistance elements by mutational adaptations, acquisition of genetic material, or alteration of gene expression. This resulted in (i) modification of the antimicrobial target, (ii) a decrease in drug-uptake, (iii) activation/increase of efflux mechanisms to extrude the harmful molecule, or (iv) global changes in important metabolic pathways via modulation of regulatory networks (Munita and Arias, 2016).
The genes encoding for the respective resistance mechanism can be found both, within the genome and on plasmids; thereby, greatly limiting the therapeutic options (Wright, 2012). The number of resistant bacterial isolates increases at an alarming rate (Frieri et al., 2017), threatening treatment options of modern medicine. Hence, there is an immense need for the discovery and development of novel antibiotics with resistance-breaking properties to effectively target the multi drug resistant (MDR) pathogen bacteria that cause life-threatening infections (Morens and Fauci, 2013).
Medicinal drugs based on natural products obtained from microorganisms are playing the most important role in the treatment of bacterial infections and appear to be still the most promising source of future antibiotics (Kumar and Kumar, 2016). This became clear, since the standard research approach performed in the recent decades, from gene via target using high-throughput screening to generate a lead, delivered no innovative antibiotics. Chemical compound libraries, optimized for oral bioavailability, have non-suitable physicochemical properties, especially for passing through Gram-negative cell membranes. On the other hand, natural products, optimized for bacterial activity, but not for human application can be associated with toxicity and low in vivo activity. A drawback in natural product research is that it seems like the low-hanging fruits are already harvested. Often, known compounds had been re-isolated. Combined with the fact that the economic value of a new antibiotic can be close to zero, thereby facing development costs of around 1.000 Mio€, since for innovative novel resistance breaking antibiotics, only small margins can be expected. An innovative novel antibiotic will receive the status of a reserve antibiotic, which will result in relatively low sales figures. Therefore, companies and research groups left the field: Today only 50 groups worldwide are active in antibiotic research with a total of ∼500 people (The Boston Consulting Group, 2017).
To increase the chance of success for bioprospecting projects, which aim to identify novel lead structures for antibiotic development, these must be based on a good rational. In nature, there will be still many different potential sources to discover such leads. Rhizospheric soil in general, with its enormous biological diversity, remains a most important target for screening projects; since there is a universal dissemination of antibiotics among rhizospheric microorganisms. The latter seem to shape the microbiome of the specific biological niche and using specialized metabolites of interest for communication and antagonism (Raaijmakers and Mazzola, 2012; Ghanmi et al., 2016). Many pharmaceutically important antibiotics have been identified in the past from this bioresource, e.g., vancomycin produced by Streptomyces orientalis isolated from a soil sample from Borneo (Griffith, 1981), kanamycin produced by a soil bacterium Streptomyces kanamyceticus (Umezawa et al., 1957), and erythromycin first isolated in 1952 from the soil bacterium Saccharopolyspora erythraea (Staunton and Wilkinson, 1997). Bacterial genera reported so far as a bioresource with a high chance to detect compounds of interest are Actinomycetes (Hotam et al., 2013; Tiwari and Gupta, 2013), Bacilli (Sumi et al., 2015), and Pseudomonads (Mukherjee et al., 2014; de Oliveira et al., 2016). In the present project so far unexplored arid sampling sites of Southern Tunisia were investigated, since the arid environment results in high competition between organisms. Several strains with antimicrobial properties were isolated and characterized. From one isolated Bacillus strain, an antimicrobial compound was isolated and further bioactive natural products were identified by LC/MS.
Materials and Methods
Sampling Sites
Samples were collected from different arid areas located in South Tunisia (Figure 1). Three rhizospheric soil samples, of herbaceous vegetation, were collected aseptically from Matmata (33°54′86′′ N, 9°96′13′′ E), the national park of Bou-Hedma (34°47′45′′ N, 9°48′21′′ E) and an arid shallow aquifer in Chott-Djerid (33°94′16′′ N, 8°44′52′′ E). Matmata has an arid climate with hot, dry summers and a short, highly variable humid period in winter with mean annual precipitation < 150 mm (Dearing et al., 1996). Bou-Hedma national park has a low arid climate with an approximate mean annual rainfall of 180 mm, a mean annual temperature of 17.2°C, and minimum and maximum monthly mean temperatures of 3.8°C in December and 36.2°C in July, respectively (Le Houérou, 2001). The Chott-Djerid is a flat area, with a mean altitude of 15 m. The mean annual rainfall for the area is around 100 mm (Richards and Vita-Finzi, 1982). The texture of the three soil samples was sandy to sandy-loamy.
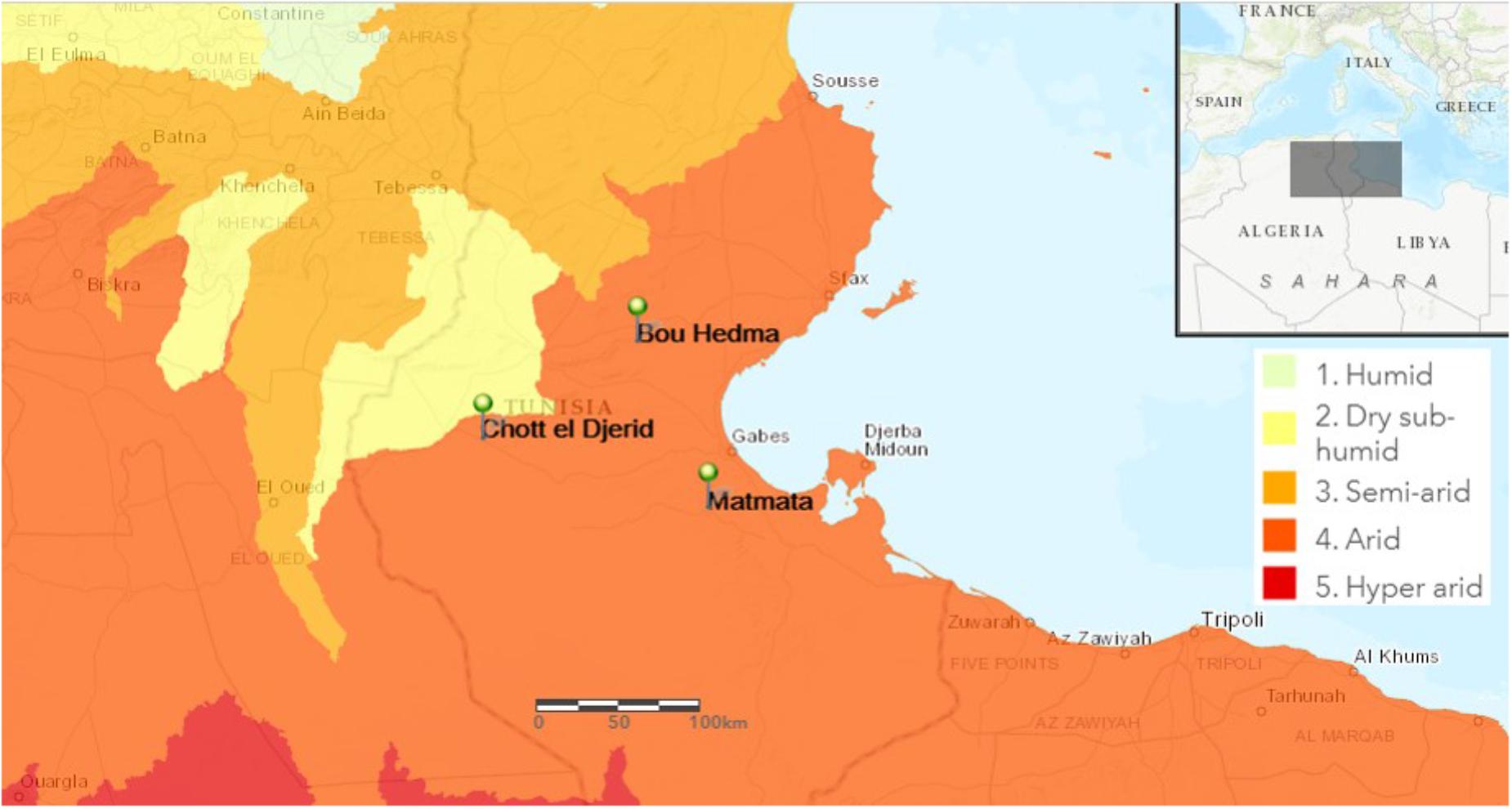
FIGURE 1. Map of Tunisia showing the location of the sampling sites. Bou-Hedma national park (Sidibouzid), Chott-Djerid (Tozeur), and Matmata (Gabès).
Soil Sampling and Isolation of Bacterial Strains
Each soil collection was made from 10 to 15 cm depth below ground surface. Samples were placed in sterile flasks and transported immediately to the laboratory, where they were air-dried for 2 h at 45°C and sieved prior use. For isolation purpose 1 g of the dried samples was dissolved in 10 mL of sterile distilled water. Soil samples were shaken vigorously for 2 min and the suspensions were treated by heat for 10 min at 80°C, except the soil suspension of Chott-Djerid. A serial dilution in sterile physiological salt solution (0.9% NaCl) up to 10−4 was prepared and an aliquot of 0.1 mL was spread over Luria-Bertani (LB) medium agar plates. Plates were incubated at 37°C for 24 h. After incubation, colonies were isolated, recorded and subjected to antimicrobial activity screening. Purified strains that inhibited the growth of at least one of the test microorganisms, were selected, cryo-conserved in 20% v/v glycerol stocks, and stored at −20°C. The code used for the isolates is (B) for Bou-Hedma national park, (C) for Chott-Djerid, and (M) for Matmata.
Growth Conditions
The isolated bacterial strains were cultivated in LB broth, which contains 10 g peptone, 5 g yeast extract and 10 g NaCl per L. The pH was adjusted to 7 with 0.01M HCl and 0.01M NaOH. Incubation was performed at 37°C and agitation of 200 rpm. The cultures were inoculated into broth medium with 1% (v/v) inoculum.
Bacterial Strains
Gram-positive test bacteria used: The reference strain Staphylococcus aureus ATCC 29213, and three clinical isolates, i.e., S. aureus, S. epidermidis, and S. saprophyticus. The last three bacterial strains were kindly provided by the Tunisian Sahloul hospital at Sousse. Gram-negative test bacteria: Two clinical isolates of Salmonella typhimurium and six Escherichia coli strains (one clinical isolate E. coli MA, three reference strains E. coli ATCC 25922, ATCC 35218 and KL 16; two in vitro mutants E. coli KL 16.2a and E. coli KL 16.2b, derived from the reference strain E. coli KL 16 by selection at twofold MIC of ciprofloxacin. These strains carry point mutations in the gyrA and parC genes (Ser83Leu in GyrAand Ser80Ile in ParC, respectively). E. coli MA, carry three mutations, two in gyrA (Ser83Leu and Asp87Asn) and one in parC (Ser80Ile). The mutant strains were recovered from patients with urinary tract infection (Bachoual et al., 1998); and a clinical E. coli isolate from the strain collection of the Tunisian Sahloul hospital at Sousse.
Antimicrobial Activity
Primary Screening
The antibacterial activity of pure isolates was determined by spot assay on Mueller Hinton (MH) agar. In brief, the test bacteria, i.e., E. coli ATCC 25922 and S. aureus ATCC 29213 were plated on agar plates before isolated soil bacteria were spotted on top. Plates were incubated for 24 h at 37°C. After incubation, bacterial spots showing an inhibition zone were selected for a secondary screening, which was performed in triplicate by disk agar diffusion on MH agar plates against several test organisms. Cell free supernatant (50 μL) was used to saturate a sterilized Whatman filter paper disc (6 mm), allowed to dry at room temperature and placed onto agar plates inoculated with 107 CFU/mL of the test bacteria. Plates were incubated at 37°C for 24 h. Antimicrobial activity was evaluated by measuring the diameter of the inhibition zone around each paper disc. All tests were performed in triplicate. The average results are presented in Supplementary Table 1.
Screening of Large-Scale Extract
Strain Bacillus M21a was fermented as described at point 2.9. The organic phase of this fermentation was obtained by liquid/liquid extraction between fermentation broth and ethyl acetate. The resulting organic phase was subjected to FLASH chromatography (BUCHI Reveleris X2, column silica 40 g). The collected fractions were tested for their antibacterial activity on agar plates and on 96 well plates. For the 96 well plate assay, wells were loaded with respective amounts of 200, 100, and 50 μg of extract. The antibiotic ampicillin (final concentration of 20, 10, and 5 μg per well) and DMSO (10, 5, and 2.5 μL per well) were used as positive and negative control against the test bacteria, respectively. Volume per well was 200 μL. After loading the samples, the 96 well plate was measured at 600 nm to determine the absorbance (time T0). At T0, the OD600 was 0.1. After incubation at 30°C for 24 h, the absorbance was measured again (time T1). The growth inhibition of the test bacteria (in %) was calculated by using the formula: % inhibition = (ODT1 − ODT0)/[AV (− control)] ∗ 100. Wells with values ≤ 30% were considered to have an antibacterial effect.
Identification of the Producer Strains
The isolates were preliminary characterized based on colony morphology and cell characteristics. Subsequently, identification by 16S rDNA sequencing was performed. Therefore, the 16S rDNA of the isolates was amplified by PCR using universal primers pA (5′AGAGTTTGATCCTGGCTCAG 3′) and pH (5′AAGGAGGTGATCCAGCCCCA 3’). Amplification was done according to the following profile: an initial denaturation step at 94°C for 10 min, followed by 30 amplification cycles of 94°C for 45 s, 56°C for 45 s, and 72°C for 2 min, and a final extension step of 72°C for 4 min. The PCR product was subjected to agarose gel electrophoresis at 80V for 30 min. The DNA fragment in the agar was visualized by ultraviolet fluorescence after ethidium bromide staining, then excised and purified using a kit (Wizard® SV Gel and PCR Clean Up System, Promega).
The resulting fragments were cloned into pGEM-T vector (Promega, Madison, WI, United States), introduced into chemical competent E. coli XL-1 Blue cells by transformation. The plasmids were isolated using the PureYieldMiniprep kit (Promega, Madison, WI, United States) and the inserts were sequenced from both sides using T7and SP6 primers (GATC BioTech AG Company, Konstanz, Germany). Vector fragments in the sequencing result were removed using VecScreen-Blast and the full 16S rDNA were assembled. The 16S rDNA sequences were blasted with the available 16S rRNA gene sequences contained in the GenBank database1.
Gram Staining and Mobility Test of Bioactive Bacterial Isolates
A smear of bacterial isolate was applied onto a clean glass slide and heated gently over a flame. The fixed bacteria were covered with a thin film of crystal violet for 1 min and washed gently under slow running water. Gram’s iodine solution was flooded over the glass slide for 1 min and washed with water. Ethanol (80%) was used to decolorize the sample. Counter staining was performed with fuchsine for 2 min. Glass slides were washed, air dried, and analyzed.
For the bacterial mobility test, a small drop of bacterial culture was placed in the center of a clean microscope slide, covered with a cover glass, and then examined microscopically for the motility of the bacterial cells.
Phylogenetic Analysis
Sequences of each isolate were refined using BioEdit sequence Alignment Editor (Hall, 1999), in which the sequences obtained from both primers were assembled to obtain consensus sequences. To analyze the relationships of the isolates to known bacterial species, the 29 sequences from this study and sequences of type strains, which had the closest relationship, were initially aligned using the MUSCLE Multiple alignment (Edgar, 2004). Based on the homology of 16S rDNA sequences, the evolutionary distances were computed through MEGA (version 7.0) program (Kumar et al., 2016) with p-distance using neighbor-joining method (Saitou and Nei, 1987). Gaps were treated as missing data. Further, the bootstrap values were calculated from 1000 replications to represent the evolutionary history of the bacterial isolates (Felsenstein, 1985).
Isolation of 1-acetyl-β-carboline
Two Bacillus strains, i.e., M21 (Nasfi et al., 2018) and M21a, were identified positive for the production of 1-acetyl-β-carboline. For isolation, both strains were inoculated into LB broth and incubated at 30°C under shaking condition. However, strain M21 lost its viability and therefore all downstream steps were performed using strain M21a. In order to isolate the bioactive compound(s), a single colony of Bacillus sp. M21a was subcultured in LB broth and incubated at 30°C for 24 h. The resulting culture was used to inoculate forty 5 L flasks containing 1 L LB broth each. Flasks were incubated at 30°C for 48 h with agitation at 140 rpm in presence of 20 g/L autoclaved amberlite XAD-16, a polymeric resin often used as a first step in downstream processing to separate bioactive compounds from bacterial growth media. The resin was collected by filtration and washed sequentially with 5 L distilled water to remove impurities. Thereafter, the resin was resuspended in a mixture of MeOH-acetone and maintained at 26°C for 18 h with agitation followed by filtration. The resulting filtrate was concentrated by a rotary evaporator at 40°C under vacuum to yield the crude extract. This extract was partitioned using liquid-liquid separation between MeOH 60% in water and DCM. The organic phase was bioassayed for activity by disc diffusion method against E. coli and B. megaterium and was subsequently subjected to successive chromatographic purification. Medium pressure (“Flash”) chromatography was performed on a silica gel 40 column, using an elution gradient with A: Petroleum ether and B: acetone with A:B from 100–0 to 0–100% (Reveleris Silica 40 μm, 12 g; Flow rate 40 mL/min; 400 mg – 8 g sample). Purity of the active fraction(s) was further examined using HPLC with an RP column (Xterra RP 18–5 μm). At a concentration of 5 mg/mL, a 20 μL aliquot was applied onto the RP column. The mobile phase consisted of an isocratic gradient of 60% MeOH (solvent B) in Milli-Q water (solvent A). The flow rate of the mobile phase was set at 0.8 mL/min for 45 min. Elution was monitored at a wavelength of 210 nm and fractions were collected manually. Resulting fractions were dried using rotary evaporators. Resulting precipitates were dissolved in MeOH for antibacterial activity testing. Fractions from a given retention time that showed antibacterial activity were pooled from different HPLC runs and concentrated.
Structure Elucidation of the Purified Compound
High-resolution mass analysis of the bioactive compound was performed using a MicrOTOF-QII mass spectrometer instrument (Bruker Daltonics GmbH, Germany).
1H and 13C-NMR spectra were recorded on a Bruker 600 Ascend spectrometer. Purified and lyophilized bioactive compound was dissolved in MeOH-d4. NMR spectra were referenced to residual solvent signals of methanol at δH3.35 and δC49.0. Coupling constants (J) are given in Hz. 1H and 13C-NMR spectroscopic assignment analyses were performed using correlation spectroscopy (COSY) and heteronuclear multi-bond correlation (HMBC) spectroscopy.
LC-MS/MS Data Processing – Molecular Networking
Mass data were converted to mzXML format with Bruker’s Data Analysis software and uploaded to the Global Natural Product Social (GNPS) molecular networking tool2. In GNPS Wang et al. (2016), the data were subjected to molecular networking using the online workflow at GNPS. The data were clustered with MS-Cluster with a parent mass tolerance of 0.5 Da and a MS/MS fragment ion tolerance of 0.2 Da to create consensus spectra. A network was then created where edges parameters were cosine score above 0.2 and more than three matched peaks. The network was then searched against GNPS’s spectral libraries. The network analysis was exported from GNPS and analyzed in Cytoscape (Shannon et al., 2003).
Results
Soil Bacteria Isolation and Antibacterial Activities
In total 373 bacterial strains were isolated from rhizosphere samples of pseudo-savannah vegetation from three different arid regions in Tunisia. Thereunder, 25 isolates from Bou-Hedma, 140 from Chott-Djerid and 208 from Matmata. All strains were fast growing organisms and produced single colonies after 24h incubation at 37°C. Of these strains 29 (7.8%) showed moderate to strong antibacterial activity in the first screenings against E. coli ATCC 25922 and S. aureus ATCC 29213. These active strains were analyzed for their morphology and all bacteria showed a rod shape appearance. Gram-staining revealed that except for isolate C6, all isolates were Gram-positive bacteria. Ten of the 29 appeared non-motile, while the majority showed motility by microscopic investigation. The morphological characteristics (shape, margin, color, cell shape, motility, and Gram-staining) and the motility of the 29 soil bacteria are summarized in Supplementary Table 2.
To evaluate if the initial screening results could be verified, all strains were re-tested against a panel of Gram-positive and Gram-negative test strains; thereby all strains retained their activity (Supplementary Table 1). The most active strains were derived from all three sampling sites. Most interestingly, 93% were active against Gram-negative bacteria. Against Gram-positive bacteria very prominent activities were observed. Only strain C2 showed no activity against Gram-negative strains. Indeed, strain C2 showed the narrowest spectrum of activity, inhibiting solely the Gram-positives S. aureus ATCC 29213 and S. epidermidis (Supplementary Table 1). Four strains, i.e., C5, C6, C7, and C11, all from one sampling site, showed the largest activity spectrum with inhibition zones against all tested bacteria, except the clinical E. coli isolate.
16S rDNA Sequence Analysis
To obtain an insight into the diversity and phylogeny of the isolated strains showing antibiotic activity, these isolates were identified by 16S rRNA gene sequence analysis. The sequences obtained for the strains were compared to sequences deposited in publicly accessible databases. This revealed that the strains belong to three different bacterial phyla, i.e., Firmicutes, Actinobacteria, and Proteobacteria. However, Bacillaceae represented by far the most prominent family (86%), followed by Brevibacteriaceae (2%) and Pseudomonadaceae (2%). In fact, out of the 28 soil isolates, 26 were members of the genus Bacillus, one was Brevibacterium halotolerans and one was Pseudomonas stutzeri (Figure 2 and Table 1). Hence, in this project, strains of the genus Bacillus were found to dominate the strain collection. It seemed that they represent the most isolated bacterium from rhizospheric soil samples, which is in accordance with published data, since it was reported that the genus Bacillus is very common in soil by Amin et al. (2015). However, it must be considered that their ability to form highly resistant endospores is on the one hand the key for their successful colonization of a wide variety of environmental habitats, especially dry arid biological niches. On the other hand, the isolation strategy applied clearly favors spore formers.
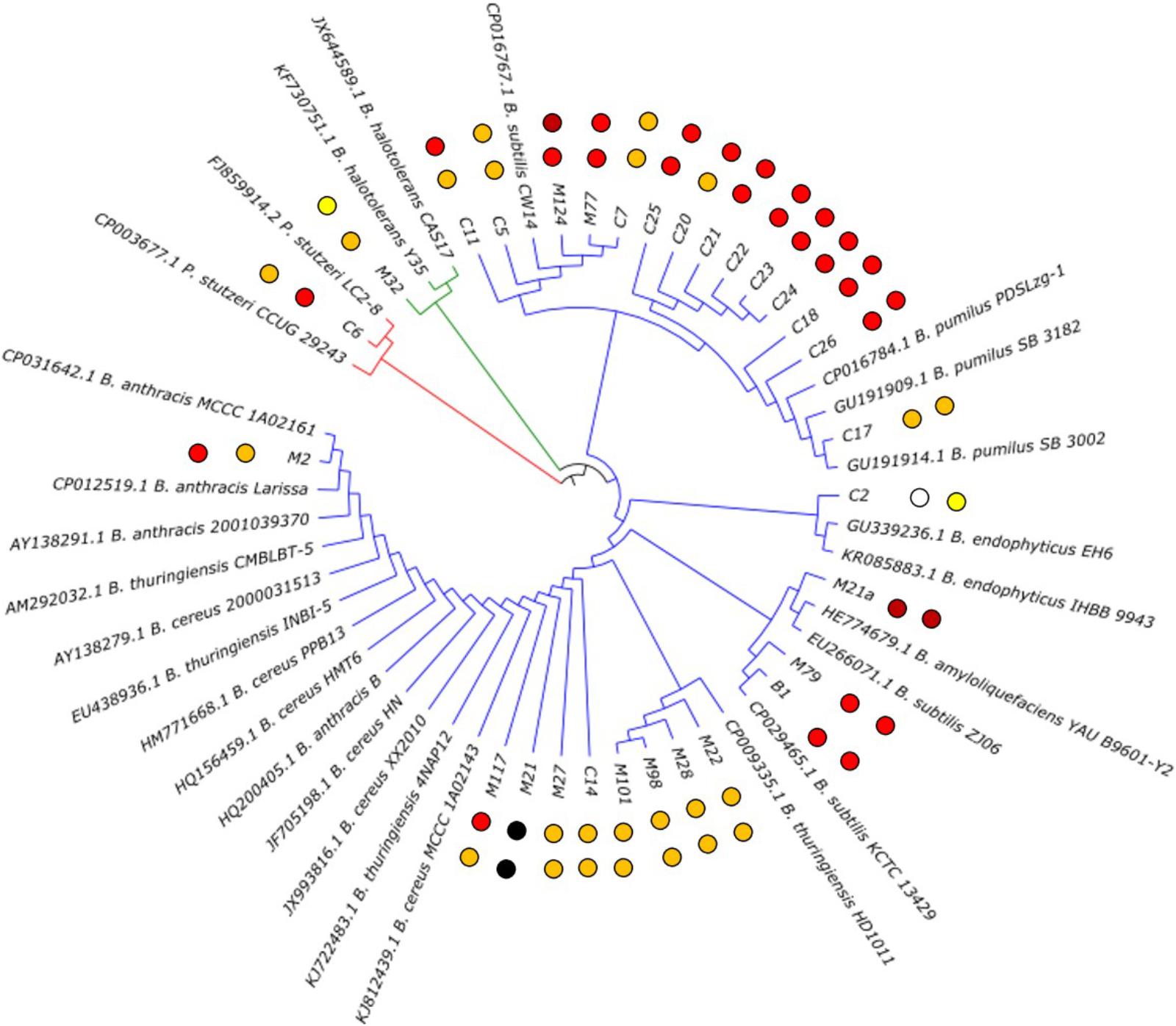
FIGURE 2. Phylogenetic tree of the isolated bioactive bacteria. The two closest homologoues based on 16 rDNA analysis for each isolate are given. The isolates from this study are carrying their identifier (compare Table 1). The observed antibacterial activity against E. coli ATCC25922 (inner dots) and S. aureus ATCC 29213 (outer dots) is indicated by dark red (strong activity), red (good activity), orange (moderate activity) and yellow (weak activity) dots. Strain M21 lost its viability during the project; therefore, the values could not be determined.
Isolation of 1-acetyl-β-carboline
Determination of the Fermentation Conditions
To get first insights into the metabolomic basis of the antibacterial activities observed, it was projected to isolate the underlying compound. First, a favorable culture medium was selected, to enable isolation of the antibacterial compound by high enough production yields. Therefore, strain M21a was cultured in 14 different media for 48 h at 30°C. Then the fermentation broth was tested by agar diffusion test against the test strains E. coli and B. megaterium. The composition of the culture media tested is given in Supplementary Table 3. All media selected promoted the growth of Bacillus sp. M21a, yielding visible biomass production. However, concerning the antibacterial activity, LB medium gave the best results against both, Gram-positive and Gram-negative test bacteria. In contrast, fermentation in M8 medium resulted in the lowest antibacterial activity observed. For all other 12 culture media, antibacterial activity was detected only against the Gram-positive test strain B. megaterium and not against the Gram-negative E. coli. Since we aimed to find the reason for the anti-Gram-negative activity, LB medium was chosen for the up-scaling.
Extraction, Purification and Structure Elucidation of 1-acetyl-β-carboline
For the isolation of the target compound, the producer strain Bacillus sp. M21a was fermented in 40 L LB medium. The organic phase of this fermentation broth was obtained by liquid-liquid extraction. The isolation of the compound was performed in a bioactivity-based manner. From the fermentation, 8 g dry weight were obtained and revealed a strong activity against E. coli (18 mm) and B. megaterium (30 mm) at a concentration of 5 mg/mL and 1 mg/mL, respectively. The bioactive crude extract was fractionated using Flash chromatography, which resulted in seven fractions. Subsequent bioactivity assay revealed the loss of activity against E. coli. However, one fraction, i.e., fraction 1, maintained activity against B. megaterium. For further fractionation, fraction 1 was separated by an analytical HPLC run. Three fractions had been collected and a 96 well plate assay was performed against the Gram-positive Arthrobacter crystallopoietes. We found that 200 μg of Fr1.1 showed a good antibacterial activity, whereby the other fractions showed no inhibition.
To finally purify the active compound, fraction Fr1.1 was purified by an additional HPLC separation. A peak with an adsorption maximum of 210 nm was observed at 12 min. This peak was manually collected and we obtained 1.8 mg of the bioactive compound.
By high-resolution LC-MS analysis, the mass was determined to be m/z 211.0907 [M + H]+ (Supplementary Figure 1). This mass fitted to a compound with the molecular formula C13H10N2O (calculated m/z for [M + H]+: 211.0871). To identify the bioactive compound unambiguously, NMR analyses were performed. The 1H-NMR spectrum revealed signals for six aromatic protons (δH7.32; 7.61; 7.71; 8.23; 8.47; and 8.32), two ortho-coupled doublets at δH7.71 and 8.23 (J = 7.9 Hz) and four multiplet protons of which two were triplets at δH7.32 and 7.61 (J = 7.9 Hz) (Supplementary Table 4 and Supplementary Figure 2). These data indicated the presence of a 1,2-disubstituted aromatic ring. In the aliphatic region, the 1H-NMR spectrum showed the presence of an aromatic bounded methyl group singlet at δH2.83. The 13C-NMR spectrum showed signals for 13 carbon atoms (Supplementary Figure 3). Among these, six aromatic methine C-atoms (δC129.8; 130.2; 113.4; 122.5; 138.54; and 120.1) and five sp2 quaternary carbons (δC143.3; 121.5; 132.3; 130.7; and 137.1) (Tab. S4). The remaining two carbons were considered as an acetyl group attached to C-9, indicated by the HMBC correlation between H-13 and C-9. The COSY (Supplementary Figure 4) and HMBC data (Supplementary Figure 5) led to reveal the structure of 1-acetyl-β-carboline (Figure 3) that was confirmed by the comparison with literature data (Lee et al., 2013).
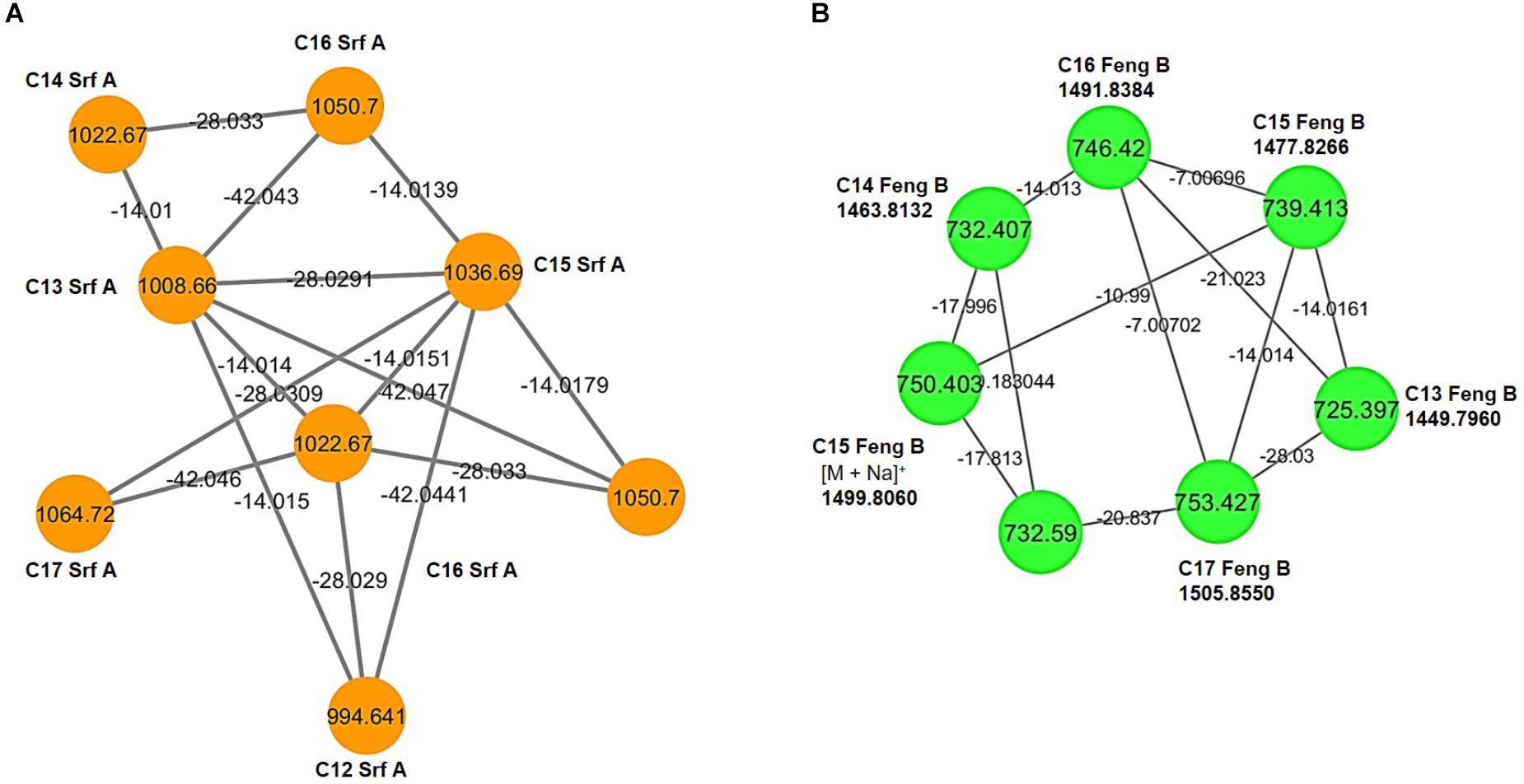
FIGURE 4. Molecular networks of Bacillus sp. M21a extracts. (A) Surfactin A homologs ([M + H]+; orange), the m/z differences of 14.01, 28.03, and 42.04 indicate molecules with different lengths of fatty acid chains within this cluster. (B) Fengycin B cluster from double charged precursor ions m/z ([M + 2H]2+; green). Nodes represent the precursor ion and bridges indicate the mass difference between individuals nodes. Labels at the nodes name the respective dereplicated molecule. Srf, surfactin; Feng, fengycin, the number indicates the length of the lipid tail.
Identification of Bioactive Lipopeptides
In addition to the here isolated carboline, derivatives of the known fungicides bacillomycin and fengycin were identified by mass-based analyses (Figure 4). These bioactive lipopeptides from the methanolic extract of a solid cultivation of Bacillus sp. M21a were detected using LC/MS in positive ion mode. In the MS spectra, three clusters of molecular mass ions belonging to the iturin, surfactin and fengycin lipopeptide families were found. These included four bacillomycins, members of the iturin family, having fatty acyl chain lengths of C13–C16, three fengycins A (from C14 to C16 and three fengycins B (C15–C17), and six surfactins (C12–C17).
Bacillomycin Identification
The protonated molecular mass ions [M + H]+ at m/z 1017.51, 1031.52, 1045.53, and 1059.55 (Supplementary Figure 3A) were assigned as putative homologs of bacillomycin D, a variant of the iturin molecule group, with C13, C14, C15, and C16 β-amino fatty acids, respectively (Cao et al., 2012). On the basis of NORINE’s database, which contains the most common non-ribosomal peptides, these compounds were identified as C13-bamD (C47H72N10O15), C14-bamD (C48H74N10O15), C15-bamD (C49H76N10O15), and C16-bamD (C50H78N10O15). The sequence of the compound C14-Bam D with an m/z of 1,031.5 was determined from series of N-terminal and proline-directed fragments (Supplementary Figure 4A). The peptide ring of this compound was cleaved both at the peptide bond between its amino fatty acid residue (β-NH2-FA) and threonine at position 7 (Thr7) as well as at the N-terminal of proline 4 (Pro4). In the first case, fragment ions at m/z 340.2, 504.2, and 617.3 were detected and corresponded to [M + H-Tyr-Asn-Pro-Glu-Ser-Thr]+, [M + H-Asn-Pro-Glu-Ser-Thr]+, and [M + H-Pro-Glu-Ser-Thr]+, respectively. In the second case, fragment ions at m/z 227.1, 314.1, 441.3, 617.3, 754.4, and 917.4 were observed and corresponded to [M + H-Ser-Thr-FA-Asn-Tyr-Asn]+, [M + H-Thr-FA-Asn-Tyr-Asn]+, [M + H-FA-Asn-Tyr-Asn]+, [M + H-Asn-Tyr-Asn]+, [M + H-Asn-Tyr]+, and [M + H-Asn]+, respectively. The MS/MS spectrum of [M + H] + ion at m/z 1045.5 presented fragments with m/z at 517.3, 618.3, 654.4, 732.4, 768.4, 829.4, 931.5, and 958.5. The corresponding amino acid sequence is given in Supplementary Figure 4B. In lower intensity Bacillus sp. M21a produced C16-Bam D and its fragmentation pattern is given in Supplementary Figure 4C.
Fengycin Identification
The LC-MS data and the molecular networking analysis showed that the ions corresponding to fengycins were [M + 2H]+ (m/z 718.392) for C14-fenA (C70H106N12O20), [M + 2H] +(m/z 725.397) for C15-fenA (C71H108N12O20), [M + 2H] + (m/z 732.407) for C16-fenA (C72H110N12O19), [M + 2H] + (m/z 739.413) for C15-fenB (C72H110N12O20), [M + 2H] + (m/z 746.42) for C16-fenB (C74H114N12O20), and [M + 2H] + (m/z 753.427) for C17-fenB (C75H116N12O20) (Supplementary Figure 3B). The fragmentation patterns of C15-FenA, C16-FenA, C15-FenB and C17-FenB are given in Supplementary Figure 5.
Surfactin Identification
The isolate M21a was also able to produce surfactins. Ion peaks at m/z 994.64, 1008.65, 1022.67, 1036.68, 1050.69, and 1064.71 were obtained and correspond to C12 to C17-srf, respectively (Supplementary Figure 3C). The MS/MS spectra of surfactin isoforms showed common fragments [M + H]+ at m/z 685.45, 671.43, and 699.46 (Supplementary Figure 6). These ions are from characteristic amino acid sequences, previously reported as Val/Leu/Asp/Val/Leu/Leu, Leu/Leu/Asp/Val/ Leu/Leu, Leu/Leu/Asp-OMe/Val/Leu/Leu and Leu/Leu/Asp/Leu/Leu/Leu, respectively (Bonmatin et al., 1995; Tang et al., 2010; Biniarz and Lukaszewicz, 2017). The MS/MS spectrum of [M + H]+ ion at m/z 1036.6869 presented fragments corresponding to losses of amino acids Leu/Leu/Asp/Val/Leu/Leu, with m/z 923.6029, 810.5212, 695.4953, 596.4275, 483.3442, and 370.2595, respectively (Supplementary Figure 4). The m/z 370.2595 corresponded to glutamic acid residue with aliphatic fatty acid chains containing 15 carbons, indicating similarities between the proposed surfactin A (C15) produced by Bacillus sp. M21a and the known surfactin (Liao et al., 2016). Additional fragment ions [M + H]+ confirmed the presence of amino acids sequence as m/z 227.1766 [Leu + Leu + H] +, m/z 328.1873 [Leu + Asp + Val + H] +, m/z 441.2721 [Leu + Asp + Val + Leu + H]+, and m/z 554.3560 [Leu + Asp + Val + Leu + Leu + H] + (Supplementary Figure 5). The molecular networking analysis showed compounds with m/z differences of 14.01, 28.03, and 42.04, suggesting molecules with different lengths of fatty acid chains within the same isoform family (Figure 4). Surfactin isoforms [M + H]+ are displayed in Table 2.
Discussion
The development of effective medicinal drugs has been revolutionizing the treatment of many human diseases. Lead structures for these drugs were detected by natural product research, since it seems that nature represents a most important resource for biologically active compounds. However, concerning antibiotics, which can be regarded as option of choice to combat bacterial infections, most of the drugs in application were already discovered several decades ago (Pidot et al., 2014). The increase of highly resistant pathogenic bacteria could put us back into a situation comparable to the pre-antibiotic era. Thus, there is an urgent need for a new age of antibiotic discovery (Khan and Khan, 2016). Ninety percent of all antibiotics, which are in clinical use today, are derived from microorganisms (Katz and Baltz, 2016) and the majority of the modern classes of antibiotics was discovered by antimicrobial activity-based screening approaches of microorganisms isolated and cultured predominantly from soil (Rolain et al., 2016). In other words, bacteria have so far been and can be also expected to remain the most promising resource for antibiotics in the future, since the majority of drugs has been developed from lead structures on the basis of bacterial natural products (Elbandary et al., 2018). Proven proliferative antibiotic-producing bacterial species include Actinomycetes, predominantly the genus Streptomyces (Hug et al., 2018), myxobacteria (Mulwa et al., 2018), cyanobacteria (Senhorinho et al., 2015), Bacilli (Sumi et al., 2015), and Pseudomonads (Gross and Loper, 2009). Even though many compounds have been already isolated from the before mentioned bacteria, it must be considered that uncultured bacteria sum up to over 99% of all species in external environments (Ling et al., 2015). Hence, there could still be a multitude of metabolites awaiting discovery, and even for well-studied producer organisms it was shown that they harbor many more biosynthetic gene clusters (BGC) for the production of specialized metabolites than natural products described from them (Pidot et al., 2014).
Only a few reported surveys exist on the bacterial diversity in arid areas, where bacterial species should be able to deal with relatively high temperatures, salt concentrations, and radiation. Hence, arid and desert habitats represent special ecosystems, and it can be expected that the chance to isolate uncommon bacteria with new metabolic capabilities is high (Trabelsi et al., 2016). In the present study, bacteria were isolated from Tunisian underexplored arid areas. Thereunder, 28 rhizospheric bacteria showed the ability to produce natural products effective against both Gram-positive and Gram-negative bacteria. The majority, 26 isolates, belonged to the Firmicutes phylum (genus Bacillus); one isolate belonged to the Actinobacteria phylum (genus Brevibacterium) and one to the Proteobacteria phylum (genus Pseudomonas). This is in accordance to the fact that Bacillus species are dominant soil bacteria. Their abilities of endospore-formation and antibiotics production have to be regarded as an advantage for the colonization of environments (Moshafi et al., 2011; Amin et al., 2015). Antimicrobial peptides of Bacilli were regarded as a promising starting point in the search for new antibacterial drugs (Sumi et al., 2015), and several known antibacterial compounds had been isolated, e.g., subtilin (Kim et al., 2012), surfactin (Jacques, 2011), and macrolactin A (Lu et al., 2008). Even though in this screening only one actinobacterial strain was isolated, they have been shown to be a resource with an unprecedented diversity of biosynthetic pathways, awaiting to be exploited for the identification of novel scaffolds. The latter could then contribute to the filling of the antibiotics development pipeline. Brevibacterium halotolerans SA87, a close homolog to the here isolated strain, has been shown to produce a variety of specialized metabolites with distinct bioactivities. It was shown that B. halotolerans SA87inhibits the growth of both Gram-positive and Gram-negative pathogens (Ahmed et al., 2015). Concerning Pseudomonas species, many specialized metabolites are reported and reviewed (Gross and Loper, 2009). This group of bacteria adapts to different stress environments and produces a wide range of bioactive metabolites with antimicrobial activity and various Pseudomonas species are able to biosynthesize compounds with a broad biological activity (Robles-Huízar et al., 2017). Pseudomonas stutzeri CMG1030, the closest homolog to strain C6, was reported to biosynthesize zafrin, an antibacterial compound, which showed strong antibacterial activity against Gram-positive as well as against Gram-negative bacteria (Uzair et al., 2008).
The strain Bacillus sp. M21a showed strong antimicrobial activity against Gram-positive and Gram-negative test strains and was chosen for further investigation and isolation of the bioactive compound. However, after fractionation the activity against E. coli was lost. This might be due degradation of the active compound(s), or to synergistic effects between different compounds, which were separated by the fractionation. The Gram-positive active compound was identified as 1-acetyl-β-carboline. The structure of this compound was unambiguously proven by NMR experiments and the data obtained were in good agreement with reported literature values (Huang et al., 2011; Lee et al., 2013). This compound was previously isolated from several types of organisms. Thereunder plants like Ailanthus malabarica and Hypodematiumsquamuloso-pilosum (Joshi et al., 1977; Zhou et al., 1998), a fungus Neosartorya pseudofischeri (Lan et al., 2016), a sponge Tedaniaignis (Dillman and Cardellina, 1991), as well as several bacteria, e.g., Steptomyces and Pseudomonas spp. (Proksa et al., 1990; Shin et al., 2010; Huang et al., 2011; Chen et al., 2013; Lee et al., 2013; Broberg et al., 2017). The β-carboline alkaloid displays various biological activities, e.g., antitumor, antimicrobial, antiviral, and antiparasitic (Cao et al., 2007; Lee et al., 2013). 1-acetyl-β-carboline showed antibacterial activity against both, MSSA and MRSA strains. The reported activities have to be considered as moderate, however, the MRSA strain tested was inhibited more efficiently by 1-acetyl-β-carboline (MICs in the range of 32–128 μg/mL) than by the β-lactams tested (ampicillin, penicillin and oxacillin, MICs in the range of 64–512 μg/mL). Further, 1-acetyl-β-carboline was described to possess synergistic effects with ampicillin and penicillin. Shin et al. (2010) found that the fractional inhibitory concentration (FIC) indices of 1-acetyl-β-carboline in combination with ampicillin and of 1-acetyl-β-carboline in combination with penicillin were in the range of 0.156–0.313 and of 0.188–0.375 in combination with 32 and 64 μg/mL of 1-acetyl-β-carboline against the MRSA strains tested. Thereby, a synergistic effect was observed. However, it did not exhibit a synergistic antibacterial effect against most of the MRSA strains tested, if combined with oxacillin. The same difference in synergistic effects has been also observed in combinations of dieckol and β-lactams (Lee et al., 2008), as well as epigallocatechin gallatein combination with β-lactams (Zhao et al., 2001). The mechanism of the observed synergism between 1-acetyl-β-carboline and β-lactams is unknown. It was speculated that a possible reason for the synergistic effect can be attributed to the fact that both molecules attack the same target of the cell wall, but on different sites due to their different structures (Shin et al., 2010). Another study also reported that 1-acetyl-β-carboline in contrast to β-lactams was active against both MRSA and MSSA (Lee et al., 2013). In light of these findings, it seems reasonable that the antibacterial activity of 1-acetyl-β-carboline is not related to PBP2, the main target site of β-lactams in the MSSA cell wall, since it was also active against MRSA cells, which have PBP2a in the cell wall. The latter has a low affinity toward β-lactams. The biosynthesis of 1-acetyl-β-carboline was described for Marinactinospora thermotolerans. In this bacterium, a BGC, consisting of the three genes mcbA, mcbB and mcbC, was identified to be responsible for the generation of the β-carboline scaffold. This scaffold is generated by a Pictet-Spengler (PS) reaction, using tryptophan (or tryptamine) and an aldehyde as substrates. The PS reaction is an important reaction for the synthesis of natural products especially in those bearing indole and isoquinoline alkaloids as scaffolds in their complex structures (Heravi et al., 2018). The novel enzyme McbB encoded in this BGC was characterized to catalyze the PS cyclization/decarboxylation/oxidation process. By heterologous expression experiments in Streptomyces lividans TK64 and Escherichia coli BL21, it was found that mcbB was sufficient in E. coli for the production of 1-acetyl-3-carboxy-β-carboline, which represents the major product. Furthermore, two minor products, i.e., 1-acetyl-β-carboline and 1-acetyl-3-hydroxy-β-carboline, are generated (Chen et al., 2013). By in vivo gene inactivation and bioinformatic tools, Chen et al. (2013) assigned the other proteins encoded in the BGC as a CoA ligase (McbA) and a decarboxylase (McbC).
It was reported before that 1-acetyl-β-carboline produced by Streptomyces sp. 04DH52 exhibits only a moderate antibacterial activity against Gram-negative bacteria with MICs ranging from 64 to 256 μg/mL (Shin et al., 2010). Also Lee et al. (2013) reported that 1-acetyl-β-carboline, isolated from Pseudomonas sp. UJ-6, showed antibacterial activity against Gram-negative bacteria with MICs ranging from 32 to 128 μg/mL. However, all the reported activities are in the moderate to low range. In our hands, Bacillus sp. M21a extracts lost E. coli activity after fractionation by Flash chromatography. This can be explained by a number of possible reasons. When we tested the organic phase of Bacillus sp. M21, many compounds were present in this mixture and might be interacting with one another to result in the inhibition observed. When the compounds were separated by using Flash chromatography, these could not interact anymore, which resulted in the loss of E. coli inhibition. It can be speculated that the compound 1-acetyl-beta-carboline was likely working with an unkown compound to yield the inhibitory effect against E. coli. Separation of the compounds abolished the synergistic effects they had in combination. Another reason why this occurred might be the instability of the active component during the Flash fractionation and dryness process, due to light or high temperature sensitivity leading to changes in the conformation of the active molecule, before testing the activity again. Another factor could have accounted for such a loss of activity; it might be a question of concentration that means that after fractionation we lost an amount of the active compound and its concentration, in one of the collected fractions, wasn’t sufficient to inhibit the test E. coli strain.
In addition to the antibacterial compound, further fungicides were identified. These natural products are involved in the plant growth promoting effects described for various Bacilli. Fengycins, composed of 10 amino acids linked to a fatty acid chain with 14–19 carbon atoms, exhibit a strong antifungal activity due to their interaction with the cell membrane, which results in an increased cell permeability, finally leading to an ultrastructural destruction of pathogenic fungi (Yang et al., 2015). Fengycins were described to possess an antibacterial activity against the Gram-positive S. epidermidis and the Gram negative E. coli (Huang et al., 2006; Roy et al., 2013). Huang et al. (2006) reported also that fengycins showed an antiviral activity against Newcastle disease virus and bursal disease virus. An antitumoral activity has also been attributed to this decalipopeptide (Sivapathasekaran et al., 2010; Yin et al., 2013; Ditmer, 2014). The inhibition of plant fungal pathogens by bacillomycin D and fengycins, was shown to be based on the induction of ROS production (Tang et al., 2014; Han et al., 2015; Gu et al., 2017). The bacillomycin D analogs have been mostly assayed for their antifungal activities (Tabbene et al., 2016), especially against phytopathogenic fungi (Moyne et al., 2001; Tanaka et al., 2014; Kim et al., 2016). Recently, bacillomycin D has showed antitumoral activities (Hajare et al., 2013; Gong et al., 2014; Qian et al., 2015; Sun et al., 2018). Surfactin possesses a number of biological activities. It is known for its antiviral, antibacterial and antitumoral properties (Meena and Kanwar, 2015; Moro et al., 2018).
Conclusion
It was shown that the strain collection of rhizospheric bacteria from Tunisian arid areas has a potential for the discovery of compounds with antibacterial and antifungal activities. Bacillomycin D, fengycin A and B, and surfactin were identified based on LC-MS data and molecular networking analysis. In addition, 1-acetyl-β-carboline was isolated as pure compound, which is known to have synergistic effects with other antibiotics. Overall, the strain collection is dominated by the Gram-positive genus Bacillus, which seems to play an important role in shaping the microbiome by the production of bioactive compounds.
Author Contributions
GK, TS, and RB designed and planned the research. ZN isolated and identified the bacterial strains. ZN and HB isolated natural products. SK guided laboratory work and analyzed the NMR data. All authors analyzed and interpreted the results and commented on the manuscript prepared by ZN and TS.
Funding
The main part of this research was supported by a bilateral grant (TUNGER-7) of the Tunisian Ministry of Higher Education and Scientific Research and the German Federal Ministry of Education and Research (BMBF, 01DH16010). ZN obtained a fellowship from the Tunisian ministry of higher education, scientific research and information and communication technologies.
Conflict of Interest Statement
The authors declare that the research was conducted in the absence of any commercial or financial relationships that could be construed as a potential conflict of interest.
Acknowledgments
We thank Alexander Bogdanov and Antonio Dávila-Céspedes for their support in NMR and Flash experiments. ZN gratefully acknowledges her fellowship from the Tunisian ministry of higher education, scientific research and information and communication technologies.
Supplementary Material
The Supplementary Material for this article can be found online at: https://www.frontiersin.org/articles/10.3389/fmicb.2018.02742/full#supplementary-material
Footnotes
References
Ahmed, H. A., Ebrahim, W., Mikhailovna, P. A., Henrich, B., and Proksch, P. (2015). Extraction and Identification of some metabolites produced by antagonistic apple plant bacteria Brevibacterium halotolerans. Int. J. Adv. Res. 3, 1208–1217.
Amin, M., Rakhisi, R., and Ahmady, A. Z. (2015). Isolation and Identification of Bacillus species from soil and evaluation of their antibacterial properties. Avicenna J. Clin. Microb. Infec. 2:e23233. doi: 10.1007/s10534-012-9606-y
Bachoual, R., Tankovic, J., and Soussy, C. J. (1998). Analysis of the mutations involved influoroquinolone resistance of in vivo and in vitro mutants of Escherichia coli. Microb. Drug. Resist. 4, 271–276. doi: 10.1089/mdr.1998.4.271
Biniarz, P., and Lukaszewicz, M. (2017). Direct quantification of lipopeptide biosurfactants in biological samples via HPLC and UPLC-MS requires sample modification with an organic solvent. Appl. Microbiol. Biotechnol. 101, 4747–4759. doi: 10.1007/s00253-017-8272-y
Bonmatin, J. M., Labbe, H., Grangemard, I., Peypoux, F., Maget-Dana, R., Ptak, M., et al. (1995). Production, isolation and characterization of [Leu4]- and [Ile4]surfactins from Bacillus subtilis. Lett. Pept. Sci. 2, 41–47. doi: 10.1007/BF00122922
Broberg, A., Bjerketorp, J., Andersson, P., Sahlberg, C., and Levenfors, J. J. (2017). Labradorins with antibacterial activity produced by Pseudomonas sp. Molecules 22:1072. doi: 10.3390/molecules22071072
Cao, R., Peng, W., Wang, Z., and Xu, A. (2007). β-Carboline alkaloids: biochemical and pharmacological functions. Curr. Med. Chem. 14, 479–500. doi: 10.2174/092986707779940998
Cao, Y., Xu, Z., Ling, N., Yuan, Y., Yang, X., Chen, L., et al. (2012). Isolation and identification of lipopeptides produced by B. subtilis SQR 9 for suppressing Fusarium wilt of cucumber. Sci. Hortic. 135, 32–39. doi: 10.1016/j.scienta.2011.12.002
Chen, Q., Ji, C., Song, Y., Huang, H., Ma, J., Tian, X., et al. (2013). Discovery of McbB, an enzyme catalyzing the β-carboline skeleton construction in the Marinacarboline biosynthetic pathway. Angew. Chem. Int. Ed. 52, 9980–9984. doi: 10.1002/anie.201303449
de Oliveira, A. G., Spago, F. R., Simionato, A. S., Navarro, M. O. P., da Silva, C. S., Barazetti, A. R., et al. (2016). Bioactive organocopper compound from Pseudomonas aeruginosa inhibits the growth of Xanthomonas citri subsp. citri. Front. Microbiol. 7:113. doi: 10.3389/fmicb.2016.00113
Dearing, J. A., Livingstone, I. P., and Zhou, L. P. (1996). A late quaternary magnetic record of tunisian loess and its climatic significance. Geophys. Res. Lett. 23, 189–192. doi: 10.1029/95GL03132
Dillman, R. L., and Cardellina, J. H. II (1991). Aromatic secondary metabolites from the sponge tedaniaignis. J. Nat. Prod. 54, 1056–1061. doi: 10.1021/np50076a021
Ditmer, E. M. (2014). Evaluation of the Anti-Proliverative Effect of the Lipopeptides Iturin A and Fengycin of Bacillus spp. and the Viral Fusionprotein PTD4-VP3 on Human Cancer and Normal Cells. Ph.D. thesis, Universidade Federal do Rio Grande do Sul, Brazil.
Edgar, R. C. (2004). “MUSCLE: multiple sequence alignment with improved accuracy and speed,” in Proceedings of the IEEE Computational Systems Bioinformatics Conference (CSB), (Stanford, CA: IEEE). doi: 10.1109/CSB.2004.1332560
Elbandary, A. A., Hessain, A. M., El-Hariri, M. D., Seida, A. A., Moussa, I. M., Mubarak, A. S., et al. (2018). Isolation of antimicrobial producing actinobacteria from soil samples. Saud. J. Biol. Sci. 25, 44–46. doi: 10.1016/j.sjbs.2017.05.003
Felsenstein, J. (1985). Confidence limits on phylogenies: an approach using the bootstrap. Evolution 39, 783–791. doi: 10.1111/j.1558-5646.1985.tb00420.x
Frieri, M., Kumar, K., and Boutin, A. (2017). Antibiotic resistance. J. Infect. Public Health 10, 369–378. doi: 10.1016/j.jiph.2016.08.007
Ghanmi, F., Carré-Mlouka, A., Vandervennet, M., Boujelben, I., Frikha, D., Ayadi, H., et al. (2016). Antagonistic interactions and production of halocin antimicrobial peptides among extremely halophilic prokaryotes isolated from the solar saltern of Sfax. Tunisia. Extremophiles 20, 363–374. doi: 10.1007/s00792-016-0827-9
Gong, Q., Zhang, C., Lu, F., Zhao, H., Bie, X., and Lu, Z. (2014). Identification of bacillomycin D from Bacillus subtilis fmbJ and its inhibition effects against Aspergillus flavus. Food Control 36, 8–14. doi: 10.1016/j.foodcont.2013.07.034
Griffith, R. S. (1981). Introduction to vancomycin. Rev. Infect. Dis. 3, 200–204. doi: 10.1093/clinids/3.Supplement_2.S200
Gross, H., and Loper, J. E. (2009). Genomics of secondary metabolite production by Pseudomonas spp. Nat. Prod. Rep. 26, 1408–1446. doi: 10.1039/b817075b
Gu, Q., Yang, Y., Yuan, Q., Shi, G., Wu, L., Lou, Z., et al. (2017). Bacillomycin D produced by Bacillus amyloliquefaciens is involved in the antagonistic interaction with the plant -pathogenic fungus Fusarium graminearum. Appl. Environ. Microbiol. 83:e01075-17. doi: 10.1128/AEM.01075-17
Hajare, S. N., Subramanian, M., Gautam, S., and Sharma, A. (2013). Induction of apoptosis in human cancer cells by a Bacillus lipopeptide bacillomycin D. Biochimie 95, 1722–1731. doi: 10.1016/j.biochi.2013.05.015
Hall, T. A. (1999). BioEdit: a user-friendly biological sequence alignment editor and analysis program for Windows 95/98/NT. Nucl. Acids. Symp. Ser. 41, 95–98.
Han, Q., Wu, F., Wang, X., Qi, H., Shi, L., Ren, A., et al. (2015). The bacterial lipopeptide iturins induce Verticillium dahliae cell death by affecting fungal signalling pathways and mediate plant defence responses involved in pathogen -associated molecular pattern -triggered immunity. Environ. Microbiol. 17, 1166–1188. doi: 10.1111/1462-2920.12538
Heravi, M., Zadsirjan, V., and Malmir, M. (2018). Application of the asymmetric pictet–spengler reaction in the total synthesis of natural products and relevant biologically active compounds. Molecules 23:943. doi: 10.3390/molecules23040943
Hotam, S. C., Bhavana, S., Anju, R. S., and Saurabh, S. (2013). Diversity and versatility of actinomycetes and its role in antibiotic production. J. Appl. Pharm. Sci. 3, S83–S94.
Huang, H., Yao, Y., He, Z., Yang, T., Ma, J., Tian, X., et al. (2011). Antimalarial β-carboline and indolactam alkaloids from Marinactinospora thermotolerans, a deep sea isolate. J. Nat. Prod. 74, 2122–2127. doi: 10.1021/np200399t
Huang, X., Lu, Z., Zhao, H., Bie, X., Lü, F., and Yang, S. (2006). Antiviral activity of antimicrobial lipopeptide from Bacillus subtilis fmbj against pseudorabies virus, porcine parvovirus, newcastle disease virus and infectious bursal disease virus in vitro. Int. J. Peptide Res. Ther. 12, 373–377. doi: 10.1007/s10989-006-9041-4
Hug, J. J., Bader, C. D., Remškar, M., Cirnski, K., and Müller, R. (2018). Concepts and methods to access novel antibiotics from actinomycetes. Antibiotics 7:44. doi: 10.3390/antibiotics7020044
Jacques, P. (2011). “Surfactin and other lipopeptides from Bacillus spp,” in Biosurfactants. Microbiology Monographs, Vol. 20, ed. G. Soberón-Chávez (Berlin: Springer).
Joshi, B. S., Kamat, V. N., and Gawad, D. H. (1977). Some β-carboline alkaloids of ailanthus malabarica DC. Heterocycles 7, 193–200. doi: 10.3987/S-1977-01-0193
Katz, L., and Baltz, R. H. (2016). Natural product discovery: past, present, and future. J. Ind. Microbiol. Biotechnol. 43, 155–176. doi: 10.1007/s10295-015-1723-5
Khan, S. N., and Khan, A. U. (2016). Breaking the spell: combating multidrug resistant ‘superbugs. Front. Microbiol. 7:174. doi: 10.3389/fmicb.2016.00174
Kim, J. D., Jeon, B. J., Han, J. W., Park, M. Y., Kang, S. A., and Kim, B. S. (2016). Evaluation of the endophytic nature of Bacillus amyloliquefaciens strain GYL4 and its efficacy in the control of anthracnose. Pest. Manag. Sci. 72, 1529–1536. doi: 10.1002/ps.4181
Kim, S. Y., Lee, N. K., Han, E. J., and Paik, H. D. (2012). Characterization of subtilin KU43 Produced by Bacillus subtilis KU43 isolated from traditional Korean fermented foods. Food Sci. Biotechnol. 21, 1433–1438. doi: 10.1007/s10068-012-0188-9
Kumar, D., and Kumar, S. (2016). Antimicrobial metabolites and antibiotics obtained from different environmental sources. Int. J. Pharm. Res. Allied Sci. 5, 85–90.
Kumar, S., Stecher, G., and Tamura, K. (2016). MEGA7: molecular evolutionary genetics analysis version 7.0 for bigger datasets. Mol. Biol. Evol. 33, 1870–1874. doi: 10.1093/molbev/msw054
Lan, W. J., Fu, S. J., Xu, M. Y., Liang, W. L., Lam, C. K., Zhong, G. H., et al. (2016). Five new cytotoxic metabolites from the marine fungus Neosartorya pseudofischeri. Mar. Drugs 14:18. doi: 10.3390/md14010018
Le Houérou, H. N. (2001). Biogeography of the arid steppeland north of the Sahara. J. Arid. Environ. 48, 103–128. doi: 10.1006/jare.2000.0679
Lee, D. S., Eom, S. H., Jeong, S. Y., Shin, H. J., Je, J. Y., Lee, E. W., et al. (2013). Anti-methicillin-resistant Staphylococcus aureus (MRSA) substance from the marine bacterium Pseudomonas sp. UJ-6. Environ. Toxicol. Pharm. 35, 171–177. doi: 10.1016/j.etap.2012.11.011
Lee, D. S., Kang, M. S., Hwang, H. J., Eom, S. H., Yang, J. Y., Lee, M. S., et al. (2008). Synergistic effect between dieckol from Ecklonia stolonifera and β-lactams against methicillin-resistant Staphylococcus aureus. Biotechnol. Bioprocess Eng. 13:765. doi: 10.1007/s12257-008-0162-9
Liao, J.-H., Chen, P.-Y., Yang, Y.-L., Kan, S.-C., Hsieh, F.-C., and Liu, Y.-C. (2016). Clarification of the antagonistic effect of the lipopeptides produced by Bacillus amyloliquefaciens BPD1 against Pyricularia oryzae via in situ MALDI-TOF IMS analysis. Molecules 21:1670. doi: 10.3390/molecules21121670
Ling, L. L., Schneider, T., Peoples, A. J., Spoering, A. L., Engels, I., Conlon, B. P., et al. (2015). A new antibiotic kills pathogens without detectable resistance. Nature 517:455. doi: 10.1038/nature14098
Lu, X. L., Xu, Q. Z., Liu, X. Y., Cao, X., Ni, K. Y., and Jiao, B. H. (2008). Marine drugs–macrolactins. Chem. Biodivers. 5, 1669–1674. doi: 10.1002/cbdv.200890155
Meena, K. R., and Kanwar, S. S. (2015). Lipopeptides as the antifungal and antibacterial agents: applications in food safety and therapeutics. BioMed Res. Int. 2015:473050. doi: 10.1155/2015/473050
Morens, D. M., and Fauci, A. S. (2013). Emerging infectious diseases: threats to human health and global stability. PLoS Pathog. 9:e1003467. doi: 10.1371/journal.ppat.1003467
Moro, G. V., Almeida, R. T. R., Napp, A. P., Porto, C., Pilau, E. J., Lüdtke, D. S., et al. (2018). Identification and ultra-high-performance liquid chromatography coupled with high-resolution mass spectrometry characterization of biosurfactants, including a new surfactin, isolated from oil-contaminated environments. Microb. Biotechnol. 11, 759–769. doi: 10.1111/1751-7915.13276
Moshafi, M. H., Forootanfar, H., Ameri, A., Shakibaie, M., Dehghan-Noudeh, G., and Razavi, M. (2011). Antimicrobial activity of Bacillus sp. strain FAS 1 isolated from soil. Pakistan J. Pharm. Sci. 24, 269–275.
Moyne, A. L., Shelby, R., Cleveland, T. E., and Tuzun, S. (2001). Bacillomycin D: an iturin with antifungal activity against Aspergillus flavus. J. Appl. Microbiol. 90, 622–629. doi: 10.1046/j.1365-2672.2001.01290.x
Mukherjee, K., Mandal, S., Mukhopadhyay, B., Mandal, N. C., and Sil, A. K. (2014). Bioactive compound from Pseudomonas synxantha inhibits the growth of Mycobacteria. Microbiol. Res. 169, 794–802. doi: 10.1016/j.micres.2013.12.005
Mulwa, L. S., Jansen, R., Praditya, D. F., Mohr, K. I., Wink, J., Steinmann, E., et al. (2018). Six heterocyclic metabolites from the Myxobacterium Labilithrix luteola. Molecules 23:542. doi: 10.3390/molecules23030542
Munita, J. M., and Arias, C. A. (2016). Mechanisms of antibiotic resistance. Microbiol. Spectrum 4:10. doi: 10.1128/microbiolspec.VMBF-0016-2015
Nasfi, Z., Poehlein, A., Harms, H., Goralski, E., Fisch, K. M., Daniel, R., et al. (2018). Draft genome sequence of Bacillus sp. Strain M21, isolated from the arid area of Matmata, Tunisia. Genome Announc. 6, e323–e318. doi: 10.1128/genomeA.00323-18
Pidot, S. J., Coyne, S., Kloss, F., and Hertweck, C. (2014). Antibiotics from neglected bacterial sources. Int. J. Med. Microbiol. 304, 14–22. doi: 10.1016/j.ijmm.2013.08.011
Proksa, B., Uhrín, D., Šurdíková, M., and Fuska, J. (1990). 1-Acetyl-β-carboline, a new metabolite of Streptomyces kasugaensis. Acta Biotechnol. 10, 337–340. doi: 10.1002/abio.370100405
Qian, S., Lu, H., Meng, P., Zhang, C., Lv, F., Bie, X., et al. (2015). Effect of inulin on efficient production and regulatory biosynthesis of bacillomycin D in Bacillus subtilis fmbJ. Bioresour. Technol. 179, 260–267. doi: 10.1016/j.biortech.2014.11.086
Raaijmakers, J. M., and Mazzola, M. (2012). Diversity and natural functions of antibiotics produced by beneficial and plant pathogenic bacteria. Annu. Rev. Phytopathol. 50, 1–22. doi: 10.1146/annurev-phyto-081211-172908
Richards, G. W., and Vita-Finzi, C. (1982). Marine deposits 35,000–25,000 years old in the Chott el Djerid, southern Tunisia. Nature 295, 54–55. doi: 10.1038/295054a0
Robles-Huízar, M., De La Torre-Zavala1, S., De La Garza-Ramos,M. A., and Galan-Wong, L. J. (2017). Antimicrobial activity of secondary metabolites obtained with different carbon sources at different stages of the Pseudomonas growth curve isolated from Fresnillo, Zacatecas, México mineral soils. Afr. J. Bacteriol. Res. 9, 30–36.
Rolain, J. M., Abat, C., Jimeno, M. T., Fournier, P. E., and Raoult, D. (2016). Do we need new antibiotics? Clin. Microbiol. Infect. 22, 408–415. doi: 10.1016/j.cmi.2016.03.012
Roy, A., Mahata, D., Paul, D., Korpole, S., Franco, O. L., and Mandal, S. M. (2013). Purification, biochemical characterization and self-assembled structure of a fengycin-like antifungal peptide from Bacillus thuringiensis strain SM1. Front. Microbiol. 4:332. doi: 10.3389/fmicb.2013.00332
Saitou, N., and Nei, M. (1987). The neighbor-joining method: a new method for reconstruction of phylogenetic trees. Mol. Biol. Evol. 4, 406–425.
Senhorinho, G. N., Ross, G. M., and Scott, J. A. (2015). Cyanobacteria and eukaryotic microalgae as potential sources of antibiotics. Phycologia 54, 271–282. doi: 10.2216/14-092.1
Shannon, P., Markiel, A., Ozier, O., Baliga, N. S., Wang, J. T., Ramage, D., et al. (2003). Cytoscape: a software environment for integrated models of biomolecular interaction networks. Genome Res. 13, 2498–2504. doi: 10.1101/gr.1239303
Shin, H. J., Lee, H. S., and Lee, D. S. (2010). The synergistic antibacterial activity of 1-acetyl-beta-carboline and beta-lactams against methicillin-resistant Staphylococcus aureus (MRSA). J. Microbiol. Biotechnol. 20, 501–505.
Sivapathasekaran, C., Das, P., Mukherjee, S., Saravanakumar, J., Mandal, M., and Sen, R. (2010). Marine bacterium derived lipopeptides: characterization and cytotoxic activity against cancer cell lines. Int. J. Peptide Res. Ther. 16, 215–222. doi: 10.1007/s10989-010-9212-1
Staunton, J., and Wilkinson, B. (1997). Biosynthesis of erythromycin and rapamycin. Chem. Rev. 97, 2611–2630. doi: 10.1021/cr9600316
Sumi, C. D., Yang, B. W., Yeo, I.-C., and Hahm, Y. T. (2015). Antimicrobial peptides of the genus Bacillus: a new era for antibiotics. Can. J. Microbiol. 61, 93–103. doi: 10.1139/cjm-2014-0613
Sun, J., Li, W., Liu, Y., Lin, F., Huang, Z., Lu, F., et al. (2018). Growth inhibition of Fusarium graminearum and reduction of deoxynivalenol production in wheat grain by bacillomycin D. J. Stored Prod. Res. 75, 21–28. doi: 10.1016/j.jspr.2017.11.002
Tabbene, O., Azaiez, S., Di Grazia, A., Karkouch, I., Ben Slimene, I., Elkahoui, S., et al. (2016). Bacillomycin D and its combination with amphotericin B: promising antifungal compounds with powerful antibiofilm activity and wound-healing potency. J. Appl. Microbiol. 120, 289–300. doi: 10.1111/jam.13030
Tanaka, K., Ishihara, A., and Nakajima, H. (2014). Isolation of anteiso-C17, iso-C17, iso-C16, and iso-C15 bacillomycin D from Bacillus amyloliquefaciens SD-32 and their antifungal activities against plant pathogens. J. Agric. Food Chem. 62, 1469–1476. doi: 10.1021/jf404531t
Tang, J.-S., Zhao, F., Gao, H., Dai, Y., Yao, Z.-H., Hong, K., et al. (2010). Characterization and online detection of surfactin isomers based on HPLC-MSn analyses and their inhibitory effects on the overproduction of nitric oxide and the release of TNF-a and IL-6 in LPS-induced macrophages. Mar. Drugs 8, 2605–2618. doi: 10.3390/md8102605
Tang, Q. Y., Bie, X. M., Lu, Z. X., Lv, F. X., Tao, Y., and Qu, X. X. (2014). Effects of fengycin from Bacillus subtilis fmbJ on apoptosis and necrosis in Rhizopus stolonifer. J. Microbiol. 52, 675–680. doi: 10.1007/s12275-014-3605-3
The Boston Consulting Group (2017). Breaking Through the Wall: a Call for Concerted Action on Antibiotics Research and Development. Berlin: Follow-up report for the German Guard Initiative.
Tiwari, K., and Gupta, R. K. (2013). Diversity and isolation of rare actinomycetes: an overview. Crit. Rev. Microbiol. 39, 256–294. doi: 10.3109/1040841X.2012.709819
Trabelsi, I., Oves, D., Manteca, A., Genilloud, O., Altalhi, A., and Nour, M. (2016). Antimicrobial activities of some actinomycetes isolated from different rhizospheric soils in Tunisia. Curr. Microbiol. 73, 220–227. doi: 10.1007/s00284-016-1053-5
Umezawa, H., Ueda, M., Maeda, K., Yagishita, K., Kondo, S., Okami, Y., et al. (1957). Production and isolation of a new antibiotic: kanamycin. J. Antibiot. 10:181.
Uzair, B., Ahmed, N., Ahmad, V. U., Mohammad, F. V., and Edwards, D. H. (2008). The isolation, purification and biological activity of a novel antibacterial compound produced by Pseudomonas stutzeri. FEMS Microbiol. Lett. 279, 243–250. doi: 10.1111/j.1574-6968.2007.01036.x
Wang, M., Carver, J. J., Phelan, V. V., Sanchez, L. M., Garg, N., Peng, Y., et al. (2016). Sharing and community curation of mass spectrometry data with GNPS. Nat. Biotechnol. 34:828. doi: 10.1038/nbt.3597
Wohlleben, W., Mast, Y., Stegmann, E., and Ziemert, N. (2016). Antibiotic drug discovery. Microb. Biotechnol. 9, 541–548. doi: 10.1111/1751-7915.12388
Wright, G. D. (2012). Antibiotics: a new hope. Chem. Biol. 19, 3–10. doi: 10.1016/j.chembiol.2011.10.019
Yang, H., Li, X., Li, X., Yu, H., and Shen, Z. (2015). Identification of lipopeptide isoforms by MALDI -TOF -MS/MS based on the simultaneous purification of iturin, fengycin, and surfactin by RP -HPLC. Anal. Bioanal. Chem. 407, 2529–2542. doi: 10.1007/s00216-015-8486-8
Yin, H., Guo, C., Wang, Y., Liu, D., Lv, Y., Lv, F., et al. (2013). Fengycin inhibits the growth of the human lung cancer cell line 95D through reactive oxygen species production and mitochondria-dependent apoptosis. Anticancer Drugs 24, 587–598. doi: 10.1097/CAD.0b013e3283611395
Zhao, W. H., Hu, Z. Q., Okubo, S., Hara, Y., and Shimamura, T. (2001). Mechanism of synergy between epigallocatechin gallate and β-lactams against methicillin-resistant Staphylococcus aureus. Antimicrob. Agents Chemother. 45, 1737–1742. doi: 10.1128/AAC.45.6.1737-1742.2001
Keywords: Bacilli, natural products, antibiotics, carboline, fungicides
Citation: Nasfi Z, Busch H, Kehraus S, Linares-Otoya L, König GM, Schäberle TF and Bachoual R (2018) Soil Bacteria Isolated From Tunisian Arid Areas Show Promising Antimicrobial Activities Against Gram-Negatives. Front. Microbiol. 9:2742. doi: 10.3389/fmicb.2018.02742
Received: 19 September 2018; Accepted: 26 October 2018;
Published: 13 November 2018.
Edited by:
José E. Barboza-Corona, Universidad de Guanajuato, MexicoReviewed by:
Norma Margarita de la Fuente Salcido, Universidad Autónoma de Coahuila, MexicoMariusz Cycoń, Medical University of Silesia, Poland
Copyright © 2018 Nasfi, Busch, Kehraus, Linares-Otoya, König, Schäberle and Bachoual. This is an open-access article distributed under the terms of the Creative Commons Attribution License (CC BY). The use, distribution or reproduction in other forums is permitted, provided the original author(s) and the copyright owner(s) are credited and that the original publication in this journal is cited, in accordance with accepted academic practice. No use, distribution or reproduction is permitted which does not comply with these terms.
*Correspondence: Till F. Schäberle, till.f.schaeberle@agrar.uni-giessen.de