- 1Sorbonne Université, CNRS, Integrative Biology of Marine Models (LBI2M), Station Biologique de Roscoff (SBR), Roscoff, France
- 2Amadéite SAS, “Pôle Biotechnologique” du Haut du Bois, Bréhan, France
- 3Génomique Métabolique, Genoscope, Institut François Jacob, CEA, CNRS, Université d’Evry, Université Paris-Saclay, Evry, France
- 4VIM, INRA, Université Paris-Saclay, Jouy-en-Josas, France
About half of seaweed biomass is composed of polysaccharides. Most of these complex polymers have a marked polyanionic character. For instance, the red algal cell wall is mainly composed of sulfated galactans, agars and carrageenans, while brown algae contain alginate and fucose-containing sulfated polysaccharides (FCSP) as cell wall polysaccharides. Some marine heterotrophic bacteria have developed abilities to grow on such macroalgal polysaccharides. This is the case of Pseudoalteromonas carrageenovora 9T (ATCC 43555T), a marine gammaproteobacterium isolated in 1955 and which was an early model organism for studying carrageenan catabolism. We present here the genomic analysis of P. carrageenovora. Its genome is composed of two chromosomes and of a large plasmid encompassing 109 protein-coding genes. P. carrageenovora possesses a diverse repertoire of carbohydrate-active enzymes (CAZymes), notably specific for the degradation of macroalgal polysaccharides (laminarin, alginate, FCSP, carrageenans). We confirm these predicted capacities by screening the growth of P. carrageenovora with a large collection of carbohydrates. Most of these CAZyme genes constitute clusters located either in the large chromosome or in the small one. Unexpectedly, all the carrageenan catabolism-related genes are found in the plasmid, suggesting that P. carrageenovora acquired its hallmark capacity for carrageenan degradation by horizontal gene transfer (HGT). Whereas P. carrageenovora is able to use lambda-carrageenan as a sole carbon source, genomic and physiological analyses demonstrate that its catabolic pathway for kappa- and iota-carrageenan is incomplete. This is due to the absence of the recently discovered 3,6-anhydro-D-galactosidase genes (GH127 and GH129 families). A genomic comparison with 52 Pseudoalteromonas strains confirms that carrageenan catabolism has been recently acquired only in a few species. Even though the loci for cellulose biosynthesis and alginate utilization are located on the chromosomes, they were also horizontally acquired. However, these HGTs occurred earlier in the evolution of the Pseudoalteromonas genus, the cellulose- and alginate-related loci being essentially present in one large, late-diverging clade (LDC). Altogether, the capacities to degrade cell wall polysaccharides from macroalgae are not ancestral in the Pseudoalteromonas genus. Such catabolism in P. carrageenovora resulted from a succession of HGTs, likely allowing an adaptation to the life on the macroalgal surface.
Introduction
Marine macroalgae are complex multicellular photosynthetic organisms, evolutionary distinct from land plants, and constitute a large primary biomass in coastal ecosystems. Polysaccharides constitute about half of the algal tissues and can be found either as carbon storage or cell wall compounds (Kloareg and Quatrano, 1988). Like plant polysaccharides, macroalgal polysaccharides can be neutral (e.g., starch, laminarin, cellulose), or polyanionic (Ficko-Blean et al., 2015). Red algae (Rhodophyta) typically contain sulfated xylans or sulfated galactans (agars or carrageenans) in their cell wall (Deniaud et al., 2003). Among them, the main three types of carrageenans include kappa-, iota-, and lambda-carrageenan, chains of disaccharidic galactans which contain one, two or three sulfate groups, respectively (Michel et al., 2006). Brown algae (Phaeophyceae) produce laminarin as storage compound and contain fucose-containing sulfated polysaccharides (FCSP) and alginate in their cell wall (Popper et al., 2011; Deniaud-Bouët et al., 2017). Alginates account for 10–45% of the dry weight of brown algal cell wall. They are anionic polymers made of alpha-(1,4)-L-guluronate and beta-(1,4)-D-mannuronate aligned in blocks (Mabeau and Kloareg, 1987; Kloareg and Quatrano, 1988; Deniaud-Bouët et al., 2014). These different types of polysaccharides represent a large choice of carbon sources for marine heterotrophic bacteria (MHB, Egan et al., 2013; Martin et al., 2014). To utilize such carbon sources, MHB produce specific carbohydrate-active enzymes (CAZymes), classified as glycoside hydrolases (GH), polysaccharide lyases (PL) or other types of modifying enzymes (Lombard et al., 2014) and sulfatases (Barbeyron et al., 2016a). Indeed, several members of the Bacteroidetes, Planctomycetes or Gammaproteobacteria, secrete sulfatases, agarases or carrageenases to degrade red macroalgal galactans or present alginolytic systems allowing the degradation of brown algal polysaccharides (Michel et al., 2006; Thomas et al., 2012; Wegner et al., 2013; Martin et al., 2014; Neumann et al., 2015). In Bacteroidetes, gene clusters are described as polysaccharide utilization loci (PUL) including CAZymes, recognition proteins, transporters and coregulated genes that act together for the detection and uptake of a specific polysaccharide (Bjursell et al., 2006). The specificity of PULs unique to Bacteroidetes is that they include a pair of susC and susD homologs. These genes, respectively, encode a TonB-dependent receptor (TBDR), localized in the outer membrane of gram-negative bacteria and known to actively transport large molecules to the periplasmic space (Noinaj et al., 2010), and a carbohydrate-binding lipoprotein (Martens et al., 2009). Similar carbohydrate utilization loci are also found in other bacterial phyla, usually including a standalone TBDR gene but without susC-like gene. Such gene clusters have been recently proposed to be also named PULs (Ficko-Blean et al., 2017; Grondin et al., 2017).
Among the Gammaproteobacteria, the Pseudoalteromonas genus represents exclusively MHB and they are usually producers of biologically active extracellular agents and active producer of biofilms (Bowman, 2007). They can secrete anti-microbial or anti-viral compounds, display anti-fouling activities or produce macroalgal polysaccharide-degrading enzymes. They are frequently associated with marine eukaryotic hosts such as fishes, mussels, sponges or macroalgae (Holmström and Kjelleberg, 1999; Martin et al., 2014). For instance, the genomic analysis of Pseudoalteromonas tunicata, isolated from the green macroalga Ulva lactuca and the tunicate Ciona intestinalis, showed its ability to attach to marine surfaces, to form biofilms and to produce bioactive compounds (Thomas et al., 2008). Pseudoalteromonas species can also degrade complex polysaccharides and such capacities can be acquired by horizontal gene transfers (HGT) as recently shown for the pectin degradation pathway in P. haloplanktis ANT/505 (Hehemann et al., 2017). Our study will focus on Pseudoalteromonas carrageenovora 9T, which was isolated among a pool of bacteria from marine waters and algae around Nova Scotia (Yaphe and Baxter, 1955) and was the first microorganism shown to produce carrageenases and carrageenan-specific sulfatases (McLean and Williamson, 1979a,b, 1981). The GH16 family kappa-carrageenase from P. carrageenovora was the first to be genetically (Barbeyron et al., 1994), biochemically (Potin et al., 1995), and structurally characterized (Michel et al., 1999, 2001). Its lambda-carrageenase was also purified and characterized and the corresponding gene cglA was cloned (Johnston and McCandless, 1973; Guibet et al., 2007), defining the GH150 family (Lombard et al., 2014). P. carrageenovora is also able to degrade fucoidin (former name for FCSP) and alginate from the brown alga Fucus vesiculosus (Yaphe and Morgan, 1959; Akagawa-Matsushita et al., 1992). Furthermore, an arylsulfatase was cloned from this gammaproteobacterium (Barbeyron et al., 1995). This protein is the first characterized member of the S4 family of sulfatases, which belong to the zinc-dependent beta-lactamase superfamily (Barbeyron et al., 2016a). All these activities likely reflect that the bacterium P. carrageenovora has a macroalgae-associated lifestyle. To further investigate to which extent this bacterium is adapted to such lifestyle, we have explored the carbohydrate metabolism in P. carrageenovora’s genome and validated the predicted activities by profiling bacterial growth on selected carbon sources. Our work has also brought new insights on the following evolutionary and ecological questions: (i) are macroalgal polysaccharide-degrading genes commonly found in Pseudoalteromonas genomes? (ii) did P. carrageenovora acquire such activities by HGT from other MHB? (iii) are there genetic traits suggesting an adaptation of P. carrageenovora to an algae-associated lifestyle?
Materials and Methods
Culture Conditions and Catabolic Profiling of P. carrageenovora 9T
For all subsequent experiments, pre-cultures of P. carrageenovora were prepared in 5 mL ZoBell medium 2216E (5 g.L-1 tryptone, 1 g.L-1 yeast extract, sea water; (ZoBell, 1941) at 20°C and under an agitation of 400 rpm, until reaching the maximum growth phase (OD600 of about 2). Bacterial growth of P. carrageenovora was screened using a carbohydrate collection as followed. Triplicated cultures were prepared in sterile 48-well plates on a medium consisting of 200 μL of a marine mineral medium (MMM) (for one liter of medium: 24.7 g NaCl, 6.3 g MgSO4.7H2O, 4.6 g MgCl2.H2O, 0.7 g KCl, 20 mg FeSO4.7H2O, 200 mg NaHCO3, 0.6 g CaCl2, 100 mg K2HPO4, a mix of 11 vitamins (5 mg pyridoxine HCl, 1 mg nicotinic acid, 1 mg thiamine HCl, 1 mg riboflavin, 1 mg D,L-pantothenic acid, 10 mg 4-aminobenzoic acid, 1 mg D-biotin, 1 mg folic acid, 1 mg cyanocobalamine, 5 mg orotic acid, 1 mg ascorbic acid), Tris-HCl 50 mM pH 8, and 2 g NH4Cl) and 800 μL of one of the 44 oligo- or poly-saccharides (Supplementary Table S1) with a final concentration of 4 g.L-1 (Thomas et al., 2011). Cells from the 5 mL pre-culture of P. carrageenovora were washed twice in the same volume of artificial seawater (for one liter of water: 24.7 g NaCl, 6.3 g MgSO4.7H2O, 4.6 g MgCl2.H2O, 0.7 g KCl) after centrifugation for 10 min at 4,000 g at room temperature. This inoculum was added to each well for a final OD600 of about 0.05 and cultures were grown at 20°C at 400 rpm. The growth of P. carrageenovora with each carbon source was measured after 1 week at OD600 using the Spark® Multimode Microplate reader (Tecan).
Genomic DNA Extraction
Material for genomic DNA extraction was prepared by transferring 1 mL of the pre-culture of P. carrageenovora in 200 mL ZoBell medium 2216E at 20°C, with shaking at 200 rpm, until reaching the maximum growth phase. The culture was then centrifuged at 3,000 g for 15 min and the resulting pellet was stored at -20°C until further processing. Genomic DNA from P. carrageenovora was extracted following the method from Barbeyron et al. (1984) with some modifications. Five to ten grams of cells were suspended in 25 mL of 50 mM Tris-HCl (pH 8)-25% sucrose. A volume of 5 mL of lysis buffer (50 mM Tris-HCl (pH 8), 5 mM EDTA, 50 mM NaCl) and 50 mg of lysozyme were then added and the resulting mix was incubated for 15 min at room temperature to form spheroplasts. After the addition of 6 mL of 100 mM EDTA (pH 8), lysis started during the incubation of 10 min on ice and finished after the addition of 1.5 mL of 2% SDS and 25 mL of lysis solution (50 mM Tris-HCl (pH 8), 100 mM EDTA, 100 mM NaCl). Proteins were hydrolyzed with 40 mg of proteinase K and the mix was incubated for 1 h at 50°C. Sodium perchlorate (1 M) was added to break DNA-proteins bonds. Nucleic acids were then purified following a phenol/chloroform extraction. The aqueous phase containing the nucleic acids was recuperated and the remaining proteins were removed by a chloroform/isoamyl alcohol 24:1 extraction. Nucleic acids were precipitated with 0.6 volume of isopropanol on a glass stick, washed in 70% EtOH, dehydrated in 99% EtOH and dried overnight. Nucleic acids were then resuspended in 20–30 mL of a solution of 10 mM Tris-HCl (pH 8) and 1 mM EDTA and kept at 4°C until further processing.
Genome Sequencing and Genome Assembly
P. carrageenovora 9T genome was sequenced using a combination of Sanger (ABI3730, Applied Biosystems) and Solexa (GAIIx, 2 × 74 paired-end reads with 300 bp insert size) reads. The 19,117,159 GAIIx-filtered sequence (estimated coverage of 300×) reads were assembled in 51 contigs (>1 kb) using Velvet (Zerbino and Birney, 2008). Contigs were ordered using an optical map (Argus system, OpGene, MD, United States) and the SpeI restriction enzyme (Latreille et al., 2007). All gaps (except three in the large chromosome) were filled using PCR sequencing. The Velvet contigs and the Sanger reads were assembled using the whole-genome shotgun assembler Phrap and the assembly was visualized with the interface Consed (Ewing et al., 2005). The final assembly encompassing two chromosomes and a plasmid was validated using the optical map.
Genome Annotation
Automatic functional annotation of all genes was done through the MicroScope platform (Vallenet et al., 2017). CAZymes were specifically identified by homology (at least 30% sequence identity on 80% sequence length) with characterized enzymes selected in each family of the CAZY database (Lombard et al., 2014)1. Annotations were then cross-validated using the BlastP alignments against the UniProtKB/SwissProt database (The UniProt Consortium, 2014) and Pfam domain predictions (Punta et al., 2012)2. Each sequence was assigned to a CAZyme family and, when it was possible, to an EC number. Sulfatase genes were assigned to their corresponding SulfAtlas (sub)family (Barbeyron et al., 2016a)3. Manual annotation of key genes was done using the MicroScope platform and the ngKLAST software (Nguyen and Lavenier, 2009).
Phylogeny of 16S rRNA Gene and Other Selected Genes
The phylogenetic position of P. carrageenovora 9T, was determined among 87 other Pseudoalteromonas strains (52 strains with a publicly available genome and 35 taxonomically characterized strains) and selected representative sequences of other genera of the Alteromonadales order. The 16S rRNA sequences from 409 species of Alteromonadales were aligned using MAFFT v.7 with the L-INS-i algorithm (Katoh and Standley, 2013). The multiple sequence alignment was visualized using the Jalview software v.11.0 (Clamp et al., 2004) and non-aligned regions were removed. A total of 1,423 positions were used for the phylogeny. Phylogeny was made using MEGA v.5.1 (Tamura et al., 2011). The evolutionary history was inferred using Neighbor-Joining method (Saitou and Nei, 1987) and the evolutionary distances were computed using the Kimura-two-parameters as evolutive model (Kimura, 1980). The gaps-containing sites were treated using the Pairwise-deletion option. The reliability of the trees was tested by bootstrap analysis using 1,000 resamplings of the dataset (Felsenstein, 2009).
Data Availability
Annotated genome sequences were deposited in EMBL, accession number GCA_900239935.
Results
General Carbohydrate Degrading and Biosynthesis Capacities of P. carrageenovora
The genome of P. carrageenovora is composed of a larger chromosome of 3,620 kb and 3,183 coding DNA sequences (CDS, chromosome I), a smaller chromosome of 820 kb and 708 CDS (chromosome II), and a plasmid of 143 kb and 109 CDS. Each replicon has an average GC content of 39.55, 39.13, and 37.74%, respectively (Table 1). The genome encodes several CAZymes, including 47 GH and 5 PL that may degrade endogenous and exogenous carbohydrates, and 31 glycosyltransferases (GT) for carbohydrate biosynthesis, which correspond to 22 GH families, 4 PL families and 13 GT families, respectively (Supplementary Table S2). The genome also encodes 10 sulfatases, 9 belonging to four S1 subfamilies and one to the S4 family, and they may participate in the degradation of sulfated carbohydrates (Barbeyron et al., 2016a). The CAZyme repertoire includes functions of the carbohydrate metabolism regularly found in Bacteria such as the ability to synthesize and recycle its own peptidoglycan and its own glycogen. However, most CAZymes and sulfatases display signal peptides and are predicted to be periplasmic, membrane-anchored or secreted, suggesting that these enzymes target external carbohydrate substrates. Some of the CAZyme and sulfatase genes are located close to putative TBDR (oligosaccharide import into the periplasm), major facility superfamily (MFS)-transporter or sodium/solute symporters (SSS, cytoplasm uptake of monosaccharide or small sugars), and transcriptional regulators, and thus are part of PULs. Eleven of the 58 TBDR genes found in the genome belong to nine PULs likely specific for macroalgal polysaccharides for instance, alginate, the brown algal storage polysaccharide laminarin, or various carrageenans (Supplementary Table S3).
To validate these activities, growth of P. carrageenovora was monitored on a marine mineral minimum medium (MMM) containing one of 44 carbohydrate sources (Supplementary Table S1). They include mono- and di-saccharides, as well as polysaccharides that can either be found in algal cell wall or storage (e.g., agar, porphyran, several types of carrageenans, alginate, laminarin), in vascular plants (e.g., cellulose, xylan, xyloglucan) or in animal tissues (e.g., chondroitin sulfate A and C, hyaluronic acid). P. carrageenovora could grow on two of the four monosaccharides tested, three of the four disaccharides and on 11 of the other 36 polysaccharides. The bacterium was able to grow on storage polysaccharides from algae such as starch (growth on amylopectin) and brown algal laminarin as well as on cell wall polysaccharides from brown algae such as alginate and fucoidan (FCSP from Pelvetia caniculata and Ascophyllum nodosum), and from red algae such as lambda-carrageenan (Table 2). The bacterium was not able to grow on polysaccharides unique to land plants or animals, suggesting a specificity for macroalgal degradation.
We screened the genome for the genes needed to use each carbon source. In most cases, the utilization of a given carbon source could be explained by the presence of all the necessary genes (Table 2 and Supplementary Table S4). In the cases of the two brown algal FCSPs, the bacterium grew but the genome contains no GH107 fucoidanase gene (Colin et al., 2006). However, the knowledge on FCSP biodegradation is currently very limited (Deniaud-Bouët et al., 2017) and one can expect that novel, uncharacterized CAZyme families are involved in this catabolism. In other cases, genes homologous to characterized CAZymes were present in the genome but the bacterium did not grow on the corresponding substrate. In the case of amylose, this substrate is not soluble in water as its helical structure is tightly packed, making it not as accessible to the bacterium as it is under the amylopectin form. Despite the presence of a potentially secreted cellulase in the genome (GH5_2-CBM5 family, PCAR9_b0611), the bacterium did not grow on crystalline cellulose from plants. However, the GH5 family is highly polyspecific (Aspeborg et al., 2012) and this GH5 enzyme most likely acts on other substrates such as hemicellulose. Despite the presence of three GH16 beta-glucanases, there was also no growth on mixed-linked glucan (lichenan, beta-1,3-1,4-glucan). Still, P. carrageenovora is able to use laminarin (beta-1,3-glucan), suggesting that these GH16 enzymes are strict laminarinases. Other explanations for such discrepancies between growth and genomic content may be that the bacterium is not able to detect or uptake these polysaccharides, or that genes corresponding to polysaccharide degradation are not expressed in the MMM conditions.
Carrageenan Catabolism in P. carrageenovora
Carrageenan-Specific Genes Are Located on the Plasmid
CAZymes and sulfatases from P. carrageenovora’s plasmid are almost exclusively involved in the catabolism of carrageenans and represent large proportions of the CDS content, with 11% GH and 7.34% sulfatases (Table 1). Indeed, the genes of the characterized kappa-carrageenase PcCgkA (Michel et al., 2001) and lambda-carrageenase PcCglA (Guibet et al., 2007) are located on the plasmid (PCAR9_p0048 and PCAR9_p0052, respectively). There are also plasmid-encoded proteins homologous to carrageenan-specific enzymes characterized in other marine gammaproteobacteria [PCAR9_p0019 and PCAR9_p0029: 61 and 76% sequence identity with the exo-beta-carrageenase (GH42-like) and the endo-beta-carrageenase (GH16) from Paraglaciecola hydrolytica S66, (Schultz-Johansen et al., 2018); PCAR9_p0028: 95% with the GH82 iota-carrageenase from Pseudoalteromonas sp. An33 (Martin et al., 2016)] or from the flavobacterium Z. galactactanivorans Dsij (Ficko-Blean et al., 2017): two iota-carrageenan G4S-sulfatases (PCAR9_p0023 and PCAR9_p0034, 53 and 40% with ZgCgsA, respectively), 3,6-anhydro-D-galactose dehydrogenase (PCAR9_p0040, 48% with ZgDauA), 3,6-anhydro-D-galactonate cycloisomerase (PCAR9_p0041, 40% with ZgDauB), 2-keto-3-deoxy-D-galactonate kinase (PCAR9_p0042, 32% with ZgDauC) and 2-keto-3-deoxy-D-galactonate aldolase (PCAR9_p0042, 39% with ZgDauD). All these genes are located in a large PUL encompassing 33 genes (PUL 7, Figure 1 and Supplementary Table S5). This PUL also contains a third S1 sulfatase (PCAR9_p0022, S1_NC) which likely desulfates carrageenan although its regioselectivity is uncertain, three TBDR genes (PCAR9_p0026, PCAR9_p0031, PCAR9_p0046) and an MFS-transporter (PCAR9_p0037) which are potentially responsible for the uptake of oligo-carrageenans and carrageenan-derived monosaccharides.
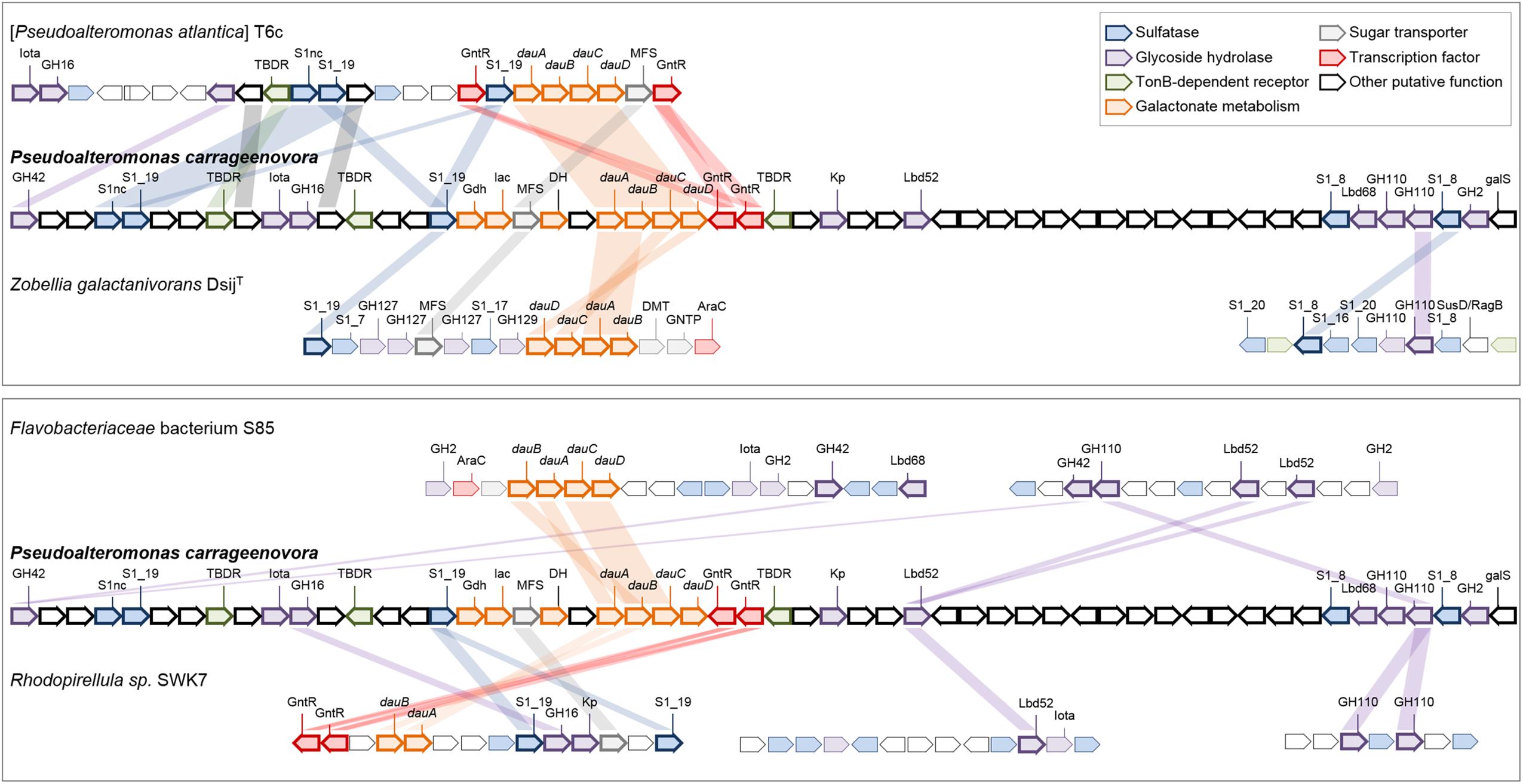
FIGURE 1. Genes of PUL 7 involved in the catabolism of carrageenan in the genome of P. carrageenovora 9T and orthologous genes from other MHB taxa. Each arrow indicates a separate gene. Orthologous genes in the genome of [Pseudoalteromonas atlantica] T6c (gene locus ID: Patl_0879 to Patl_0901), the Planctomycetes Rhodopirellula sp. SWK7 (gene locus ID: ANOQ_1350023 to ANOQ_1350036 on the left, ANOQ_120161 to ANOQ_120173 in the middle, and ANOQ_790111 to ANOQ_790117 on the right) and the Bacteroidetes Zobellia galactanivorans DsijT (gene locus ID: ZGAL_3145 to ZGAL_3159 on the left and ZGAL_2194 to ZGAL_2203 on the right) and Flavobacteriaceae bacterium S85 (gene locus ID: AFPK_670040 to AFPK_670057 on the left and AFPK_440002 to AFPK_440015 on the right) are depicted by hues colored according to their putative function (further details in Supplementary Table S8). When available, the family of the gene is indicated above the arrow. Kp, Kappa-carrageenase; Iota, iota-carrageenase; Gdh, Galactonate dehydratase; lac, putative sugar lactone lactonase; DH, short-chain dehydrogenase/reductase; dauA, 3,6-anhydro-D-galactose dehydrogenase; dauB, 3,6-anhydro-D-galactonate cycloisomerase; dauC, 2-keto-3-deoxy-D-galactonate kinase; dauD, 2-keto-3-deoxy-D-galactonate aldolase; GntR, Galactonate operon transcriptional repressor; Lbd52 and Lbd68, Pre-lambda-carrageenases; galS, HTH-type transcriptional regulator. The bacterium strain [Pseudoalteromonas atlantica] T6c probably belongs to the genus Paraglaciecola, has shown by a 100% sequence similarity with the hit of accession number CP000388 using Blast search on EZBioCloud.
Altogether, P. carrageenovora is well equipped for the degradation of kappa family carrageenans (with its kappa-, iota-, and beta-carrageenases and some carrageenan sulfatases) and for the import of these degradation products (Figure 2). The presence of the dauABCD genes suggests that this bacterium has the capacity to convert released 3,6-anhydro-D-galactose moieties into pyruvate and glyceraldehyde-3-P (Ficko-Blean et al., 2017). The bacterium is also able to use D-galactose utilization as the Leloir pathway (Leloir, 1951) is complete on chromosome I (aldose-1-epimerase, PCAR9_a31484; galactokinase, PCAR9_a31471; galactose-1-phosphate uridylyltransferase, PCAR9_a31470, and UDP-galactose-4-epimerase, PCAR9_a31469), suggesting that this catabolic route is ancestral to the acquisition of the plasmid. Interestingly, PUL 7 also includes two genes potentially involved in the De Ley-Doudoroff pathway (D-galactonate dehydratase, PCAR9_p0035; sugar lactone lactonase PCAR9_p0036). Two neighboring genes from chromosome I (D-galactose dehydrogenase, PCAR9_a31477; 2-dehydro-3-deoxygalactonokinase, PCAR9_a31476) might complete this alternative route for the D-galactose utilization (De Ley and Doudoroff, 1957). However, P. carrageenovora seems to lack 3,6-anhydro-D-galactosidases. These enzymes are found in the GH127 and GH129 families and are essential for releasing 3,6-anhydro-D-galactoses from oligo-carrageenans (Ficko-Blean et al., 2017). Surprisingly, GH127 and GH129 genes are found neither on the plasmid nor on the chromosomes, suggesting that P. carrageenovora is unable to release 3,6-anhydro-D-galactose from carrageenan degradation products and thus unable to use kappa-family carrageenans alone (Figure 2).
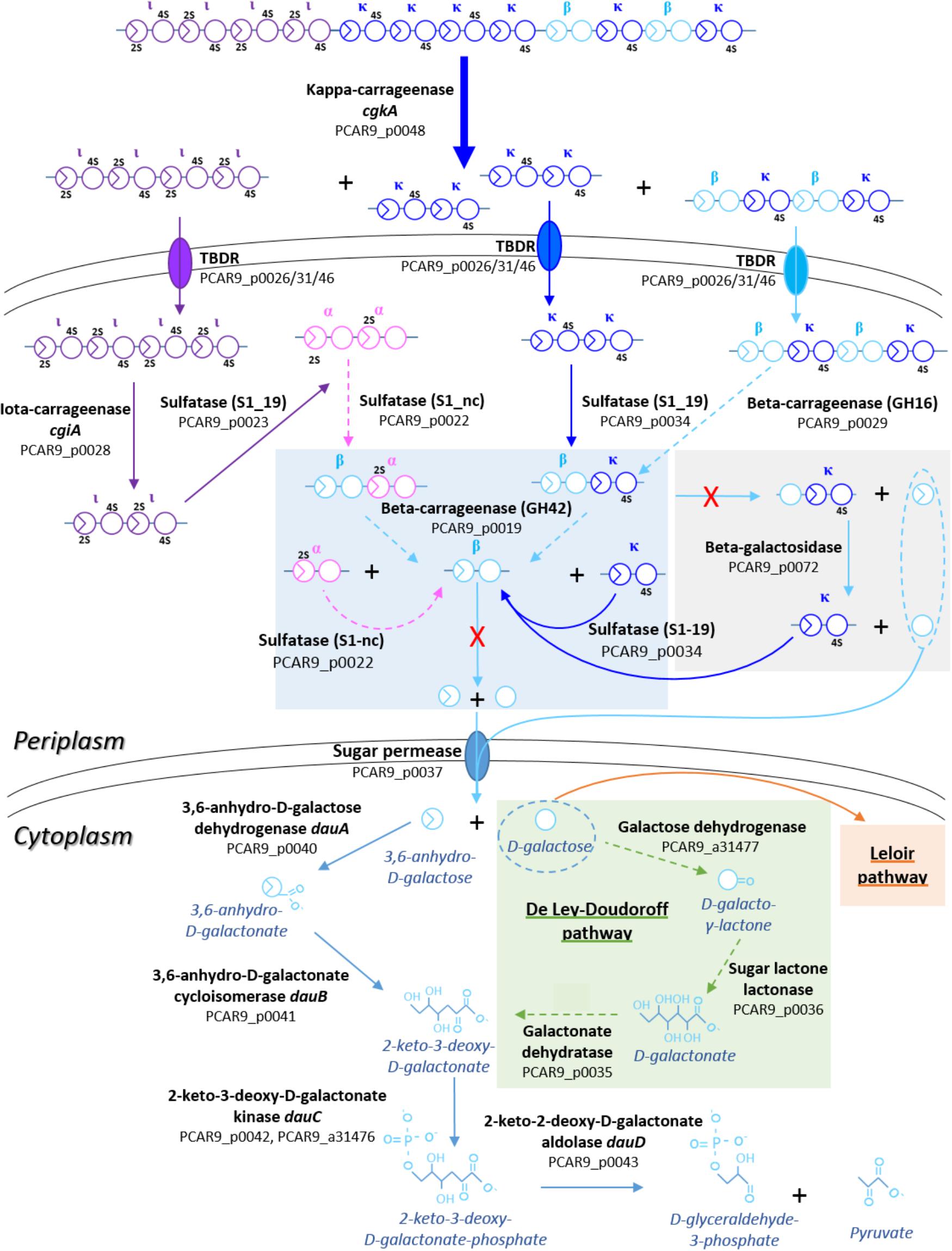
FIGURE 2. Theoretical representation of degradation pathways of kappa family carrageenans by enzymes encoded in the carrageenolytic plasmid of P. carrageenovora. Arrows in bold indicate enzymatic activities experimentally demonstrated, simple arrows indicate activities predicted by sequence homology with enzymes characterized in other organisms; dashed arrows indicate putative activities. Genes associated with enzymatic activities are indicated in brackets, in bold if experimentally checked or followed by a question mark if hypothetical. Steps specific to each carrageenan are colored in purple for iota-/alpha- and in blue for kappa-/iota-carrageenan. A red cross indicates the missing GH127/129 in the pathway.
Two other PULs are located on the plasmid. PUL 8 (PCAR9_p0067-PCAR9_p0073) includes a second GH150 lambda-carrageenase, surrounded by a GH2 beta-galactosidase, two GH110 enzymes and two S1_8 sulfatases (Figure 1 and Supplementary Table S5). Interestingly, the GH110 family currently contains 1,3-alpha-galactosidases from gut Bacteroides which catalyze the removal of alpha-1,3-linked galactose residues of blood group B antigens (Liu et al., 2007). This suggests that P. carrageenovora GH110 enzymes are also 1,3-alpha-galactosidases, but most likely acting on oligo-lambda-carrageenans released by GH150 lambda-carrageenases (which cleave beta-1,4 linkages). The PUL 8 GH2 beta-galactosidase likely acts in synergy with the GH110 enzymes to completely hydrolyze oligo-lambda-carrageenans into D-galactose, similarly, to the interplay between GH127/GH129 and GH2 which alternatively cleave alpha-1,3 and beta-1,4 linkages in kappa family oligo-carrageenans (Ficko-Blean et al., 2017). Finally, the third PUL (PUL 9, PCAR9_p0107-PCAR9_p0113) includes a family S1_8 sulfatase, two family S1_15 sulfatases, a galactokinase, a TBDR and a SSS transporter genes. The presence of an S1_8 sulfatase (also found in PUL 8) and of a galactokinase suggests that PUL 9 is involved in carrageenan catabolism, although it is less certain than in the cases of PULs 7 and 8.
Physiological Analyses of Carrageenan Utilization
In parallel to the genomic analyses, growth experiments were undertaken using two types of medium: (i) a ZoBell medium gelified with agar, kappa- or iota-carrageenans (Figures 3A–C), and (ii) a liquid MMM complemented by D-galactose, 3,6-anhydro-D-galactose or a collection of carrageenans (Figure 3D). Growth experiments on gelified rich medium confirmed the ability of P. carrageenovora to hydrolyze kappa- and iota- carrageenans. Its capacity to degrade kappa- and lambda-carrageenans is well known in the literature (Yaphe and Baxter, 1955; Michel et al., 2001; Guibet et al., 2007), but it is the first time that P. carrageenovora is shown to be an iota-carrageenolytic bacterium. This suggests that the GH82 enzyme PCAR9_p0028 is indeed an expressed and active iota-carrageenase. In MMM, bacterial growth was observed on D-galactose as well as on 3,6-anhydro-D-galactose, consistently with the presence of the Leloir pathway and of the dauABCD genes in the genome. However, P. carrageenovora was unable to grow with kappa family carrageenans (i.e., kappa-, iota-, and beta-carrageenans) as sole carbon sources, confirming that these catabolic pathways are incomplete, most likely due to the absence of GH127 and GH129 genes in the genome. In contrast, P. carrageenovora grew well with lambda-carrageenan, confirming that this bacterium possesses all the enzymes necessary for using this sulfated galactan which has the particularity to lack 3,6-anhydro-D-galactose in its structure. It also validates that lambda-carrageenan catabolic pathway is separate from the iota-/kappa-carrageenan one.
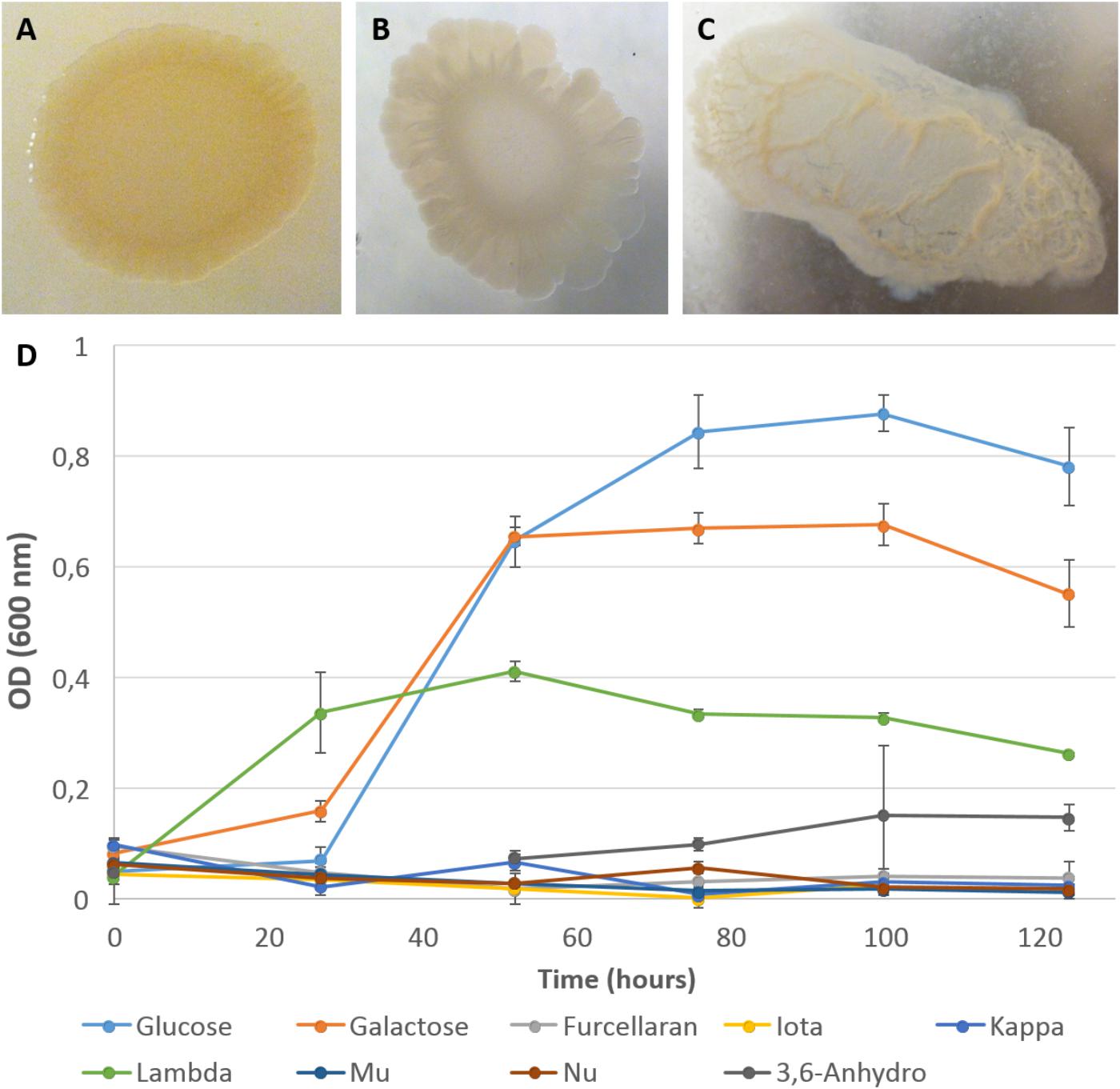
FIGURE 3. Growth of P. carrageenovora on a selection of galactans. Colonies of P. carrageenovora on ZoBell medium 2216E gelose plates containing 1.5% agar (A), 2% iota-carrageenan (B), or 1% kappa-carrageenan (C), 13 days after inoculation. Colonies on iota- and kappa-carrageenan plates showed a depression and liquefaction in the respective media. Kinetic of P. carrageenovora growth was monitored by measuring optical density (OD600) on a minimum mineral medium with either one of the nine carbohydrate sources tested. Curves indicate the mean optical density measured for the biological triplicates and bars indicate the standard deviation (D). Glucose, D-glucose; Galactose, D-galactose; Iota, iota-carrageenan; Kappa, kappa-carrageenan; Lambda, lambda-carrageenan; Mu, mu-carrageenan; Nu, nu-carrageenan; 3,6-Anhydro, 3,6-anhydro-D-galactose.
Conservation of the Carrageenan Catabolisms in the Pseudoalteromonas Genus
To determine whether the carrageenolytic capacities of P. carrageenovora are common to the genus Pseudoalteromonas, we searched for homologs from PULs 7 and 8 in 52 Pseudoalteromonas genomes. Only six genomes belonging to the same late-diverging clade (LDC) in the 16S rRNA gene-based phylogeny presented the key genes of PUL 7, suggesting a recent acquisition of this PUL in these strains (Supplementary Figure S1 and Supplementary Tables S6, S7). The GH82 iota-carrageenase gene is absent in three of these genomes. Additionally, the GH150 lambda-carrageenase and GH110 genes (PUL 8) are absent from all Pseudoalteromonas genomes, suggesting their recent acquisition following that of the plasmid in P. carrageenovora. To determine the potential origin of PUL 7 and 8 genes, we searched for homologs in genomes of macroalgae-associated bacteria (Figure 1 and Supplementary Table S8). The carrageenan PUL organization in [Pseudoalteromonas atlantica] T6c (which is in fact a Paraglaciecola strain based on 16S rRNA gene phylogeny) was the most similar to that of PUL 7. The synteny of the four dauABCD genes seems to be kept between MHB within a same class (Gammaproteobacteria and Flavobacteriia). However, as in PUL 7, the dauABCD genes of Flavobacteriaceae bacterium S85 are in the vicinity of a GH82 iota-carrageenase gene while this is not the case in Z. galactanivorans DsijT, suggesting that the genome of these flavobacteria were subjected to different gene rearrangements. The genome of the Planctomycetes Rhodopirellula sp. SWK7 contains some homologs from PUL 7 that show a different arrangement. For instance, only two of the four dau genes (dauAB) are present, suggesting gene loss in the corresponding PUL. These comparative genomics of P. carrageenovora and other MHB indicated that homologs from PUL 7 were arranged most differently in species belonging to different bacterial classes. Regarding PUL 8, we have identified a similar PUL in the marine Flavobacteriaceae bacterium S85, which also contains two GH150 lambda-carrageenases next to GH2 and GH110 genes. Another PUL containing also a GH150 lambda-carrageenase and GH110 genes is found in Rhodopirellula sp. SWK7 (Figure 1). This conservation of GH110 genes alongside lambda-carrageenase genes strengthen the hypothesis that these GH110 enzymes are 1,3-alpha-galactosidases specific for oligo-lambda-carrageenans. Interestingly, the PUL 23 of Z. galactanivorans Dsij (Barbeyron et al., 2016b) includes GH110 and S1_8 sulfatases highly similar to those of the lambda-carrageenan-specific PUL from P. carrageenovora and Flavobacteriaceae bacterium sp. S85. Although its genome is lacking GH150 gene, Z. galactanivorans Dsij is able to use lambda-carrageenan as a sole carbon source. This suggests that GH110s and S1_8 sulfatases from Z. galactanivorans Dsij are most likely specific for lambda-carrageenan and act in synergy with a lambda-carrageenase belonging to a novel, unidentified GH family.
The Chromosomes Contain Carbohydrate-Related Genes Linked to the Adaptation to an Algae-Associated Ecological Niche
CAZymes and sulfatases are distributed differently between the chromosomes. Chromosome I contains enzymes involved in the catabolism of polysaccharides with 0.79% of the total number of CDS being GH, 0.76% CDS being PL and 0.06% CDS being sulfatases, and in the biosynthesis of polysaccharides with 0.76% CDS being GT. Chromosome II contains 1.42% CDS are GH and 0.85% CDS are GT but no PL nor sulfatases (Table 1 and Supplementary Table S2). These CAZymes and sulfatases include functions conserved in Bacteria (e.g., peptidoglycan or glycogen recycling) and functions rather specific to the genus Pseudoalteromonas while others may result from HGT events. CAZymes and sulfatases of the chromosomes are mostly involved in algal polysaccharide degradation and life in the biofilm.
Alginate Catabolism
Algal polysaccharide catabolism is mostly localized on chromosome I. It encodes five secreted alginate lyases belonging to four polysaccharide lyase families (families PL6, PL7, PL17, and PL18). Three of these alginate lyase genes belong to a 14-gene cluster resembling the alginate utilization system characterized in Z. galactanivorans Dsij (Thomas et al., 2012). This cluster is named here PUL 1 (Figure 4 and Supplementary Table S3). There are two potential endo-alginate lyases from PL6 family (PCAR9_a20870, PCAR9_a20878) and one exo-alginate lyase from PL17 family (PCAR9_ a20871). The three genes contain a cleaved signal peptide, predicting export of the protein in the periplasm or the extracellular medium. PUL 1 contains other gene-encoding enzymes predicted to be involved in alginate degradation: KgdF (PCAR9_a20872) which catalyzes the conversion of 4,5-unsaturated monouronates to 4-deoxy-L-erythro-5-hexoseulose acid (DEH, Hobbs et al., 2016); a sugar oxidoreductase (PCAR9_a20869) and a carbohydrate kinase (gene KdgK, PCAR9_a20874) whose homologs in Z. galactanivorans Dsij have been shown to convert DEH to 2-keto-3-deoxy-D-gluconate, and then to 2-keto-3-deoxy-6-phosphogluconate (KDPG) as final product (Thomas et al., 2012). KDPG can be further assimilated in the Entner-Doudoroff pathway (Preiss and Ashwell, 1962a,b). PUL 1 also encodes sugar transporters (an MFS transporter, PCAR9_a20873, and two TBDR, PCAR9_a20879, PCAR9_a20880) and a regulation factor gene (PCAR9_a20881). The two other alginate lyase genes are distant from PUL 1: a potential exo-alginate lyase belonging to the PL7_5 family (PCAR9_a20415) and predicted to be localized in the outer membrane as a lipoprotein and a PL18 family alginate lyase (PCAR9_a31210) appended to a carbohydrate-binding module of family 16. Therefore, P. carrageenovora’s genome contains all necessary genes involved in alginate catabolism and this was confirmed by bacterial growth on alginate as the sole carbon source (Table 2). Comparative genomics of PUL 1 indicate that the gene synteny is conserved in 19 Pseudoalteromonas genomes, belonging to the previously mentioned LDC in the phylogenetic tree (Supplementary Figure S1 and Supplementary Table S6). Comparison of PUL 1 to the genome of MHB from different lineages, namely [P. atlantica] T6c, Zobellia galactanivorans DsijT, and Flavobacteriaceae bacterium S85 (Figure 4), indicated the presence of homologs encoding for either one or two of the polysaccharide lyases (PL6 family, gene alyA6, and PL17_2 family, gene alyA3), some transporting protein (MFS, TBDR) and some of the degradation proteins (KdgF and KdgK) in these genomes. The other two alginate lyases (PL7_5 family, PCAR9_a20415, and PL18 family, PCAR9_a31210) are localized elsewhere on chromosome I. Homologs of the PL7_5 and PL18 alginate lyases are found in 12 of the 19 genomes containing PUL 1. Other genomes contain either one or both genes and belong to the LDC in the phylogeny (Supplementary Figure S1 and Supplementary Tables S6, S7). Homologs of PCAR9_a20415 (PL7_5) are also found in [P. atlantica] T6c, Z. galactanivorans DsijT (gene alyA5) and Flavobacteriaceae bacterium S85 while no homologs of PCAR9_a31210 (PL18) were found in these 3 species.
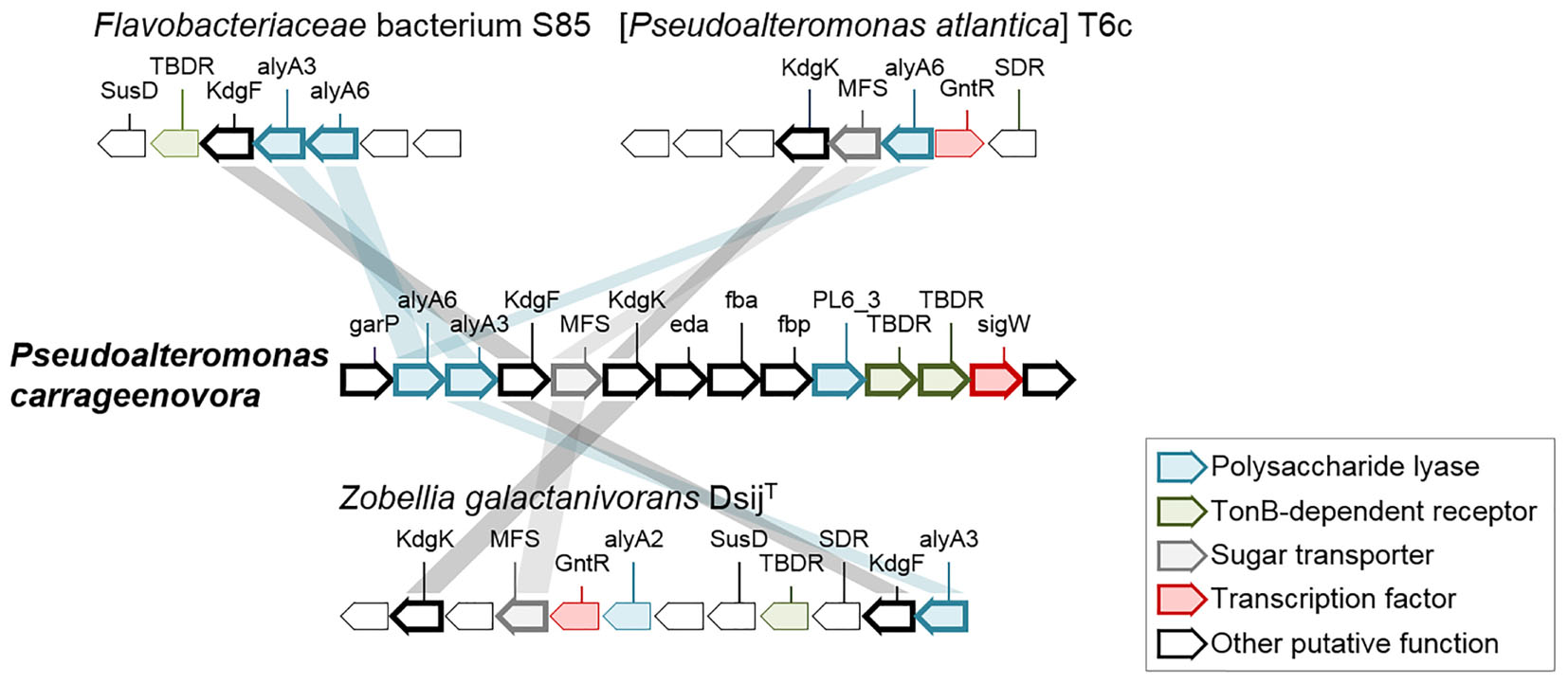
FIGURE 4. Genes of PUL 1 comprising enzymes involved in the catabolism of alginate in the genome of P. carrageenovora. Each arrow indicates a separate gene. Orthologous genes in the genome of [Pseudoalteromonas atlantica] T6c (gene locus ID: Patl_3654 to Patl_3661), the Bacteroidetes Zobellia galactanivorans DsijT (gene locus ID: ZGAL_2613 to ZGAL_2624) and Flavobacteriaceac bacterium S85 (gene locus ID: AFPK_720026 to AFPK_720032) are depicted by hues colored according to their putative function. When available, the family of the gene is indicated above the arrow. PL6, PL7, PL17_2, polysaccharide lyases from family 6, family 7, family 17_2; garR, 2-hydroxy-3-oxopropionate reductase; KdgF, Pectin degradation protein; KdgK, 2-dehydro-3-deoxygluconokinase; eda, KHG/KDPG aldolase; fba, Fructose-biphosphate aldolase; fbp, Fructose-1,6-biphosphatase class 1 2; MFS, Major Facilitator Superfamily; sigW, GntR, Sigma/transcriptional factor; SDR, short-chain dehydrogenase/reductase.
Laminarin Catabolism
Beta-1,3-glucans include storage glucans such as laminarin in brown algae (Michel et al., 2010) and chrysolaminarin in diatoms (Myklestad, 1989), cell wall polymers of fungi and oomycetes (Aronson et al., 1967; Bartnicki-Garcia, 1968) and of brown algae (Raimundo et al., 2017). Both chromosomes of P. carrageenovora show putative activities on beta-1,3-glucans. Chromosome II contains a gene cluster (PUL 5) which includes a putative TBDR (PCAR9_b0364) and a GH16 endo-1,3-beta-glucanase (PCAR9_b0365) which could be active on very different types of biologically unrelated beta-1,3-glucans. Notably, PUL 5 is conserved in 47 of the 52 Pseudoalteromonas genomes suggesting an ancestral character of the genus. Chromosome I encodes two other GH16 endo-1,3-beta-glucanases (PCAR9_a21197 and PCAR9_a30350). Orthologs of these GH16 enzymes are only found in 2 species (P. hodoensis H7T and P. sp. SM9913), suggesting recent horizontal acquisitions (Supplementary Figure S1 and Supplementary Tables S3, S6). Growth experiments have confirmed that this laminarin utilization system is functional (Table 2).
Starch Catabolism
Chromosome I contains a large gene cluster (PCAR9_a21164-PCAR9_a21179, PUL 2) predicted to be responsible for importing and utilizing extracellular starch, the storage polysaccharide of green and red algae (Supplementary Table S3). These predictions are consistent with the ability of P. carrageenovora to grow with starch as the sole carbon source (Table 2). Noticeably, PUL 2 is conserved in the 52 analyzed Pseudoalteromonas genomes.
Biosynthesis and Catabolism of Polysaccharides in the Biofilm
Bacteria associated with macroalgae may either be endo- or epiphytic (Egan et al., 2013). Additionally, Pseudoalteromonas species are known to efficiently produce biofilms (Bowman, 2007). Accordingly, the chromosomes of P. carrageenovora contain functions involved in exopolysaccharide biosynthesis and degradation in the biofilm. There is a bacterial cellulose synthase (bcs) operon on chromosome I (genes PCAR9_a20703-PCAR9_a20711), following the type IIa synteny: bcsGFE, bcsRQABZC, as defined by (Römling and Galperin, 2015). Cellulose is synthesized on the cytoplasmic side of the inner membrane and it results from the functional coupling of a transmembrane cellulose synthase (gene bscA, GT2 family) and a cyclic-di-GMP-binding protein (bcsB) located in the periplasm. Other genes of the operon are involved in enzymatic activity and product yield. It includes gene bcsC, encoding a periplasmic protein to guide cellulose outside of the cell; genes bscZ and bcsE which are involved in cellulose production, such as the packing of fibrils; gene bcsQ, encoding a protein with a role in cellular localization of the BCS complex; and gene bcsR, involved in biofilm formation (Supplementary Table S3). Twenty-six of the other Pseudoalteromonas genomes contain the corresponding type IIa synteny. In the 16S rRNA gene phylogeny, 19 Pseudoalteromonas strains of the LDC harbor the bcs operon in their genomes while the other 7 strains are distributed along the phylogeny (Supplementary Figure S1 and Supplementary Table S6). This suggests that this operon has been acquired in Pseudoalteromonas genomes from independent HGT events.
Sucrose is a disaccharide which can be used as substrate by bacteria to produce exopolysaccharides (Flemming and Wingender, 2010). Chromosome II contains two PULs involved in sucrose utilization, PUL 4: PCAR9_b0346-PCAR9_b0351 and PUL 6: PCAR9_b0546-PCAR9_b0549, which include the necessary genes for sucrose import, sucrose catabolism and transcription regulation (Supplementary Table S3). PULs 4 and 6 are present in a majority of Pseudoalteromonas genomes (32 and 27, respectively) and are distributed along the phylogenetic tree (Supplementary Figure S1 and Supplementary Table S6). Several bacterial species contribute to biofilm formation and structure maintenance by producing levan, an extracellular beta-2,6-polyfructan with extensive branching through beta-2,1-linkages (Laue et al., 2006). This EPS is synthesized from sucrose by an extracellular levansucrase (Osman et al., 1986). This enzyme is found on chromosome I (GH68 family, PCAR9_a30563), as well as a levanbiose-producing levanase (GH32 family, PCAR9_a30564). Therefore, P. carrageenovora likely produces extracellular levan as another structural polysaccharide of the biofilm. Homologs are present only in two Pseudoalteromonas strains, which are distant from P. carrageenovora in the phylogenetic tree (Supplementary Figure S1 and Supplementary Table S6), suggesting an acquisition from independent HGT events.
Potential Virulence of P. carrageenovora
As expected, P. carrageenovora’s plasmid contains the genes of the replication/segregation system which is essential for plasmid maintenance (rep and parA/B, PCAR9_p0001-PCAR9_p0004, Friedman and Austin, 1988). It also encodes a gene cluster (PCAR9_p0005-PCAR9_p0014) potentially involved in a virulent character of P. carrageenovora. This cluster includes a two-component signal transduction system (a sensor histidine kinase QseC, PCAR9_p0008 and a DNA-binding response regulator QseB, PCAR9_p0009), a putative high affinity iron transporter gene (PCAR9_p0006), two long-chain acyl-CoA synthetase genes (PCAR9_p0012 and PCAR9_p0013) and a thermostable hemolysin gene (PCAR9_p0014). Iron transporters and two-component systems are known to play a role in the activation of virulence, as observed in the phytopathogen Erwinia amylovora (Zhao et al., 2009). The two long-chain acyl-CoA synthetase genes (PCAR9_p0012 and PCAR9_p0013) present in the cluster may regulate, for instance, cytosolic enzymes, ion channels, ion pumps, or genes (Faergeman and Knudsen, 1997). Hemolysin genes are also well-known bacterial virulence factors in several species of Vibrio involved in hemolysis of erythrocytes and in cytotoxicity (Nishibuchi and Kaper, 1995). When activated by iron, the QseB/QseC system of P. carrageenovora might be involved in the synthesis of the thermostable hemolysin. This gene cluster is conserved in 24 Pseudoalteromonas genomes distributed across the phylogenetic tree (Supplementary Figure S1 and Supplementary Table S7). Similar potential virulence clusters are also found in the genome of the pathogenic Gammaproteobacteria Vibrio alginolyticus 12G01, from which the thermostable hemolysin was characterized and shown to be toxic to zebrafish (Jia et al., 2010), and of the Alphaproteobacteria Nautella sp. R11, a red algal pathogen (Fernandes et al., 2011).
Discussion
Marine bacteria of the Pseudoalteromonas genus are frequently found at the surface of macroalgae (Hollants et al., 2011; Martin et al., 2014). Some strains are capable of assimilating macroalgal polysaccharides (Goecke et al., 2010; Martin et al., 2015). Our genomic and physiological analyses demonstrate that this is the case of P. carrageenovora, which is able to feed on carrageenans and other macroalgal polysaccharides (starch, laminarin, alginate and fucoidans, Table 2 and Supplementary Table S4).
The most surprising outcome of our genomic study is the presence of a plasmid harboring all the carrageenan-related genes of the genome of P. carrageenovora. Plasmids usually represent a selective advantage for bacteria living under environmental pressure as they often carry antibiotic resistance genes or genes allowing the use of specific compounds such as novel carbohydrate sources. They are transmitted between bacteria through HGTs, which happen more often in conditions such as those in biofilms, representing places of high cell density and metabolic activity (Sørensen et al., 2005). Noticeably, plasmids are not ubiquitously present in genomes of the Pseudoalteromonas genus (Médigue et al., 2005; Qin et al., 2011). Carrageenan-specific genes are conserved in only six closely related Pseudoalteromonas strains (Supplementary Figure S1 and Supplementary Table S6), suggesting they also possess a similar carrageenan-related plasmid only recently acquired. This plasmid includes the genes encoding kappa and lambda family carrageenases but no 3,6-anhydro-D-galactosidases (GH127 and GH129 families, Ficko-Blean et al., 2017). Therefore, P. carrageenovora is able to damage red algal tissues containing kappa family carrageenans, but it is not able to assimilate alone the resulting products. Within a red algal epibacterial community, P. carrageenovora could grow with D-galactose and 3,6-anhydro-D-galactose released by other bacteria with a complete catabolic pathway for kappa family carrageenans, such as Z. galactanivorans Dsij (Ficko-Blean et al., 2017). Notably, none of the analyzed Pseudoalteromonas genomes display GH127 or GH129 genes, suggesting that these genes were already absent from the plasmid when it was acquired by Pseudoalteromonas strains. In contrast, the growth experiments demonstrate that the lambda-carrageenan catabolism is complete. Our comparative genomic analyses suggest that this catabolism is essentially conferred by PUL 8 and highlights the GH110 enzymes as strong candidates for the still unidentified lambda-carrageenan 1,3-alpha-D-galactosidase activity. Among the 52 Pseudoalteromonas genomes, the genome of P. carrageenovora is the only one possessing GH150 lambda-carrageenase genes and the PUL 8. The most parsimonious scenario is that these genes were not initially present on the ancestral plasmid and were subsequently acquired through another HGT event. Overall, this plasmid gives an ecological advantage to P. carrageenovora to use carrageenophyte red macroalgae as a source of nutrient. This edge might be increased by the additional presence of a potential cluster of virulence, as seen in some red algal pathogens (Fernandes et al., 2011).
The other carbohydrate metabolisms are encoded by chromosomes I and II. Even though they are localized on the main chromosomes, these pathways do not have the same evolutionary histories. Starch and laminarin catabolisms (PUL 2 and PUL 5, respectively) are conserved in almost all Pseudoalteromonas genomes, suggesting an ancestral acquisition in the genus (Supplementary Table S4). This ancient character is consistent with the prevalence of these polymers in coastal environments. Starch and laminarin are the storage polysaccharides of macroalgae (red/green algae and brown algae, respectively), and of abundant phytoplankton (starch: dinoflagellates; chrysolaminarin: diatoms, haptophytes). The ability to use these abundant polymers is an adaptive advantage for Pseudoalteromonas strains living in the water column as well as on the surface of algae. The majority of the Pseudoalteromonas strains (32/52 genomes) can degrade sucrose, but only three (including P. carrageenovora) can use the released fructose to synthesize the exopolysaccharide levan. Such EPS are important to build biofilm structures and Pseudoalteromonas species are known to play important roles in marine biofilms (Holmström and Kjelleberg, 1999; Bowman, 2007). Alginate catabolism in P. carrageenovora is essentially due to PUL 1 which is very similar to one alginate utilization system characterized in Z. galactanivorans Dsij. Thomas and coworkers provided phylogenetic evidence that alginate-specific PULs originated from marine flavobacteria and were independently transferred several times to marine Gammaproteobacteria (Thomas et al., 2012). Our comparative genomic analyses strengthen this hypothesis, since PUL 1 is only conserved in the Pseudoalteromonas strains of the late diverging clade (LDC; Supplementary Figure S1). Therefore, alginate catabolism is much older than carrageenan catabolism in the Pseudoalteromonas genus, but it nonetheless resulted from an ancient HGT event which most likely involved the common ancestor of the LDC. Considering its level of conservation within the LDC, alginate catabolism is surely a helpful trait for these strains to colonize the brown algal surface. Consistently, all the 22 Pseudoalteromonas strains isolated from the brown alga Ascophyllum nodosum by Martin and coworkers were alginolytic, whereas only a minority were carrageenolytic (Martin et al., 2015). Interestingly, most strains with the cellulose biosynthesis (bcs) operon also belong to the same LDC. For these strains, this suggests that the bcs operon was transferred alongside the alginate PUL during the same HGT event. Nonetheless, cellulose biosynthesis was acquired independently three other times in Pseudoalteromonas evolution (Supplementary Figure S1). Like levan, cellulose is secreted as part of the EPS matrix and has a structuring or surface attachment role in biofilms (Flemming and Wingender, 2010; Römling and Galperin, 2015). It also allows cell protection from hazardous effect of UV radiation (Scott Williams and Cannon, 1989). Therefore, cellulose biosynthesis can be considered as another trait for the adaptation to a life at the surface of macroalgae.
Conclusion
In conclusion, the Pseudoalteromonas common ancestor likely had limited capacities to use complex carbohydrates, essentially focused on the storage polysaccharides of phytoplankton and macroalgae (laminarin and starch) which are abundant in coastal environments. Sucrose utilization is also a relatively ancient metabolic route in the Pseudoalteromonas genus. However, the adaptive traits genuinely specific for a macroalgae-associated lifestyle were acquired much later in Pseudoalteromonas evolutionary history through a succession of HGT events. A major event was the acquisition of alginate catabolism by the common ancestor of the LDC (likely from marine flavobacteria), a catabolism which is largely conserved in Pseudoalteromonas strains isolated from macroalgae. Probably the same HGT event has provided the capacity to synthesize cellulose, which helps to build biofilms on hosts and abiotic surfaces. In contrast, the ability to degrade kappa family carrageenan is more recent, due to a plasmid only found in 7 Pseudoalteromonas strains. Interestingly, these carrageenolytic strains are also found in the LDC. It seems that the acquisition of a first trait specific to macroalgae colonization (alginate catabolism) has favored subsequent HGTs. This could be explained by the close proximity between cells within a macroalgal biofilm, which facilitates genetic exchange (Hausner and Wuertz, 1999; Flemming and Wingender, 2010). Finally, P. carrageenovora is a particularly emblematic example of such successive acquisitions of adaptive traits since this species has even more recently acquired the pathway for the utilization of lambda-carrageenan.
Author Contributions
AG, TB, and GuM designed the study. AG and TB carried out cultivation experiments and DNA extraction of the bacterium. GM performed the optical-mapping. ED and DV performed genome assembly, cleaning and automatic annotation with substantial intellectual contribution. AG performed genome annotation. AG, TB, MM-M, and GuM analyzed and interpreted the data. AG wrote the first draft of the manuscript and GuM contributed substantially to the revisions. All authors read and approved the final manuscript.
Conflict of Interest Statement
The authors declare that the research was conducted in the absence of any commercial or financial relationships that could be construed as a potential conflict of interest.
Acknowledgments
We warmly thank Philippe Potin, François Thomas and Elizabeth Ficko-Blean for fruitful discussions, Laëtitia Mest and Nolwen Le Duff for help in bacterial growth and Aurélie Préchoux for providing some substrates. This work has benefited from the facilities and expertise of the high-throughput sequencing platform of i2bc (Centre de Recherche de Gif-sur-Yvette) and of the CEA/GENOSCOPE (http://www.genoscope.cns.fr/). We also wish to thank for providing computational resources and bioinformatic analyses: the INRA MIGALE bioinformatics platform (http://migale.jouy.inra.fr), the LABGeM (CEA/Genoscope & CNRS UMR 8030), the France Génomique National infrastructure and the French Institute of Bioinformatics (IFB), which are funded as part of the investment expenditure program managed by the Agence Nationale pour la Recherche (ANR), references ANR-10-INBS-09 and ANR-11-INBS-0013, respectively. GM, TB, DV, and ED acknowledge support from the ANR with regard to the BLUE ENZYMES project (reference ANR-14-CE19-0020-01). AG, TB, and GM are also grateful to ANR for its support with regards to the investment expenditure program IDEALG (http://www.idealg.ueb.eu/, reference ANR-10-BTBR-04). We thank the two reviewers for their helpful comments on the earlier version of the manuscript.
Supplementary Material
The Supplementary Material for this article can be found online at: https://www.frontiersin.org/articles/10.3389/fmicb.2018.02740/full#supplementary-material
Footnotes
References
Akagawa-Matsushita, M., Matsuo, M., Koga, Y., and Yamasato, K. (1992). Alteromonas atlantica sp. nov. and Alteromonas carragenovora sp. nov., bacteria that decompose algal polysaccharides. Int. J. Syst. Bacteriol. 42, 621–627. doi: 10.1099/00207713-42-4-621
Aronson, J. M., Cooper, B. A., and Fuller, M. S. (1967). Glucans of oomycete cell walls. Science 155, 332–335. doi: 10.1126/science.155.3760.332
Aspeborg, H., Coutinho, P. M., Wang, Y., Brumer, H., and Henrissat, B. (2012). Evolution, substrate specificity and subfamily classification of glycoside hydrolase family 5 (GH5). BMC Evol. Biol. 12:186. doi: 10.1186/1471-2148-12-186
Barbeyron, T., Brillet-Guéguen, L., Carré, W., Carrière, C., Caron, C., Czjzek, M., et al. (2016a). Matching the diversity of sulfated biomolecules: creation of a classification database for sulfatases reflecting their substrate specificity. PLoS One 11:e0164846. doi: 10.1371/journal.pone.0164846
Barbeyron, T., Thomas, F., Barbe, V., Teeling, H., Schenowitz, C., Dossat, C., et al. (2016b). Habitat and taxon as driving forces of carbohydrate catabolism in marine heterotrophic bacteria: example of Zobellia galactanivorans DsijT. Environ. Microbiol. 18, 4610–4627. doi: 10.1111/1462-2920.13584
Barbeyron, T., Henrissat, B., and Kloareg, B. (1994). The gene encoding the kappa-carrageenase of Alteromonas carrageenovora is related to beta-1,3-1,4-glucanases. Gene 139, 105–109. doi: 10.1016/0378-1119(94)90531-2
Barbeyron, T., Kean, K., and Forterre, P. (1984). DNA adenine methylation of GATC sequences appeared recently in the Escherichia coli lineage. J. Bacteriol. 160, 586–590.
Barbeyron, T., Potin, P., Richard, C., Collin, O., and Kloareg, B. (1995). Arylsulphatase from Alteromonas carrageenovora. Microbiology 141, 2897–2904. doi: 10.1099/13500872-141-11-2897
Bartnicki-Garcia, S. (1968). Cell wall chemistry, morphogenesis, and taxonomy of fungi. Annu. Rev. Microbiol. 22, 87–108. doi: 10.1146/annurev.mi.22.100168.000511
Bjursell, M. K., Martens, E. C., and Gordon, J. I. (2006). Functional genomic and metabolic studies of the adaptations of a prominent adult human gut symbiont, Bacteroides thetaiotaomicron, to the suckling period. J. Biol. Chem. 281, 36269–36279. doi: 10.1074/jbc.M606509200
Bowman, J. P. (2007). Bioactive compound synthetic capacity and ecological significance of marine bacterial genus Pseudoalteromonas. Mar. Drugs 5, 220–241. doi: 10.3390/md20070017
Clamp, M., Cuff, J., Searle, S. M., and Barton, G. J. (2004). The Jalview Java alignment editor. Bioinformatics 20, 426–427. doi: 10.1093/bioinformatics/btg430
Colin, S., Deniaud, E., Jam, M., Descamps, V., Chevolot, Y., Kervarec, N., et al. (2006). Cloning and biochemical characterization of the fucanase FcnA: definition of a novel glycoside hydrolase family specific for sulfated fucans. Glycobiology 16, 1021–1032. doi: 10.1093/glycob/cwl029
De Ley, J., and Doudoroff, M. (1957). The metabolism of D-galactose in Pseudomonas saccharophila. J. Biol. Chem. 227:757.
Deniaud, E., Quemener, B., Fleurence, J., and Lahaye, M. (2003). Structural studies of the mix-linked β-(1→3)/β-(1→4)–D-xylans from the cell wall of Palmaria palmata (Rhodophyta). Int. J. Biol. Macromol. 33, 9–18. doi: 10.1016/S0141-8130(03)00058-8
Deniaud-Bouët, E., Hardouin, K., Potin, P., Kloareg, B., and Hervé, C. (2017). A review about brown algal cell walls and fucose-containing sulfated polysaccharides: cell wall context, biomedical properties and key research challenges. Carbohydr. Polym. 175, 395–408. doi: 10.1016/j.carbpol.2017.07.082
Deniaud-Bouët, E., Kervarec, N., Michel, G., Tonon, T., Kloareg, B., and Hervé, C. (2014). Chemical and enzymatic fractionation of cell walls from fucales: insights into the structure of the extracellular matrix of brown algae. Ann. Bot. 114, 1203–1216. doi: 10.1093/aob/mcu096
Egan, S., Harder, T., Burke, C., Steinberg, P., Kjelleberg, S., and Thomas, T. (2013). The seaweed holobiont: understanding seaweed-bacteria interactions. FEMS Microbiol. Rev. 37, 462–476. doi: 10.1111/1574-6976.12011
Ewing, B., Ewing, B., Hillier, L., Hillier, L., Wendl, M. C., Wendl, M. C., et al. (2005). Base-calling of automated sequencer traces using. Genome Res. 8, 175–185. doi: 10.1101/gr.8.3.175
Faergeman, N. J., and Knudsen, J. (1997). Role of long-chain fatty acyl-CoA esters in the regulation of metabolism and in cell signalling. Biochem. J. 323, 1–12. doi: 10.1042/bj3230001
Felsenstein, J. (2009). Confidence limits on phylogenies: an approach using the bootstrap. Evolution. 39, 783–791. doi: 10.2307/2408678
Fernandes, N., Case, R. J., Longford, S. R., Seyedsayamdost, M. R., Steinberg, P. D., Kjelleberg, S., et al. (2011). Genomes and virulence factors of novel bacterial pathogens causing bleaching disease in the marine red alga Delisea pulchra. PLoS One 6:e27387. doi: 10.1371/journal.pone.0027387
Ficko-Blean, E., Hervé, C., and Michel, G. (2015). Sweet and sour sugars from the sea: the biosynthesis and remodeling of sulfated cell wall polysaccharides from marine macroalgae. Perspect. Phycol. 2, 51–64. doi: 10.1127/pip/2015/0028
Ficko-Blean, E., Préchoux, A., Thomas, F., Rochat, T., Larocque, R., Zhu, Y., et al. (2017). Carrageenan catabolism is encoded by a complex regulon in marine heterotrophic bacteria. Nat. Commun. 8, 1–38. doi: 10.1038/s41467-017-01832-6
Flemming, H.-C., and Wingender, J. (2010). The biofilm matrix. Nat. Rev. Microbiol. 8, 623–633. doi: 10.1038/nrmicro2415
Friedman, S. A., and Austin, S. J. (1988). The P1 plasmid-partition system synthesizes two essential proteins from an autoregulated operon. Plasmid 19, 103–112. doi: 10.1016/0147-619X(88)90049-2
Goecke, F., Labes, A., Wiese, J., and Imhoff, J. F. (2010). Chemical interactions between marine macroalgae and bacteria. Mar. Ecol. Prog. Ser. 409, 267–300. doi: 10.3354/meps08607
Grondin, J. M., Tamura, K., Déjean, G., Abbott, D. W., and Brumer, H. (2017). Polysaccharide utilization loci: fuelling microbial communities. J. Bacteriol. 860–816. doi: 10.1128/JB.00860-16
Guibet, M., Colin, S., Barbeyron, T., Genicot, S., Kloareg, B., Michel, G., et al. (2007). Degradation of λ-carrageenan by Pseudoalteromonas carrageenovora λ-carrageenase: a new family of glycoside hydrolases unrelated to κ- and ι-carrageenases. Biochem. J. 404, 105–114. doi: 10.1042//BJ20061359
Hausner, M., and Wuertz, S. (1999). High rates of conjugation in bacterial biofilms as determined by quantitative in situ analysis. Appl. Environ. Microbiol. 65, 3710–3713.
Hehemann, J. H., Truong, L., Van Unfried, F., Welsch, N., Kabisch, J., Heiden, S. E., et al. (2017). Aquatic adaptation of a laterally acquired pectin degradation pathway in marine gammaproteobacteria. Environ. Microbiol. 19, 2320–2333. doi: 10.1111/1462-2920.13726
Hobbs, J. K., Lee, S. M., Robb, M., Hof, F., Barr, C., Abe, K. T., et al. (2016). KdgF, the missing link in the microbial metabolism of uronate sugars from pectin and alginate. Proc. Natl. Acad. Sci. U.S.A. 113, 6188–6193. doi: 10.1073/pnas.1524214113
Hollants, J., Leroux, O., Leliaert, F., Decleyre, H., de Clerck, O., and Willems, A. (2011). Who is in there? exploration of endophytic bacteria within the siphonous green seaweed bryopsis (Bryopsidales, Chlorophyta). PLoS One 6:e26458. doi: 10.1371/journal.pone.0026458
Holmström, C., and Kjelleberg, S. (1999). Marine Pseudoalteromonas species are associated with higher organisms and produce biologically active extracellular agents. FEMS Microb. Ecol. 30, 285–293. doi: 10.1016/S0168-6496(99)00063-X
Jia, A., Woo, N. Y. S., and Zhang, X. H. (2010). Expression, purification, and characterization of thermolabile hemolysin (TLH) from Vibrio alginolyticus. Dis. Aquat. Organ. 90, 121–127. doi: 10.3354/dao02225
Johnston, K. H., and McCandless, E. L. (1973). Enzymic hydrolysis of the potassium chloride soluble fraction of carrageenan: properties of “λ-carrageenases” from Pseudomonas carrageenovora. Can. J. Microbiol. 19, 779–788. doi: 10.1139/m73-127
Katoh, K., and Standley, D. M. (2013). MAFFT multiple sequence alignment software version 7: improvements in performance and usability. Mol. Biol. Evol. 30, 772–780. doi: 10.1093/molbev/mst010
Kimura, M. (1980). A simple method for estimating evolutionary rates of base substitutions through comparative studies of nucleotide sequences. J. Mol. Evol. 16, 111–120. doi: 10.1007/BF01731581
Kloareg, B., and Quatrano, R. S. (1988). Structure of the cell walls of marine algae and ecophysiological functions of the matrix polysaccharides. Ocean. Mar. Biol. Annu. Rev. 26, 259–315.
Latreille, P., Norton, S., Goldman, B. S., Henkhaus, J., Miller, N., Barbazuk, B., et al. (2007). Optical mapping as a routine tool for bacterial genome sequence finishing. BMC Genomics 8:321. doi: 10.1186/1471-2164-8-321
Laue, H., Schenk, A., Li, H., Lambertsen, L., Neu, T. R., Molin, S., et al. (2006). Contribution of alginate and levan production to biofilm formation by Pseudomonas syringae. Microbiology 152, 2909–2918. doi: 10.1099/mic.0.28875-0
Leloir, L. F. (1951). The enzymatic transformation of uridine diphosphate glucose into a galactose derivative. Arch. Biochem. Biophys. 33, 186–190. doi: 10.1016/0003-9861(51)90096-3
Liu, Q. P., Sulzenbacher, G., Yuan, H., Bennett, E. P., Pietz, G., Saunders, K., et al. (2007). Bacterial glycosidases for the production of universal red blood cells. Nat. Biotechnol. 25, 454–464. doi: 10.1038/nbt1298
Lombard, V., Golaconda Ramulu, H., Drula, E., Coutinho, P. M., and Henrissat, B. (2014). The carbohydrate-active enzymes database (CAZy) in 2013. Nucleic Acids Res. 42, D490–D495. doi: 10.1093/nar/gkt1178
Mabeau, S., and Kloareg, B. (1987). Isolation and analysis of the cell walls of brown algae?: Fucus spiralis, F. ceranoides, F. vesiculosus, F. serratus, Bifurcaria bifurcata and Laminaria digitata. J. Exp. Bot. 38, 1573–1580. doi: 10.1093/jxb/38.9.1573
Martens, E. C., Koropatkin, N. M., Smith, T. J., and Gordon, J. I. (2009). Complex glycan catabolism by the human gut microbiota: the bacteroidetes sus-like paradigm. J. Biol. Chem. 284, 24673–24677. doi: 10.1074/jbc.R109.022848
Martin, M., Barbeyron, T., Martin, R., Portetelle, D., Michel, G., and Vandenbol, M. (2015). The cultivable surface microbiota of the brown alga Ascophyllum nodosum is enriched in macroalgal-polysaccharide-degrading bacteria. Front. Microbiol. 6:1487. doi: 10.3389/fmicb.2015.01487
Martin, M., Portetelle, D., Michel, G., and Vandenbol, M. (2014). Microorganisms living on macroalgae: diversity, interactions, and biotechnological applications. Appl. Microbiol. Biotechnol. 98, 2917–2935. doi: 10.1007/s00253-014-5557-2
Martin, M., Vandermies, M., Joyeux, C., Martin, R., Barbeyron, T., Michel, G., et al. (2016). Discovering novel enzymes by functional screening of plurigenomic libraries from alga-associated Flavobacteriia and Gammaproteobacteria. Microbiol. Res. 18, 52–61. doi: 10.1016/j.micres.2016.03.005
McLean, M. W., and Williamson, F. B. (1979a). Glycosulphatase from Pseudomonas carrageenovora purification and some properties. Eur. J. Biochem. 101, 497–505. doi: 10.1111/j.1432-1033.1979.tb19744.x
McLean, M. W., and Williamson, F. B. (1979b). Kappa-carrageenase from Pseudomonas carrageenovora. Eur. J. Biochem. 93, 553–558. doi: 10.1111/j.1432-1033.1979.tb12854.x
McLean, M. W., and Williamson, F. B. (1981). Neocarratetraose 4-O-Monosulphate beta-hydrolase from Pseudomonas carrageenovora. Eur. J. Biochem. 456, 447–456. doi: 10.1111/j.1432-1033.1981.tb05084.x
Médigue, C., Krin, E., Pascal, G., Barbe, V., Bernsel, A., Bertin, P. N., et al. (2005). Coping with cold: the genome of the versatile marine Antarctica bacterium Pseudoalteromonas haloplanktis TAC125. Genome Res. 15, 1325–1335. doi: 10.1101/gr.4126905
Michel, G., Barbeyron, T., Flament, D., Vernet, T., Kloareg, B., and Dideberg, O. (1999). Expression, purification, crystallization and preliminary X-ray analysis of the iota-carrageenase from Pseudoalteromonas carrageenovora. Acta Crystallogr. Sect. D Biol. Crystallogr. 55, 918–920. doi: 10.1107/S0907444998018526
Michel, G., Chantalat, L., Duee, E., Barbeyron, T., Henrissat, B., Kloareg, B., et al. (2001). The κ-carrageenase of P. carrageenovora features a tunnel-shaped active site: a novel insight in the evolution of clan-B glycoside hydrolases. Structure 9, 513–525. doi: 10.1016/S0969-2126(01)00612-8
Michel, G., Nyval-Collen, P., Barbeyron, T., Czjzek, M., and Helbert, W. (2006). Bioconversion of red seaweed galactans: a focus on bacterial agarases and carrageenases. Appl. Microbiol. Biotechnol. 71, 23–33. doi: 10.1007/s00253-006-0377-7
Michel, G., Tonon, T., Scornet, D., Cock, J. M., and Kloareg, B. (2010). Central and storage carbon metabolism of the brown alga Ectocarpus siliculosus: insights into the origin and evolution of storage carbohydrates in Eukaryotes. New Phytol. 188, 67–81. doi: 10.1111/j.1469-8137.2010.03345.x
Myklestad, S. M. (1989). Production, chemical structure, metabolism, and biological function of the (1→3)-linked, β-D-glucans in diatoms. Biol. Oceanogr. 6, 313–326.
Neumann, A. M., Balmonte, J. P., Berger, M., Giebel, H. A., Arnosti, C., Voget, S., et al. (2015). Different utilization of alginate and other algal polysaccharides by marine Alteromonas macleodii ecotypes. Environ. Microbiol. 17, 3857–3868. doi: 10.1111/1462-2920.12862
Nguyen, V. H., and Lavenier, D. (2009). PLAST: parallel local alignment search tool for database comparison. BMC Bioinformatics 10:329. doi: 10.1186/1471-2105-10-329
Nishibuchi, M., and Kaper, J. B. (1995). Thermostable direct hemolysin gene of Vibrio parahaemolyticus: a virulence gene acquired by a marine bacterium. Infect. Immun. 63, 2093–2099.
Noinaj, N., Guillier, M., Barnard, T. J., and Buchanan, S. K. (2010). TonB-dependent transporters: regulation, structure, and function. Annu. Rev. Microbiol. 64, 43–60. doi: 10.1146/annurev.micro.112408.134247
Osman, S. F., Fett, W. F., and Fishman, M. L. (1986). Exopolysaccharides of the phytopathogen Pseudomonas syringae pv. glycinea. J. Bacteriol. 166, 66–71. doi: 10.1128/jb.166.1.66-71.1986
Popper, Z. A., Michel, G., Hervé, C., Domozych, D. S., Willats, W. G. T., Tuohy, M. G., et al. (2011). Evolution and diversity of plant cell walls: from algae to flowering plants. Annu. Rev. Plant Biol. 62, 567–590. doi: 10.1146/annurev-arplant-042110-103809
Potin, P., Richard, C., Barbeyron, T., Henrissat, B., Gey, C., Petillot, Y., et al. (1995). Processing and hydrolytic mechanism of the cgkA-encoded κ-carrageenase of Alteromonas carrageenovora. Eur. J. Biochem. 228, 971–975. doi: 10.1111/j.1432-1033.1995.0971m.x
Preiss, J., and Ashwell, G. (1962a). Alginic acid metabolism in bacteria. I. Enzymatic formation of unsaturated oligosaccharides and 4-deoxy-L-erythro-5-hexoseulose uronic acid. J. Biol. Chem. 237,309–316.
Preiss, J., and Ashwell, G. (1962b). Alginic acid metabolism in bacteria. II. The enzymatic reduction of 4-deoxy-L-erythro-5-hexoseulose uronic acid to 2-keto-3-deoxy-D-gluconic acid. J. Biol. Chem. 237, 317–321.
Punta, M., Coggill, P. C., Eberhardt, R. Y., Mistry, J., Tate, J., Boursnell, C., et al. (2012). The Pfam protein families database. Nucleic Acids Res. 40, D290–D301. doi: 10.1093/nar/gkr1065
Qin, Q.-L., Li, Y., Zhang, Y.-J., Zhou, Z.-M., Zhang, W.-X., Chen, X.-L., et al. (2011). Comparative genomics reveals a deep-sea sediment-adapted life style of Pseudoalteromonas sp. SM9913. ISME J. 5, 274–284. doi: 10.1038/ismej.2010.103
Raimundo, S. C., Pattathil, S., Eberhard, S., Hahn, M. G., and Popper, Z. A. (2017). β-1,3-glucans are components of brown seaweed (Phaeophyceae) cell walls. Protoplasma 254, 997–1016. doi: 10.1007/s00709-016-1007-6
Römling, U., and Galperin, M. Y. (2015). Bacterial cellulose biosynthesis: diversity of operons, subunits, products, and functions. Trends Microbiol. 23, 545–557. doi: 10.1016/j.tim.2015.05.005
Saitou, N., and Nei, M. (1987). The neighbor-joining method - a new method for reconstructing phylogenetic trees. Mol. Biol. Evol. 4,406–425.
Schultz-Johansen, M., Bech, P. K., Hennessy, R. C., Glaring, M. A., Barbeyron, T., Czjzek, M., et al. (2018). A novel enzyme portfolio for red algal polysaccharide degradation in the marine bacterium Paraglaciecola hydrolytica S66T encoded in a sizeable polysaccharide utilization locus. Front. Microbiol. 9:839. doi: 10.3389/fmicb.2018.00839
Scott Williams, W. S., and Cannon, R. E. (1989). Alternative environmental roles for cellulose produced by Acetobacter xylinum. Appl. Environ. Microbiol. 55, 2448–2452.
Sørensen, S. J., Bailey, M., Hansen, L. H., Kroer, N., Wuertz, S., Sorensen, S. J., et al. (2005). Studying plasmid horizontal transfer in situ: a critical review. Nat. Rev. Microbiol. 3, 700–710. doi: 10.1038/nrmicro1232
Tamura, K., Peterson, D., Peterson, N., Stecher, G., Nei, M., and Kumar, S. (2011). MEGA5: molecular evolutionary genetics analysis using maximum likelihood, evolutionary distance, and maximum parsimony methods. Mol. Biol. Evol. 28, 2731–2739. doi: 10.1093/molbev/msr121
Thomas, F., Barbeyron, T., and Michel, G. (2011). Evaluation of reference genes for real-time quantitative PCR in the marine flavobacterium Zobellia galactanivorans. J. Microbiol. Methods 84, 61–66. doi: 10.1016/j.mimet.2010.10.016
Thomas, F., Barbeyron, T., Tonon, T., Génicot, S., Czjzek, M., and Michel, G. (2012). Characterization of the first alginolytic operons in a marine bacterium: from their emergence in marine Flavobacteriia to their independent transfers to marine Proteobacteria and human gut Bacteroides. Environ. Microbiol. 14, 2379–2394. doi: 10.1111/j.1462-2920.2012.02751.x
Thomas, T., Evans, F. F., Schleheck, D., Mai-Prochnow, A., Burke, C., Penesyan, A., et al. (2008). Analysis of the Pseudoalteromonas tunicata genome reveals properties of a surface-associated life style in the marine environment. PLoS One 3:e3252. doi: 10.1371/journal.pone.0003252
Vallenet, D., Calteau, A., Cruveiller, S., Gachet, M., Lajus, A., Josso, A., et al. (2017). MicroScope in 2017: an expanding and evolving integrated resource for community expertise of microbial genomes. Nucleic Acids Res. 45, D517–D528. doi: 10.1093/nar/gkw1101
Wegner, C. E., Richter-Heitmann, T., Klindworth, A., Klockow, C., Richter, M., Achstetter, T., et al. (2013). Expression of sulfatases in Rhodopirellula baltica and the diversity of sulfatases in the genus Rhodopirellula. Mar. Genomics 9, 51–61. doi: 10.1016/j.margen.2012.12.001
Yaphe, W., and Baxter, B. (1955). the enzymic hydrolysis of carrageenin. Appl. Microbiol. 3, 380–383.
Yaphe, W., and Morgan, K. (1959). Enzymic hydrolysis of fucoidin by Pseudomonas atlantica and Pseudomonas carrageenovora. Nature 183, 761–762. doi: 10.1038/183761b0
Zerbino, D. R., and Birney, E. (2008). Velvet: algorithms for de novo short read assembly using de Bruijn graphs. Genome Res. 18, 821–829. doi: 10.1101/gr.074492.107
Zhao, Y., Wang, D., Nakka, S., Sundin, G. W., and Korban, S. S. (2009). Systems level analysis of two-component signal transduction systems in Erwinia amylovora: role in virulence, regulation of amylovoran biosynthesis and swarming motility. BMC Genomics 10:245. doi: 10.1186/1471-2164-10-245
Keywords: carrageenan, CAZymes, alginate, Gammaproteobacteria, Pseudoalteromonas, marine bacteria, algal holobiont, biofilm
Citation: Gobet A, Barbeyron T, Matard-Mann M, Magdelenat G, Vallenet D, Duchaud E and Michel G (2018) Evolutionary Evidence of Algal Polysaccharide Degradation Acquisition by Pseudoalteromonas carrageenovora 9T to Adapt to Macroalgal Niches. Front. Microbiol. 9:2740. doi: 10.3389/fmicb.2018.02740
Received: 08 August 2018; Accepted: 26 October 2018;
Published: 22 November 2018.
Edited by:
Alison Buchan, University of Tennessee, Knoxville, United StatesReviewed by:
Tomoo Sawabe, Hokkaido University, JapanXiao-Hua Zhang, Ocean University of China, China
Copyright © 2018 Gobet, Barbeyron, Matard-Mann, Magdelenat, Vallenet, Duchaud and Michel. This is an open-access article distributed under the terms of the Creative Commons Attribution License (CC BY). The use, distribution or reproduction in other forums is permitted, provided the original author(s) and the copyright owner(s) are credited and that the original publication in this journal is cited, in accordance with accepted academic practice. No use, distribution or reproduction is permitted which does not comply with these terms.
*Correspondence: Angélique Gobet, YW5nZWxpcXVlZ29iZXRAZ21haWwuY29t Gurvan Michel, Z3VydmFuLm1pY2hlbEBzYi1yb3Njb2ZmLmZy; Z3VydmFuQHNiLXJvc2NvZmYuZnI=