- 1State Key Laboratory of Applied Microbiology Southern China, Guangdong Provincial Key Laboratory of Microbial Culture Collection and Application, Guangdong Microbial Culture Collection Center (GDMCC), Guangdong Open Laboratory of Applied Microbiology, Guangdong Institute of Microbiology, Guangzhou, China
- 2CAS Key Laboratory of Tropical Marine Bio-resources and Ecology, Guangdong Key Laboratory of Marine Materia Medica, RNAM Center for Marine Microbiology, South China Sea Institute of Oceanology, Chinese Academy of Sciences, Guangzhou, China
- 3Center for Disease Control and Prevention of Guangdong Province, Guangzhou, China
- 4University of Chinese Academy of Sciences, Beijing, China
Multidrug-resistant (MDR) Escherichia coli poses a great challenge for public health in recent decades. Polymyxins have been reconsidered as a valuable therapeutic option for the treatment of infections caused by MDR E. coli. A plasmid-encoded colistin resistance gene mcr-1 encoding phosphoethanolamine transferase has been recently described in Enterobacteriaceae. In this study, a total of 123 E. coli isolates obtained from patients with diarrheal diseases in China were used for the genetic analysis of colistin resistance in clinical isolates. Antimicrobial resistance profile of polymyxin B (PB) and 11 commonly used antimicrobial agents were determined. Among the 123 E. coli isolates, 9 isolates (7.3%) were resistant to PB and PCR screening showed that seven (5.7%) isolates carried the mcr-1 gene. A hybrid sequencing analysis using single-molecule, real-time (SMRT) sequencing and Illumina sequencing was then performed to resolve the genomes of the seven mcr-1 positive isolates. These seven isolates harbored multiple plasmids and are MDR, with six isolates carrying one mcr-1 positive plasmid and one isolate (14EC033) carrying two mcr-1 positive plasmids. These eight mcr-1 positive plasmids belonged to the IncX4, IncI2, and IncP1 types. In addition, the mcr-1 gene was the solo antibiotic resistance gene identified in the mcr-1 positive plasmids, while the rest of the antibiotic resistance genes were mostly clustered into one or two plasmids. Interestingly, one mcr-1 positive isolate (14EC047) was susceptible to PB, and we showed that the activity of MCR-1-mediated colistin resistance was not phenotypically expressed in 14EC047 host strain. Furthermore, three isolates exhibited resistance to PB but did not carry previously reported mcr-related genes. Multilocus sequence typing (MLST) showed that these mcr-1 positive E. coli isolates belonged to five different STs, and three isolates belonged to ST301 which carried multiple virulence factors related to diarrhea. Additionally, the mcr-1 positive isolates were all susceptible to imipenem (IMP), suggesting that IMP could be used to treat infection caused by mcr-1 positive E. coli isolates. Collectively, this study showed a high occurrence of mcr-1 positive plasmids in patients with diarrheal diseases of Guangzhou in China and the abolishment of the MCR-1 mediated colistin resistance in one E. coli isolate.
Introduction
Escherichia coli is an important member of the intestinal microbiota of humans and animals (Finegold et al., 1983). Most E. coli strains are harmless and even benefit the host by preventing colonization of the harmful pathogens (Chang et al., 2004). However, some E. coli strains have evolved into pathogenic E. coli by the acquisition of virulence factors through plasmids, transposons, bacteriophages, and/or pathogenicity islands (Nataro and Kaper, 1998). Pathogenic E. coli has been recognized as an important cause of extraintestinal and intestinal infections in both humans and companion animals (Russo and Johnson, 2003; Wiles et al., 2008). In addition, the emergence of E. coli strains showing resistance to broad-spectrum of antimicrobial agents had been reported in the 1980s (Novick, 1981). Emergence of multidrug-resistant (MDR) E. coli has become an urgent global health threat due to the lack of effective antimicrobial agents in recent decades.
Polymyxins (including colistin) have been reconsidered as a valuable therapeutic option for the treatment of infections caused by Gram-negative bacteria with MDR including E. coli (Biswas et al., 2012). In 2015, a plasmid-encoded colistin resistance gene mcr-1 encoding phosphoethanolamine transferase was described in Enterobacteriaceae isolated from humans and livestock in China (Liu et al., 2016). Since then, plasmid-mediated polymyxin resistance by mcr-1 has been reported worldwide in livestock, food, and humans (Poirel et al., 2017). Currently, eight types of mcr genes [mcr-1 (1626 bp), mcr-2 (1617 bp), mcr-3 (1626 bp), mcr-4 (1626 bp), mcr-5 (1644 bp), mcr-6 (1617 bp), mcr-7 (1620 bp), and mcr-8 (1698 bp)] have been described in E. coli and other Gram-negative bacteria (Liu et al., 2016; Xavier et al., 2016; AbuOun et al., 2017; Borowiak et al., 2017; Carattoli et al., 2017; Yin et al., 2017; Wang et al., 2018;Yang et al., 2018). Monitoring of colistin-resistance and MDR as well as determining the genetic source of the colistin-resistant and MDR in clinical isolates are thus needed in the clinical treatments of E. coli-related infections.
The purpose of this study was to determine the prevalence of polymyxin resistance and MDR among the 123 E. coli isolates obtained from patients with diarrheal diseases in China from 2013 to 2016. The profile of resistance to 12 commonly used antimicrobial agents including polymyxin B (PB) was analyzed in these isolates. In order to determine the distribution of the resistance genes in the mcr-1 positive isolates, we sequenced and de novo assembled the chromosomes and the plasmids of the seven mcr-1 positive isolates. Since a recent study showed the shufflon is one of the most difficult regions for de novo genome assembly especially for those of IncI2 plasmids carrying the mcr-1 gene (Sekizuka et al., 2017), a hybrid sequencing analysis approach using the single-molecule, real-time (SMRT) sequencing and Illumina sequencing was performed to avoid the possible misassemble caused by these shufflons.
Materials and Methods
Bacterial Identification and PCR
In total, 123 E. coli isolates were collected from the fecal samples of patients with diarrheal diseases during 2013 to 2016 by the Guangdong Provincial Center for Disease Control and Prevention (CDC) in China. These isolates were subsequently identified as E. coli strains using PCR amplification of the 16S rRNA gene using the commonly used primer pair 27F/1492R (Weisburg et al., 1991) (Supplementary Table S1). The presence of the mcr-1 gene in these isolates was screened via PCR using primers list in Supplementary Table S1 as described previously (Liu et al., 2016; Xavier et al., 2016). The presence of mcr-1-pap2 region in the rhmT gene was confirmed by PCR with the primers RhmT-F and RhmT-R (Supplementary Table S1).
Susceptibility Testing
The antibiotics tested were ampicillin (AMP), PB, cefoxitin (CFX), ceftazidime (CAZ), imipenem (IMP), cefotaxime (CTX), cefepime (FEP), ciprofloxacin (CIP), gentamycin (GEN), sulfamethoxazole–trimethoprim (SXT), chloramphenicol (CM), and tetracycline (TET) in this study (Supplementary Table S2). The antibiotic resistance level was described by the minimum inhibitory concentrations (MICs) determined using a custom-made 96-well MIC panel (Xingbo Biotech, Shanghai, China). Results were interpreted according to the criteria of the Clinical and Laboratory Standards Institute (CLSI) (Clinical and Laboratory Standards Institute, 2017).
Whole-Genome Sequencing
Whole-genome sequencing of seven mcr-1 positive isolates was performed by Shanghai Majorbio Bio-Pharm Technology Co., Ltd. using Illumina HiSeq 4000 sequencing technology with a 350-bp size library. Paired-end Illumina reads were assembled with SOAP denovo v2.041. The gaps of seven mcr-1 positive isolates were closed using a PacBio RS II system (Pacific Biosciences, Menlo Park, United States) with a 10-kb library and P6/C4 chemistry. De novo assembly was performed with HGAP v3 (Pacific Biosciences). The complete genome sequence was annotated using Glimmer 3.022 and BLASTN. Multilocus sequence typing (MLST) profiles, serotyping, virulence factors, antibiotic resistance gene contents and plasmid incompatibility groups were analyzed through the website of the Center for Genomic Epidemiology3. The nucleotide sequences of the genomes and plasmids of seven mcr-1 positive isolates have been submitted to GenBank with accession Nos. CP024127-CP024158 and listed in Table 1.
Construction of Strains and Vectors
The pUC19 and pEX18Gm vectors were used to express mcr-1 gene in E. coli K-12 or in 14EC047. The coding region and its promoter sequence of mcr-1 genes were amplified with primer pair listed in Supplementary Table S1 with genomic DNAs from 14EC001 and 14EC047 as well as pHNSHP45 plasmid DNA as template, respectively. PCR products (pHNSHP45 plasmid DNA as template) were digested with BamHI and SalI and purified with using a gel extraction kit (Qiagen, Hilden, Germany). The purified PCR products were ligated into the pEX18Gm and transferred into E. coli K-12 and 14EC047 by electroporation. Furthermore, the mcr-1 gene and their promoter sequence from 14EC001 and 14EC047 were ligated into a cloning vector pUC19 yielding pUC19-mcr-1. pUC19-mcr-1 was then transferred into E. coli K-12 by electroporation. The correct constructs were verified by DNA sequencing.
Results and Discussion
Antimicrobial Resistance Profile of Clinical E. coli Isolates
The results of the susceptibility testing for all isolates are summarized in Figure 1. Most isolates studied (n = 98; 79.7%) were resistant to at least one antimicrobial agents, and one strain was resistance to 11 antimicrobial agents tested. Among these strains, resistance to three antimicrobial agents (21.1%) was most frequent, and the frequency of MDR E. coli isolates was 61.0% (Figure 1B and Supplementary Table S2). Similarly, a high proportion of MDR E. coli in human isolates was also described previously in the United States (Tadesse et al., 2012). These isolates were most frequently resistance to AMP (n = 83, 67.5%), SXT (n = 63, 53.7%), and TET (n = 79, 64.2%). These isolates were most susceptible to IMP and the resistance rate to IMP was only 0.8%. Furthermore, nine isolates (7.3%) had higher MIC values of PB (4 or 8 mg/L) than the rest of the isolates (MIC < 2.0 mg/L) (Supplementary Table S2). The prevalence of colistin resistance in clinical isolates found in this study is higher than the previous reports (usually lower than 1%) (Gales et al., 2011; Lu et al., 2018). In China, colistin has been widely used for the treatment of diarrhea in food-producing animals for decades, especially pigs and poultry. The transmission of colistin resistance gene mcr-1 has been reported to be associated with the food chain (Zurfluh et al., 2017). Thus, the high prevalence of mcr-1 in these diarrheal patients might be associated with food-producing animals. Our analysis showed that these nine colistin resistance strains were all MDR isolates, with resistance to at least three different antimicrobial agents. Nevertheless, eight out of the nine colistin resistance strains were susceptible to IMP, suggesting that IMP could be used to treat infection caused by colistin resistance E. coli.
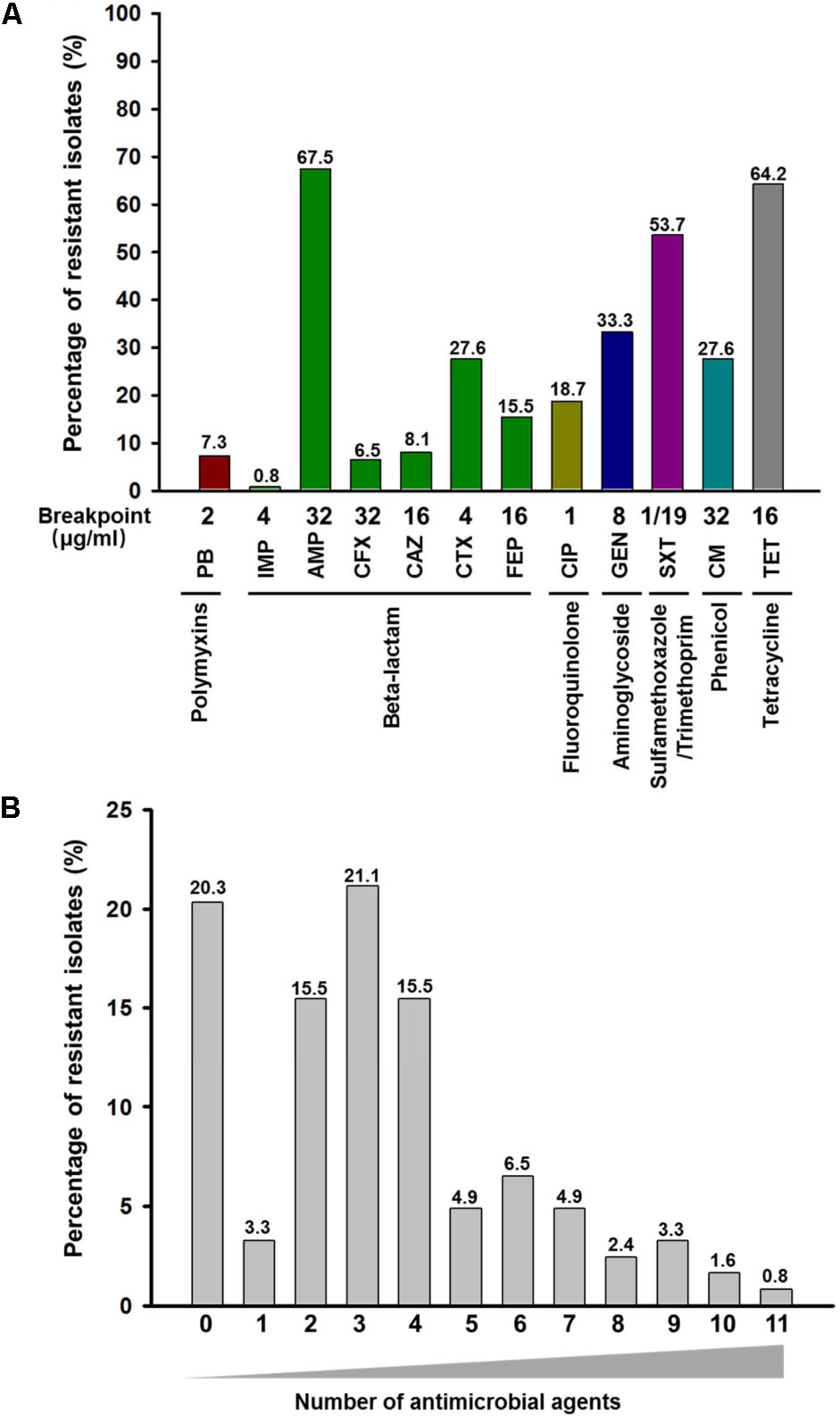
FIGURE 1. Frequency and distribution of resistance to antimicrobial agents among 123 E. coli isolates obtained from clinical specimens in China. (A) Frequency of resistance to each antimicrobial agent among 123 E. coli isolates. The number on top of each column represents the percentage of resistant isolates to each antimicrobial agent. PB, polymyxin B; IMP, imipenem; AMP, ampicillin; CFX, cefoxitin; CAZ, ceftazidime; CTX, cefotaxime; FEP, cefepime; CIP, ciprofloxacin; GEN, gentamycin; SXT, sulfamethoxazole-trimethoprim; CM, chloramphenicol; TET, tetracycline. (B) Occurrence of multidrug resistance among 123 E. coli isolates. The x-axis indicates the number of antimicrobial agents. The number on top of each column represents the percentage of resistant isolates to different numbers of antimicrobial agents.
The relatively low detection of IMP resistance in clinical E. coli isolates of Guangzhou (China) is consistent with previous report in Germany (Falgenhauer et al., 2016) and Vietnam (Hoang et al., 2017), respectively. However, the distribution of other types of resistance detected this study is different from the clinical E. coli isolates obtained in the United States (Karlowsky et al., 2002; Tadesse et al., 2012; Lob et al., 2016). It has been suggested that differences in resistance profile of geographically distinct regions maybe caused by the different use of antimicrobial agents in these regions (Gupta et al., 2001; Sannes et al., 2004).
Prevalence and Genetic Source of Colistin Resistance in Clinical E. coli Isolates
We first used PCR screening to detect the presence of the mcr-1 gene in these 123 isolates, and 7 isolates (5.7%) were positive for mcr-1 (Supplementary Figure S1). Among the nine isolates with higher MIC values of PB, six of them were detected to have the mcr-1 gene by PCR screening but three of them without the mcr-1 gene. To screen other mcr genes among three colistin-resistant mcr-1-negative isolates, whole-genome sequencing was performed. Sequencing analysis revealed that these three colistin-resistant isolates harbored no mcr genes using sequences of the eight mcr-1 related genes. Among the three isolates, strains of 14EC035 and 14EC043 exhibit similar MIC values of PB as the mcr-1 positive strains. However, 14EC045 exhibits a much higher MIC value of PB (>32 mg/L) than the MCR-mediated colistin resistance. Sequencing of the pmrA, pmrB, pmrC, pmrD, mgrB, phoP, and phoQ was performed using whole genome sequencing and two chromosomal mutation in PhoQ [Glu to Asp at amino acid position 464 (E464D) and Ala to Thr at amino acid position 482 (A482T)] were detected in 14EC045. E464D and A482T mutations in phoQ previously observed by Delannoy et al. (2017) in colistin resistance mcr-1 negative E. coli isolates. Thus, we suggested that two mutations in the chromosomal encoded gene phoQ might be responsible for the colistin resistance in E. coli 14EC045.
To further determine the distribution of mcr genes and to avoid misassemble cause by shufflons, the genomes of these seven isolates were determined using a combination of Illumina HiSeq 4000 sequencing technology and SMRT (also known as PacBio RS II system) sequencing technology. The genome features of the seven mcr-1 positive isolates are summarized in Table 1. Sequencing analysis revealed that these seven isolates all carried at least one copy of the mcr-1 gene, and the mcr-1 genes were most carried on plasmids, which are the primary vehicles for the dissemination of antibiotic resistance genes (Carattoli, 2013). Since the discovery of the mcr-1 gene in China in 2015 (Liu et al., 2016), mcr-1 positive strains have been found in different Enterobacteriaceae from various sources worldwide (Poirel et al., 2017). Furthermore, mcr-1 has been found on plasmids representing a diverse range of incompatibility groups. In particular, mcr-1 carrying plasmids belonging to the IncI2, IncHI2, and IncX4 families account for the majority of such sequences submitted to GenBank (Snesrud et al., 2016). In this study, a total of eight mcr-1 carrying plasmids were recovered, with the isolate 14EC033 yielding two distinct mcr-1 carrying plasmids. The eight mcr-1 positive plasmids (30–70 kb in size) included five IncI2 type, two IncX4 type and one IncP1 type. The mcr-1 genes on the eight plasmids shared 100% sequence identity with that of pHNSHP45 (Figure 2). A BLASTN comparison was carried out for the eight mcr-1 carrying plasmids identified in this study and the corresponding plasmids reported previously (Figure 2). All of the Incl2-type plasmids share a high homology with pHNSHP45. The IncP1-type plasmid of p14EC001a shared high similarity (100% coverage and 99% identity) with the plasmid pMCR_1511 (KX377410.1), which was isolated from Klebsiella pneumoniae WCHKP1511 (Zhao et al., 2017). In contrast, the IncX4-type plasmids of p14EC007a and p14EC033b shared 99% identity with the plasmid pColR598_1 of E. coli ColR598 (MF175190.1), which was recently described in Switzerland (Zurfluh et al., 2017).
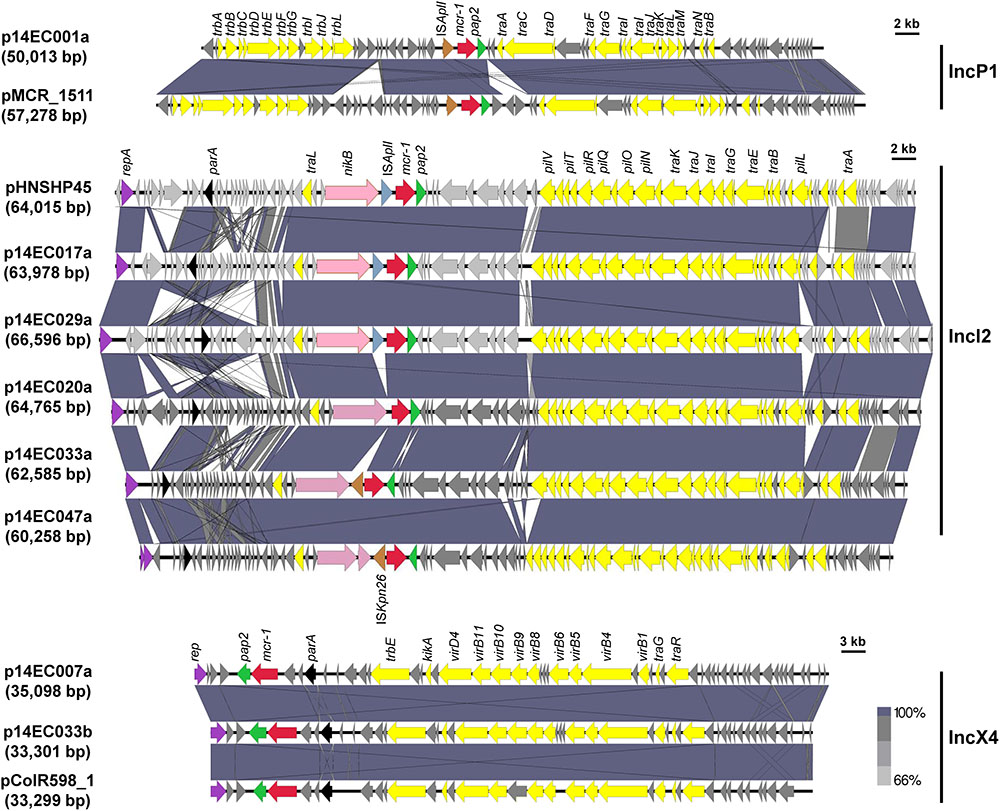
FIGURE 2. Sequence alignment of the eight mcr-1 carrying plasmids and pMCR_1511, pHNSHP45, or pColR598_1. Arrows show the directions of putative open reading frames (ORFs), and the length of the arrow is proportional to the size of each ORF. The gene mcr-1 is marked in red. ISApl1, ISKpn26, and pap2 gene are marked in blue gray, brown, and green. Nickel transport system permease gene (nikA), replication initiation protein (repA), and partition protein (parA) are marked in pink, purple, and black. Conjugal transfer genes are marked in yellow. Regions of homology between sequences (>66%) are indicated by the graded shading.
Moreover, isolate 14EC001 contains two copies of the mcr-1 gene, with one on an IncP1-type plasmid (p14EC001a) and the other one on the host chromosome (Figure 3 and Supplementary Table S3). Comparative analysis revealed that the sequence of mcr-1-pap2 region (2,600 bp in length) inserted in the host chromosome of 14EC001 is identical to a region in the plasmid p14EC001a of isolate 14EC001 (Supplementary Figure S2A). This insertion region contains one copy of the mcr-1 gene and its downstream gene pap2 and is inserted inside the rhmT gene in the host chromosome of 14EC001. Gene rhmT encodes a putative L-rhamnonate transporter which is a member of the major facilitator superfamily (MFS) and is likely to be in the rhmRDTA operon. MFS is one of the two largest families of membrane transporters found on Earth and ubiquitously distributed in bacteria, archaea, and eukarya (Reddy et al., 2012). Furthermore, rhmT and the rhmRDTA operon, without the mcr-1-pap2 region, are highly conserved among E. coli strains including the commensal E. coli K-12 strain (Supplementary Figure S2A). PCRs using primers flanking the rhmT gene further confirmed the presence of an extra 2.6 kb region in the coding region of rhmT in strain 14EC001 but not in other E. coli strains (Supplementary Figure S2B). To investigate whether chromosome-encoded mcr-1 is still functional, we amplified a 1983-bp DNA fragment containing the mcr-1 gene and its 357 bp upstream region using the genomic DNA of 14EC001 as a template and cloned it into pUC19 (pUC19-mcr-1 chromosome) (Supplementary Figure S2C); the resistance of the E. coli K-12 transformant to PB was determined. The results showed that the transformant with the mcr-1 gene from the chromosome exhibited increased resistance to PB, with its MIC increasing from <0.5 mg/L (empty pUC19) to 4.0 mg/L (pUC19-mcr-1 chromosome). The same MIC value of PB was obtained when a 2827-bp DNA fragment containing the mcr-1 gene and its 1274 bp upstream region (including ISApl1 element) was amplified using p14EC001a as a template and cloned into pUC19 (pUC19-mcr-1 plasmid) (Supplementary Figure S2C). Indeed, strain 14EC001 exhibited the highest MIC of PB (8 mg/L), while the MICs of the other the PB-resistant isolates were all 4 mg/L (Supplementary Table S2).
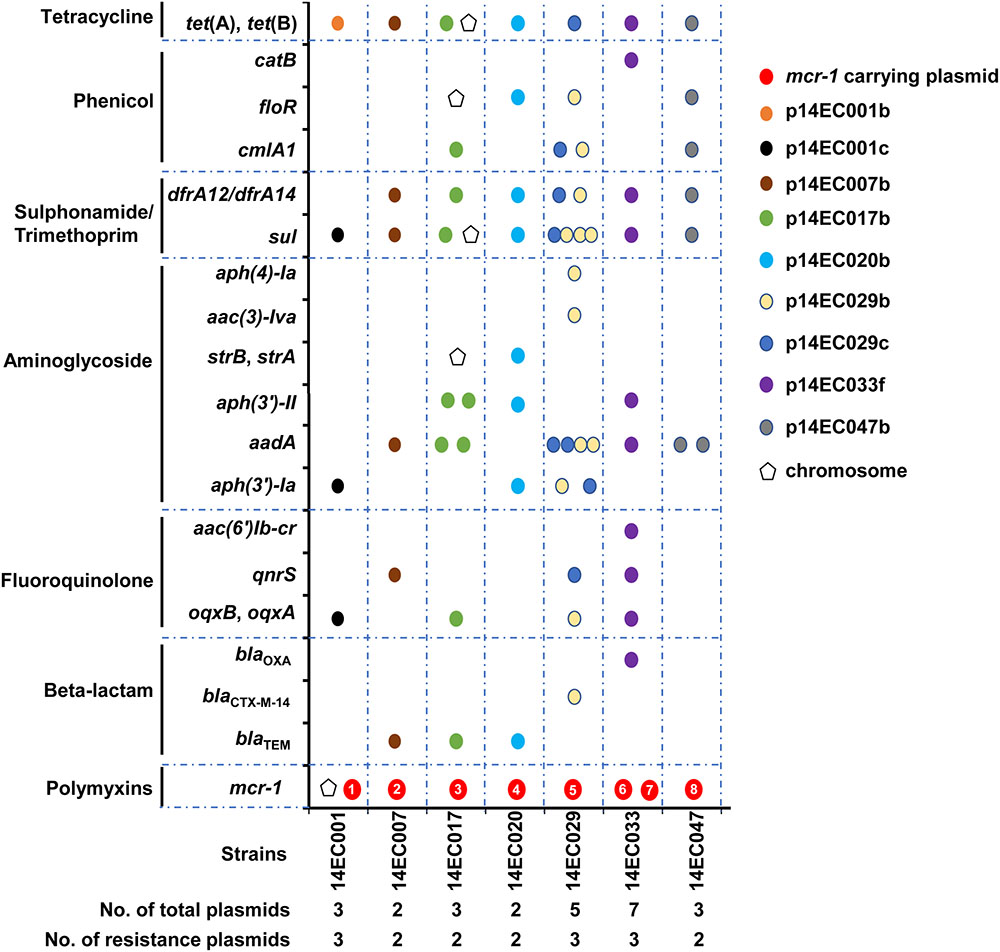
FIGURE 3. Distribution of the antimicrobial resistance gene is indicated by pentagon (chromosome) or circle (plasmid) in the seven mcr-1 positive strains. The eight mcr-1 carrying plasmids are indicated by red circles and are numbered as 1–8 for p14EC001a, p14EC007a, p14EC017a, p14EC020a, p14EC029a, p14EC033a, p14EC033b, and p14EC047a, respectively.
One mcr-1 Positive Isolate Does Not Express the Colistin-Resistance Phenotype
Unexpectedly, one mcr-1 positive isolate, 14EC047, is susceptible to PB (MIC < 0.5 mg/L). Isolate 14EC047 harbored three different plasmids including one mcr-1 charring plasmid. Genetic analysis showed that insertion elements were found in four mcr-1 carrying plasmids. In plasmids of p14EC017a and p14EC029a, a single copy of ISApl1 is located upstream of mcr-1. In plasmids of p14EC047a and p14EC033a, a single copy of ISKpn26 is located upstream of mcr-1 (Figure 2). To test whether the presence of the ISKpn26 affects mcr-1 activity, we cloned the mcr-1 gene and the promoter region containing ISKpn26 from 14EC047 into pUC19 vector to make pUC19-mcr-1. When electroplated into E. coli K-12 host, the transformant exhibited increased colistin resistance (MIC = 4.0 mg/L), suggesting that ISKpn26 does not affect the colistin resistance conferred by MCR-1 (Supplementary Figure S3). This is consistent with a recent report that the insertion of ISKpn26 to the upstream of mcr-1 in E. coli isolates ZJ148 and ZJ1653 did not affect the colistin resistance (Shen et al., 2018). We then reasoned that the host strain 14EC047 might suppress the activity of MCR-1. Next, we cloned the mcr-1 gene from pHNSHP45 into pEX18Gm vector to construct pEX18Gm-mcr-1. As expected, transformant E. coli K-12/pEX18Gm-mcr-1 exhibited increased colistin resistance (MIC = 4.0 mg/L). In contrast, transformant 14EC047/pEX18Gm-mcr-1 was susceptible to colistin (MIC < 0.5 mg/L) (Supplementary Figure S3). This result showed that the colistin resistance conferred by MCR-1 was abolished in 14EC047. Further investigation is needed to elucidate the underlying mechanism. Since high expression of mcr-1 in E. coli could result in reduced growth rate and competitive ability during infection, mcr-1 silencing might be a compensatory adaptation in pathogenic isolates (Yang et al., 2017).
Distribution of the Virulence Factors in mcr-1 Positive Isolates
The E. coli isolates analyzed in this study were recovered from diarrheal patients in China and diarrhea is one of the major clinical manifestations of E. coli (Gibbs et al., 2004). MLST analysis assigned these seven mcr-1-positive isolates to five distinct sequence types (STs), with three isolates belonging to ST301 and the rest of them belonging to ST793, ST117, ST88, and ST2064. ST117 and ST88 have been previously reported in mcr-1 positive pathogenic E. coli (Maluta et al., 2014; Manges et al., 2015). However, ST301, ST793, and ST2064 were not reported previously in mcr-1 positive E. coli isolates. ST10 are widely distributed in human diarrheagenic E. coli infection in Hangzhou of China (Yu et al., 2018), but in this study, we found ST301 was more common in mcr-1 positive diarrheagenic E. coli infection in Guangzhou of China from year 2013–2016.
Next, the distribution of virulence factors were then analyzed through in silico analysis (see footnote 4) among the seven mcr-1 positive isolates (Table 1). A total of 26 different virulence genes were identified among these mcr-1 positive isolates, and all seven mcr-1 positive isolates were positive for the gad gene which encodes protein involved in the glutamate decarboxylase (GAD) system. The GAD system is important for E. coli to survive in low pH conditions such as passage through the stomach after ingestion (Lin et al., 1996). Three isolates (14EC007, 14EC017, and 14EC047) belonging to ST301 all carried virulence genes including nleB, espF, espA, eae, tir, katP, and etpD besides gad. Previous studies have suggested that virulence factors such as eae, nleA, nleB, nleC, lpfA genes, which are associated with diarrhea were present in these isolates (Menard and Dubreuil, 2002; Afset et al., 2006). Two isolates (14EC001 belonging to ST793 and 14EC029 belonging to ST88) carried genes related to enterotoxin such as astA, ltcA, and stb. Enterotoxin is a major cause of diarrhea in infants in developing countries and in travelers to those regions (Gupta et al., 2008). Additionally, isolate 14EC020 of ST117 carried virulence factors including gad, iss, lpfA, ireA, hlyE, iroN, and cma. This study showed that mcr-1 positive isolates possessed a broad range of virulence factors.
Distribution of the Antimicrobial Resistance Genes in mcr-1 Positive Isolates
Identification of resistance genes in the genome of the seven isolates was performed using ResFinder from the Center for Genomic Epidemiology (see footnote 3). In addition to the seven classes of antibiotic agents tested in the custom-made 96-well MIC panel, resistance genes related to rifampicin, MLS, and fosfomycin resistance were also determined. As shown in Figure 3, all isolates harbored multiple plasmids (up to seven plasmids), and all isolates harbored two to three plasmids with antibiotic resistance genes. Mobile genetic elements, particularly plasmids, are associated with the acquisition and dissemination of antimicrobial resistance genes in E. coli (Schroeder et al., 2004; Kadlec and Schwarz, 2008). When carried on transposons and plasmids, the resistance genes could be transmitted both intraspecies and interspecies (Galani et al., 2010; Huang et al., 2012). Our studies demonstrated that most of the resistance genes detected were carried on plasmids. In particular, except for mcr-1 and tet (B) genes, many resistance genes were located within one single plasmid even in the strain harboring multiple plasmids. Moreover, all the mcr-1 carrying plasmids only encode resistance to polymyxin. Isolates 14EC007 and 14EC020 each harbored two plasmids and showed resistance to six different classes of antimicrobial agents, with the mcr-1 gene located on one plasmid and all of the rest resistance genes on the other plasmid. Isolates 14EC029 and 14EC033 harbored the highest number of plasmids and the highest number of resistance genes. These two isolates each harbored one IncI2 type mcr-1 carrying plasmid. Additionally, the two plasmids in 14EC029 carried 9 and 15 resistance genes, respectively, and while no resistance gene was found in the rest of the two plasmids. Similarly, isolate 14EC033 harbored one IncX4 type mcr-1 carrying plasmid and another plasmid carrying 11 resistance genes including AAR-3 gene conferring resistance to rifampicin. None of the resistance genes was identified in the rest of the four plasmids in 14EC033 (Figure 3 and Supplementary Table S3). One exception is plasmid p14EC001b in isolate 14EC001, which only carried tet (B) gene encoding TET resistance but the rest of the TET resistance genes were clustered with other resistance genes in other plasmids.
In terms of the distribution of the resistance genes in these isolates, in addition to resistance to polymyxin, all of these strains carry genes encoding resistance to aminoglycoside, SXT, and TET. Genes encoding various extended spectrum β-lactamases were identified in five (but not in 14EC001 and 14EC047) of the seven mcr-1 positive isolates, exhibiting resistant to at least one antimicrobial agent within this class. Although six of seven mcr-1 positive isolates were resistant to AMP, only one of these isolates showed resistance to other antibiotics tested of this class. Isolate 14EC029, which was positive for blaCTX-M-14, was determined to be resistant to AMP and CTX. The tet (B) or tet (A) was present in the seven mcr-1 positive isolates, all displaying TET resistance. Additionally, all seven mcr-1 positive isolates resistance to SXT, since these isolates carried trimethoprim resistance gene dfrA12 or dfrA14, and sulfamethoxazole resistance gene sul3 or sul2 (except for 14EC001 carried sul3 but no trimethoprim resistance gene). Six mcr-1 positive isolates exhibited resistance to CM, but only five isolates were positive for cmlA1, floR, floR2, or catB encoding CM resistance. Isolate 14EC001 displaying the resistance to CM without cml or floR or catB determinants carried oqxAB gene, and a previous study showed that oqxAB could also confer the resistance to CM (Hansen et al., 2004). Six of seven mcr-1 positive isolates present increased resistance to CIP. Among them, five isolates carried qnrS, aac(6′)Ib-cr, oqxB, oqxA determinants and one isolate (14EC020) had a chromosomal mutation in GyrA (Ser to Ala at amino acid position 83) that is known to cause ciprofloxacin resistance (Vila et al., 1994). Resistance to gentamicin was mediated mainly by the aac (3′) gene (Ramirez and Tolmasky, 2010), which is present in four of seven mcr-1 positive isolates and displaying gentamicin resistance aac(6′)Ib-cr gene responsible for resistance to the aminoglycosides kanamycin, but susceptibility to gentamicin (Robicsek et al., 2006).
Furthermore, our analysis in the clinical isolates in China revealed that mcr-1 is the only resistance gene found on these mcr-1 carrying plasmids. These plasmids do not encode identified virulence factors. Recent studies demonstrated that increased expression of mcr-1 in E. coli results in decreased bacterial growth, bacterial cell membrane impairment and attenuated virulence in animal infection model, suggesting that the expression of the mcr-1 gene should be tightly regulated in E. coli (Yang et al., 2017). Furthermore, co-occurrence of mcr-1 and other antimicrobial resistance genes on the same plasmid has been reported earlier (Bai et al., 2016; Haenni et al., 2016; Malhotra-Kumar et al., 2016; Yang et al., 2016; Zhi et al., 2016; Hadjadj et al., 2017; Zheng et al., 2017). However, these plasmids are usually larger than 200 kb and contain multiple plasmid replicons with more than one replication proteins (Supplementary Table S4). Previous studies have suggested that megaplasmids (>100 kb) are usually fused by smaller plasmids (Zheng et al., 2013). For example, plasmid pHNSHP45-2, which was isolated from porcine E. coli strain SHP45 where the first mcr-1 gene was reported in 2015 is 251 kb (Zhi et al., 2016). Plasmid pHNSHP45 (64 kb), which was also present in E. coli strain SHP45 is the first reported mcr-1 carrying plasmid and it contains only this resistance gene. Although mcr-1 is the only resistance gene on mcr-1 carrying plasmid in E. coli isolates obtained from 2013 to 2016 in this study, fusion of mcr-1 carrying plasmid with other plasmids carrying multiple resistance genes in the future would promote the co-transfer of these antimicrobial resistance genes. Several studies have documented a link between the antimicrobial use and the development of antimicrobial resistance (Goossens et al., 2005; Bergman et al., 2009). Inappropriate use of one of these antimicrobial agents would potentially accelerate the co-transfer of several resistance genes. This emphasizes the need for ongoing monitoring of resistance patterns to ensure appropriate antibiotic use by clinicians.
Author Contributions
HZ and BL conceptualized and designed the project. BL, XW, BK, XZ, WW, and YG did investigation, data curation, and data analysis. BL, XW, and HZ did supervision and visualization. BL, XW, and HZ wrote, reviewed, and edited the original draft.
Funding
This work was supported by the National Science Foundation of China (Grant Nos. 31290233, 41230962, 41406189, and 31470141), the Chinese Academy of Sciences (Grant No. XDA11030402), the China Postdoctoral Science Foundation (Grant No. 2017M622649), and the Science and Technology Innovation Leading Talent Program of Guangdong Province (Grant No. 2015TX01N036). XW is the recipient of the 1000-Youth Elite Program award (a program for the recruitment of global experts to China).
Conflict of Interest Statement
The authors declare that the research was conducted in the absence of any commercial or financial relationships that could be construed as a potential conflict of interest.
Supplementary Material
The Supplementary Material for this article can be found online at: https://www.frontiersin.org/articles/10.3389/fmicb.2018.02514/full#supplementary-material
Footnotes
- ^http://soap.genomics.org.cn/
- ^http://www.cbcb.umd.edu/software/glimmer/
- ^http://www.genomicepidemiology.org/
References
AbuOun, M., Stubberfield, E. J., Duggett, N. A., Kirchner, M., Dormer, L., Nunez-Garcia, J., et al. (2017). mcr-1 and mcr-2 variant genes identified in Moraxella species isolated from pigs in Great Britain from 2014 to 2015. J. Antimicrob. Chemother. 72, 2745–2749. doi: 10.1093/jac/dkx286
Afset, J. E., Bruant, G., Brousseau, R., Harel, J., Anderssen, E., Bevanger, L., et al. (2006). Identification of virulence genes linked with diarrhea due to atypical enteropathogenic Escherichia coli by DNA microarray analysis and PCR. J. Clin. Microbiol. 44, 3703–3711. doi: 10.1128/Jcm.00429-06
Bai, L., Hurley, D., Li, J., Meng, Q., Wang, J., Fanning, S., et al. (2016). Characterisation of multidrug-resistant Shiga toxin-producing Escherichia coli cultured from pigs in China: co-occurrence of extended-spectrum beta-lactamase- and mcr-1-encoding genes on plasmids. Int. J. Antimicrob. Agents 48, 445–448. doi: 10.1016/j.ijantimicag.2016.06.021
Bergman, M., Nyberg, S. T., Huovinen, P., Paakkari, P., Hakanen, A. J., and Re, F. S. G. A. (2009). Association between antimicrobial consumption and resistance in Escherichia coli. Antimicrob. Agents Chemother. 53, 912–917. doi: 10.1128/Aac.00856-08
Biswas, S., Brunel, J. M., Dubus, J. C., Reynaud-Gaubert, M., and Rolain, J. M. (2012). Colistin: an update on the antibiotic of the 21st century. Expert Rev. Anti Infect. Ther. 10, 917–934. doi: 10.1586/eri.12.78
Borowiak, M., Fischer, J., Hammerl, J. A., Hendriksen, R. S., Szabo, I., and Malorny, B. (2017). Identification of a novel transposon-associated phosphoethanolamine transferase gene, mcr-5, conferring colistin resistance in d-tartrate fermenting Salmonella enterica subsp enterica serovar Paratyphi B. J. Antimicrob. Chemother. 72, 3317–3324. doi: 10.1093/jac/dkx327
Carattoli, A. (2013). Plasmids and the spread of resistance. Int. J. Med. Microbiol. 303, 298–304. doi: 10.1016/j.ijmm.2013.02.001
Carattoli, A., Villa, L., Feudi, C., Curcio, L., Orsini, S., Luppi, A., et al. (2017). Novel plasmid-mediated colistin resistance mcr-4 gene in Salmonella and Escherichia coli, Italy 2013, Spain and Belgium, 2015 to 2016. Euro. Surveill. 22, 18–22. doi: 10.2807/1560-7917.ES.2017.22.31.30589
Chang, D. E., Smalley, D. J., Tucker, D. L., Leatham, M. P., Norris, W. E., Stevenson, S. J., et al. (2004). Carbon nutrition of Escherichia coli in the mouse intestine. Proc. Natl. Acad. Sci. U.S.A. 101, 7427–7432. doi: 10.1073/pnas.0307888101
Clinical and Laboratory Standards Institute (2017). Performance Standards for Antimicrobial Susceptibility Testing, 26th Edn. Wayne, PA: CLSI
Delannoy, S., Le Devendec, L., Jouy, E., Fach, P., Drider, D., and Kempf, I. (2017). Characterization of colistin-resistant Escherichia coli isolated from diseased pigs in France. Front. Microbiol. 8:2278. doi: 10.3389/fmicb.2017.02278
Falgenhauer, L., Waersada, S. E., Yao, Y. C., Imirzalioglu, C., Kasbohrer, A., Roesler, U., et al. (2016). Colistin resistance gene mcr-1 in extended-spectrum beta-lactamase-producing and carbapenemase-producing Gram-negative bacteria in Germany. Lancet Infect. Dis. 16, 282–283. doi: 10.1016/S1473-3099(16)00009-8
Finegold, S. M., Sutter, V. L., and Mathisen, G. E. (1983). Human Intestinal Microflora in Health and Disease, ed. D. J. Hentges (New York, NY: Academic Press), 3–31.
Galani, I., Souli, M., Mitchell, N., Chryssouli, Z., and Giamarellou, H. (2010). Presence of plasmid-mediated quinolone resistance in Klebsiella pneumoniae and Escherichia coli isolates possessing bla(VIM-1) in Greece. Int. J. Antimicrob. Agents 36, 252–254. doi: 10.1016/j.ijantimicag.2010.05.004
Gales, A. C., Jones, R. N., and Sader, H. S. (2011). Contemporary activity of colistin and polymyxin B against a worldwide collection of Gram-negative pathogens: results from the SENTRY Antimicrobial Surveillance Program (2006-09). J. Antimicrob. Chemother. 66, 2070–2074. doi: 10.1093/jac/dkr239
Gibbs, P. S., Petermann, S. R., and Wooley, R. E. (2004). Comparison of several challenge models for studies in avian colibacillosis. Avian Dis. 48, 751–758. doi: 10.1637/7176-030404R
Goossens, H., Ferech, M., Stichele, R. V., Elseviers, M., and Grp, E. P. (2005). Outpatient antibiotic use in Europe and association with resistance: a cross-national database study. Lancet 365, 579–587. doi: 10.1016/S0140-6736(05)70799-6
Gupta, K., Sahm, D. F., Mayfield, D., and Stamm, W. E. (2001). Antimicrobial resistance among uropathogens that cause community-acquired urinary tract infections in women: a nationwide analysis. Clin. Infect. Dis. 33, 89–94. doi: 10.1086/320880
Gupta, S. K., Keck, J., Ram, P. K., Crump, J. A., Miller, M. A., and Mintz, E. D. (2008). Part III. analysis of data gaps pertaining to enterotoxigenic Escherichia coli infections in low and medium human development index countries, 1984-2005. Epidemiol. Infect. 136, 721–738. doi: 10.1017/S095026880700934x
Hadjadj, L., Riziki, T., Zhu, Y., Li, J., Diene, S. M., and Rolain, J. M. (2017). Study of mcr-1 gene-mediated colistin resistance in Enterobacteriaceae isolated from humans and animals in different countries. Genes 8:394. doi: 10.3390/genes8120394
Haenni, M., Poirel, L., Kieffer, N., Chatre, P., Saras, E., Metayer, V., et al. (2016). Co-occurrence of extended spectrum beta lactamase and MCR-1 encoding genes on plasmids. Lancet Infect. Dis. 16, 281–282. doi: 10.1016/S1473-3099(16)00007-4
Hansen, L. H., Johannesen, E., Burmolle, M., Sorensen, A. H., and Sorensen, S. J. (2004). Plasmid-encoded multidrug efflux pump conferring resistance to olaquindox in Escherichia coli. Antimicrob. Agents Chemother. 48, 3332–3337. doi: 10.1128/AAC.48.9.3332-3337.2004
Hoang, P. H., Awasthi, S. P., Do Nguyen, P., Nguyen, N. L. H., Nguyen, D. T. A., Le, N. H. L., et al. (2017). Antimicrobial resistance profiles and molecular characterization of Escherichia coli strains isolated from healthy adults in Ho Chi Minh City, Vietnam. J. Vet. Med. Sci. 79, 479–485. doi: 10.1292/jvms.16-0639
Huang, S. Y., Zhu, X. Q., Wang, Y., Liu, H. B., Dai, L., He, J. K., et al. (2012). Co-carriage of qnrS1, floR, and bla(CTX-M-14) on a multidrug-resistant plasmid in Escherichia coli isolated from pigs. Foodborne Pathog. Dis. 9, 896–901. doi: 10.1089/fpd.2012.1131
Kadlec, K., and Schwarz, S. (2008). Analysis and distribution of class 1 and class 2 integrons and associated gene cassettes among Escherichia coli isolates from swine, horses, cats and dogs collected in the BfT-GermVet monitoring study. J. Antimicrob. Chemother. 62, 469–473. doi: 10.1093/jac/dkn233
Karlowsky, J. A., Kelly, L. J., Thornsberry, C., Jones, M. E., and Sahm, D. F. (2002). Trends in antimicrobial resistance among urinary tract infection isolates of Escherichia coli from female outpatients in the United States. Antimicrob. Agents Chemother. 46, 2540–2545. doi: 10.1128/AAC.46.8.2540-2545.2002
Lin, J. S., Smith, M. P., Chapin, K. C., Baik, H. S., Bennett, G. N., and Foster, J. W. (1996). Mechanisms of acid resistance in enterohemorrhagic Escherichia coli. Appl. Environ. Microbiol. 62, 3094–3100.
Liu, Y. Y., Wang, Y., Walsh, T. R., Yi, L. X., Zhang, R., Spencer, J., et al. (2016). Emergence of plasmid-mediated colistin resistance mechanism MCR-1 in animals and human beings in China: a microbiological and molecular biological study. Lancet Infect. Dis. 16, 161–168. doi: 10.1016/s1473-3099(15)00424-7
Lob, S. H., Nicolle, L. E., Hoban, D. J., Kazmierczak, K. M., Badal, R. E., and Sahm, D. F. (2016). Susceptibility patterns and ESBL rates of Escherichia coli from urinary tract infections in Canada and the United States, SMART 2010-2014. Diagn. Microbiol. Infect. Dis. 85, 459–465. doi: 10.1016/j.diagmicrobio.2016.04.022
Lu, H., Wang, C., Dong, G., Xu, C., Zhang, X., Liu, H., et al. (2018). Prevalence and molecular characterization of Escherichia coli clinical isolates carrying mcr-1 in a Chinese teaching hospital from 2002 to 2016. Antimicrob. Agents Chemother. 62:e02623-17. doi: 10.1128/AAC.02623-17
Malhotra-Kumar, S., Xavier, B. B., Das, A. J., Lammens, C., Butaye, P., and Goossens, H. (2016). Colistin resistance gene mcr-1 harboured on a multidrug resistant plasmid. Lancet Infect. Dis. 16, 283–284. doi: 10.1016/S1473-3099(16)00012-8
Maluta, R. P., Logue, C. M., Casas, M. R. T., Meng, T., Guastalli, E. A. L., Rojas, T. C. G., et al. (2014). Overlapped sequence types (STs) and serogroups of avian pathogenic (APEC) and human extra-intestinal pathogenic (ExPEC) Escherichia coli isolated in Brazil. PLoS One 9:e105016. doi: 10.1371/journal.pone.0105016
Manges, A. R., Harel, J., Masson, L., Edens, T. J., Portt, A., Reid-Smith, R. J., et al. (2015). Multilocus sequence typing and virulence gene profiles associated with Escherichia coli from human and animal sources. Foodborne Pathog. Dis. 12, 302–310. doi: 10.1089/fpd.2014.1860
Menard, L. P., and Dubreuil, J. D. (2002). Enteroaggregative Escherichia coli heat-stable enterotoxin 1 (EAST1): a new toxin with an old twist. Crit. Rev. Microbiol. 28, 43–60. doi: 10.1080/1040-840291046687
Nataro, J. P., and Kaper, J. B. (1998). Diarrheagenic Escherichia coli. Clin. Microbiol. Rev. 11, 142–201.
Novick, R. P. (1981). The development and spread of antibiotic resistant bacteria as a consequence of feeding antibiotics to livestock. Ann. N. Y. Acad. Sci. 368, 23–59. doi: 10.1111/j.1749-6632.1981.tb15430.x
Poirel, L., Jayol, A., and Nordmann, P. (2017). Polymyxins: antibacterial activity, susceptibility testing, and resistance mechanisms encoded by plasmids or chromosomes. Clin. Microbiol. Rev. 30, 557–596. doi: 10.1128/CMR.00064-16
Ramirez, M. S., and Tolmasky, M. E. (2010). Aminoglycoside modifying enzymes. Drug Resist. Updat. 13, 151–171. doi: 10.1016/j.drup.2010.08.003
Reddy, V. S., Shlykov, M. A., Castillo, R., Sun, E. I., and Saier, M. H. (2012). The major facilitator superfamily (MFS) revisited. FEBS J. 279, 2022–2035. doi: 10.1111/j.1742-4658.2012.08588.x
Robicsek, A., Strahilevitz, J., Jacoby, G. A., Macielag, M., Abbanat, D., Park, C. H., et al. (2006). Fluoroquinolone-modifying enzyme: a new adaptation of a common aminoglycoside acetyltransferase. Nat. Med. 12, 83–88. doi: 10.1038/nm1347
Russo, T. A., and Johnson, J. R. (2003). Medical and economic impact of extraintestinal infections due to Escherichia coli: focus on an increasingly important endemic problem. Microbes Infect. 5, 449–456. doi: 10.1016/S1286-4579(03)00049-2
Sannes, M. R., Kuskowski, M. A., and Johnson, J. R. (2004). Geographical distribution of antimicrobial resistance among Escherichia coli causing acute uncomplicated pyelonephritis in the United States. FEMS Immunol. Med. Microbiol. 42, 213–218. doi: 10.1016/j.femsim.2004.05.004
Schroeder, C. M., White, D. G., and Meng, J. H. (2004). Retail meat and poultry as a reservoir of antimicrobial-resistant Escherichia coli. Food Microbiol. 21, 249–255. doi: 10.1016/S0740-0020(03)00074-1
Sekizuka, T., Kawanishi, M., Ohnishi, M., Shima, A., Kato, K., Yamashita, A., et al. (2017). Elucidation of quantitative structural diversity of remarkable rearrangement regions, shufflons, in IncI2 plasmids. Sci. Rep. 7:928. doi: 10.1038/s41598-017-01082-y
Shen, Y., Wu, Z., Wang, Y., Zhang, R., Zhou, H. W., Wang, S., et al. (2018). Heterogeneous and flexible transmission of mcr-1 in hospital-associated Escherichia coli. mBio 9:e00943-18. doi: 10.1128/mBio.00943-18
Snesrud, E., He, S., Chandler, M., Dekker, J. P., Hickman, A. B., McGann, P., et al. (2016). A model for transposition of the colistin resistance gene mcr-1 by ISApl1. Antimicrob. Agents Chemother. 60, 6973–6976. doi: 10.1128/aac.01457-16
Tadesse, D. A., Zhao, S. H., Tong, E., Ayers, S., Singh, A., Bartholomew, M. J., et al. (2012). Antimicrobial drug resistance in Escherichia coli from humans and food animals, United States, 1950-2002. Emerg. Infect. Dis. 18, 741–749. doi: 10.3201/eid1805.111153
Vila, J., Ruiz, J., Marco, F., Barcelo, A., Goni, P., Giralt, E., et al. (1994). Association between double mutation in gyra gene of ciprofloxacin-resistant clinical isolates of Escherichia coli and Mics. Antimicrob. Agents Chemother. 38, 2477–2479. doi: 10.1128/Aac.38.10.2477
Wang, X., Wang, Y., Zhou, Y., Li, J., Yin, W., Wang, S., et al. (2018). Emergence of a novel mobile colistin resistance gene, mcr-8, in NDM-producing Klebsiella pneumoniae. Emerg. Microbes Infect. 7:122. doi: 10.1038/s41426-018-0124-z
Weisburg, W. G., Barns, S. M., Pelletier, D. A., and Lane, D. J. (1991). 16S ribosomal DNA amplification for phylogenetic study. J. Bacteriol. 173, 697–703. doi: 10.1128/jb.173.2.697-703.1991
Wiles, T. J., Kulesus, R. R., and Mulvey, M. A. (2008). Origins and virulence mechanisms of uropathogenic Escherichia coli. Exp. Mol. Pathol. 85, 11–19. doi: 10.1016/j.yexmp.2008.03.007
Xavier, B. B., Lammens, C., Ruhal, R., Kumar-Singh, S., Butaye, P., Goossens, H., et al. (2016). Identification of a novel plasmid-mediated colistin-resistance gene, mcr-2, in Escherichia coli, Belgium, June 2016. Euro. Surveill. 21, 8–13. doi: 10.2807/1560-7917.ES.2016.21.27.30280
Yang, Q., Li, M., Spiller, O. B., Andrey, D. O., Hinchliffe, P., Li, H., et al. (2017). Balancing mcr-1 expression and bacterial survival is a delicate equilibrium between essential cellular defence mechanisms. Nat. Commun. 8:2054. doi: 10.1038/s41467-017-02149-0
Yang, Y. Q., Li, Y. X., Lei, C. W., Zhang, A. Y., and Wang, H. N. (2018). Novel plasmid-mediated colistin resistance gene mcr-7.1 in Klebsiella pneumoniae. J. Antimicrob. Chemother. 73, 1791–1795. doi: 10.1093/jac/dky111
Yang, Y. Q., Zhang, A. Y., Ma, S. Z., Kong, L. H., Li, Y. X., Liu, J. X., et al. (2016). Co-occurrence of mcr-1 and ESBL on a single plasmid in Salmonella enterica. J. Antimicrob. Chemother. 71, 2336–2338. doi: 10.1093/jac/dkw243
Yin, W. J., Li, H., Shen, Y. B., Liu, Z. H., Wang, S. L., Shen, Z. Q., et al. (2017). Novel plasmid-mediated colistin resistance gene mcr-3 in Escherichia coli. mBio 8:e00543-17. doi: 10.1128/mBio.00543-17
Yu, F., Chen, X., Zheng, S. F., Han, D. S., Wang, Y. Y., Wang, R. N., et al. (2018). Prevalence and genetic diversity of human diarrheagenic Escherichia coli isolates by multilocus sequence typing. Int. J. Infect. Dis. 67, 7–13. doi: 10.1016/j.ijid.2017.11.025
Zhao, F. F., Feng, Y., Lu, X. J., McNally, A., and Zong, Z. Y. (2017). IncP plasmid carrying colistin resistance gene mcr-1 in Klebsiella pneumoniae from hospital sewage. Antimicrob. Agents Chemother. 61:e02229-16. doi: 10.1128/AAC.02229-16
Zheng, B. W., Yu, X., Xu, H., Guo, L. H., Zhang, J., Huang, C., et al. (2017). Complete genome sequencing and genomic characterization of two Escherichia coli strains co-producing MCR-1 and NDM-1 from bloodstream infection. Sci. Rep. 7:17885. doi: 10.1038/s41598-017-18273-2
Zheng, J. S., Peng, D. H., Ruan, L. F., and Sun, M. (2013). Evolution and dynamics of megaplasmids with genome sizes larger than 100 kb in the Bacillus cereus group. BMC Evol. Biol. 13:262. doi: 10.1186/1471-2148-13-262
Zhi, C. P., Lv, L. C., Yu, L. F., Doi, Y. H., and Liu, J. H. (2016). Dissemination of the mcr-1 colistin resistance gene. Lancet Infect. Dis. 16, 292–293. doi: 10.1016/S1473-3099(16)00063-3
Keywords: multidrug-resistant, clinical isolates, Escherichia coli, mcr-1, plasmid
Citation: Li B, Ke B, Zhao X, Guo Y, Wang W, Wang X and Zhu H (2018) Antimicrobial Resistance Profile of mcr-1 Positive Clinical Isolates of Escherichia coli in China From 2013 to 2016. Front. Microbiol. 9:2514. doi: 10.3389/fmicb.2018.02514
Received: 09 May 2018; Accepted: 02 October 2018;
Published: 23 October 2018.
Edited by:
Fernando Baquero, Instituto Ramón y Cajal de Investigación Sanitaria, SpainReviewed by:
Ximin Zeng, The University of Tennessee, Knoxville, United StatesJian-Hua Liu, South China Agricultural University, China
Copyright © 2018 Li, Ke, Zhao, Guo, Wang, Wang and Zhu. This is an open-access article distributed under the terms of the Creative Commons Attribution License (CC BY). The use, distribution or reproduction in other forums is permitted, provided the original author(s) and the copyright owner(s) are credited and that the original publication in this journal is cited, in accordance with accepted academic practice. No use, distribution or reproduction is permitted which does not comply with these terms.
*Correspondence: Honghui Zhu, zhuhh@gdim.cn