- 1Institute of Plant Protection, Jiangsu Key Laboratory for Food Quality and Safety-State Key Laboratory Cultivation Base of Ministry of Science and Technology, Jiangsu Academy of Agricultural Sciences, Nanjing, China
- 2School of Medicine, Yangzhou Polytechnic College, Yangzhou, China
- 3Department of Biological Sciences, University of South Carolina, Columbia, SC, United States
- 4Institute of Botany, Jiangsu Province and Chinese Academy of Sciences, Nanjing, China
Xanthomonas oryzae pv. oryzae (Xoo) causes rice bacterial blight (BB), one of the most widespread and destructive diseases in rice-growing regions worldwide. Melatonin enhances pathogen resistance by inducing plant innate immunity, but the direct effect of melatonin on plant pathogenic bacteria is poorly understood. In this study, we investigated the direct effects of melatonin on Xoo. Exogenous melatonin at 200 μg/mL significantly inhibited the proliferation of Xoo and reduced the mRNA expression of five genes involved in cell division. This concentration of melatonin also inhibited the motility and biofilm formation of Xoo. Notably, melatonin was observed to alter the length of Xoo cells. To provide deeper insights into the mechanisms underlying this antibacterial activity, we examined global gene expression changes in Xoo strain PXO99 in response to the application of 200 μg/mL melatonin using RNA sequencing (RNA-Seq). A wide range of differentially expressed genes (DEGs) related to catalytic activity and metal-binding activity were downregulated in Xoo cells in response to the melatonin treatment. In addition, DEGs responsible for carbohydrate and amino acid metabolism were also downregulated. These results suggest that the inhibitory mechanism of melatonin on Xoo proliferation may involve the regulation of cell division in combination with a reduction in the concentration or activity of enzymes involved in metabolism.
Introduction
Melatonin (N-acetyl-5-methoxytryptamine) is a highly evolutionarily conserved molecule that exists in the microbe (Manchester et al., 2015), insect (Vivien-Roels et al., 1984), animal (Menendez-Pelaez and Reiter, 1993), and plant kingdoms (Dubbels et al., 1995). In animals, melatonin was discovered in the bovine pineal gland in 1958 (Lerner et al., 1958). This indoleamine is a well-known animal neurohormone involved in numerous cellular and physiological functions, such as sleep (Garfinkel et al., 1995), circadian rhythms (Jung-Hynes et al., 2010), stem cell differentiation (Radio et al., 2006), and scavenging of reactive oxygen species (ROS) and reactive nitrogen species (RNS) (Reiter et al., 2016). In plants, melatonin was simultaneously discovered by two research groups in 1995 (Dubbels et al., 1995; Hattori et al., 1995).
Since then, melatonin has been found in a variety of plant species (Kolar and Machackova, 2005). Plant melatonin is involved in many significant plant processes, including plant growth (Chen et al., 2009; Arnao and Hernández-Ruiz, 2017) and defence against both biotic (Vielma et al., 2014; Shi et al., 2016) and abiotic stresses (Byeon and Back, 2016; Zhang et al., 2017). In microbes, exogenous melatonin acts as a biocide against some fungi and bacteria (Wang et al., 2001; Hu et al., 2017). Melatonin shows antibacterial activity against Gram-positive and Gram-negative bacteria at a low concentration in vitro (Atroshi et al., 1998; Konar et al., 2000; Ozturk et al., 2000; Karakas et al., 2013). In vivo, the exogenous application of melatonin was shown to suppress PstDC3000 propagation in Arabidopsis leaves (Lee et al., 2014). Melatonin may prevent the uptake of free iron by bacteria (Limson et al., 1998; Tekbas et al., 2008), inhibit constitutive bacterial protein secretion (Bubis and Zisapel, 1995), and reduce intracellular substrates that are important for bacterial growth (Tekbas et al., 2008). However, the mechanisms underlying these inhibitory effects of melatonin on bacteria have been little studied.
Bacterial blight (BB) of rice caused by X. oryzae pv. oryzae (Xoo) is one of the most destructive diseases in most rice-growing countries, especially those in Asia (Mansfield et al., 2012). This disease leads to leaf blight during the growing season, hindering photosynthesis and diminishing the production and quality of crops (Mahmood et al., 2006). Despite attempts to control BB by broad-spectrum breeding with high-yield cultivars, this disease remains a major constraint on rice production (Suh et al., 2013). Earlier research demonstrated that N-acetylserotonin methyltransferase (ASMT), the last enzyme involved in the synthesis of melatonin, was induced during Xoo infection (Wei et al., 2016). However, there have been no reports on the relationship between melatonin and Xoo.
In this study, we used Xoo strain PXO99 to determine whether melatonin exhibits antibacterial activity against this pathogen. In addition, the relationships between melatonin and cell division and morphology were investigated. We also used RNA sequencing (RNA-Seq) to explore how melatonin, in its role as an antibiotic, inhibits the growth of PXO99. A genome-wide expression profiling analysis clearly demonstrated that many genes involved in metabolic and transcription processes were downregulated. Our results could help to gain a better understanding of the mechanisms by which melatonin inhibits the proliferation of Gram-negative bacteria.
Materials and Methods
Bacterial Strain and Plants
Xoo strain PX099 was streaked on nutrient agar (NA) medium (beef extract, 3 g/L; yeast extract, 1 g/L; polypeptone, 5 g/L; sucrose, 10 g/L; and agar, 15 g/L) and incubated at 28°C for 2 days. Rice seedlings of the Nipponbare (Oryza sativa spp. Nipponbare) cultivar were germinated and grown in a growth chamber under an alternating 12-h light, 30°C/12-h dark, 28°C cycle with a photon flux density of 200 μmol/m2.s1. Rice leaves were inoculated with Xoo strain PXO99 (race P6) for pathogenicity tests using the leaf clipping method (Kauffman et al., 1973). Tobacco plants (Nicotiana benthamiana, Nb) were grown in a growth chamber under an alternating 12-h light/12-h dark cycle at 25°C with a photon flux density of 120 μmol/m2.s1. Tobacco leaves were inoculated with PXO99 for hypersensitive reaction (HR) assays using the needleless syringe method (Xu et al., 2015). Statistical analyses were performed using SPSS Version 20.0. The variables were analyzed using Student’s t-tests and were tested for significance at the P < 0.05 (∗), P < 0.01 (∗∗), P < 0.001 (∗∗∗), and P < 0.0001 (∗∗∗∗) levels.
Measurement of the Effect of Melatonin on Bacterial Growth
Xoo strain P6 was incubated with shaking in nutrient broth (NB) medium (NA without agar) at 28°C until an OD600 = 1.0 (early logarithmic phase) was reached. The cells were harvested and resuspended in an equal volume of sterilized ddH2O. Next, 0.5 mL of the cell suspension was added to 50 mL of NB liquid medium containing different concentrations of melatonin (0, 200, 400, or 1000 μg/mL). Methanol (MeOH) solvent without melatonin (0 μg/mL) served as a control. All cultures were shaken (200 rpm) at 28°C in the dark, and the OD600 was measured every 3 h until bacterial growth reached the stationary phase. Each experiment was performed three times, with three replicates per experiment.
Transmission Electron Microscope (TEM) Observations
The concentration of fresh bacteria in sterilized ddH2O was adjusted to OD600 = 1.0. Next, 0.5 mL of cell suspension was added into 50-mL fresh NB medium containing different concentrations of melatonin (0, 200, or 400 μg/mL). Methanol (MeOH) solvent without melatonin (0 μg/mL) served as a control. All cultures were grown at 28°C with shaking at 200 rpm for 12 h. Bacterial samples were placed on copper mesh grids with formvar membranes and negatively stained with phosphotungstic acid (2% v/v, pH = 6.7). The samples were then observed using a TEM (Hitachi H-7650) at 80 kV and photographed with a Gatan832 CCD camera (Gatan, Pleasanton, CA, United States).
Determination of Cell Motility and Biofilm Formation
Swimming motility and biofilm formation assays were performed as described previously (Tian et al., 2015). The concentration of fresh bacteria in sterilized ddH2O was adjusted to OD600 = 1.0. Next, a 5-μL aliquot of the bacterial suspension was spotted onto semi-solid NA (0.3% agar) containing different concentrations of melatonin (0, 10, 40, or 250 μg/mL). Methanol (MeOH) solvent without melatonin (0 μg/mL) served as a control. Cell motility was monitored after a 96 h incubation at 28°C in darkness. Each experiment was performed three times, with five replicates per experiment. For the biofilm formation assay, a 30-μL cell suspension was inoculated into 3 mL NB liquid medium containing different concentrations of melatonin (0, 10, 40, or 250 μg/mL). After inoculation, the cultures were incubated at 28°C for 5 days without shaking. After gently removing the cultures, the cells adhered to the culture tubes were stained with two volumes of 10% (w/v) crystal violet solution and incubated at 28°C without shaking for 1 h, followed by gentle washing with sterilized ddH2O three times, and air drying for 1 h. The crystal violet in the stained cells was dissolved using destaining buffer [40% methanol (v/v), 10% glacial acetic acid (v/v), 50% ddH2O (v/v)], and the absorbance at 595 nm (OD595) was measured using a spectrophotometer (Eppendorf, Germany). Each experiment was performed three times, with six replicates each time.
Measurement of Endogenous Melatonin
To determine the melatonin content of Xoo cells, a direct sample extraction method was used. The concentration of fresh bacteria in sterilized ddH2O was adjusted to OD600 = 1.0. Next, 0.5 mL of cell suspension was added to 50 mL of fresh NB medium containing different concentrations of melatonin (0 or 200 μg/mL). Methanol (MeOH) solvent without melatonin (0 μg/mL) served as a control. All cultures were grown at 28°C with shaking at 200 rpm for 24 h. The two cultures were adjusted to the same concentration (OD600 = 1.0) and washed with sterilized ddH2O three times. Next, the cultures were centrifuged and the pellets were suspended in 10 mL of acetonitrile buffer. The bacterial cells were homogenized using a sonicator (Scientz, Ningbo). After centrifugation, the supernatants were subjected to LC-MS as described previously (Huang and Mazza, 2011).
RNA Sequencing and Data Analysis
RNA was extracted from strain PXO99 treated with MeOH (M0) and 200 μg/mL melatonin (M200) and used for RNA sequencing. After a 21 h incubation, bacterial cultures at OD600 = 1.0 (early logarithmic phase) in broth were harvested. Total RNA was extracted from the mock and melatonin-treated samples using TRIzol reagent (Invitrogen, Carlsbad, CA, United States) according to the procedure recommended by the manufacturer. The following steps were then completed by a commercial company (Genepioneer Biotechnologies Corporation, Nanjing, China). Three micrograms of RNA per sample was used for library construction. For direct comparisons, two libraries (M0 and M200) were prepared in the same manner and sequenced on an Illumina HiSeq Xten platform. We selected genes with a log2FC > 2 and p < 0.01 for further analysis. Differentially expressed genes (DEGs) between the melatonin-treated and mock samples were analyzed by Gene Ontology (GO) and Kyoto Encyclopaedia of Genes and Genomes (KEGG) enrichment.
RNA Extraction and Quantitative Real-Time PCR Analysis
Specific primers for quantitative real-time PCR (qRT-PCR) were designed with Primer 5 (version 5) using the corresponding gene sequences from the NCBI database (Supplementary Table S1). Total RNA was isolated using TRIzol reagent (Invitrogen, Carlsbad, CA, United States) according to the procedure recommended by the manufacturer, treated with DNase I (Takara, Japan) to eliminate genomic DNA, and then converted into cDNA using a PrimeScriptTM RT Reagent Kit (Takara, Japan). Next, qRT-PCR was performed using diluted cDNA and SYBR Green PCR Master Mix (Takara, Japan) on a Quant Studio 6 Real Time PCR system (Thermo Fisher Scientific, United States). The expression data, given as quantification cycle (Cq), were collected and statistically processed using the 2-Δ(ΔCp) method. RecA was used as an internal control, and each experiment was conducted three times with three replicates.
Results and Discussion
Melatonin Inhibits Xoo Growth
Melatonin has been previously observed to play multiple roles in a wide variety of significant processes in plants, animals, and humans (Dollins et al., 1994; Guerrero and Reiter, 2002; Shi et al., 2015; Fu et al., 2017). However, the impact of melatonin on agriculturally relevant bacteria has not been explored. To this end, we assessed the bacterial growth rates of Xoo treated with methanol (mock control) and various concentrations of melatonin (Figure 1A). The OD600 value of PXO99 at 24 h pre-treated with melatonin (200 μg/mL) was approximately 1.0, only half that of the control group (Figure 1B). Thus, 200 μg/mL of melatonin effectively reduced the growth of PXO99. When the concentration was elevated, the bacterial density showed a greater decrease. No PXO99 cells survived 24 h in broth containing 1000 μg/mL melatonin or 50 μg/mL kanamycin. Melatonin represses the growth of human pathogenic bacteria at certain concentrations (Hu et al., 2017), including that of Streptococcus agalactiae at 2 μg/mL (Atroshi et al., 1998) and Saccharomyces cerevisiae at 1000 μg/mL (Konar et al., 2000). Our growth inhibition results showed that melatonin inhibited PXO99 growth in a concentration-dependent manner, and the inhibitory effect may be dose dependent. In plants, we also observed that 200 μg/mL melatonin suppressed the HR induced by PXO99 on tobacco leaves (Supplementary Figure S1). Overexpression of a melatonin-induced gene (OsMAPK12-1) resulted in plants with enhanced disease resistance against PXO99 (Xiao et al., 2017). Melatonin-induced plant resistance is mediated by MAPK signaling (Lee and Back, 2017) Thus, whether the suppression of HR caused by melatonin-induced plant innate immunity or melatonin inhibiting the proliferation of PXO99 caused the observed plant disease resistance still requires further investigation.
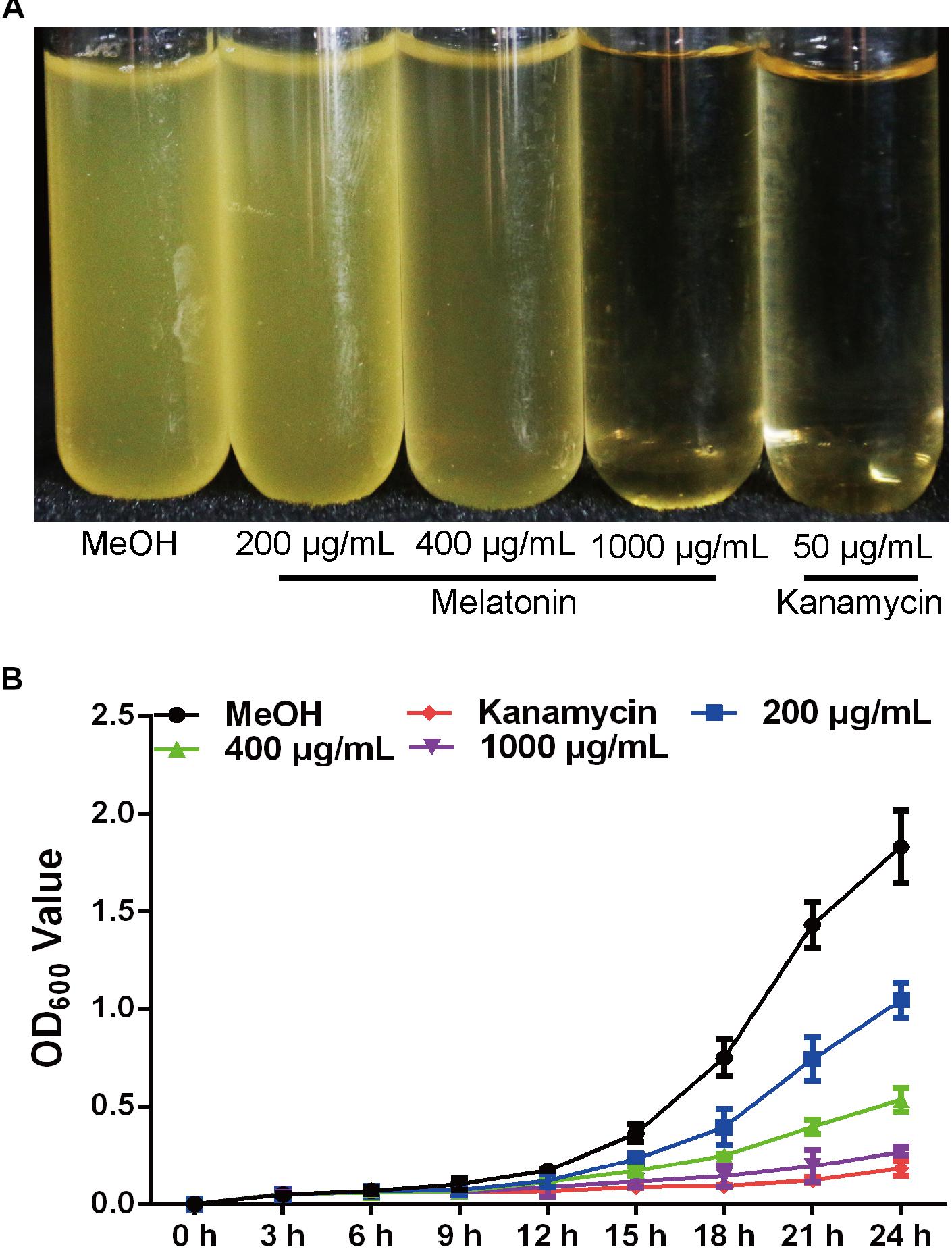
FIGURE 1. Melatonin inhibits the growth of Xoo. (A) The state of PXO99 under melatonin treatment. (B) Statistical analysis of growth curves of PXO99 treated with different concentrations of melatonin (μg/mL) and cultured for 24 h.
Melatonin Reduces Xoo Swimming Motility but Increases Biofilm Formation
Swimming motility is necessary for biofilm formation and is crucial for bacterial attachment (O’Toole and Kolter, 1998; de Kerchove and Elimelech, 2008). However, the impact of melatonin on bacterial swimming motility has rarely been reported. To study the influence of melatonin on bacterial motility, the swimming motility diameter of Xoo was measured in the presence and absence of melatonin. In initial experiments, we observed that a higher concentration of melatonin disrupted the swimming ability of Xoo. Thus, melatonin was used at no more than 200 μg/mL in subsequent tests. As shown in Figure 2A, the colony diameters in plates with 10 μg/mL melatonin were decreased by more than 30% compared with that observed in the mock control. When the melatonin concentration was increased, the swimming motility diameter was further decreased. The colony diameters in plates with 200 μg/mL melatonin were reduced by more than half compared with that observed in the mock control. Thus, melatonin affected the motility of Xoo in a concentration-dependent manner. These results suggest that the inhibition of bacterial motility by melatonin may occur through increasing cell death, although further investigation of this possibility is necessary.
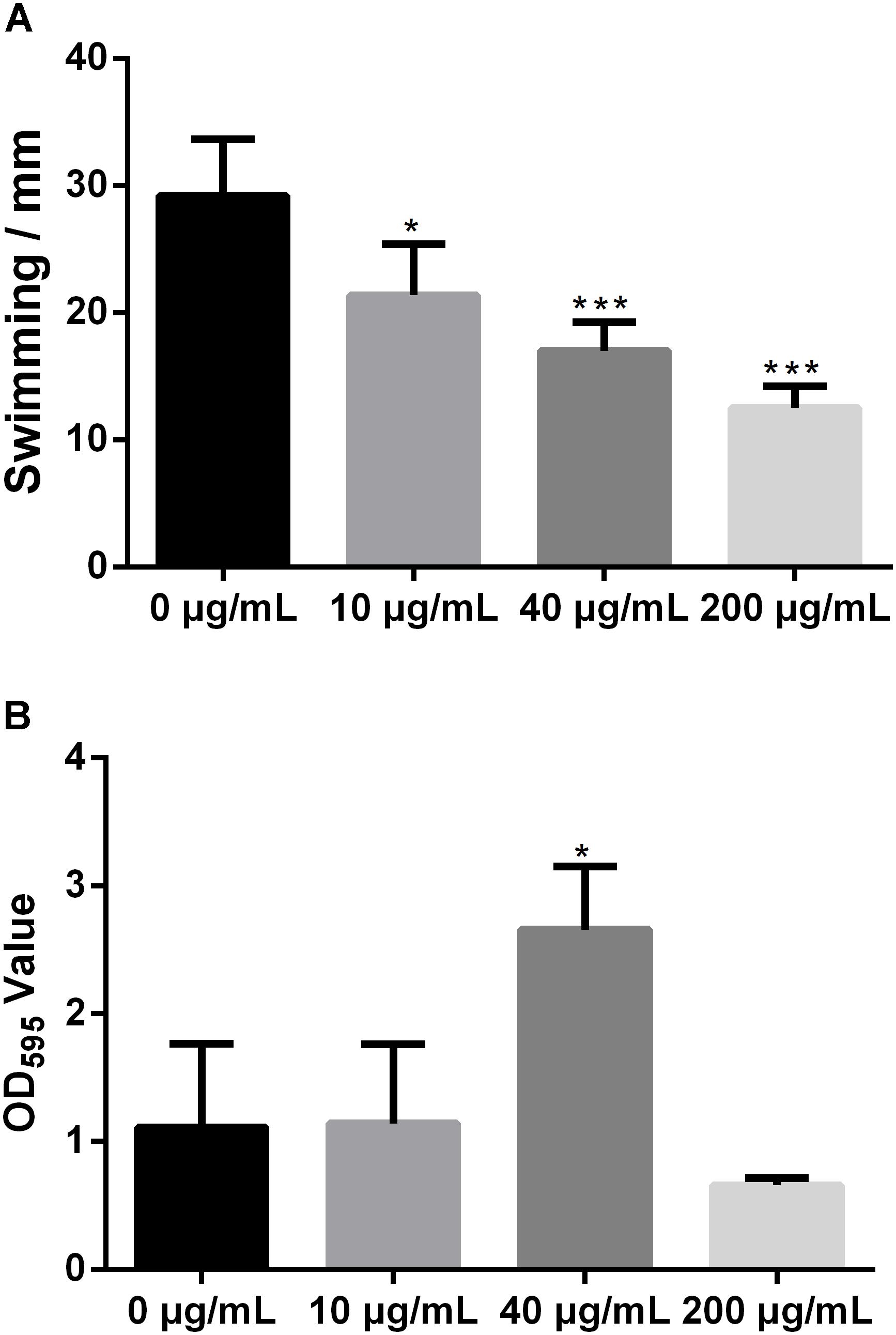
FIGURE 2. Statistical analysis of the swimming motility (A) and biofilm formation of Xoo (B) treated with melatonin. ∗P < 0.05, ∗∗∗P < 0.001.
Biofilm formation plays a crucial role in plant pathogen infections (Parsek and Singh, 2003). Melatonin was reported to inhibit the biofilm formation of Candida parapsilosis and S. aureus ATCC29213 at 2.9 and 340 μg/mL, respectively (Yang et al., 2014; Romic et al., 2016). Biofilm-associated pathogens can form light-colored rings on the wall of a culture tube at the interface between air and broth. To evaluate the effect of melatonin on the attachment of Xoo, the biofilm formation of PXO99 in response to melatonin challenge was analyzed. As shown in Figure 2B, the presence of 10 μg/mL melatonin slightly increased the biofilm formation of PXO99. When the melatonin concentration was increased, the CV absorbance at OD595 showed a greater increase. The observed OD595 value from tubes containing 40 μg/mL melatonin was threefold higher than that of the mock control. However, the opposite effect was observed when melatonin was present at higher concentrations. The OD595 value in the tubes containing melatonin at 200 μg/mL was 40% lower than that of the control. Thus, the effects of melatonin on PXO99 biofilm formation did not resemble the observed effects on swimming motility or growth inhibition. When melatonin was present at a high concentration, no swimming motility, or biofilm formation was observed. Our results suggest that melatonin may induce biofilm formation in Xoo at low concentrations but inhibit its formation at high concentrations. Interestingly, we observed that both the bacterial abundance and lesion length in rice leaves infected with PXO99 treated with melatonin (200 μg/mL) was similar to that of the control group (Supplementary Figure S2). Moreover, the HR in tobacco leaves induced by PXO99 treated with melatonin (200 μg/mL) was similar to that of the control group (Supplementary Figure S3). Thus, the results indicated that melatonin may not affect Xoo pathogenicity.
Xoo Becomes Highly Enriched With Melatonin
Melatonin has been observed to easily pass through cell walls (Tekbas et al., 2008). In this study, we evaluated the content of endogenous melatonin in PXO99 cell treated with melatonin by LC-MS. The endogenous melatonin was 14.43 ng in POX99 cells that were harvested from 30 mL broth cultures after incubating for 24 h. In contrast, 156.13 ng of endogenous melatonin was detected in POX99 cells that were incubated with exogenous melatonin and harvested from 30 mL broth cultures after incubating for 24 h (Figure 3A). The results showed that melatonin can easily pass through the cell wall and become enriched in Xoo cells. The endogenous melatonin detected in the treatment group was approximately 100 times that in the control group (Figure 3B). This disruption in normal endogenous melatonin levels in Xoo may inhibit the proliferation of this bacterium. Because melatonin was detected in PXO99, we can assume that Xoo has the ability to synthesize melatonin and may have a biosynthetic pathway that is similar to that present in plants or animals. However, the function of melatonin in Xoo needs further study.
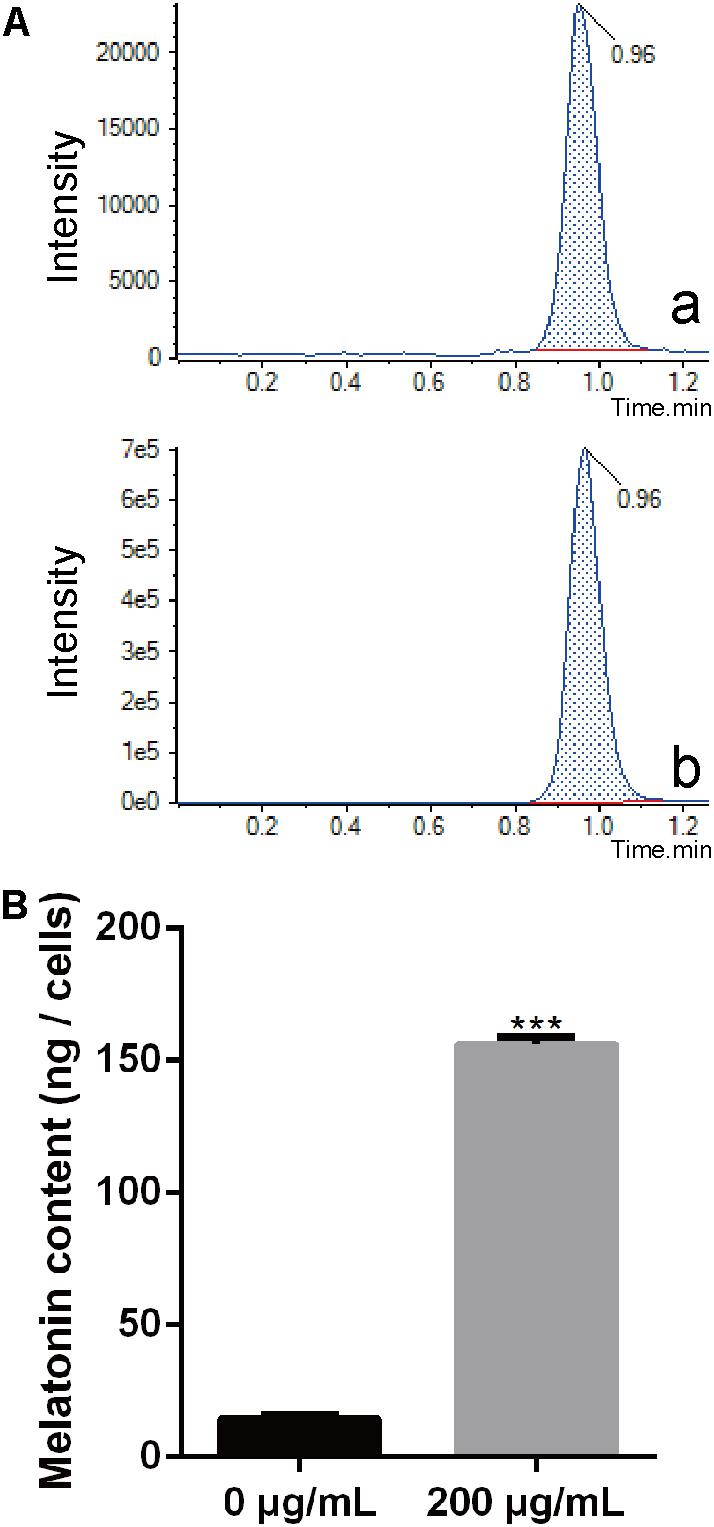
FIGURE 3. Extraction and identification of melatonin in Xoo. (A) Chromatogram corresponding to melatonin. (a) Chromatogram corresponding to melatonin collected from PXO99 cells not treated with melatonin (Sigma). (b) Chromatogram corresponding to melatonin collected from PXO99 cells pre-treated with melatonin (Sigma). (B) Statistical analysis of melatonin (ng/cells) from PXO99 cells. UV response: 280 nm. ∗∗∗P < 0.001.
Melatonin Inhibits Xoo Cell Division
Bacterial cell division occurs by the formation of a Z-ring at the site of division (Lutkenhaus and Addinall, 1997). The dynamics of the Z-ring are regulated by the cell division-related genes ZapE and FtsZ, and the role of ZapA is to recruit ZapB to the inner face of the Z-ring (Galli and Gerdes, 2010; Marteyn et al., 2014). To investigate whether melatonin inhibits bacterial proliferation by disrupting or inhibiting cell division, the mRNA expression of nine cell division-related genes in PXO99 challenged with melatonin (200 g/mL) was analyzed by qRT-PCR. The mRNA expression of many internal genes has been reported to be affected by melatonin treatment (Sheshadri et al., 2018). In preliminary experiments, we tested the stability of two PXO99 internal candidate reference genes and observed that RecA was the more stable of the two in PXO99 cells treated with melatonin. As shown in Figure 4, four cell division-related genes (FetQ, ZapE, FetL, and FetE) were upregulated, and five (ZipA, FetB, ZapA, FetD, and FetZ) were downregulated in Xoo cells treated with melatonin compared to the control cells. Our results indicate that the melatonin treatment resulted in a decrease in Xoo cell division. Because bacterial proliferation depends on the ability of cells to divide (Pardee, 1989), the reduction in cell division could result in an inhibition of Xoo growth.
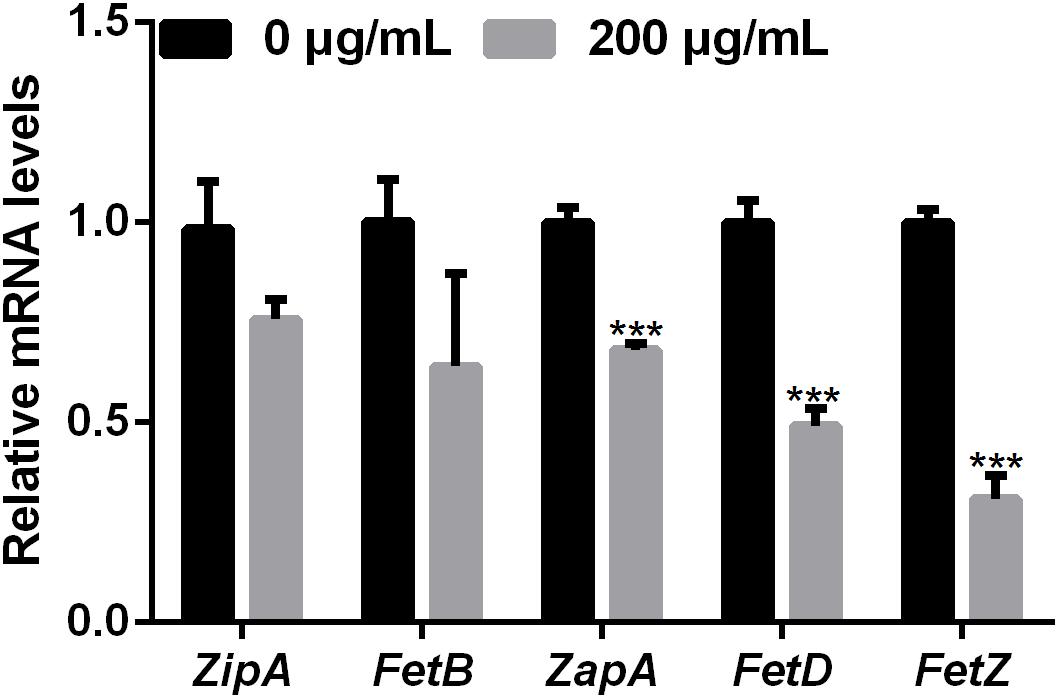
FIGURE 4. qRT-PCR analysis of the mRNA expression of cell-division-related genes in Xoo treated with melatonin. ∗∗∗P < 0.001.
Melatonin Alters Xoo Morphology
A previous study showed that P. infestans cells treated with melatonin exhibited reduced lipid droplet production and inhibited the proliferation of P. infestans (Zhang et al., 2017). In this study, we investigated the effect of melatonin on the cellular morphology of PXO99 by making TEM observations. As shown in Figure 5, both bacterial size and shape were easily distinguished by TEM using a negative staining method. The width and length of individual PXO99 cells ranges from 0.6 to 1.0 μm and from 1.0 to 2.7 μm, respectively, and our observations agreed with these specifications (Figure 5A). By contrast, the width of PXO99 cells treated with melatonin was slightly shorter than in the control, and the length of PXO99 treated with 200 μg/mL of melatonin exhibited a significant reduction (20%) compared to the control (Figure 5B). These data indicate that the reduction in the cell length of PXO99 treated with melatonin may result from the inhibition of Xoo proliferation.
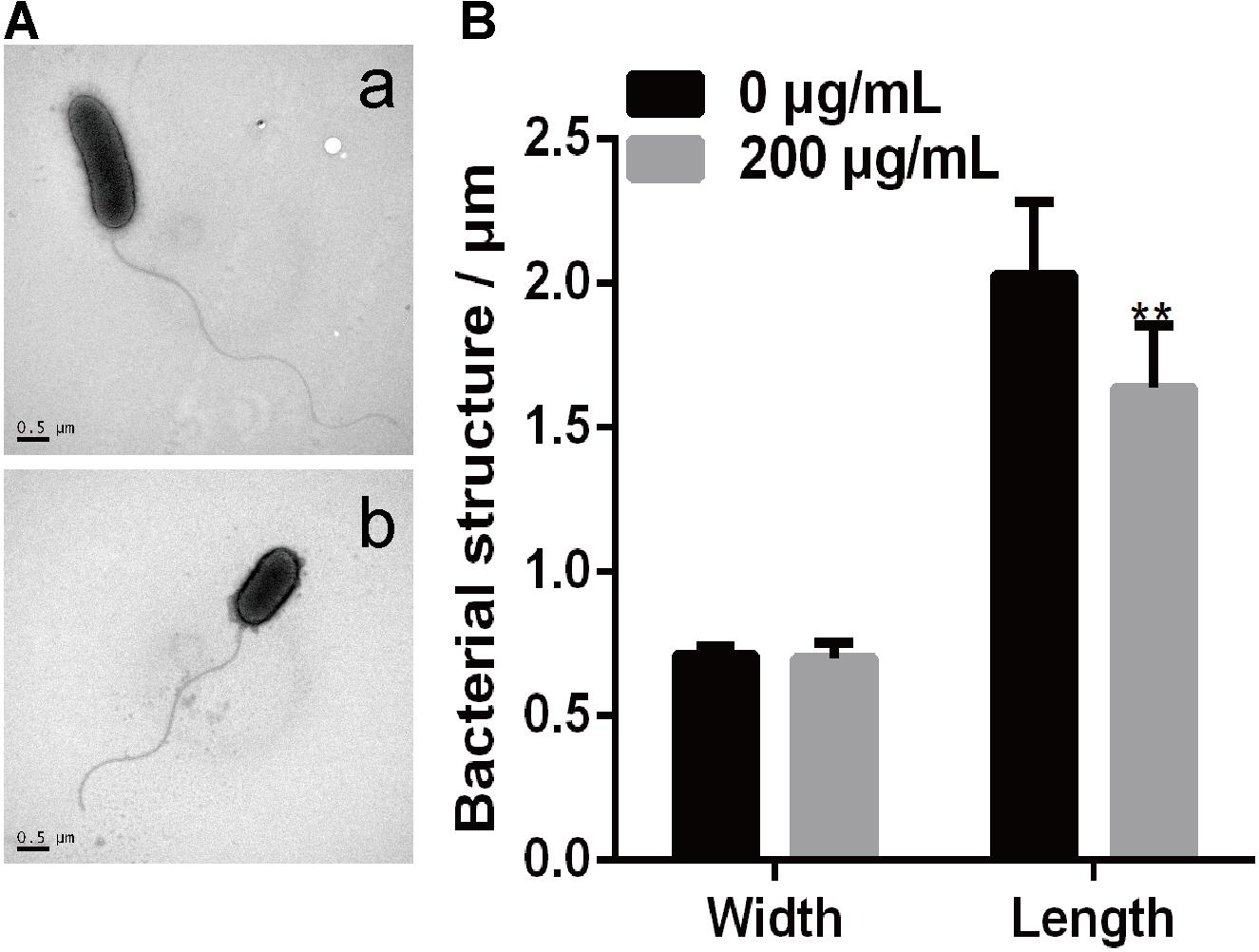
FIGURE 5. Observations of Xoo morphology following treatment with melatonin. (A) The morphology of PXO99 cells. (a) The morphology of PXO99 cells not treated with melatonin. (b) The morphology of PXO99 cells treated with melatonin (200 μg/mL). (B) Statistical analysis of the width and length of PXO99 cells. Bar = 0.5 μm.
RNA-Seq Transcriptome Analysis of Melatonin-Treated Xoo
To further investigate the mechanism of the effects of melatonin on Xoo proliferation, total RNA from PXO99 cells that were treated or untreated with melatonin was collected and analyzed by RNA-Seq. An analysis of the gene expression changes obtained from the RNA-Seq assay showed that 138 genes had alterations in mRNA transcript levels in response to a melatonin challenge at 21 h post-treatment (Table 1), corresponding to 2.73% of the Xoo genome. Fourteen genes were upregulated, and 124 genes were downregulated, and these DEGs were characterized both by using the GO database, which provides annotation information regarding cellular components, molecular functions and biological processes, and by using the KEGG database. Of the 14 upregulated genes, four were enriched in flagellar components, four were enriched in transporter activity, and three were involved in metabolic processes Flagella are used for motility in PXO99. Whether the four flagellar genes regulated by melatonin are involved in swimming motility or biofilm formation requires further study. Transporters are well known to play a crucial role in substantial exchanges between cells and the outside environment, and the upregulated genes involved in transporter activity and metabolic processes may help PXO99 survive. Among the observed downregulated genes, a notable overrepresentation of genes associated with membrane and cellular components was observed in the cellular component category (Figure 6A). Moreover, genes encoding catalytic activity-related proteins were overrepresented in the molecular function category (Figure 6B). Consistently, a notable overrepresentation of genes associated with metabolic processes in the biological processes category was observed (Figure 6C). In the metabolic processes, 41 genes were dominant in the biological processes category (Figure 6C). Consistently, 56 and 27 genes involved in catalytic activity and metal-binding activity, respectively, were dominant in the category of molecular functions (Figure 6B). To verify the reliability of the transcriptomes, 18 randomly selected genes were analyzed using qRT-PCR. The results were consistent with the sequencing data (Figure 7). Genes related to oxidative phosphorylation, citrate cycle, protein secretion, and two component systems were downregulated in PXO99 treated with melatonin.
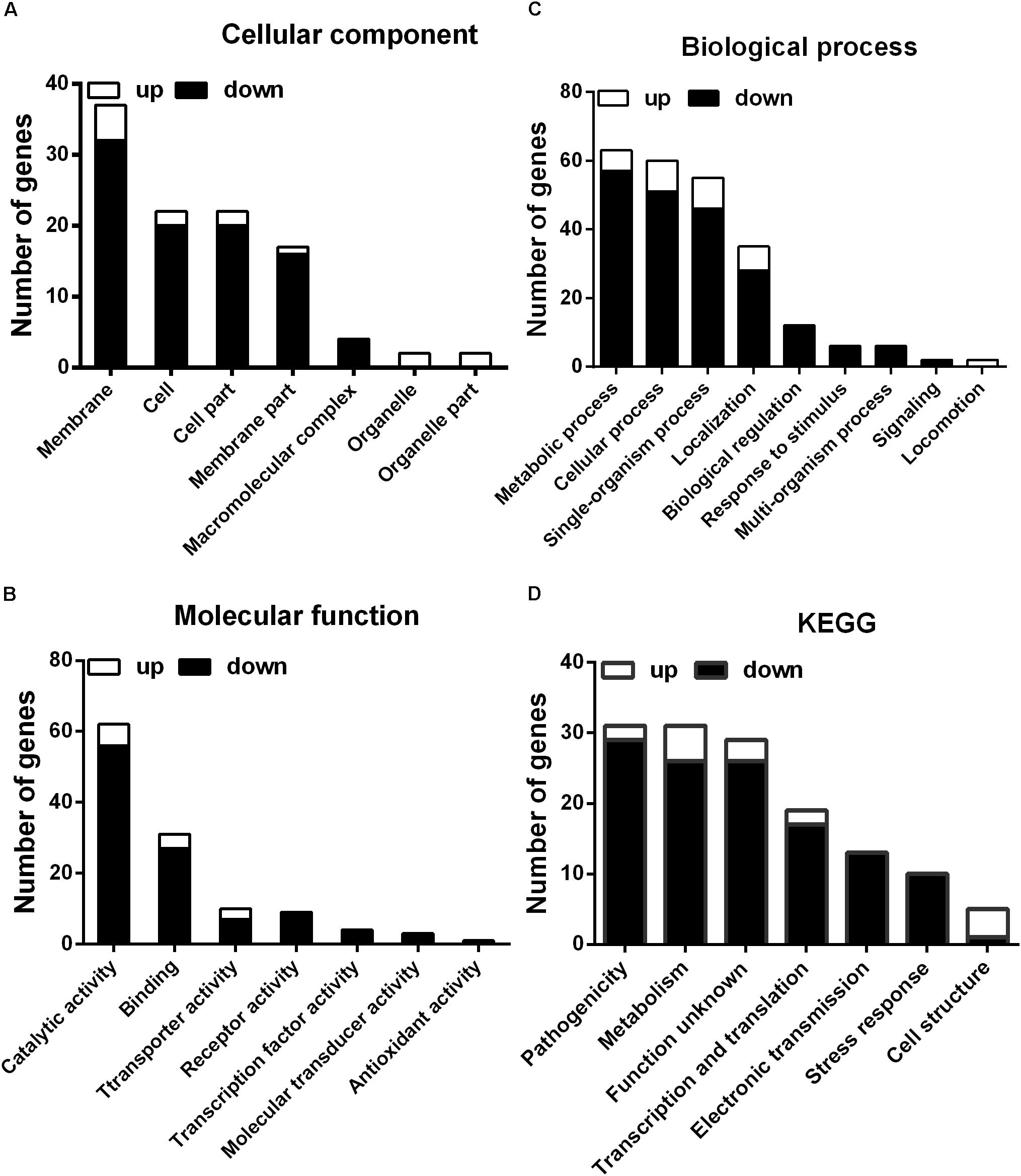
FIGURE 6. Classification of differentially expressed genes by gene ontology (GO) enrichment and cellular mapping. (A) Number of differentially expressed genes. (B) Cellular component classifications. (C) Molecular function. (D) Biological process.
Melatonin Regulates Xoo Metabolism
Metabolism is an important characteristic of bacteria, and melatonin was reported to significantly reduce the expression mRNA of genes associated with metabolism in microbes (Zhang et al., 2017). In this study, we observed that genes involved in carbohydrate and amino acid metabolism were enriched (Figure 8). The best carbon and nitrogen sources for Xoo growth are sucrose and glutamate (Singh, 2016). Interestingly, we observed that many genes involved in sucrose and glutamate metabolism were downregulated in PXO99 when challenged with melatonin.
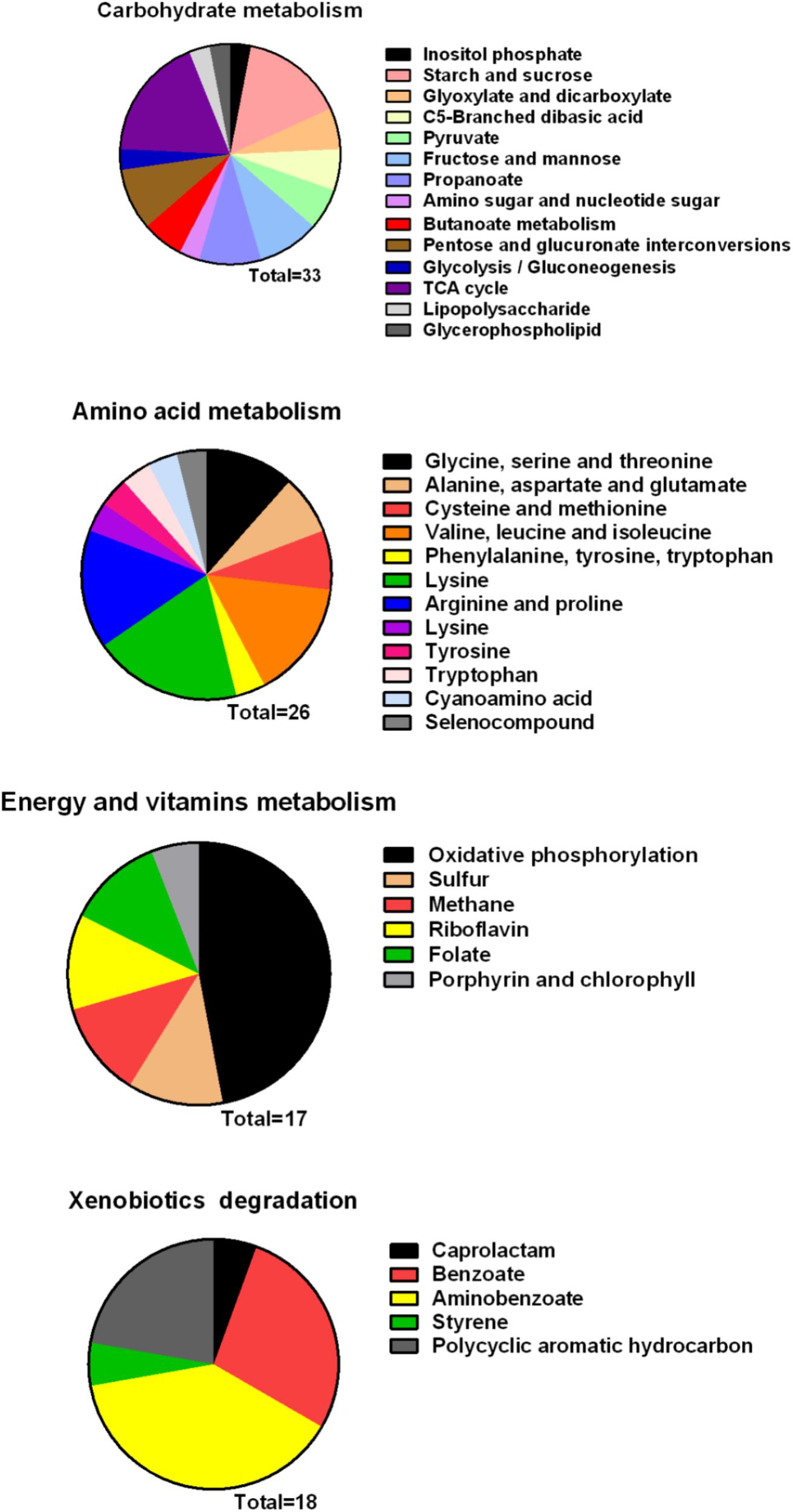
FIGURE 8. Classification of differential genes in the metabolism in PXO99 under melatonin treatment.
Bacterial pathogens are known to require iron for replication and infection (Schaible and Kaufmann, 2004; White and Yang, 2009; Skaar, 2010). Xoo requires ferrous sulfate for optimal proliferation and modulates copper redistribution in rice during infection (Yuan et al., 2010). According to the RNA-Seq results, the mRNA expression of the transporter TonB, which is responsible for iron absorption (Yue et al., 2003), was downregulated in PXO99 challenged with melatonin (Table 1). Other DEGs related to metal binding were also downregulated (Figure 6B). The mRNA levels of genes that encode metal-ion binding and cation binding proteins were previously observed to be downregulated in rice leaves treated with melatonin, similar to our results (Liang et al., 2015). The content of endogenous melatonin in melatonin-treated PXO99 was approximately 100 times that of the control group (Figure 3). Interestingly, 18 genes involved in xenobiotic metabolism were downregulated (Figure 8). Melatonin has a strong ability to bind copper and iron(III) (Limson et al., 1998). Thus, we speculate that melatonin can cause a free iron deficiency in bacterial cells and inhibit growth through the metal-binding activity of melatonin or by reducing the concentration and activity of metal-binding enzymes.
Phosphate is most commonly used in energy metabolic processes and serves as a buffering agent in cells (Lardy and Wellman, 1952). In this study, the mRNA expression of the transcription factor PhoU, which function in environmental phosphate (Pi) sensing and transportation (Muda et al., 1992), was reduced in PXO99 cells treated with melatonin (Table 1). In addition, DEGs encoding proteins located on the cell membrane related to phosphate transporter and phosphate binding proteins involved in energy metabolism were both downregulated (Figure 8). In humans, melatonin inhibits cancer cell growth by preventing the cell membrane from assimilating linoleic fatty acid (Blask et al., 1999). The results of this study indicated that the inhibitory mechanism of melatonin on bacterial growth may be related to reducing phosphate levels, although a detailed characterization of these mechanisms will require further investigation.
Conclusion
In this study, we investigated the potential effects of melatonin on X. oryzae pv. oryzae. Our data showed that melatonin can cross the cell wall and become enriched in Xoo cells, inhibiting the cell division and proliferation of this bacterium. Importantly, melatonin altered the cell structure and reduced the motility and attachment ability of Xoo cells. The results of the transcriptome analysis suggest that the inhibitory effects of melatonin on Xoo proliferation may occur through (i) decreasing cell division and (ii) reducing the concentration and activity of enzymes involved in metabolism. This work provides new insights into the inhibitory effect of melatonin on bacterial growth and gene expression.
Author Contributions
FL, ZF, and XC designed the study. XC and CS performed the experiments. XC, CS, and YZ analyzed the data. XC, CS, and PL drafted the manuscript. FL, IP, JQ, and ZF reviewed and edited the manuscript.
Funding
This research was supported by grants from the National Natural Science Foundation of China (31571974), the Special Fund for Agro-scientific Research in the Public Interest (201303015), the Natural Science Foundation of Jiangsu Province of China (BK20170606), and National Key R&D program of China (2017YFD0200900).
Conflict of Interest Statement
The authors declare that the research was conducted in the absence of any commercial or financial relationships that could be construed as a potential conflict of interest.
Acknowledgments
The authors thank Professor Jianping CHEN (Ningbo University, China) for his language editing of this manuscript.
Supplementary Material
The Supplementary Material for this article can be found online at: https://www.frontiersin.org/articles/10.3389/fmicb.2018.02280/full#supplementary-material
FIGURE S1 | The hypersensitive reaction triggered by Xoo on tobacco leaves pretreated with melatonin. The tobacco leaves were inoculated with different concentrations of Xoo (ddH2O, OD600 = 0.2, 0.4, 0.8). (a) Tobacco leaves without the melatonin treatment and (b) tobacco leaves pretreated with 200 μg/mL melatonin for 12 h.
FIGURE S2 | Effects of exogenous melatonin on the pathogenicity of PXO99 and the hypersensitive response. (A) Phenotype of rice leaves inoculated with PXO99 treated with melatonin (200 μg/mL). (B) Lesion length on rice leaves inoculated with PXO99 treated with melatonin (200 μg/mL). (C) Bacterial population in rice leaves inoculated with PXO99 treated with melatonin (200 μg/mL). Bar = 2 cm.
FIGURE S3 | The hypersensitive reaction of tobacco leaves triggered by Xoo pretreated with melatonin. Xoo cells were pretreated with melatonin (200 μg/mL) for 24 h. (A) The tobacco leaves inoculated with ddH2O, Xoo without melatonin treatment, Xoo pretreated with melatonin (200 μg/mL). (B) The mRNA expression of HrpD6 in response to the melatonin treatment.
Footnotes
References
Arnao, M. B., and Hernández-Ruiz, J. (2017). Growth activity, rooting capacity, and tropism: three auxinic precepts fulfilled by melatonin. Acta Physiol. Plant 39:127. doi: 10.1007/s11738-017-2428-3
Atroshi, F., Rizzo, A., Westermarck, T., and Ali-vehmas, T. (1998). Effects of tamoxifen, melatonin, coenzyme Q10, and L-carnitine supplementation on bacterial growth in the presence of mycotoxins. Pharmacol. Res. 38, 289–295. doi: 10.1006/phrs.1998.0363
Blask, D. E., Sauer, L. A., Dauchy, R. T., Holowachuk, E. W., Ruhoff, M. S., and Kopff, H. S. (1999). Melatonin inhibition of cancer growth in vivo involves suppression of tumor fatty acid metabolism via melatonin receptor-mediated signal transduction events. Cancer Res. 59, 4693–4701.
Bubis, M., and Zisapel, N. (1995). Facilitation and inhibition of G-protein regulated protein secretion by melatonin. Neurochem. Int. 27, 177–183. doi: 10.1016/0197-0186(95)00027-6
Byeon, Y., and Back, K. (2016). Low melatonin production by suppression of either serotonin N-acetyltransferase or N-acetylserotonin methyltransferase in rice causes seedling growth retardation with yield penalty, abiotic stress susceptibility, and enhanced coleoptile growth under anoxic conditions. J. Pineal Res. 60, 348–359. doi: 10.1111/jpi.12317
Chen, Q., Qi, W. B., Reiter, R. J., Wei, W., and Wang, B. M. (2009). Exogenously applied melatonin stimulates root growth and raises endogenous indoleacetic acid in roots of etiolated seedlings of Brassica juncea. J. Plant Physiol. 166, 324–328. doi: 10.1016/j.jplph.2008.06.002
de Kerchove, A. J., and Elimelech, M. (2008). Bacterial swimming motility enhances cell deposition and surface coverage. Environ. Sci. Technol. 42, 4371–4377. doi: 10.1021/es703028u
Dollins, A. B., Zhdanova, I. V., Wurtman, R. J., Lynch, H. J., and Deng, M. H. (1994). Effect of inducing nocturnal serum melatonin concentrations in daytime on sleep, mood, body temperature, and performance. Proc. Natl. Acad. Sci. U.S.A. 91, 1824–1828. doi: 10.1073/pnas.91.5.1824
Dubbels, R., Reiter, R. J., Klenke, E., Goebel, A., Schnakenberg, E., Ehlers, C., et al. (1995). Melatonin in edible plants identified by radioimmunoassay and by high performance liquid chromatography-mass spectrometry. J. Pineal Res. 18, 28–31. doi: 10.1111/j.1600-079X.1995.tb00136.x
Fu, J., Wu, Y., Miao, Y., Xu, Y., Zhao, E., Wang, J., et al. (2017). Improved cold tolerance in elymus nutans by exogenous application of melatonin may involve ABA-dependent and ABA-independent pathways. Sci. Rep. 7:39865. doi: 10.1038/srep39865
Galli, E., and Gerdes, K. (2010). Spatial resolution of two bacterial cell division proteins: ZapA recruits ZapB to the inner face of the Z-ring. Mol. Microbiol. 76, 1514–1526. doi: 10.1111/j.1365-2958.2010.07183.x
Garfinkel, D., Laudon, M., Nof, D., and Zisapel, N. (1995). Improvement of sleep quality in elderly people by controlled-release melatonin. Lancet 346, 541–544. doi: 10.1016/S0140-6736(95)91382-3
Guerrero, J. M., and Reiter, R. J. (2002). Melatonin-immune system relationships. Curr. Top. Med. Chem. 2, 167–179. doi: 10.2174/1568026023394335
Hattori, A., Migitaka, H., Iigo, M., Itoh, M., Yamamoto, K., Ohtani-Kaneko, R., et al. (1995). Identification of melatonin in plants and its effects on plasma melatonin levels and binding to melatonin receptors in vertebrates. Biochem. Mol. Biol. Int. 35, 627–634.
Hu, W., Deng, C., Ma, Z., Wang, D., Fan, C., Li, T., et al. (2017). Utilizing melatonin to combat bacterial infections and septic injury. Br. J. Pharmacol. 174, 754–768. doi: 10.1111/bph.13751
Huang, X., and Mazza, G. (2011). Application of LC and LC-MS to the analysis of melatonin and serotonin in edible plants. Crit. Rev. Food Sci. Nutr. 51, 269–284. doi: 10.1080/10408398.2010.529193
Jung-Hynes, B., Reiter, R. J., and Ahmad, N. (2010). Sirtuins, melatonin and circadian rhythms: building a bridge between aging and cancer. J. Pineal Res. 48, 9–19. doi: 10.1111/j.1600-079X.2009.00729.x
Karakas, F., Karakas, A., Turker, A., and Yildirim, A. (2013). Antibacterial and antitumor activities of melatonin hormone. Spatula DD 3, 33–39. doi: 10.5455/spatula.20130422052142
Kauffman, H. E., Reddy, A. P. K., Hsieh, S. P. Y., and Merca, S. D. (1973). Improved technique for evaluating resistance of rice varieties to Xanthomonas oryzae. Plant Dis. Rep. 57, 537–541.
Kolar, J., and Machackova, I. (2005). Melatonin in higher plants: occurrence and possible functions. J. Pineal Res. 39, 333–341. doi: 10.1111/j.1600-079X.2005.00276.x
Konar, V. V., Yilmaz, O., Ozturk, A. I., Kirba gbreve, S., and Arslan, M. (2000). Antimicrobial and biological effects of bomphos and phomphos on bacterial and yeast cells. Bioorg. Chem. 28, 214–225. doi: 10.1006/bioo.2000.1173
Lardy, H. A., and Wellman, H. (1952). Oxidative phosphorylations; role of inorganic phosphate and acceptor systems in control of metabolic rates. J. Biol. Chem. 195, 215–224.
Lee, H. Y., and Back, K. (2017). Melatonin is required for H2 O2 - and NO-mediated defense signaling through MAPKKK3 and OXI1 in Arabidopsis thaliana. J. Pineal Res. 62:e12379. doi: 10.1111/jpi.12379
Lee, H. Y., Byeon, Y., and Back, K. (2014). Melatonin as a signal molecule triggering defense responses against pathogen attack in arabidopsis and tobacco. J. Pineal Res. 57, 262–268. doi: 10.1111/jpi.12165
Lerner, A. B., Case, J. D., Takahashi, Y., Lee, T. H., and Mori, W. (1958). Isolation of melatonin, the pineal gland factor that lightens melanocyteS1. J. Am. Chem. Soc. 80, 2587–2587. doi: 10.1021/ja01543a060
Liang, C., Zheng, G., Li, W., Wang, Y., Hu, B., Wang, H., et al. (2015). Melatonin delays leaf senescence and enhances salt stress tolerance in rice. J. Pineal Res. 59, 91–101. doi: 10.1111/jpi.12243
Limson, J., Nyokong, T., and Daya, S. (1998). The interaction of melatonin and its precursors with aluminium, cadmium, copper, iron, lead, and zinc: an adsorptive voltammetric study. J. Pineal Res. 24, 15–21. doi: 10.1111/j.1600-079X.1998.tb00361.x
Lutkenhaus, J., and Addinall, S. G. (1997). Bacterial cell division and the Z ring. Annu. Rev. Biochem. 66, 93–116. doi: 10.1146/annurev.biochem.66.1.93
Mahmood, T., Jan, A., Kakishima, M., and Komatsu, S. (2006). Proteomic analysis of bacterial-blight defense-responsive proteins in rice leaf blades. Proteomics 6, 6053–6065. doi: 10.1002/pmic.200600470
Manchester, L. C., Coto-Montes, A., Boga, J. A., Andersen, L. P., Zhou, Z., Galano, A., et al. (2015). Melatonin: an ancient molecule that makes oxygen metabolically tolerable. J. Pineal Res. 59, 403–419. doi: 10.1111/jpi.12267
Mansfield, J., Genin, S., Magori, S., Citovsky, V., Sriariyanum, M., Ronald, P., et al. (2012). Top 10 plant pathogenic bacteria in molecular plant pathology. Mol. Plant Pathol. 13, 614–629. doi: 10.1111/j.1364-3703.2012.00804.x
Marteyn, B. S., Karimova, G., Fenton, A. K., Gazi, A. D., West, N., Touqui, L., et al. (2014). ZapE is a novel cell division protein interacting with FtsZ and modulating the Z-ring dynamics. mBio 5:e00022-e14. doi: 10.1128/mBio.00022-14
Menendez-Pelaez, A., and Reiter, R. J. (1993). Distribution of melatonin in mammalian tissues: the relative importance of nuclear versus cytosolic localization. J. Pineal Res. 15, 59–69. doi: 10.1111/j.1600-079X.1993.tb00511.x
Muda, M., Rao, N. N., and Torriani, A. (1992). Role of PhoU in phosphate transport and alkaline phosphatase regulation. J. Bacteriol. 174, 8057–8064. doi: 10.1128/jb.174.24.8057-8064.1992
O’Toole, G. A., and Kolter, R. (1998). Flagellar and twitching motility are necessary for Pseudomonas aeruginosa biofilm development. Mol. Microbiol. 30, 295–304. doi: 10.1046/j.1365-2958.1998.01062.x
Ozturk, A. I., Yilmaz, O., Kirbag, S., and Arslan, M. (2000). Antimicrobial and biological effects of ipemphos and amphos on bacterial and yeast strains. Cell Biochem. Funct. 18, 117–126. doi: 10.1002/(SICI)1099-0844(200006)18:2<117::AID-CBF863>3.0.CO;2-1
Pardee, A. B. (1989). G1 events and regulation of cell proliferation. Science 246, 603–608. doi: 10.1126/science.2683075
Parsek, M. R., and Singh, P. K. (2003). Bacterial biofilms: an emerging link to disease pathogenesis. Annu. Rev. Microbiol. 57, 677–701. doi: 10.1146/annurev.micro.57.030502.090720
Radio, N. M., Doctor, J. S., and Witt-Enderby, P. A. (2006). Melatonin enhances alkaline phosphatase activity in differentiating human adult mesenchymal stem cells grown in osteogenic medium via MT2 melatonin receptors and the MEK/ERK (1/2) signaling cascade. J. Pineal Res. 40, 332–342. doi: 10.1111/j.1600-079X.2006.00318.x
Reiter, R. J., Mayo, J. C., Tan, D. X., Sainz, R. M., Alatorre-Jimenez, M., and Qin, L. (2016). Melatonin as an antioxidant: under promises but over delivers. J. Pineal Res. 61, 253–278. doi: 10.1111/jpi.12360
Romic, M. D., Klaric, M. S., Lovric, J., Pepic, I., Cetina-Cizmek, B., Filipovic-Grcic, J., et al. (2016). Melatonin-loaded chitosan/pluronic(R) F127 microspheres as in situ forming hydrogel: an innovative antimicrobial wound dressing. Eur. J. Pharm. Biopharm. 107, 67–79. doi: 10.1016/j.ejpb.2016.06.013
Schaible, U. E., and Kaufmann, S. H. (2004). Iron and microbial infection. Nat. Rev. Microbiol. 2, 946–953. doi: 10.1038/nrmicro1046
Sheshadri, S. A., Nishanth, M. J., Yamine, V., and Simon, B. (2018). Effect of Melatonin on the stability and expression of reference genes in Catharanthus roseus. Sci. Rep. 8, 2222. doi: 10.1038/s41598-018-20474-2
Shi, H., Chen, Y., Tan, D. X., Reiter, R. J., Chan, Z., and He, C. (2015). Melatonin induces nitric oxide and the potential mechanisms relate to innate immunity against bacterial pathogen infection in Arabidopsis. J. Pineal Res. 59, 102–108. doi: 10.1111/jpi.12244
Shi, H., Wei, Y., and He, C. (2016). Melatonin-induced CBF/DREB1s are essential for diurnal change of disease resistance and CCA1 expression in Arabidopsis. Plant Physiol. Biochem. 100, 150–155. doi: 10.1016/j.plaphy.2016.01.018
Singh, B. (2016). Sugar, amino acids and nitrate as nitrogenous source of fertilization influence growth of Xanthomonas oryzae pv. oryzae and Aspergillus species in hypersensitive response development of tobacco crop. Int. J. Plant Reprod. Biol. 8, 88–93. doi: 10.14787/ijprb
Skaar, E. P. (2010). The battle for iron between bacterial pathogens and their vertebrate hosts. PLoS Pathog. 6:e1000949. doi: 10.1371/journal.ppat.1000949
Suh, J. P., Jeung, J. U., Noh, T. H., Cho, Y. C., Park, S. H., Park, H. S., et al. (2013). Development of breeding lines with three pyramided resistance genes that confer broad-spectrum bacterial blight resistance and their molecular analysis in rice. Rice 6:5. doi: 10.1186/1939-8433-6-5
Tekbas, O. F., Ogur, R., Korkmaz, A., Kilic, A., and Reiter, R. J. (2008). Melatonin as an antibiotic: new insights into the actions of this ubiquitous molecule. J. Pineal Res. 44, 222–226. doi: 10.1111/j.1600-079X.2007.00516.x
Tian, Y., Zhao, Y., Wu, X., Liu, F., Hu, B., and Walcott, R. R. (2015). The type VI protein secretion system contributes to biofilm formation and seed-to-seedling transmission of acidovorax citrulli on melon. Mol. Plant Pathol. 16, 38–47. doi: 10.1111/mpp.12159
Vielma, J. R., Bonilla, E., Chacin-Bonilla, L., Mora, M., Medina-Leendertz, S., and Bravo, Y. (2014). Effects of melatonin on oxidative stress, and resistance to bacterial, parasitic, and viral infections: a review. Acta Trop. 137, 31–38. doi: 10.1016/j.actatropica.2014.04.021
Vivien-Roels, B., Pevet, P., Beck, O., and Fevre-Montange, M. (1984). Identification of melatonin in the compound eyes of an insect, the locust (Locusta migratoria), by radioimmunoassay and gas chromatography-mass spectrometry. Neurosci. Lett. 49, 153–157. doi: 10.1016/0304-3940(84)90152-6
Wang, H. X., Liu, F., and Ng, T. B. (2001). Examination of pineal indoles and 6-methoxy-2-benzoxazolinone for antioxidant and antimicrobial effects. Comp. Biochem. Physiol. C. Toxicol Pharmacol. 130, 379–388. doi: 10.1016/S1532-0456(01)00264-2
Wei, Y., Zeng, H., Hu, W., Chen, L., He, C., and Shi, H. (2016). Comparative transcriptional profiling of melatonin synthesis and catabolic genes indicates the possible role of melatonin in developmental and stress responses in rice. Front. Plant Sci. 7:676. doi: 10.3389/fpls.2016.00676
White, F. F., and Yang, B. (2009). Host and pathogen factors controlling the rice-Xanthomonas oryzae interaction. Plant Physiol. 150, 1677–1686. doi: 10.1104/pp.109.139360
Xiao, X., Zhijuan, T., Xiuqiong, L., Yuhui, H., Boling, L., Wenfang, X., et al. (2017). Overexpressing OsMAPK12-1 inhibits plant growth and enhances resistance to bacterial disease in rice. Funct. Plant Biol. 44, 694–704. doi: 10.1071/fp16397
Xu, H., Zhao, Y., Qian, G., and Liu, F. (2015). XocR, a LuxR solo required for virulence in Xanthomonas oryzae pv. oryzicola. Front. Cell Infect. Microbiol. 5:37. doi: 10.3389/fcimb.2015.00037
Yang, H. P., Tsang, P. C., and Tsang, P. W. (2014). Melatonin inhibits biofilm formation in Candida parapsilosis. J. Mycol. Med. 24, 360–361. doi: 10.1016/j.mycmed.2014.05.003
Yuan, M., Chu, Z., Li, X., Xu, C., and Wang, S. (2010). The bacterial pathogen Xanthomonas oryzae overcomes rice defenses by regulating host copper redistribution. Plant Cell 22, 3164–3176. doi: 10.1105/tpc.110.078022
Yue, W. W., Grizot, S., and Buchanan, S. K. (2003). Structural evidence for iron-free citrate and ferric citrate binding to the TonB-dependent outer membrane transporter FecA. J. Mol. Biol. 332, 353–368. doi: 10.1016/S0022-2836(03)00855-6
Zhang, S., Zheng, X., Reiter, R. J., Feng, S., Wang, Y., Liu, S., et al. (2017). Melatonin attenuates potato late blight by disrupting cell growth, stress tolerance, fungicide susceptibility and homeostasis of gene expression in phytophthora infestans. Front. Plant Sci. 8:1993. doi: 10.3389/fpls.2017.01993
Keywords: melatonin, Xanthomonas oryzae pv. oryzae, antibacterial action, growth, transcriptome
Citation: Chen X, Sun C, Laborda P, Zhao Y, Palmer I, Fu ZQ, Qiu J and Liu F (2018) Melatonin Treatment Inhibits the Growth of Xanthomonas oryzae pv. oryzae. Front. Microbiol. 9:2280. doi: 10.3389/fmicb.2018.02280
Received: 01 June 2018; Accepted: 06 September 2018;
Published: 04 October 2018.
Edited by:
Jack Wong, The Chinese University of Hong Kong, Hong KongReviewed by:
Maximino Manzanera, Universidad de Granada, SpainShahper Nazeer Khan, Aligarh Muslim University, India
Copyright © 2018 Chen, Sun, Laborda, Zhao, Palmer, Fu, Qiu and Liu. This is an open-access article distributed under the terms of the Creative Commons Attribution License (CC BY). The use, distribution or reproduction in other forums is permitted, provided the original author(s) and the copyright owner(s) are credited and that the original publication in this journal is cited, in accordance with accepted academic practice. No use, distribution or reproduction is permitted which does not comply with these terms.
*Correspondence: Fengquan Liu, ZnFsaXUyMDAxMUBzaW5hLmNvbQ== Jingping Qiu, NzgxMTA0OTM1QHFxLmNvbQ==
†These authors have contributed equally to this work