- School of Life Science, East China Normal University, Shanghai, China
Recently, an enzyme named protein-glutaminase (PG) has been identified as a new type of enzyme with significant potential for deamidation of food proteins. The enzyme is shown to be expressed as a pre-pro-protein with a putative signal peptide of 21 amino acids, a pro-sequence of 114 amino acids, and a mature PG of 185 amino acids. The microbial enzyme PG specifically catalyzes deamidation of proteins without protein hydrolysis pretreatment and only reacts with glutamine residues in the side-chains of proteins or long peptides. All these attributes suggest that it has a great potential for food industrial applications. However, until recently, there have been relatively few studies of the PG-producing strains. A strain named Chryseobacterium proteolyticum QSH1265 which can produce PG was isolated from a soil sample collected in Songjiang, Shanghai, China. Its enzyme activity was about 0.34 ± 0.01 U/mL when using carboxybenzoxy-Gln-Gly as a substrate. The strain can produce acid from D-glucose, maltose, L-arabinose sucrose, glycerol, and mannitol but not fructose, and it is also positive for indole production and urease. Here we describe the complete genome sequence of this strain via PacBio RSII sequencing. The C. proteolyticum QSH1265 genome consists of a circular chromosome with total length of 4,849,803 bp without any plasmids. All of 4563 genes were predicted including 4459 genes for protein-coding and 104 RNA-relative genes with an average G+C content of 36.16%. The KEGG and COG annotation provide information for the specific function of proteins encoded in the genome, such as proteases, chromoproteins, stress proteins, antiporters, etc. A highly conserved hypothetical protein shares a promoter with the gene encoding the protein-glutaminase enzyme. The genome sequence and preliminary annotation provide valuable genetic information for further study of C. proteolyticum.
Introduction
Vegetable protein-containing (especially protein from soy) foods and beverages have become popular among consumers because of their potential health benefits. Since 2003, both the soy protein-based market and sales have shown a strong increase (Suppavorasatit et al., 2013). However, vegetable proteins usually contain a high level of glutamine and asparagine which may crosslink with other amino acids through hydrogen bonds, resulting in low solubility and undesirable “off” flavors in aqueous solutions (Shih et al., 1992). Low solubility has limited the utilization of proteins, especially vegetable proteins in the food industry. Low solubility and “off” flavors can be solved by changing the conformation of proteins by physical, chemical, and enzymatic modification (Seo et al., 2008; Liu et al., 2010). Deamidation, which has proved to be one of the most promising protein modification methods, can improve the solubility, emulsification, foaming, and other functional properties of food proteins by increasing the number of negative charges that decrease the isoelectric point of the protein (Hamada and Swanson, 1994), which results from converting amide groups into carboxyl groups with the concomitant release of ammonia.
Due to its high efficiency, mild reaction conditions, strong specificity, and safety, enzymatic protein deamidation is becoming more desirable than chemical and physical treatments for food systems (Panyam and Kilara, 1996). Some enzymes, transglutaminase, peptidoglutaminases, and proteases for this purpose have been explored. However, side reactions are inevitable for transglutaminase and proteases due to the primary catalytic reactions of these two enzymes are not deamidation itself, and the substrates of peptidoglutaminases are limited to short-size peptides (Kikuchi et al., 1971). Thus, an ideal enzyme is required that catalyzes the deamidation of protein rather than short peptides.
Protein-glutaminase (PG) is a novel deamidation enzyme obtained from purified culture supernatant of Chryseobacterium proteolyticum strain 9670T (Yamaguchi and Yokoe, 2000). Compared with the other deamidation enzymes, deamidation of protein is the primary catalytic reaction of PG. It only reacts with glutamine residues in the side-chains of proteins or long peptides, instead of asparagine residues and free glutamine. According to previous reports, PG is a monomeric single polypeptide consisting of 185 amino acids with an isoelectric point of 10.0 and a molecular weight of 19.86 kDa. The enzyme is expressed as a pre-pro-protein with a putative signal peptide of 21 amino acids, a pro-region of 114 amino acids and a mature PG of 185 amino acids (Yamaguchi et al., 2001).
The species C. proteolyticum, belongs to the genus Chryseobacterium of the family Flavobacteriaceae, (Table 1) which can produce PG. The safety of both the strain and its production was verified in 2007 (Scheuplein et al., 2007). Previous studies had a much narrower focus, concentrating on the properties, structure, and applications of PG instead of the enzyme producing strain (Yamaguchi et al., 2001; Yong et al., 2004, 2006; Miwa et al., 2010; Liu et al., 2011; Cui et al., 2013; Miwa et al., 2013). It is urgent to enrich strains to improve the production of PG. A PG producing strain, C. proteolyticum QSH1265, was the first wild strain isolated in April, 2014 from soil in the Songjiang district of Shanghai, China by enrichment cultivation with carboxybenzoxy (CBZ)-Gln-Gly as the only nitrogen source. Its enzyme activity was about 0.34 ± 0.01 U/mL when using CBZ-Gln-Gly as a substrate (Supplementary Figure S1). However, the low enzyme producing ability of wild strains, as well as the genomic diversity and an incomplete understanding of the genetic features of C. proteolyticum, has greatly limited the application of PG in the food industry. Here, we present the genome sequence and the genomic information of C. proteolyticum QSH1265.
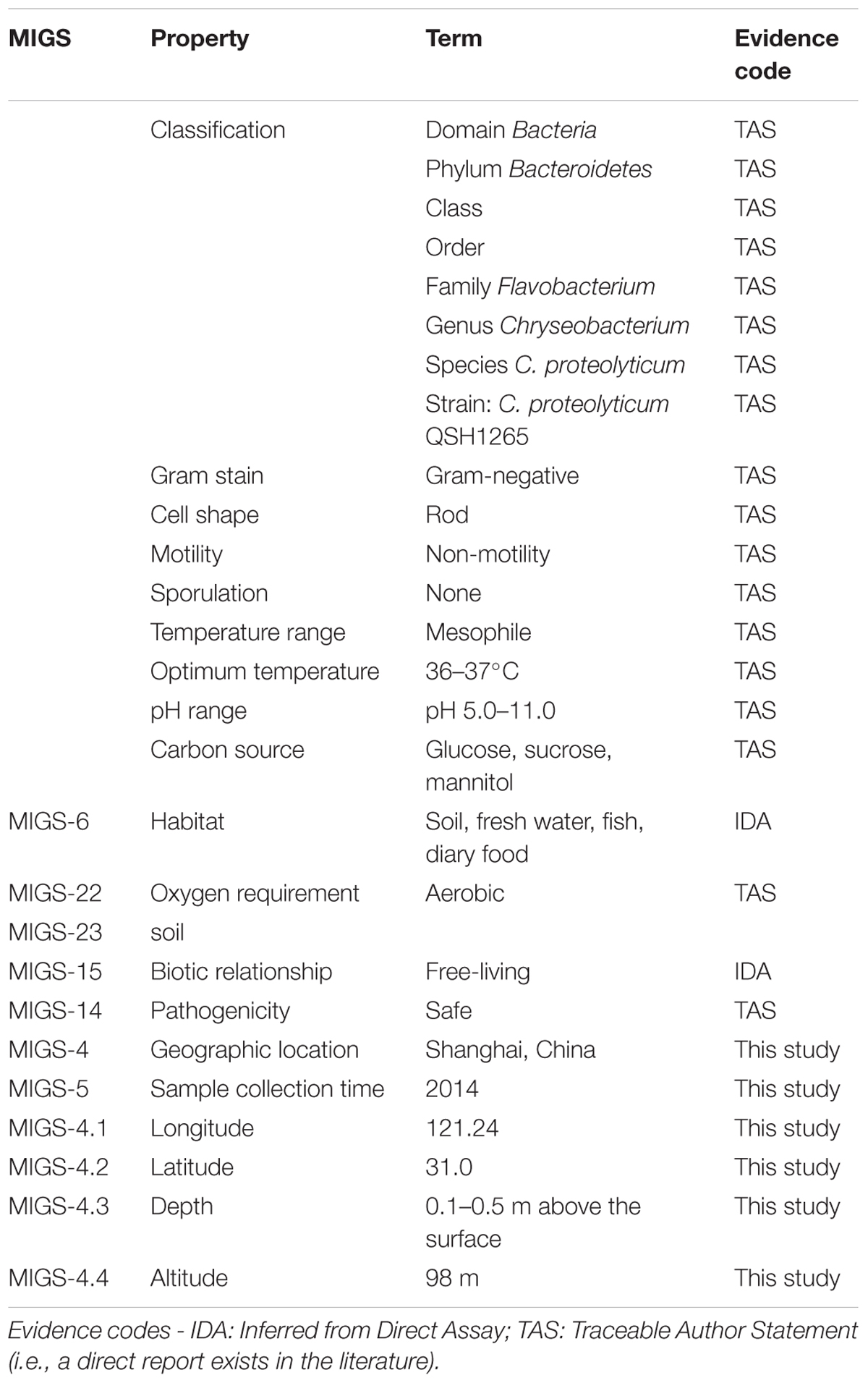
TABLE 1. Classification and general features of C. proteolyticum QSH1265 according to the MIGS recommendations.
Materials and Methods
Bacterial Strain Information and Phenotypic Characteristics
The cells of C. proteolyticum QSH1265 are Gram-negative, aerobic, non-spore forming bacteria. Lacking flagella, they are smooth surfaced, short and rod-shaped; in alkaline environments they turn red (Yamaguchi and Yokoe, 2000). Electron microscopy reveals that the length of cells varies from 0.5 to 1.8 μm and the width is between 0.3 and 0.6 μm (Figure 1). The optimum temperature and pH environment for growth for this organism is 36–37°C and pH 5 to 11 (Table 1). C. proteolyticum QSH1265 can produce acid from D-glucose, maltose, L-arabinose sucrose, glycerol, and mannitol but not fructose. It is also positive for indole production and the activity of catalase and urease but negative for hydrolysing of starch (data not shown).
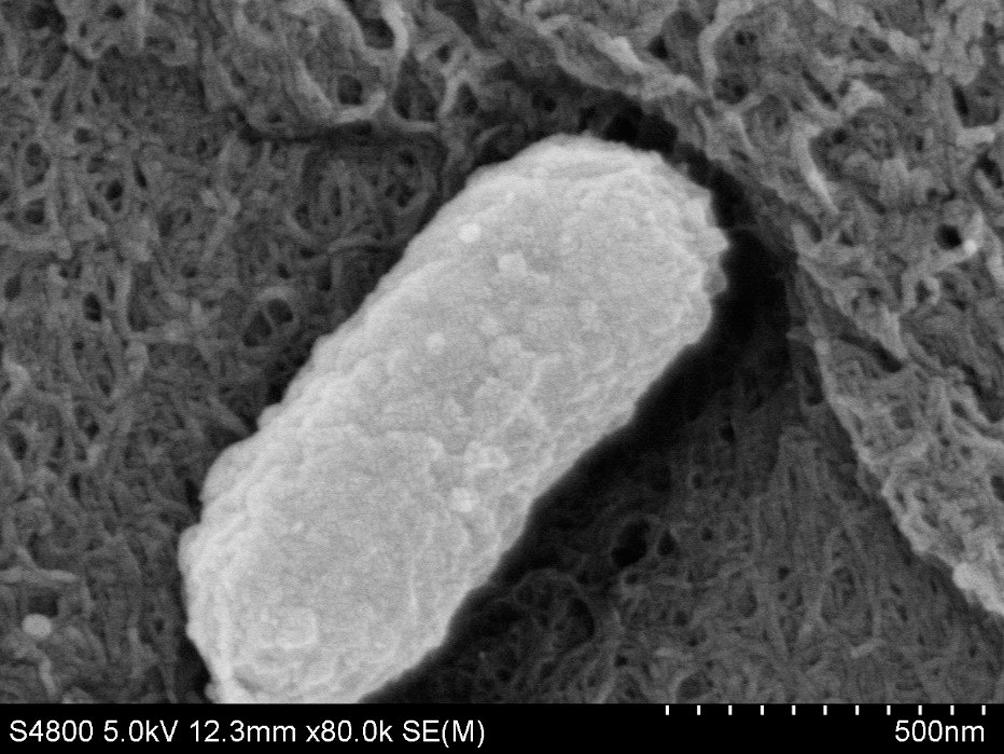
FIGURE 1. Scanning electron micrograph of strain C. proteolyticum QSH1265 cells from 12 h of culture. Strain QSH1265 is a medium rod-shaped bacterium with a length of 0.5 to 1.8 μm and a diameter of 0.3–0.6 μm. The scale bar, indicates 0.5 μm.
Growth Conditions and Genomic DNA Preparation
Chryseobacterium proteolyticum strain QSH1265 was grown in a culture medium consisting of 1.0% polypeptone, 0.2% yeast extract, and 0.1% MgSO4. Strains were cultured on nutrition agar plates and then inoculated in the above medium. The cells were collected after being aerobically incubated at 30°C for 12 h with reciprocal shaking at 200 r/min. The full genomic DNA of QSH1265 was extracted using the Microbial DNA extraction kit (Takara, Tokyo, Japan) according to the manufacturer’s instructions. Then 1% agarose gel electrophoresis and nanodrop spectrometer were used to detect the quality and quantity.
Genome Sequencing and Assembly
Chryseobacterium proteolyticum strain QSH1265 was selected to sequence using the PacBio Rs II single Molecule Real Time (SMRT) sequencing technology, the third-generation sequencing platform at the Personalbio Co., Ltd., in Shanghai, China. The full genome sequencing and sequence assembly was completed in 2016 and its genome sequence was deposited in GenBank (SRR7156726). The strain has been preserved in the China General Microbiological Culture Collection Centre (CGMCC). Basic genome sequencing information is shown in Table 2.
All the sequencing data were assembled using the software Hierarchical Genome assembly process (HGAP), to obtain contigs. Then Mummer software was used for co-linearity analysis, which compares the relative position of each contig in the genome. Next, the gaps between contigs were filled by Illumina Miseq, the second generation sequencing platform. Finally, the sequencing results were rectified using the software, Pilon.
Sub-Systematic Analysis and Functional Annotation of ORFs in Genome
Automated genome annotation was completed by the following ways: Gene ontology (GO) annotation was assigned to each of ORFs by Blast2GO software, which analyzed the best hits of the BLAST results (Conesa and Götz, 2008). Ortholog information and metabolic pathway annotation of protein coding genes are mainly completed by the KAAS automatic annotation system of Kyoto Encyclopedia of Genes, Genomes (KEGG) (Moriya et al., 2007). The whole genome coding DNA sequences (CDSs) and transfer RNAs were identified using the software, Glimmer 3.0 (Delcher et al., 1999) and tRNAscan-SE 1.4 (Lowe and Eddy, 1997). Ribosomal RNAs were predicted using RNAmmer1.2 (Lagesen et al., 2007). Directly repeated sequences (DRs) and spacers of the full genome sequences were predicted with the CRISPR recognition tool (Bland et al., 2007). Additionally, the gene island was predicted using IslandViewer (Langille and Brinkman, 2009), whereas signal peptide sequences and genes with transmembrane helices were predicted using the software SignalP 4.1 and TransMembrane prediction using Hidden Markov Models (TMHMM). Finally, the DNA sequence, gene and predicted non-coding RNA sequences were integrated into the standard GenBank format, then the circle map of the genome was drawn in CGView (Stothard and Wishart, 2005).
Results
16S rRNA Gene Sequence and Analysis
The 16S rRNA gene sequences of QSH1265 were compared with others from NCBI database using the Basic Local Alignment Search Tool (BLAST). After aligning the sequences using the Clustal X (v1.81) program, the phylogenetic tree was constructed with the neighbor-joining algorithm integrated in the MEGA 7.0 program. This showed that the clustering of strain QSH1265 was consistent with other species of the genus Chryseobacterium. Analysis of the 16S rRNA sequence revealed that QSH1265 is most closely related to C. proteolyticum strain 9670 (NR 112113.1) and it shares 99% homology and are grouped in one branch of the genus Chryseobacterium (Figure 2). QSH1265 was shown to be a distinct branch, but sharing 94% homology with the other strains, which also belong to the genus Chryseobacterium, such as Chryseobacterium meningosepticum (NR 115201.1), Chryseobacterium taiwanense (NR 043715.1). QSH1265 was clearly distant from Bergeyella zoohelcum (NR104718.1), a phylogenetically related species that is also a member of the Flavobacteriaceae family.
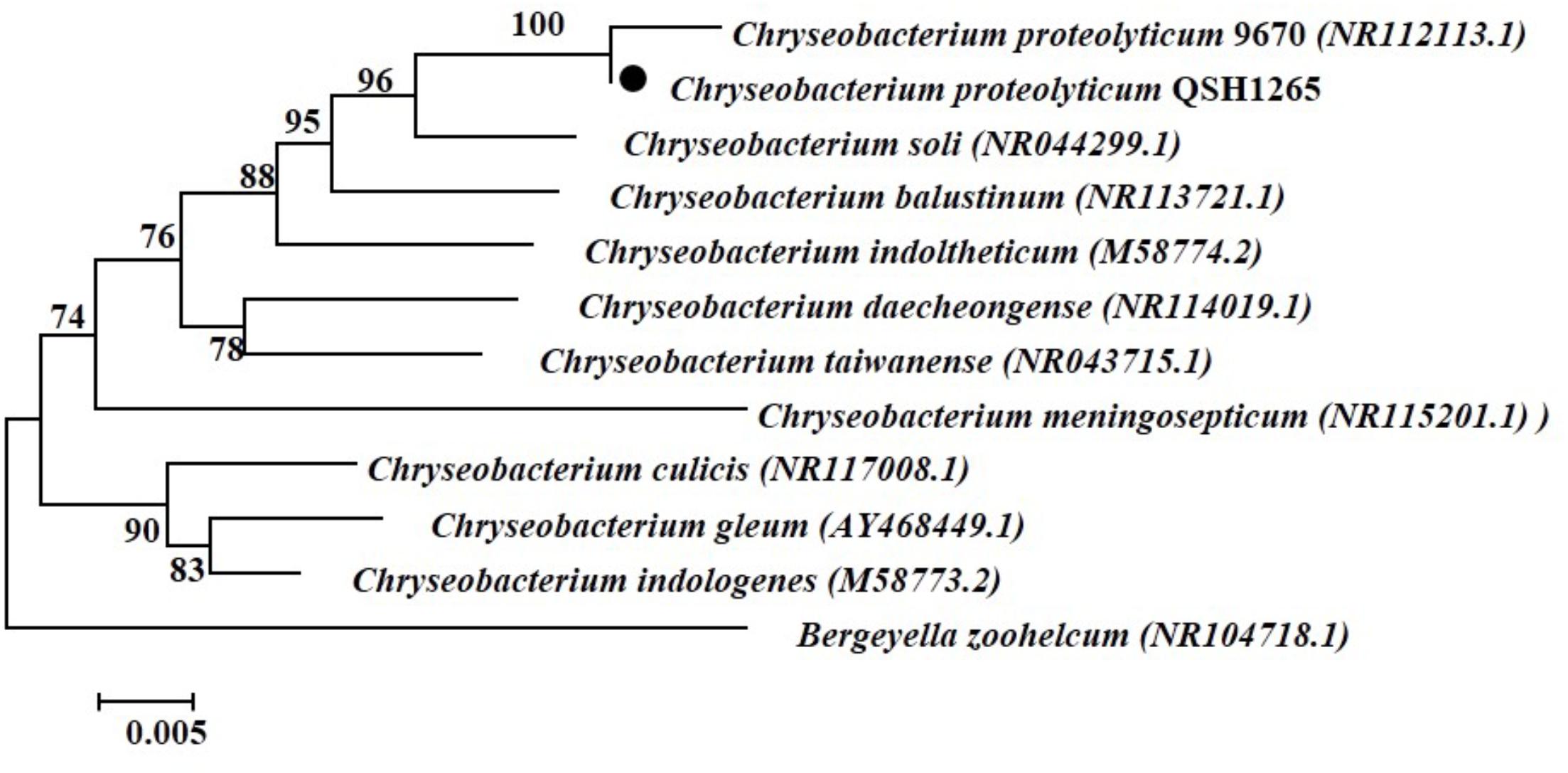
FIGURE 2. Phylogenetic tree showing the position of C. proteolyticum QSH1265 (), the type strains of other species in the genus Chryseobacterium, and the type strain of Bergeyella zoohelcum. The sequences were aligned with the CLUSTAL W program and were constructed with the neighbor-joining algorithm integrated in the MEGA 7.0 program. The phylogenetic tree was tested with 1000 bootstrap replicates. Bootstrap values are shown at the nodes. The GenBank accession numbers of the sequences are indicated the parentheses. The scale bar represents a 0.5% nucleotide sequence divergence.
Genome Properties
The whole genome of C. proteolyticum strain QSH1265 contains a single chromosome of 4,849,803 bp (Figure 3) and has an average G+C content of 36.16%. In total, 4563 genes were identified including 4459 protein-coding genes; in addition, 104 genes for RNA species-including 15 rRNA genes, 69 tRNA genes, and 20 ncRNA genes have been determined. The genome properties of QSH1265 are shown in Table 3. GO, KEGG and the clusters of orthologous genes (COG) annotation indicate the information of specific functional proteins. COG functional categories are listed in Table 4, in which 2675 of the identified genes were classified: 145 genes for translation, ribosomal structure and biogenesis, 221 genes for transcription, 121 genes for replication, recombination and repair, 16 genes for cell cycle control, cell division and chromosome partitioning, 58 genes for defense mechanisms, 127 genes for signal transduction mechanisms, and 851 genes for metabolism and so on. In addition, GO and KEGG functional categories are shown in Figure 4. Genome analysis revealed that C. proteolyticum strain QSH1265 had many functions although its genome size was relatively small. According to the analysis and forecast results of these genome, 4459 ORFs, 906 transmembrane helices, 489 signal peptides, 110 genomic islands were predicted.
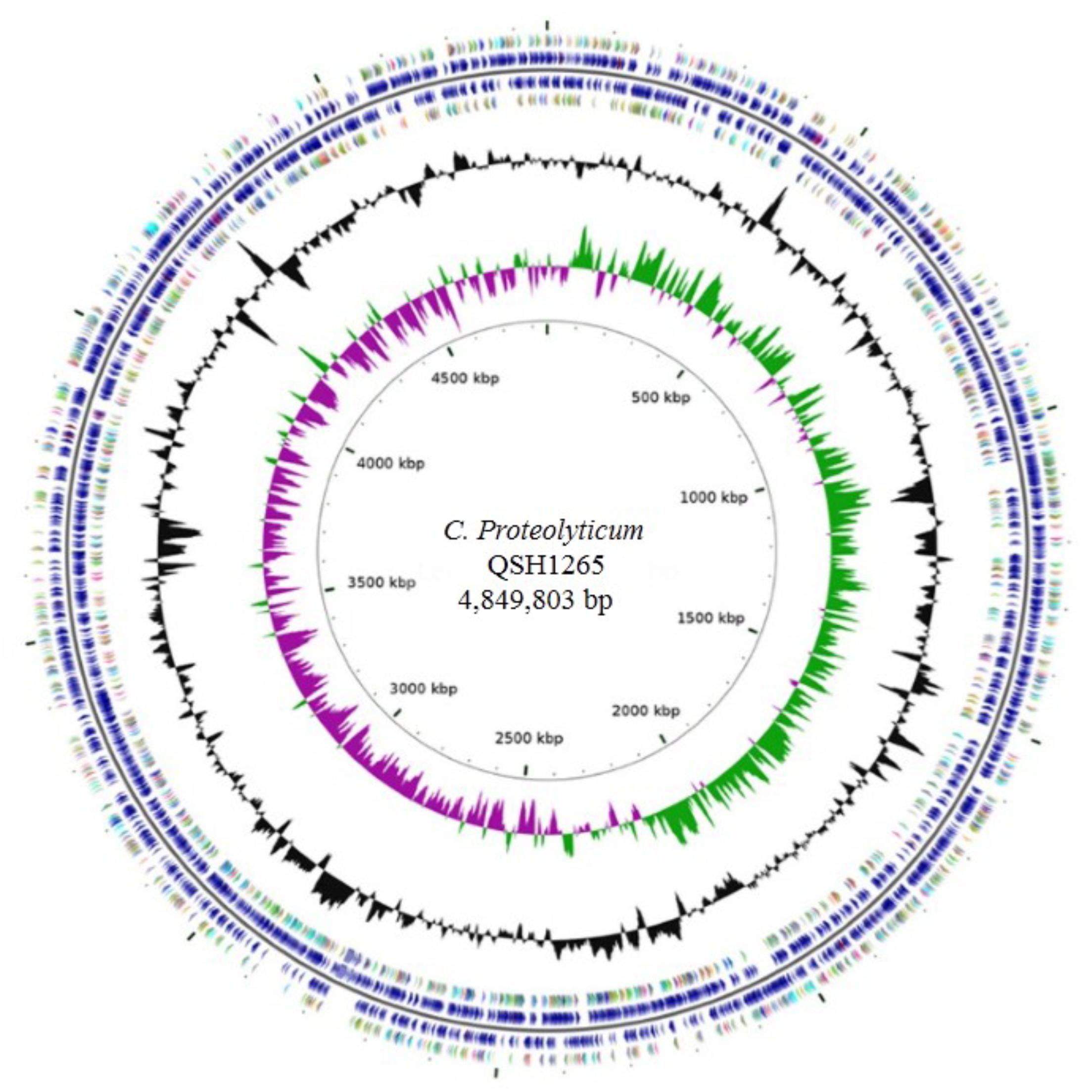
FIGURE 3. Graphical circular map of the chromosome of C. proteolyticum QSH1265. From center to the outside: the scales in the first circle indicate location in Mbp, strating with the initial coding region. The second circle shows the GC skew (in purple and green), and the value is plotted as the deviation from the average GC skew of the entire chromosome sequence. The bar in the third circle (in black and red) represents the GC content. The bars in the forth and seventh circle are colored according to COG function categories of CDS. Position of tRNA and rRNA are marked by brown and purple in the fifth and sixth circle, respectively.
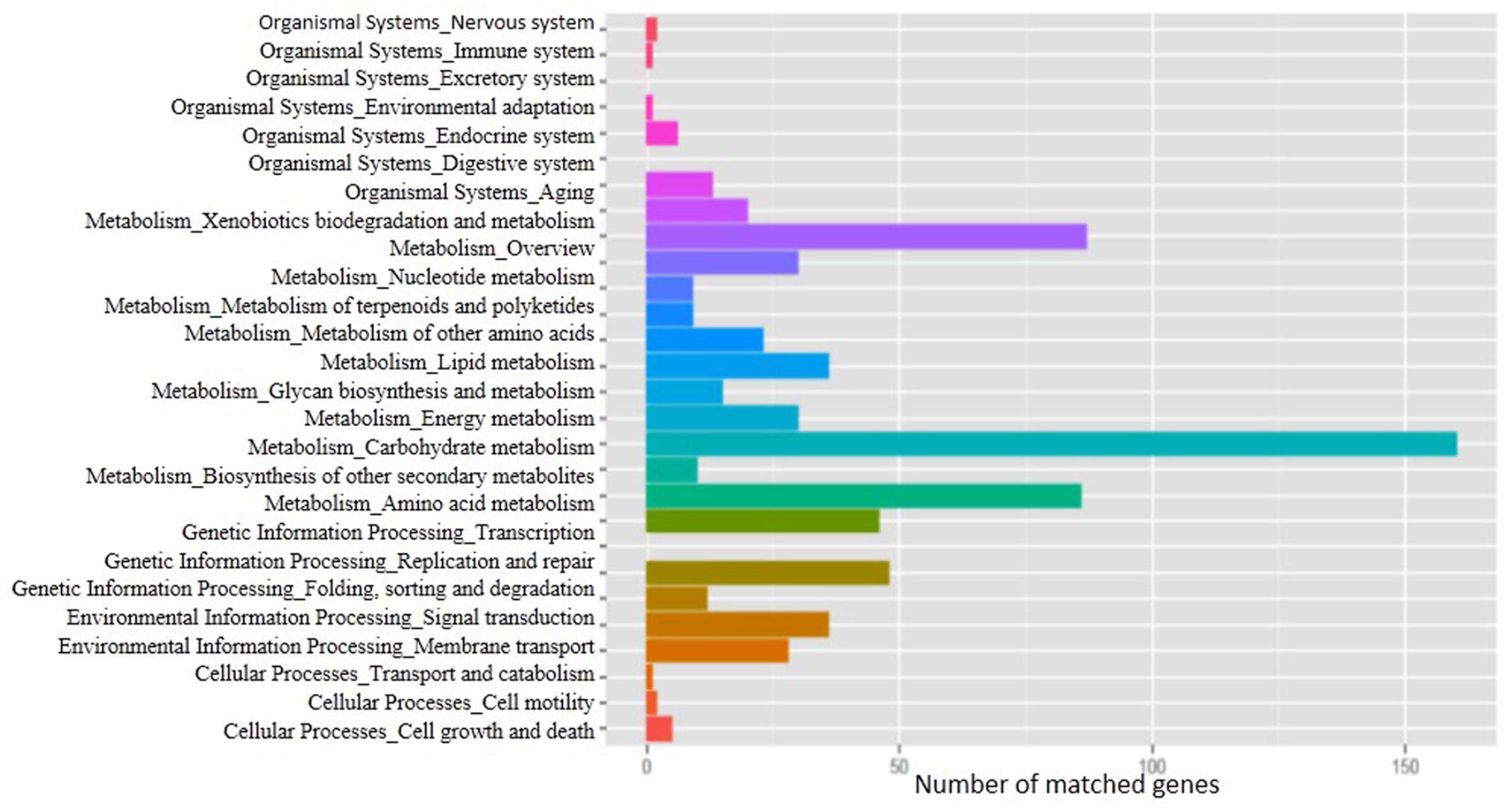
FIGURE 4. KEGG classification of C. proteolyticum QSH1265. A total of 872 genes were predicted using the KEGG pathway annotation. On the left is listed the classification of the functional genes. Different colors on the right side represent different metabolic pathways.
Analysis of Hypothetical Protein
A hypothetical protein located in the ORF of 3589 was predicted to be a highly conserved protein using the online analysis software program ProParam1. Analysis using the Softberry software2 suggested that it might share a promoter with the gene encoding the PG (the sequence was showed in Supplementary Figure S2). Analysis using SignalP 4.13 indicated that PG is likely expressed as a pre-pro-protein with a putative signal peptide of 22 amino acids, and a mature protein of 80 amino acids. The analysis of the signal peptide sequence through Protein Data Bank (PDB) database revealed that it is similar to anionic transporters and ATP-binding cassette (ABC) transporter substrate binding protein having respective homologies of 85 and 65%. ABC transporters are a group of integral membrane proteins which assist in transmembrane transport of various molecules (Snider et al., 2013). The result hints that this hypothetical protein may be associated with the secretory pathway of PG.
Methylation Modification Analysis
It is widely known that methylation modification is closely associated with gene transcription activity and significantly affects gene expression. Genome methylation modification and methyl-transferase recognition sequence motifs were analyzed using SMRT 2.3.0. In the chromosome, 322034 m4C (N4-methylcytosine), 17830 m6A (N6-methyladenine), and 1631972 other modified bases were marked as modified. Methylation analysis also demonstrates the methylation distribution of the individual genes or intergenic regions. Corresponding annotation information is included in Table 5.
Discussion
As mentioned above, PG is thought to be one of the most promising protein-deamidation enzymes and has been used to modify many proteins, including wheat gluten (Yong et al., 2006), α-Lactalbumin (Gu et al., 2001), α-zein (Yong et al., 2004), rice glutelin (Liu et al., 2011), skim milk (Miwa et al., 2010), soy protein (Suppavorasatit et al., 2011), yogurt (Miwa et al., 2014), and oat protein (Jiang et al., 2015). Miwa et al. (2013) investigated the effect of PG on heat-induced conformational changes in whey protein isolate and its relation to gel properties. Other researchers have focused on the protein structure changing, solubility, and flavor improvements induced and modified by PG (Cui et al., 2013; Suppavorasatit et al., 2013; Kunarayakul et al., 2017; Chen et al., 2018).
However, few studies about PG producing strains have been reported. Only characterization of a type strain C. proteolyticum 9670 has been published, which was isolated from soil collected in Japan by Yamaguchi and Yokoe (2000) in this strain’s enzyme producing ability was very poor, just up to 0.258 U/mL when using CBZ-Gln-Gly as a substrate. So far there’s no study reported the genome sequence of the strains.
In this study, we have reported, for the first time, the complete whole genome sequence of PG producing strain C. proteolyticum QSH1265, a soil bacterium isolated from Shanghai, China. The genome sequence of C. proteolyticum QSH1265 contains a single chromosome of 4,849,803 bp and has an average G+C content of 36.16%. In total, 4563 genes were identified including 4,459 protein-coding genes and 104 genes for RNA species. There is no plasmid in the genome, suggesting that it could be difficult to establish plasmid-based expression system. However, mapping the whole genome sequence provides valuable genetic information that can be exploited in directional mutation and transposon mutants library establishment (Chiang and Rubin, 2002). PG gene and its related genes were also detected. On the basis of this information, we could further explore some genetic tools to obtain high yield strains and reveal the bio-function of PG. Further research into resequencing (Li et al., 2009) and RNA-seq (Wang et al., 2009) of high yield strains is required to help the long-term study of C. proteolyticum and develop its potential use in the food industry.
Author Contributions
RQ and XZ designed and performed experiments, acquired data, wrote, revised, and approved final manuscript. These authors have contributed equally to this work. MT acquired data, drafted manuscript, and approved final manuscript. YL and JY interpreted data and approved final manuscript. WY acquired data, provide the material, and approved final manuscript. HG revised and approved final manuscript. JH conceived and supervised the project, secured funding, and revised and submitted the manuscript.
Funding
Informed consent was obtained from all individual participants included in the study. This work was supported by large instruments Open Foundation of East China Normal University (20152024) and Taixing Dongsheng Food Technology Ltd., China.
Conflict of Interest Statement
The authors declare that the research was conducted in the absence of any commercial or financial relationships that could be construed as a potential conflict of interest.
Supplementary Material
The Supplementary Material for this article can be found online at: https://www.frontiersin.org/articles/10.3389/fmicb.2018.01975/full#supplementary-material
Footnotes
- ^http://web.expasy.org/protparam/
- ^http://www.softberry.com/
- ^http://www.cbs.dtu.dk/services/SignalP/
References
Bland, C., Ramsey, T. L., Sabree, F., Lowe, M., Brown, K., Kyrpides, N. C., et al. (2007). CRISPR recognition tool (CRT): a tool for automatic detection of clustered regularly interspaced palindromic repeats. BMC Bioinformatics. 8:209. doi: 10.1186/1471-2105-8-209
Chen, B., Zhang, B., Li, M. N., Xie, Y., and Chen, H. Q. (2018). Effects of glutenin and gliadin modified by protein-glutaminase on pasting, rheological properties and microstructure of potato starch. Food Chem. 253, 148–155. doi: 10.1016/j.foodchem.2018.01.155
Chiang, S. L., and Rubin, E. J. (2002). Construction of a mariner-based transposon for epitope-tagging and genomic targeting. Gene 296, 179–185. doi: 10.1016/S0378-1119(02)00856-9
Conesa, A., and Götz, S. (2008). Blast2GO: a comprehensive suite for functional analysis in plant genomics. Int. J. Plant Genomics 2008:619832. doi: 10.1155/2008/619832
Cui, C., Hu, Q., Ren, J., Zhao, H., You, L., and Zhao, M. (2013). Effect of the structural features of hydrochloric acid-deamidated wheat gluten on its susceptibility to enzymatic hydrolysis. J. Agric. Food Chem. 61, 5706–5714. doi: 10.1021/jf400281v
Delcher, A. L., Harmon, D., Kasif, S., White, O., and Salzberg, S. L. (1999). Improved microbial gene identification with GLIMMER. Nucleic Acids Res. 27, 4636–4641. doi: 10.1093/nar/27.23.4636
Gu, Y. S., Matsumura, Y., Yamaguchi, S., and Mori, T. (2001). Action of protein-glutaminase on alpha-lactalbumin in the native and molten globule states. J. Agric. Food Chem. 49, 5999–6005. doi: 10.1021/jf010287z
Hamada, J. S., and Swanson, B. (1994). Deamidation of food proteins to improve functionality. Critic. Rev. Food Technol. 34, 283–292. doi: 10.1080/10408399409527664
Jiang, Z. Q., Sontag-Strohm, T., Salovaara, H., Sibakov, J., Kanerva, P., and Loponen, J. (2015). Oat protein solubility and emulsion properties improved by enzymatic deamidation. J. Cereal Sci. 64, 126–132. doi: 10.1016/j.jcs.2015.04.010
Kikuchi, M., Hayashida, H., Nakano, E., and Sakaguchi, K. (1971). Peptidoglutaminase. Enzymes for selective deamidation of gamma-amide of peptide-bound glutamine. Biochemistry 10, 1222–1229. doi: 10.1021/bi00783a019
Kunarayakul, S., Thaiphanit, S., Anprung, P., and Suppavorasatit, I. (2017). Optimization of coconut protein deamidation using protein-glutaminase and its effect on solubility, emulsification, and foaming properties of the proteins. Food Hydrocolloids 79, 197–207. doi: 10.1016/j.foodhyd.2017.12.031
Lagesen, K., Hallin, P., Rødland, E. A., Stærfeldt, H. H., Rognes, T., and Ussery, D. W. (2007). RNAmmer: consistent and rapid annotation of ribosomal RNA genes. Nucleic Acids Res. 35, 3100–3108. doi: 10.1093/nar/gkm160
Langille, M. G. I., and Brinkman, F. S. L. (2009). IslandViewer: an integrated interface for computational identification and visualization of genomic islands. Bioinformatics 25, 664–665. doi: 10.1093/bioinformatics/btp030
Li, R., Li, Y., Fang, X., Yang, H., Wang, J., Kristiansen, K., et al. (2009). SNP detection for massively parallel whole-genome resequencing. Genome Res. 19, 1124–1132. doi: 10.1101/gr.088013.108
Liu, X., Powers, J. R., Swanson, B. G., Hill, H. H., and Clark, S. (2010). High hydrostatic pressure affects flavor-binding properties of whey protein concentrate. J. Food Sci. 70, C581–C585. doi: 10.1111/j.1365-2621.2005.tb08308.x
Liu, Y., Li, X., Zhou, X., Yu, J., Wang, F., and Wang, J. (2011). Effects of glutaminase deamidation on the structure and solubility of rice glutelin. LWT – Food Sci. Technol. 44, 2205–2210. doi: 10.1016/j.lwt.2011.05.011
Lowe, T. M., and Eddy, S. R. (1997). tRNAscan-SE: a program for improved detection of transfer RNA genes in genomic sequence. Nucleic Acids Res. 25, 955–964. doi: 10.1093/nar/25.5.0955
Miwa, N., Nio, N., and Sonomoto, K. (2014). Effect of enzymatic deamidation by protein-glutaminase on the textural and microstructural properties of set yoghurt. Int. Dairy J. 36, 1–5. doi: 10.1016/j.idairyj.2013.12.002
Miwa, N., Yokoyama, K., Nio, N., and Sonomoto, K. (2013). Effect of enzymatic deamidation on the heat-induced conformational changes in whey protein isolate and its relation to gel properties. J. Agric. Food Chem. 61, 2205–2212. doi: 10.1021/jf3047626
Miwa, N., Yokoyama, K., Wakabayashi, H., and Nio, N. (2010). Effect of deamidation by protein-glutaminase on physicochemical and functional properties of skim milk. Int. Dairy J. 20, 393–399. doi: 10.1016/j.idairyj.2009.12.015
Moriya, Y., Itoh, M., Okuda, S., Yoshizawa, A. C., and Kanehisa, M. (2007). KAAS: an automatic genome annotation and pathway reconstruction server. Nucleic Acids Res. 35, 182–185. doi: 10.1093/nar/gkm321
Panyam, D., and Kilara, A. (1996). Enhancing the functionality of food proteins by enzymatic modification. Trends Food Sci. Technol. 7, 120–125. doi: 10.1016/0924-2244(96)10012-1
Scheuplein, R. J., Mizutani, A., and Yamaguchi, S. (2007). Studies on the non-pathogenicity of Chryseobacterium proteolyticum and on the safety of the enzyme: protein-glutaminase. Regul. Toxicol. Pharmacol. 49, 79–89. doi: 10.1016/j.yrtph.2007.06.001
Seo, W. H., Lee, H. G., and Baek, H. H. (2008). Evaluation of bitterness in enzymatic hydrolysates of soy protein isolate by taste dilution analysis. J. Food Sci. 73, S41–S46. doi: 10.1111/j.1750-3841.2007.00610.x
Shih, F. F., Hamada, J. S., and Marshall, W. E. (1992). Deamidation and Phosphorylation to Improve Protein Functionality in Foods. New York, NY: Springer US. doi: 10.1007/978-1-4684-8070-2_2
Snider, J., Hanif, A., Lee, M. E., Jin, K., Yu, A. R., Graham, C., et al. (2013). Mapping the functional yeast ABC transporter interactome. Nat. Chem. Biol. 9, 565–572. doi: 10.1038/nchembio.1293
Stothard, P., and Wishart, D. S. (2005). Circular Genome Visualization and Exploration Using CGView. Oxford: Oxford University Press.
Suppavorasatit, I., De Mejia, E. G., and Cadwallader, K. R. (2011). Optimization of the enzymatic deamidation of soy protein by protein-glutaminase and its effect on the functional properties of the protein. J. Agric. Food Chem. 59, 11621–11628. doi: 10.1021/jf2028973
Suppavorasatit, I., Lee, S. Y., and Cadwallader, K. R. (2013). Effect of enzymatic protein deamidation on protein solubility and flavor binding properties of soymilk. J. Food Sci. 78, C1–C7. doi: 10.1111/j.1750-3841.2012.03012.x
Wang, Z., Gerstein, M., and Snyder, M. (2009). RNA-Seq: a revolutionary tool for transcriptomics. Nat. Rev. Genet. 10, 57–63. doi: 10.1038/nrg2484
Yamaguchi, S., Jeenes, D. J., and Archer, D. B. (2001). Protein-glutaminase from Chryseobacterium proteolyticum, an enzyme that deamidates glutaminyl residues in proteins. Purification, characterization and gene cloning. Eur. J. Biochem. 268, 1410–1421. doi: 10.1046/j.1432-1327.2001.02019.x
Yamaguchi, S., and Yokoe, M. (2000). A novel protein-deamidating enzyme from Chryseobacterium proteolyticum sp. nov., a newly isolated bacterium from soil. Appl. Environ. Microbiol. 66, 3337–3343. doi: 10.1128/AEM.66.8.3337-3343.2000
Yong, Y. H., Yamaguchi, S., Gu, Y. S., Mori, T., and Matsumura, Y. (2004). Effects of enzymatic deamidation by protein-glutaminase on structure and functional properties of alpha-zein. J. Agric. Food Chem. 52, 7094–7100. doi: 10.1021/jf040133u
Keywords: Chryseobacterium proteolyticum, protein-glutaminase, deamidation, third-generation sequencing, annotation
Citation: Qu R, Zhu X, Tian M, Liu Y, Yan W, Ye J, Gao H and Huang J (2018) Complete Genome Sequence and Characterization of a Protein-Glutaminase Producing Strain, Chryseobacterium proteolyticum QSH1265. Front. Microbiol. 9:1975. doi: 10.3389/fmicb.2018.01975
Received: 08 June 2018; Accepted: 06 August 2018;
Published: 04 September 2018.
Edited by:
Chong Zhang, Nanjing Agricultural University, ChinaReviewed by:
Amy Michele Grunden, North Carolina State University, United StatesWang Lei, Northeast Electric Power University, China
Copyright © 2018 Qu, Zhu, Tian, Liu, Yan, Ye, Gao and Huang. This is an open-access article distributed under the terms of the Creative Commons Attribution License (CC BY). The use, distribution or reproduction in other forums is permitted, provided the original author(s) and the copyright owner(s) are credited and that the original publication in this journal is cited, in accordance with accepted academic practice. No use, distribution or reproduction is permitted which does not comply with these terms.
*Correspondence: Jing Huang, amh1YW5nQGJpby5lY251LmVkdS5jbg==
†These authors have contributed equally to this work