- 1Instituto de Farmacología y Morfofisiología, Facultad de Ciencias Veterinarias, Universidad Austral de Chile, Valdivia, Chile
- 2Instituto de Investigación en Ciencias Odontológicas, Facultad de Odontología, Universidad de Chile, Santiago, Chile
- 3Escuela de Química y Farmacia, Facultad de Farmacia, Universidad de Valparaíso, Valparaíso, Chile
- 4Centro de Investigación Farmacopea Chilena, Universidad de Valparaíso, Valparaíso, Chile
- 5Programa de Farmacología Molecular y Clínica, Instituto de Ciencias Biomédicas, Facultad de Medicina, Universidad de Chile, Santiago, Chile
Pathogenic trypanosomatids (Trypanosoma cruzi, Trypanosoma brucei, and Leishmania spp.) are protozoan parasites that cause neglected diseases affecting millions of people in Africa, Asia, and the Americas. In the process of infection, trypanosomatids evade and survive the immune system attack, which can lead to a chronic inflammatory state that induces cumulative damage, often killing the host in the long term. The immune mediators involved in this process are not entirely understood. Most of the research on the immunologic control of protozoan infections has been focused on acute inflammation. Nevertheless, when this process is not terminated adequately, permanent damage to the inflamed tissue may ensue. Recently, a second process, called resolution of inflammation, has been proposed to be a pivotal process in the control of parasite burden and establishment of chronic infection. Resolution of inflammation is an active process that promotes the normal function of injured or infected tissues. Several mediators are involved in this process, including eicosanoid-derived lipids, cytokines such as transforming growth factor (TGF)-β and interleukin (IL)-10, and other proteins such as Annexin-V. For example, during T. cruzi infection, pro-resolving lipids such as 15-epi-lipoxin-A4 and Resolvin D1 have been associated with a decrease in the inflammatory changes observed in experimental chronic heart disease, reducing inflammation and fibrosis, and increasing host survival. Furthermore, Resolvin D1 modulates the immune response in cells of patients with Chagas disease. In Leishmania spp. infections, pro-resolving mediators such as Annexin-V, lipoxins, and Resolvin D1 are related to the modulation of cutaneous manifestation of the disease. However, these mediators seem to have different roles in visceral or cutaneous leishmaniasis. Finally, although T. brucei infections are less well studied in terms of their relationship with inflammation, it has been found that arachidonic acid-derived lipids act as key regulators of the host immune response and parasite burden. Also, cytokines such as IL-10 and TGF-β may be related to increased infection. Knowledge about the inflammation resolution process is necessary to understand the host–parasite interplay, but it also offers an interesting opportunity to improve the current therapies, aiming to reduce the detrimental state induced by chronic protozoan infections.
Overview of Arachidonic Acid Metabolism and the Pro-Resolving Lipid Mediators
Inflammation is a pathophysiologic process that occurs in the context of broad spectra of stimuli and diseases including arthritis, asthma, trauma, and infection. During acute infection, inflammation is protective, but if it is excessive or prolonged, it harms the host, damaging tissues and impairing proper repair, and in extreme cases, it can be lethal. Repair and restoration of normal organ function are essential after an infectious disease, and these processes are accomplished after the inflammatory events are appropriately resolved. However, resolution of inflammation is a more intricate process than the mere dissipation of chemoattractant signals. It includes a set of complex events mediated by several signals, including negative feedback regulation of Toll-like receptor (TLR) signaling, production of anti-inflammatory cytokines such as interleukin (IL)-10, and biosynthesis of a superclass of novel mediators. These newly discovered mediators include biochemical species derived from lipids such as lipoxins (LXs), resolvins (Rvs), protectins (PDs), and maresins (Serhan, 2005), proteins such as Annexin A1 (Sugimoto et al., 2016) and Galectin-1 (Sundblad et al., 2017), anti-inflammatory neuropeptides such as melanocortin (MC) peptide (Delgado and Ganea, 2008; Alessandri et al., 2013), and gasotransmitters such as hydrogen sulfide and carbon monoxide (Wallace et al., 2015; Shinohara and Serhan, 2016). The concerted actions of these molecules stop leukocyte recruitment, modify cytokine production, facilitate efferocytosis, switch macrophages to a non-phlogistic phenotype, and finally, promote healing to restore organ function (Serhan, 2014).
Specialized pro-resolving mediators (SPMs), including the pro-resolving lipids, are produced via cell–cell interactions within the inflammatory exudates that control the magnitude and duration of local inflammation (Serhan and Chiang, 2013). SPMs are all products of the lipoxygenase (LO) pathway, though the lipid substrates vary (Figure 1).
SPMs Synthesis
Lipoxins
Lipoxins are eicosanoids derived from omega-6 arachidonic acid (AA), which is oxygenated at position 15 by 15-LO activity to produce 15S-hydroperoxyeicosatetraenoic acid (15S-H(p)ETE). The product of 5-LO’s action on 15-HpETE is a 5S-hydroperoxy,15S-hydro(peroxy)-DiH(p)ETE, which is rapidly converted to 5(6)-epoxytetraene. In turn, 5(6)-epoxytetraene is rapidly hydrolyzed to lipoxin A4 (LXA4) and LXB4 (Serhan, 2005). Alternatively, acetylsalicylic acid (ASA)-acetylated cyclooxygenase 2 (COX-2) produces 5-R-Hydroxyeicosatetraenoic acid (5-RHETE) from AA. 5-RHETE is a substrate of 5-LO that can be converted to 15-epi-lipoxin A4 (15-epi-LXA4), which is also named ASA-triggered lipoxin (ATL) (Serhan, 2005).
Resolvins
On the other hand, Rvs, PDs, and maresins are derived from omega-3 polyunsaturated fatty acids. There are two series of Rvs depending on the lipid substrate and enzyme activities: Rv D and E. The RvD1–4 series, as well as PDs and maresins, are derived from docosahexaenoic acid (DHA) metabolism involving 12-LO and 15-LO, and the E-series Rvs are derived from the activity of ASA-acetylated COX-2 using eicosapentaenoic acid (EPA) as a substrate (Serhan, 2007). Nevertheless, ASA can also trigger the COX-2-mediated conversion of DHA to 17R-HDHA, which 5-LO can covert to ASA-triggered Resolvin D1–4 (AT-RvD1–4) (Serhan, 2007).
Drug Induction of SPMs
As mentioned, ASA modifies COX-2 activity allowing 15-epi-LXA4 and AT-RvD1–4 production. Indeed, ASA-triggered resolving lipids are more stable than the endogenous molecules and could serve as anti-inflammatory drugs. Another fascinating group of drugs, statins, can also increase the production of pro-resolving lipids, in a way that is similar to the process mediated by ASA. Just as the acetylation of COX-2 by ASA causes a metabolic switch in COX-2, statins produce the same metabolic switch by nitrosylation. Recently, Serhan’s group reported the presence of another subtype of Rvs, 13-series Rvs, which provide protective activity against Escherichia coli infections. These lipids are produced by COX-2 in a process involving a neutrophil–endothelium interaction, and their production can also be triggered by nitrosylation induced by atorvastatin (Dalli et al., 2015). Thus, the change in COX-2 activity explains, at least in part, the anti-inflammatory properties of ASA and statins.
The Inflammation Resolution Crossroad
It is remarkable how the same enzymatic array participates in the generation of both inflammatory and resolving mediators. COX and LO activities, which are responsible for the production of prostaglandins (PGs) and leukotrienes, respectively, can switch to the production of LX, Rvs, PDs, and maresins. Indeed, AA derivatives, PGs E2 (PGE2), prostacyclin (PGs I2, PGI2), and leukotriene B4 (LTB4), participate in leukocyte recruitment to the damaged site. However, as acute inflammation progresses, a metabolic switch occurs, and LX synthesis begins. The exact moment when this switch happens is unclear; however, the dampening of the inflammatory signals may be part of the input needed to promote the metabolic changes (Gilroy and De Maeyer, 2015). These switches are accomplished by transcriptional or posttranslational modifications, which involve PGE2 and PGs D2 (PGD2) (Frolov et al., 2013; Croasdell et al., 2015). COX-mediated production of PGD2 by human PGD2 synthase (hPGD2s) activates the PGD2 receptor, DP1 (a G-protein coupled receptor [GPCR]), which in turn stimulates the production of IL-10 (an anti-inflammatory cytokine), which then blocks the path to chronic inflammation. PGD2 can also be converted to PGs J2 (PGJ2) and 15-deoxy-Δ(12,14)-PGs J2 (15-D-PGJ2) products that activate peroxisome proliferator-activated receptor (PPAR)-γ to promote resolution (Croasdell et al., 2015).
SPMs Mode of Action
The actions of LXA4 are mediated by a GPCR called formyl peptide receptor 2/lipoxin A4 receptor (FPR2/ALXR), via several signaling pathways, including the p38/mitogen-activated protein kinase (MAPK)-activated protein kinase (APK)/heat shock protein 27 (HSP27), c-Jun N-terminal kinase (JNK), and phosphatidylinositol 3-kinase (PI3K) pathways (Cooray et al., 2013), depending on the cell type. In monocytes and macrophages, LXA4 triggers the synthesis of IL-10 (Souza et al., 2007), a cytokine responsible for driving the resolution of inflammation, and enhances non-phlogistic phagocytosis of apoptotic cells (Maderna et al., 2010). Also, some effects of LXA4 are related to its ability to activate the cytosolic aryl hydrocarbon receptor (AhR), inducing the expression of suppressor of cytokine signaling (SOCS) (Machado et al., 2006).
The action of Resolvin E1 (RvE1) is intricate because it is an agonist of the seven-pass transmembrane GPCR ChemR23. Naturally, the activation of ChemR23 by low concentrations of chemerin, its natural agonist, favors chemoattraction of monocytes/macrophages and immature dendritic cells (DCs). Activation of ChemR23 increases intracellular calcium release and inhibits cAMP and MAPK extracellular signal-regulated protein kinases 1/2 (ERK1/2)-mediated signaling, by Gi/o protein (a G protein subtype) recruitment. Consequently, it leads to up-regulation of the PI3K/Akt signaling pathway and down-regulation of nuclear factor kappa-light-chain-enhancer of activated B cells (NF-κB) (Mariani and Roncucci, 2015). RvE1, via a different set of G proteins, stimulates Akt phosphorylation and ribosomal protein S6 kinase (Ohira et al., 2010). Also, RvE1 is a partial agonist of the LTB4 receptor 1 (BLT1) and competes with LTB4 for binding (Arita et al., 2007).
RvD1 can activate FRP2/ALXR and the G protein-coupled receptor 32 (GPR32) (Krishnamoorthy et al., 2012). The activation of the FPR2/ALX receptor by RvD1 suppresses cytosolic calcium and decreases activation of the calcium-sensitive kinase calcium-calmodulin-dependent protein kinase II (CaMKII). CaMKII inhibition suppresses activation of p38 and the MAP kinase-APK 2 (MAPK-APK2), which reduces Ser271 phosphorylation of 5-LO and shifts 5-LO from the nucleus to the cytoplasm (Fredman et al., 2014). Moreover, LXA4 and RvE1 counter-regulate the LTB4/LL-37 proinflammatory circuit, which is partially mediated by FPR2/ALX (Wan et al., 2011).
Physiologic Actions of SPMs
LXA4 decreases polymorphonuclear leukocyte (PMN)-mediated tissue damage, angiogenesis, and PMN proliferation and adhesion, increasing non-phlogistic phagocytosis and IL-10 production (Chandrasekharan and Sharma-Walia, 2015). RvE1 increases PMN apoptosis, LXA4 production, and microbial killing, and decreases IL-12 production, PMN transendothelial migration, and PMN infiltration; also, it inhibits NF-κB reporter gene activation and reduces organ fibrosis (Arita et al., 2007; Campbell et al., 2007; El Kebir et al., 2012). RvD1 decreases reactive oxygen species (ROS) generation and the production of pro-inflammatory cytokines. RvD1 also reduces organ fibrosis (Qu et al., 2012; Miyahara et al., 2013). RvD2 increases microbial killing and clearance, as well as the production of nitric oxide (NO∙) and PGI2 in endothelial cells (Spite et al., 2009; Chiang et al., 2012). PD1 decreases COX-2 expression, T-cell migration, and cytokine production, including that of TNF, IFN-γ, and microglial cell cytokines (Ariel et al., 2005; Qu et al., 2015). Also, PD1 has neuroprotective actions (Serhan et al., 2015). Maresins increase tissue regeneration, stimulating phagocytosis and the killing of oral pathogens by human leukocytes, and also promote the macrophage phenotype switch from M1 to M2 (Wang et al., 2015a). It is important to emphasize that both Rvs and LXs promote bacterial clearance; thus, they both constitute a pathway that impedes microorganism evasion of the immune response. Thus, the concert of the SPMs in the inflammatory broth give a change of helm heading toward a calmer environment, where leukocyte and macrophage hyperactivity decline, promoting a favorable environment for the repair.
Role of SPMs in Chronic Infectious Diseases
Infections may progress toward chronicity due to dysregulation of the inflammatory process. Thus, extensive damage may occur involving irreversible structural alterations. Furthermore, it has been shown that in chronic inflammatory states such as Alzheimer’s disease (Wang et al., 2015b), periodontitis (Van Dyke, 2017), peripheral artery disease (Miyahara et al., 2013), or cystic fibrosis (Karp et al., 2004), the levels of pro-resolving factors such as LXA4, or ATLs are decreased. Therefore, it is suggested that a deficit of resolutory ability promotes chronic inflammatory states. Evidence of the participation of lipid pro-resolving mediators in infectious diseases is abundant. For example, in experimental models of lung infections (e.g., disseminated influenza A), there is low expression of LX (Cilloniz et al., 2010), and exogenous administration of PD1 improves survival (Morita et al., 2013). Moreover, when inflammation persisted in a respiratory syncytial virus lung infection, and exogenous administration of LXs or Rvs reverses the inflammation (Shirey et al., 2014). In a self-resolving murine model of E. coli pneumonia, exogenous AT-RvD1 administration enhanced the clearance of E. coli and Pseudomonas aeruginosa in vivo, and lung macrophage phagocytosis ex vivo (Abdulnour et al., 2016). These findings were similar to the results of a lung coinfection model with Streptococcus pneumoniae and influenza A virus (Wang et al., 2017), which showed that AT-RvD1 decreased the inflammatory drive by acting on the FPR2/ALX receptor and antagonizing the effect of serum amyloid A, which is an agonist of this receptor. Thus, there is a clear relationship between bacterial or viral infection and SPMs generation.
However, the LXs-bacterial interplay in a chronic infection may be more complicated. In a mouse model of Mycobacterium tuberculosis infection, 5-LO knockout mice were more likely to survive compared with wild-type mice, which had a more protracted disease evolution. Administration of LXA4 inhibited the development of a Th1 response, which is protective in the tuberculosis model (Bafica et al., 2005). Bacteria may also inhibit the resolution of infectious processes. A recent report demonstrated that chronic P. aeruginosa infections in cystic fibrosis patients promote the hydrolysis of an endogenous epoxide-containing eicosanoid 14,15-epoxyeicosatrienoic acid (14,15-EET) to its corresponding diol, thereby destroying the signal that triggers increased biosynthesis of 15-epi-LXA4 and preventing the activation of resolution pathways that could help to eradicate the infection (Flitter et al., 2017).
Specialized pro-resolving mediators may contribute to eradicate infections and terminate the inflammatory input, as it is suggested by murine sepsis models, where there was reported that LXA4 decreased plasma IL-6, chemokine (C–C motif) ligand 2 (CCL2), IL-10, and NF-κB activity in peritoneal macrophages, reduced neutrophil migration, and increased the clearance of bacteria by neutrophils without the production of excessive free radicals (Walker et al., 2011; Wu et al., 2015). Furthermore, LX may increase the production of antibacterial proteins. Bactericidal/permeability-increasing protein (BPI) is increased in an in vitro model of Salmonella typhimurium infection when LXs are administered (Canny et al., 2002). Thus, LX may have a role in the clearance of pathogenic microorganisms. Interestingly, LXA4 decreases the release of the exotoxin pyocyanin by P. aeruginosa, reducing its pathogenicity (Wu et al., 2016). Thus, LXA4 also affects infective agents. Furthermore, RvD1 and RvD5 were shown to decrease the dose of antibiotics needed to treat E. coli or Staphylococcus aureus infections (Chiang et al., 2012). Thus, RvD1 and RvD5 can help to clear bacteria and, most importantly, this research provides new insight into how to circumvent antibiotic resistance (Chiang et al., 2012).
In addition to its action in bacterial infections, LXA4 plays a role in acute and chronic parasitic infections. However, it is controversial because in murine models of cerebral malaria, LXA4 is associated with a lower parasite burden, less cerebral inflammation, and better survival (Shryock et al., 2013). However, in a murine model of Toxoplasma gondii infection, although LXA4 production is increased and there is less cerebral inflammation (due to decreased IL-12 levels), the immune response against the parasite is dampened (Aliberti et al., 2002). Probably, this despair results are due to the distinct causative organism or by the inflammatory context where LXA4 was produced.
Trypanosoma cruzi Infections
Trypanosoma cruzi is a flagellate protozoan that causes Chagas disease (CD). The life cycle of the parasite includes survival inside muscle cells, including cardiac muscle and smooth muscle of the gastrointestinal tract (Rassi et al., 2012). The infection process involves an intense inflammatory response, which is coordinated by pro-inflammatory mediators such as PGs (Celentano et al., 1995; Cardoni and Antunez, 2004), leukotrienes, cytokines, and chemokines that increase the expression of endothelial cell adhesion molecules (ECAMs), allowing the migration of immune cells to the infection site (Golias et al., 2007). The increase in ECAMs induces vascular permeability and leukocyte recruitment (Gomes et al., 2014). These events are pivotal in the pathogenesis of chronic Chagas cardiomyopathy (CCC, the most lethal form of CD) because they facilitate leukocyte adhesion to cardiac endothelial cells and cause endothelial dysfunction. In turn, endothelial dysfunction is associated with focal ischemic events and microvascular abnormalities. Additionally, in CCC, the observed microvascular damage is worsened by platelet aggregation, which is activated by thromboxane A2 (TXA2), promoting a procoagulant environment that could exacerbate the focal ischemia (Cardoni and Antunez, 2004; Abdalla et al., 2008; Rossi et al., 2010; Prado et al., 2011). Thus, if left untreated, low-grade myocarditis initially ensues and the infection progresses from the acute to the chronic stage without necessarily involving clinical manifestations. As the infection and endothelial dysfunction persist, a myocardial remodeling process is launched, establishing CCC. Consequently, 30% of infected individuals develop cardiac complications, which can induce death by heart failure (Rassi et al., 2012; Ribeiro et al., 2012).
Role of SPMs in Acute Chagas Disease
Among the acute inflammatory mediators, AA derivatives have been described as essential drivers of the acute infection process and the chronic cardiac damage induced by the parasite. Experimental in vivo models of infection with T. cruzi showed an increase in the expression of COX-2 (Molina-Berrios et al., 2013a) and PGs E receptor 2 (EP2) in cardiac tissue (Guerrero et al., 2015). These proteins are involved in the synthesis and activity of AA derivatives, such as PGE2, TXA2, PGF2α, 6-oxo-PGF1α, LTB4, and other eicosanoids that have been observed increased after T. cruzi infection. Consequently, cells derived from T. cruzi-infected mice shows elevated levels of PGE2 (Celentano et al., 1995; Borges et al., 1998; Freire-de-Lima et al., 2000; Guerrero et al., 2015). Also, PGE2, TXA2, PGI2, PGF2α, and LTB4 levels have been found increased in plasma of mice infected with T. cruzi (Cardoni and Antunez, 2004; Molina-Berrios et al., 2013a,b; Sharma et al., 2013). Also, it is well-documented that T. cruzi itself can synthesize AA derivatives like TXA2, PGE2, and PGF2α by the action of the T. cruzi old yellow enzyme (TcOYE) (Kubata et al., 2002; Ashton et al., 2007). There is evidence that both, host- and parasite-derived autacoids contribute importantly to cardiac remodeling. In fact, COX-2, PLA2γ, or 5-LO deficient mice, infected with T. cruzi, exhibited improved survival rate and reduced cardiac tissue inflammation when compared with wild-type mice (Celentano et al., 1995; Borges et al., 1998; Cardoni and Antunez, 2004). Furthermore, there is evidence indicating that T. cruzi induces the formation of lipid bodies, specialized organelles where PGs synthesis occurs, being an important strategy for parasite growth and survival (Melo et al., 2003; D’Avila et al., 2011). The effect of the host or parasite-derived AA derivatives in the acute infection onset has been reviewed extensively elsewhere (Machado et al., 2011).
15-D-PGJ2 Is Pro-resolving in T. cruzi Infection
An aspect of growing interest is the implication of the PPAR-γ pathway in the modulation of inflammatory processes in chronic infections (Kim et al., 2015). In this regard, this pathway is related to the decrease in the transcription of genes controlled by NF-κB and the activator protein-1 (AP-1). It is well known that 15-D-PGJ2, which is a pro-resolving lipid derived from PGD2, acts as an agonist of PPAR-γ (Paumi et al., 2004). Therefore, it may have a role in pro-resolving processes in conjunction with other SPMs. Consequently, 15-D-PGJ2 has been tested as a modulator of acute and chronic heart inflammation in T. cruzi infection. 15-D-PGJ2 (1 mg/kg) decreases the inflammatory infiltrate and amastigote nest count and significantly increases the IL-10 levels (Rodrigues et al., 2010). Also, 15-D-PGJ2 attenuates acute liver damage induced by T. cruzi in mice. In this model, 15-D-PGJ2 was able to decrease fibrosis and liver damage without influencing the course of the infection itself (Penas et al., 2016). Moreover, a preliminary report from the same group suggested that treatment with 15-D-PGJ2 could regulate the number of intracellular amastigotes in the cardiac tissue via PPAR-γ-dependent and PPAR-γ-independent pathways (Penas et al., 2013).
Acetylsalicylic Acid in Acute and Chronic Chagas Disease
Due to the role of AA derivatives in T. cruzi infection, inhibition of COX activity has been proposed as a strategy for controlling parasite-induced disease. Although several non-steroidal anti-inflammatory drugs (NSAIDs), such as indomethacin, meloxicam, and celecoxib, have been assayed in mouse models of T. cruzi infection (Freire-de-Lima et al., 2000; Michelin et al., 2005; Abdalla et al., 2008; Hideko Tatakihara et al., 2008), the most studied NSAID is ASA, also known as aspirin. Interestingly, the effect of ASA seems to be mouse-species dependent. When T. cruzi-resistant mice (C57BL/6 or CD-1) are treated with ASA, parasitemia and mortality increases (Michelin et al., 2005; Hideko Tatakihara et al., 2008; Mukherjee et al., 2011). On the other hand, Balb/c mice, which are sensitive to T. cruzi infection, become healthy when ASA is administrated (Freire-de-Lima et al., 2000; Paiva et al., 2007; Hideko Tatakihara et al., 2008). Importantly, C57BL/6 mice generate more NO• than Balb/c mice after T. cruzi infection, and ASA treatment induces NO• production in Balb/c mice infected with T. cruzi (Hideko Tatakihara et al., 2008). Furthermore, in murine macrophages infected with T. cruzi, the inhibition of NO• synthesis partially prevents the effect of ASA (Lopez-Munoz et al., 2010), suggesting an important role for this mediator in the ASA effect.
More importantly, the effect of ASA has an important relationship with its dose. Most studies investigating ASA have been performed using a fixed dose of 25–50 mg/kg. However, studies using higher doses (>75 mg/kg) have shown no effect, or they have shown that ASA aggravates the damage caused by intraperitoneal or oral T. cruzi infection (Molina-Berrios et al., 2013a; Cossentini et al., 2016). The fact that ASA has an antichagasic effect only at doses <50 mg/kg has been associated to the production of 15-epi-LXA4, an ASA-triggered LX found in patients treated with low doses of ASA (Chiang et al., 2004). In Balb/c T. cruzi-infected mice, 25 and 50 mg/kg of ASA induced a significant increase in 15-epi-LXA4 production without modification of LTB4 levels. At these doses, the mice had prolonged survival, decreased mortality, and less cardiac inflammatory infiltrate. Additionally, the administration of 25 mg/kg exogenous 15-epi-LXA4 significantly decreased the parasitemia peaks and cardiac parasite load, improved the survival of the infected mice, and partially reversed the detrimental effect of high-dose ASA (Molina-Berrios et al., 2013a). Low doses of ASA also improve the vascular reactivity of mice infected with T. cruzi. Molina-Berrios et al. (2013b) evaluated the effect of ASA at 2 and 40 mg/kg and found that both regimens decreased ECAM expression and the TXA2 level. Also, 2 mg/kg/day ASA reduced the inflammatory infiltrate in mice hearts and improved the cardiac histology at 90 days post infection. Furthermore, in in vitro cardiac cells infected with T. cruzi, low doses of aspirin increased IL-1β and NO• release, and decreased transforming growth factor (TGF)-β release, and these effects disappeared when ASA concentrations were increased (Malvezi et al., 2014b).
Consistently, ATLs not only participate in the resolution of the damage produced by T. cruzi but also in the clearance of the pathogen. In an in vitro model of T. cruzi-infected macrophages, the addition of 0.3–1.25 mM of ASA significantly decreased the internalization of T. cruzi without altering macrophage viability (Carvalho de Freitas et al., 2017). Also, it has been reported that 0.625–2.5 mM of ASA decreased the internalization of T. cruzi and increased the release of IL-15 and NO•. However, co-administration of celecoxib (a COX-2 selective inhibitor) reverted the ASA effect and restored the invasive capacity of trypomastigotes, suggesting that functional COX-2 is necessary for the ASA effect. Furthermore, Boc-2 (a specific antagonist of the FRP2/ALX receptor) prevented the ASA effect, suggesting that the inhibition of invasion depends on the synthesis of 15-epi-LXA4 (Malvezi et al., 2014a).
The effects of other SPMs triggered by ASA have been studied. Ogata et al. (2016) evaluated the effect of AT-RvD1 on peripheral mononuclear cells (PBMCs) from patients with Chagas heart disease at stage B1, that is, with few heart abnormalities. Stimulation of PBMCs with a T. cruzi-derived antigen increased the production of INF-γ, TNFα, IL-10, and IL-13, while the pre-treatment of PBMCs with AT-RvD1 (100 nM) significantly reduced the production of INF-γ, with no changes in TNFα, IL-10, and IL-13. As INF-γ polarizes the immune response to a type Th1 response, and a low level of IL-10 indicates loss of the Treg response, both phenomena could be associated with the development of heart damage in CD. Therefore, decreasing INF-γ using AT-RvD1 has a beneficial role in chagasic cardiomyopathy. Furthermore, AT-RvD1 was able to reduce the percentage of necrotic PBMCs and their proliferation after stimulation with a T. cruzi antigen (Ogata et al., 2016).
Recently, an elevated plasma level of RvD1 has been found in T. cruzi-infected CD-1 mice. Also, RvD1, RvD5, and RvE2 (but not LXs, maresins, or PDs) were found in the lysates of T. cruzi trypomastigotes. Interestingly, there were no Rvs found in the lysates of T. cruzi epimastigotes or other protozoan parasites such as T. gondii, suggesting that the trypomastigotes themselves synthesize these SPMs (Colas et al., 2018). However, there was no direct evidence for a metabolic switch involving TcOYE or a yet unknown enzymatic activity that could explain a T. cruzi origin of these SPMs. There is currently no evidence of a T. cruzi 5-LO enzyme or another enzyme for SPM synthesis.
Statins and SPMs in Chronic Chagas Disease
15-epi-LXA4 can also be produced by statins. Birnbaum et al. (2006) showed that statins stimulate 15-epi-LXA4 release from myocardial tissue. This effect of statins over COX-2 could be mediated by the overexpression of inducible NO• synthase (iNOS), which in turns nitrosilates COX-2 at the Cys526 residue, giving COX-2 the ability to generate the 15R-HETE intermediate metabolite, which is cleaved by 5-LO to generate 15-epi-LXA4 (Kim et al., 2005). This 15-epi-LXA4 production also requires that 5-LO remains in the cytoplasm. Thus, 5-LO, phosphorylated by protein kinase A (PKA) is attached to the nuclear membrane, where it is committed to leukotriene synthesis (Ye et al., 2008).
In T. cruzi-infected human endothelial cells, simvastatin induces the synthesis of 15-epi-LXA4 and decreases the ECAM expression induced by the parasite. This effect was reversed by the addition of AA-861 (a 5-LO inhibitor) and replicated when using exogenous 15-epi-LXA4. Interestingly, 15-epi-LXA4 inhibited NF-κB pathway activation, decreasing the phosphorylation of the NF-kappa-B inhibitor (IκB) and the IκB kinase (IKK), and preventing NF-κB p65 nuclear translocation. Thus, the action of 15-epi-LXA4 in T. cruzi-infected endothelial cells involves the NF-κB signaling pathway (Campos-Estrada et al., 2015). In addition, in a murine model of CCC, 40 mg/kg/day simvastatin decreased endothelial activation, inflammatory infiltration, and fibrosis in heart tissue, an effect that persisted for a long time after treatment stopped. When simvastatin was administered to 5-LO-deficient mice, the anti-inflammatory effect was not observed unless exogenous 15-epi-LXA4 was also administered. Thus, there is an association between simvastatin administration, 15-epi-LXA4 production, and cardiac improvement. Furthermore, 15-epi-LXA4 was still detectable 30 days post administration, suggesting that 15-epi-LXA4 is stable in serum and is associated with the observed sustained effects (Gonzalez-Herrera et al., 2017).
Table 1 summarizes the experimental evidence showing the effect of pro-resolving mediators in T. cruzi infections in vivo. The findings support the idea that ASA and simvastatin have a positive impact on the resolution pathways, producing novel pro-resolving lipids such as 15-epi-LXA4 or AT-RvD1, modulating the inflammatory response, and decreasing ECAMs, leukocyte recruitment, and inflammatory infiltration. Also, the SPMs increase the survival rate in animal models of CD.
Leishmania spp. INFECTIONS
Leishmania spp. infections have become a paradigm to explain how the balance between Th1 and Th2 immune responses can effectively fight an intracellular parasite. In these infections, the Th1 response (mediated by TNF-α, IL-2, IL-12, and IFN-γ) exerts a protective role, while the Th2 response (mediated by IL-4, IL-5, and IL-10) is known as a disease promoter (reviewed in Maspi et al., 2016). The clinical manifestations of leishmaniasis are divided into three forms: cutaneous, mucocutaneous, and visceral leishmaniasis (VL).
Cutaneous Leishmaniasis
Cutaneous leishmaniasis (CL) is the most common form of leishmaniasis around the world. CL is characterized by self-limiting skin lesions located in body areas where sandflies usually bite, such as the face, neck, and limbs. The disease progression varies between different world regions. The old-world CL (caused by Leishmania major or Leishmania tropica) progresses over weeks to months to form a dry ulcer, but healing occurs over several months or years, leaving a scar or depigmentation of the skin. On the other hand, the new-world CL (caused by Leishmania mexicana, Leishmania amazonensis, or several parasites from the Vianna subspecies, such as L. [V] braziliensis) produces a wet ulcer that is associated with lymphadenitis or lymphadenopathy and may involve mucosal manifestations. New-world leishmaniasis is also called tegumentary leishmaniasis (TL), which can be localized or disseminated (de Vries et al., 2015).
In patients with TL, there is a different pattern of expression of the genes involved in the AA cascade between patients with localized CL (LCL) and mucocutaneous leishmaniasis (MCL). Patients with MCL (the most inflammatory form, with a lower parasite burden per lesion) have decreased expression of the genes for PGE2 synthesis enzymes (PTGS1 and PGES), whereas the expression of the gene for 5-LO (ALOX5) is increased. This divergent expression pattern correlates with the decreased levels of PGE2 and increased levels of LTB4 found in patients with MCL. Also, in this study, patients with MCL have increased expression of PTGER3, the gene coding for the PG receptor EP3 (Franca-Costa et al., 2016). This finding suggests a role for LTB4 in MCL-induced inflammation. However, studies on the role of the inflammatory AA derivatives (such as PGE2 or LTB4) at the molecular level show that this effect is highly Leishmania species-dependent (Figure 2).
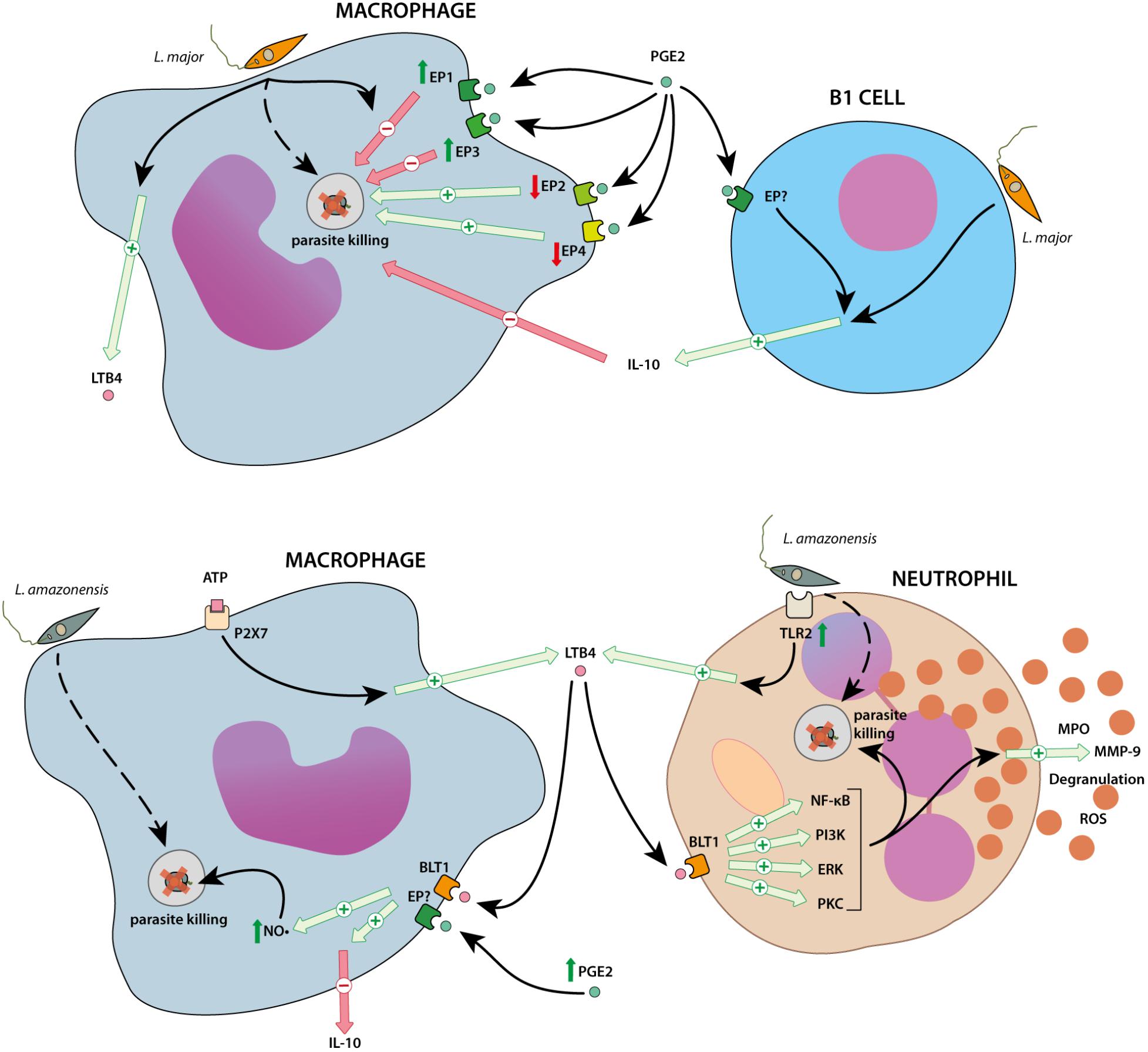
FIGURE 2. Overview of the regulation of inflammatory arachidonic acid (AA) derivatives after Leishmania major or Leishmania amazonensis infections. Cutaneous leishmaniasis causative parasites (L. major and L. amazonensis) induce the production of AA derivatives with opposite roles, depending on the Leishmania species. In L. major infection models (upper panel) the parasite induces the production of PGE2 and LTB4. Also, the expression of the EP1 and EP3 receptor is increased. The activation of these receptors by PGE2 leads to parasite survival. PGE2 also increases the release of IL-10 by the B1 cells, reducing the phagocytic activity of macrophages. On the other hand, L. amazonensis (lower panel) phagocytosis and killing by macrophages is increased by LTB4 and PGE2, and this effect is mediated by the NO• increase. Also, L. amazonensis increase the degranulation of neutrophils, increasing the anti parasitic activity of the immune system.
In the 1980s, it was reported that higher levels of PGE2 relate to reduced lymphocyte proliferation in the spleens of mice infected with L. major (Farrell and Kirkpatrick, 1987). Moreover, the increase in PGE2 and LTB4 levels induced a reduction in the Th1 cytokines TNF-α and IFN-γ, and an increase in the Th2 cytokine IL-4. This effect has been linked to the overexpression of the PG receptors EP1 and EP3 (Milano et al., 1996). Indeed, in macrophages from Balb/c mice infected with L. major, there is an increase in these receptors and downregulation of EP2 and EP4 receptors. Furthermore, agonists of EP1 and EP3 favor infection, whereas selective agonists of EP2 and EP4 decrease infection (Penke et al., 2013). PGE2 also affects the role of B-1 cells. B-1 cells modulate the phagocytic activity of macrophages; this action is dependent on IL-10 release, which in turn modulates the PGE2 levels in the media (Arcanjo et al., 2017). Also, PGE2 (via IL-10 production) increases the phagocytic activity of Balb/c mice-derived B-1CDP cells (a particular B-1 cell type with phagocytic ability; Borrello and Phipps, 1999), but it impairs the ability of the mice to resist the infection (Arcanjo et al., 2015). Finally, in a model involving human PMNs co-stimulated with ionomycin or LPS/N-formyl-methionyl-leucyl-phenylalanine (LPS/fMLP), L. major was also able to increase LTB4 release, which in turn induced a rapid and sustained decrease in LXA4 production (Plagge and Laskay, 2017).
In contrast, in mouse models of L. amazonensis infection, higher levels of LTB4 and PGE2 enhance the parasite-killing ability of murine macrophages, an effect that is dependent of NO• production and IL-10 reduction (Guimaraes et al., 2006; Serezani et al., 2006). The high levels of LTB4 from macrophages can also be induced by ATP, via P2X7 receptor activation (Chaves et al., 2014). LTB4 also has a role in neutrophil-dependent L. amazonensis killing. LTB4 activates its BLT1 receptor, inducing neutrophil degranulation, the release of metalloproteinase 9 (MMP-9) and myeloperoxidase (MPO), ROS production, and overexpression of TLR2. All these phenomena may be associated with the activation of NF-κB, PI3K, ERK, and protein kinase C (PKC) signaling (Tavares et al., 2014).
Table 2 resumes all current available evidence regarding the role of pro-resolving lipids in Leishmania spp. infections. Of note, most of the researches have been focused in CL. Regarding the role of these pro-resolving lipids in CL, RvD1 increases in patients with diffuse CL (DCL), in comparison with those with LCL, who had lower levels of RvD1 and higher levels of RvD2. This RvD1 pattern in DCL patients correlates with higher levels of Arginase-I and TGF-β and lower levels of TNF-α (Malta-Santos et al., 2017). In an in vitro model of Leishmania infection based on human cells, RvD1 increased phagocytosis of the Leishmania parasites by human macrophages. The increase of RvD1 in L. amazonensis-infected macrophages is reversed by baicalein, an inhibitor of 15-LO (Malta-Santos et al., 2017).
RvD1 and LXA4 also target AhR, a transcription factor activated by several tryptophan metabolites and some AA derivatives. AhR has been identified in Treg cells, Th17 cells, and DCs, and its activation induces the expression of IL-10 and TGF-β (Gutierrez-Vazquez and Quintana, 2018). In L. major infections, the deletion of this receptor accelerates the disease progression, increasing TNF-α production, and decreasing IL-12 and LXA4 synthesis (Elizondo et al., 2011).
Annexin A1 is a 37-kDa protein that is expressed under the control of glucocorticoids and activates FPR2/ALXR, increasing IL-10 and IL-6 in experimental models of inflammation (reviewed by Perretti and D’Acquisto, 2009). In mice infected with Leishmania [V] braziliensis, Annexin A1 expression is correlated with the lesion size, being higher 2 weeks after infection. Annexin A1 is important to control the inflammatory response while not impairing the immune system’s parasite-killing ability. Indeed, Annexin A1-KO mice display more phosphorylation of NF-κB after L. braziliesis infection. Also, Annexin A1 is increased in patients with the mucosal form of the disease, but not in those with the localized cutaneous form (Oliveira et al., 2017). In addition, the synthesis of LXA4, the primary agonist of FPR2/ALXR, is decreased in PMNs infected with L. major (Plagge and Laskay, 2017). LXA4, via FPR2/ALXR activation, enhances the phagocytic activity of PMNs in a dose- and time-dependent manner (Wenzel and Van Zandbergen, 2009).
Visceral Leishmaniasis
More than 30 years ago, it was reported that Leishmania donovani induces the synthesis of PGE2 and LTB4 in infected macrophages (Reiner and Malemud, 1984, 1985). The interaction of L. donovani with the TLR2 receptor in macrophages induces activity in the PI3K/Ca+2 axis, activating the cytosolic phospholipase A2 enzyme (cPLA2) enzyme, which release AA from membranes. Also, the Ca+2 increase activates the nuclear factor of activated T-cells (NFAT2), a transcription factor which translocates into the nucleus and induces the expression of COX-2. Both AA release and COX-2 overexpression are responsible for the resultant increased PGE2 release (Bhattacharjee et al., 2016). PGE2 is anti-inflammatory, leading to parasite survival via EP2 receptor activation. This EP2 activation triggers cAMP synthesis, with subsequent PKA activation. This second messenger cascade allows the release of IL-10 and TGF-β and reduces the levels of the inflammatory cytokines TNF-α, IL-12, and IL-17 (Figure 3). Consequently, the inhibition of COX-2 or EP2-mediated activation of PKA enhances the antiparasitic ability of immune cells in vitro and in vivo (Saha et al., 2014).
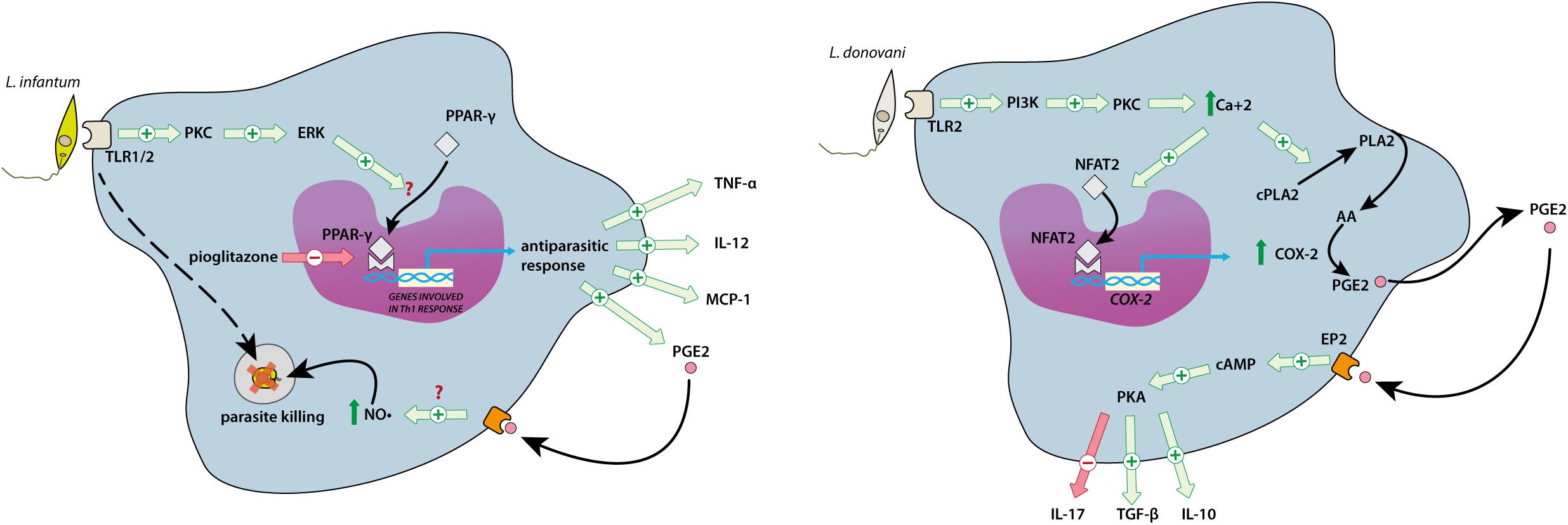
FIGURE 3. Overview of the regulation of inflammatory arachidonic acid (AA) derivatives after Leishmania infantum or Leishmania donovani infections. Visceral leishmaniasis causative parasites (L. infantum and L. donovani) induce the production of AA derivatives with opposite roles, depending of the Leishmania species. L. infantum infection (right panel) induce the release of TNF-α, IL-12, MCP-1 and PGE2 from macrophages. PGE2, in turn, induce the production of NO•, enhancing parasite killing. In contrast, L. donovani (left panel) increase the production of PGE2 by magrophages, through the activation of the PI3K pathway and the NFAT2 transcription factor. This augmented PGE2 activates the EP2 receptor in macrophages, inducing the release of regulatory cytokines such as TGF-β and IL-10, thus impairing the immune response against the parasite.
15-D-PGJ2, a pro-resolving lipid that acts as an activator of PPAR-γ receptors (Croasdell et al., 2015), decreases the parasite load in vitro and in vivo (Table 2). This antiparasitic activity correlates with a significant decrease in IL-10 and TGF-β, and a slight increase in the proinflammatory cytokines TNF-α and IL-12, suggesting that 15-D-PGJ2 favors the Th1 response against L. donovani (Vishwakarma et al., 2016).
Nevertheless, as in CL, the effect of AA derivatives is also Leishmania strain-dependent (Figure 3). Leishmania infantum is a parasite that infects a broad variety of dog populations, inducing canine VL (CVL). This parasite can also infect humans, thus dogs are a reservoir of the disease in large endemic areas of South America (Romero and Boelaert, 2010). Dogs infected with L. infantum show lower levels of PGE2 and LTB4 compared with uninfected animals. Moreover, lower levels of PGE2 and LTB4 correlate with an increase in the severity of CVL presentation (Solca et al., 2016).
At the cellular and molecular level, PGE2 increases parasite killing in macrophages infected with L. infantum; this effect is dependent on NO• release (Brandonisio et al., 2001; Panaro et al., 2001). Lymph node-derived leukocytes from dogs infected with L. infantum produce higher levels of NO•, TNF-α, and PGE2. Consequently, the pharmacological inhibition of PGE2 synthesis using indomethacin reduces TNF-α release from these cells (Venturin et al., 2016). This PGE2 release is stimulated by the activation of TLR1/2 receptors by the lipophosphoglycans of L. infantum, which induces the PKC-ERK1/2 pathway, causing the release of the Th1 cytokines TNF-α, IL-12, and monocyte chemoattractant protein-1 (MCP-1). In a dog model, PPAR-γ agonists, such as rosiglitazone, inhibit the cytokine storm induction. The fact that Th1 cytokine overexpression is associated with the inhibition of PPAR-γ (Lima et al., 2017) concurs with the fact that NSAIDs not only inhibit COX activity but can also activate this transcription factor (Lehmann et al., 1997), adding another possible mechanism of action for the antiparasitic activity of NSAIDs in in vivo L. infantum infections (Figure 3) (Panaro et al., 2001; Venturin et al., 2016). Conversely, in an L. infantum-resistant mouse model, the animals became sensitive when the activity of 5-LO was prevented. Spleen cells obtained from these mice showed that the IL-17-producing CD4+ T cells were significantly impaired, with a consequent reduction of cytokine release related to the Th17 axis (Sacramento et al., 2014).
In humans presenting with VL, the pattern of cytokines and lipids that drives the inflammatory response is diverse. In a cohort of patients from an endemic area in Brazil, patients with VL had elevated levels of IL-10, IL-6, IL-8, IL-12, RvD1, LTB4, and PGF2α. Also, these patients had lower levels of TGF-β1 and TNF-α. Moreover, this pattern of immune modulators reverted after treatment with antimonial compounds, increasing the levels of TGF-β1 and decreasing the levels of LTB4, RvD1, IL-6, IL-8, and IL-10. This study also showed that TNF-α levels were not modified by chemotherapy treatment, indicating that the inflammatory response to kill the parasite remained unaltered (Araujo-Santos et al., 2017).
Trypanosoma brucei INFECTIONS
Trypanosoma brucei subsp. are responsible for human African trypanosomiasis (HAT), also known as sleeping sickness. Currently, HAT is the most neglected disease among the so-called Tri-Tryp (T. brucei, T. cruzi, and Leishmania spp.) and the volume of research on the inflammatory aspects of the disease are below those for other trypanosomatids. HAT progresses from a hemolymphatic early stage, which is characterized by the presence of parasites in the bloodstream, to a meningoencephalitic or late stage, where the parasite crosses the blood–brain barrier and causes an inflammatory encephalitic reaction that ultimately causes the death of the human host. The early stage (1–3 weeks) begins when the tsetse fly bites its host, depositing parasites held within its saliva on the human skin. Later, the parasites spread to various peripheral organs and tissues via the lymph and blood, inducing symptoms that include general malaise, anemia, weakness, and weight loss. The late stage coincides with the parasite invasion of the central nervous system (CNS), and it is associated with neurological alterations such as sleep disorders, confusion, and mental discoordination. Neuropsychiatric symptoms increase in frequency and severity with disease progression and untreated patients progress to a final stage involving seizures, drowsiness, coma, and death (Sternberg and MacLean, 2010; Büscher et al., 2017).
Although symptoms are common for both T. brucei rhodesiense- and T. brucei gambiense-associated HAT, the clinical presentation depends on which of the two subtypes of T. brucei is involved in the infection. T. brucei gambiense is associated with a slow-progressing form of HAT, whereas T. brucei rhodesiense is related to a faster-progressing form that can cause CNS damage within a few weeks of infection (Büscher et al., 2017). The interplay between the host immune response and parasite subspecies virulence patterns determines the progression and severity of the disease for each particular patient (Ponte-Sucre, 2016).
The initial response against the parasite mainly involves a Th1 pro-inflammatory cytokine profile including TNF-α, IL-6, NO•, IL-1, and IL-12 (Schleifer and Mansfield, 1993). At the same time, T. brucei gambiense activates a Th-dependent B-cell response against the main antigenic molecule of T. brucei, variant surface glycoprotein (VSG), allowing clearance of the organisms from the blood (Mansfield and Paulnock, 2005). As reviewed by Ponte-Sucre (2016), the presence of VSG allows the immune system to exert a lytic antibody response against T. brucei, but the parasite’s ability to switch to new VSG coats generates a parasite population that are not recognized by the previously generated antibodies. Although VSG is considered the primary antigenic molecule of T. brucei, parasite DNA is released into the plasma of infected mice, acting as a pathogen-associated molecular pattern (PAMP). This DNA activates macrophages in the first days post infection, increasing IL-12 levels, probably to induce a response to control the parasite levels by enhancing Th1 cell polarization (Sternberg et al., 2005). On the other hand, T. brucei rhodesiense DNA also increases IL-10 levels, which could play a role in controlling the immune response, as this cytokine has been shown to limit immunopathology (Sternberg et al., 2005; Harris et al., 2006).
Common to other trypanosome species, the success of T. brucei infection relies on its ability to overcome the initial immune system response but to an extent that is compatible with the life of the host, avoiding a devastating “hyper-infection” (Ponte-Sucre, 2016). In this respect, resolution of the inflammatory response is one of the main events that T. brucei modulates to escape the initial immune system attack. An early study showed that suppressor macrophages obtained from mice infected with T. brucei were able to inhibit production of IL-2 and the expression of the IL-2 receptor, decreasing T-cell activation but not pro-inflammatory secretion of IL-1, which could be produced by an increase in PGs (Sileghem et al., 1989). These effects are concordant with following studies reporting that suppressor macrophages control T-cell activation in T. brucei infection through NO• synthase (NOS) up-regulation and that elevated NO• produced by macrophages derived from infected mice is also dependent on PG synthesis (Schleifer and Mansfield, 1993). At that time, it was clear that PGs had a role in mediating the initial avoidance of the immune system as, in several cell types, T. brucei elicited an increase in these eicosanoids; the discovery of a parasite PGF2α synthase further reinforced this idea (Alafiatayo et al., 1994; Kubata et al., 2000). In fact, administration of the classic COX inhibitor sodium salicylate (an ASA metabolite) to chronically T. brucei-infected Sprague-Dawley rats induced a marked increase in neurotoxicity, with an increase in mRNA levels of pro-inflammatory cytokines such as IL-1β, IL-6, and IFN-γ, and an increase in COX-2 and iNOS enzymes (Quan et al., 2000). This evidence suggests that, to some extent, PGs play a role not only in the acute phase of the infection but also in the late stage, probably controlling the parasite burden. In bloodstream forms of T. brucei rhodesiense, PGD2 and its metabolites can inhibit parasite growth and induce apoptotic-like programmed cell death through ROS generation (Figarella et al., 2005, 2006). Salmon et al. (2012) reported that T. brucei adenylate cyclases (ACs) play a role in reducing the early innate defense against live parasites by inhibiting TNF-α synthesis in infected mice. The authors demonstrated that cAMP-mediated activation of PKA affected trypanosome infection, an effect mediated by PKA signaling activation (Salmon et al., 2012). It is well known that PGs can activate cAMP-mediated responses in different cell types; hence, it is possible that these inflammatory mediators could act through these signaling pathways to control parasite survival, although there is no direct evidence for this postulation, at least regarding T. brucei.
Another relevant aspect is the control of inflammation and damage to different organs elicited by T. brucei in the late stage of the disease. In this respect, the regulatory cytokine IL-10 is induced by the parasite, decreasing the levels of NO• and TNF-α in infected mice, reducing organ damage, and favoring host survival (Guilliams et al., 2009). Also, results from several animal studies indicate that T. brucei-induced IL-10 production counters anemia; thus, this cytokine may play a crucial role in parasite and host survival (Musaya et al., 2015), which concurs with findings from research on human subjects. In the late stage, the levels of IL-10 were found to be elevated irrespective of the geographical location of the patients and the particular genotype of the strain involved in the infection (Maclean et al., 2004). In a more recent study, Kato et al. (2015) evaluated plasma and cerebrospinal fluid (CSF) cytokine levels in patients with early- or late-stage HAT. Although the authors did not find a difference in the levels of IFN-γ, TGF-β, IL-6, and IL-10 in the plasma, the CSF samples showed an up-regulation of IL-6 and IL-10 in the late-stage patients, which was associated with a reduction in severity of neurological impairment (Kato et al., 2015). In contrast, “trypanotolerant” individuals with elevated IL-10 levels and low levels of TNF-α are associated with a higher risk of developing HAT (Ilboudo et al., 2014). This evidence indicates that T. brucei can regulate both the inflammatory response in both the early and late stage of the infection, to ensure host survival. Although there is increasing evidence of inflammatory mediators such as cytokines and PGs, the “fine-tuning” of inflammation and parasite survival comprises a series of molecules and processes rather than a single mechanism.
Concluding Remarks
Trypanosomatids in general and T. cruzi and Leishmania spp. in particular are responsible for chronic disabling and potentially fatal diseases. The understanding of the pathophysiological processes in which the parasite develops is fundamental for the design of much more effective therapies. In this regard, it is very promising to contemplate modifying aspects of the inflammation induced by the parasites in order to resolve or diminish the inflammation, promoting a more efficient clearance of the microorganism by the host’s immune system. There is growing evidence addressing this point. Therefore, the use of pro-resolving lipids, mainly ASA-triggered LXs and Rvs, together with antiparasitic therapy itself, could help to prevent the damage induced by the chronic inflammation generated by this group of parasites.
In this context, SPMs such as LXA4, RvD1, and drug-induced pro-resolving lipids (such as 13-epi-LXA4 and AT-RvD1) have been proven to be effective in the control of the inflammatory response against parasite infection in most animal models. However, the fact that the parasite itself could induce the release of these agents (or eventually synthesize them) indicates that the control of acute inflammation could also be beneficial for the parasite. Thus, the use of SPMs as a strategy against trypanosomatid infections should not be a universal consideration, as the effects of SMPs are highly variable between different parasite species/strains and even different mammals. Overall, there is a need for more research to elucidate which parasites, host conditions, or even infection stages are associated with safe and effective use (or synthesis stimulation) of these SPMs.
Author Contributions
RL-M, AM-B, CC-E, PA-S, LU-L, MP-E, and JM performed the literature search, analyzed the articles, and wrote the manuscript. RL-M and JM made the figures. All authors approved the manuscript in its final version.
Funding
RL-M is funded by the Fondecyt Regular grant #1160807 (Comisión Nacional de Investigación Científica y Tecnológica, Chile). JM is funded by the Fondecyt Regular grant #1170126 (Comisión Nacional de Investigación Científica y Tecnológica, Chile), U-REDES grant URC-024/16 (Vicerrectoría de Investigación y Desarrollo, Universidad de Chile, Chile), and CONICYT/REDES grant #170126 (Comisión Nacional de Investigación Científica y Tecnológica, Chile). CC-E is funded by the PAI grant #79150077 (Comisión Nacional de Investigación Científica y Tecnológica, Chile). MP-E is funded by the FONDECYT Postdoctoral grant #3170875 (Comisión Nacional de Investigación Científica y Tecnológica, Chile). The funders had no role in the literature analyses, decision to publish, or preparation of the manuscript.
Conflict of Interest Statement
The authors declare that the research was conducted in the absence of any commercial or financial relationships that could be construed as a potential conflict of interest.
References
Abdalla, G. K., Faria, G. E., Silva, K. T., Castro, E. C., Reis, M. A., and Michelin, M. A. (2008). Trypanosoma cruzi: the role of PGE2 in immune response during the acute phase of experimental infection. Exp. Parasitol. 118, 514–521. doi: 10.1016/j.exppara.2007.11.003
Abdulnour, R. E., Sham, H. P., Douda, D. N., Colas, R. A., Dalli, J., Bai, Y., et al. (2016). Aspirin-triggered resolvin D1 is produced during self-resolving gram-negative bacterial pneumonia and regulates host immune responses for the resolution of lung inflammation. Mucosal Immunol. 9, 1278–1287. doi: 10.1038/mi.2015.129
Alafiatayo, R. A., Cookson, M. R., and Pentreath, V. W. (1994). Production of prostaglandins D2 and E2 by mouse fibroblasts and astrocytes in culture caused by Trypanosoma brucei brucei products and endotoxin. Parasitol. Res. 80, 223–229. doi: 10.1007/BF00932678
Alessandri, A. L., Sousa, L. P., Lucas, C. D., Rossi, A. G., Pinho, V., and Teixeira, M. M. (2013). Resolution of inflammation: mechanisms and opportunity for drug development. Pharmacol. Ther. 139, 189–212. doi: 10.1016/j.pharmthera.2013.04.006
Aliberti, J., Serhan, C., and Sher, A. (2002). Parasite-induced lipoxin A4 is an endogenous regulator of IL-12 production and immunopathology in Toxoplasma gondii infection. J. Exp. Med. 196, 1253–1262. doi: 10.1084/jem.20021183
Araujo-Santos, T., Andrade, B. B., Gil-Santana, L., Luz, N. F., Dos Santos, P. L., de Oliveira, F. A., et al. (2017). Anti-parasite therapy drives changes in human visceral leishmaniasis-associated inflammatory balance. Sci. Rep. 7:4334. doi: 10.1038/s41598-017-04595-8
Arcanjo, A. F., LaRocque-de-Freitas, I. F., Rocha, J. D., Zamith, D., Costa-da-Silva, A. C., Nunes, M. P., et al. (2015). The PGE2/IL-10 axis determines susceptibility of B-1 cell-derived phagocytes (B-1CDP) to Leishmania major Infection. PLoS One 10:e0124888. doi: 10.1371/journal.pone.0124888
Arcanjo, A. F., Nunes, M. P., Silva-Junior, E. B., Leandro, M., da Rocha, J. D. B., Morrot, A., et al. (2017). B-1 cells modulate the murine macrophage response to Leishmania major infection. World J. Biol. Chem. 8, 151–162. doi: 10.4331/wjbc.v8.i2.151
Ariel, A., Li, P. L., Wang, W., Tang, W. X., Fredman, G., Hong, S., et al. (2005). The docosatriene protectin D1 is produced by TH2 skewing and promotes human T cell apoptosis via lipid raft clustering. J. Biol. Chem. 280, 43079–43086. doi: 10.1074/jbc.M509796200
Arita, M., Ohira, T., Sun, Y. P., Elangovan, S., Chiang, N., and Serhan, C. N. (2007). Resolvin E1 selectively interacts with leukotriene B4 receptor BLT1 and ChemR23 to regulate inflammation. J. Immunol. 178, 3912–3917. doi: 10.4049/jimmunol.178.6.3912
Ashton, A. W., Mukherjee, S., Nagajyothi, F. N., Huang, H., Braunstein, V. L., Desruisseaux, M. S., et al. (2007). Thromboxane A2 is a key regulator of pathogenesis during Trypanosoma cruzi infection. J. Exp. Med. 204, 929–940. doi: 10.1084/jem.20062432
Bafica, A., Scanga, C. A., Serhan, C., Machado, F., White, S., Sher, A., et al. (2005). Host control of Mycobacterium tuberculosis is regulated by 5-lipoxygenase-dependent lipoxin production. J. Clin. Invest. 115, 1601–1606. doi: 10.1172/JCI23949
Bhattacharjee, A., Majumder, S., Das, S., Ghosh, S., Biswas, S., and Majumdar, S. (2016). Leishmania donovani-induced prostaglandin E2 generation is critically dependent on host toll-like receptor 2-cytosolic phospholipase A2 signaling. Infect. Immun. 84, 2963–2973. doi: 10.1128/IAI.00528-16
Birnbaum, Y., Ye, Y., Lin, Y., Freeberg, S. Y., Nishi, S. P., Martinez, J. D., et al. (2006). Augmentation of myocardial production of 15-epi-lipoxin-a4 by pioglitazone and atorvastatin in the rat. Circulation 114, 929–935. doi: 10.1161/CIRCULATIONAHA.106.629907
Borges, M. M., Kloetzel, J. K., Andrade, HF Jr, Tadokoro, C. E., Pinge-Filho, P., and Abrahamsohn, I. (1998). Prostaglandin and nitric oxide regulate TNF-alpha production during Trypanosoma cruzi infection. Immunol. Lett. 63, 1–8. doi: 10.1016/S0165-2478(98)00034-0
Borrello, M. A., and Phipps, R. P. (1999). Fibroblast-secreted macrophage colony-stimulating factor is responsible for generation of biphenotypic B/macrophage cells from a subset of mouse B lymphocytes. J. Immunol. 163, 3605–3611.
Brandonisio, O., Panaro, M. A., Sisto, M., Acquafredda, A., Fumarola, L., Leogrande, D., et al. (2001). Nitric oxide production by Leishmania-infected macrophages and modulation by cytokines and prostaglandins. Parassitologia 43(Suppl. 1), 1–6.
Büscher, P., Cecchi, G., Jamonneau, V., and Priotto, G. (2017). Human african trypanosomiasis. Lancet 390, 2397–2409. doi: 10.1016/S0140-6736(17)31510-6
Campbell, E. L., Louis, N. A., Tomassetti, S. E., Canny, G. O., Arita, M., Serhan, C. N., et al. (2007). Resolvin E1 promotes mucosal surface clearance of neutrophils: a new paradigm for inflammatory resolution. FASEB J. 21,3162–3170. doi: 10.1096/fj.07-8473com
Campos-Estrada, C., Liempi, A., Gonzalez-Herrera, F., Lapier, M., Kemmerling, U., Pesce, B., et al. (2015). Simvastatin and benznidazole-mediated prevention of Trypanosoma cruzi-induced endothelial activation: role of 15-epi-lipoxin A4 in the action of simvastatin. PLoS Negl. Trop. Dis. 9:e0003770. doi: 10.1371/journal.pntd.0003770
Canny, G., Levy, O., Furuta, G. T., Narravula-Alipati, S., Sisson, R. B., Serhan, C. N., et al. (2002). Lipid mediator-induced expression of bactericidal/permeability-increasing protein (BPI) in human mucosal epithelia. Proc. Natl. Acad. Sci. U.S.A. 99, 3902–3907. doi: 10.1073/pnas.052533799
Cardoni, R. L., and Antunez, M. I. (2004). Circulating levels of cyclooxygenase metabolites in experimental Trypanosoma cruzi infections. Mediat. Inflamm. 13, 235–240. doi: 10.1080/09637480400003022
Carvalho de Freitas, R., Lonien, S. C. H., Malvezi, A. D., Silveira, G. F., Wowk, P. F., et al. (2017). Trypanosoma cruzi: inhibition of infection of human monocytes by aspirin. Exp. Parasitol. 182, 26–33. doi: 10.1016/j.exppara.2017.09.019
Celentano, A. M., Gorelik, G., Solana, M. E., Sterin-Borda, L., Borda, E., and Gonzalez Cappa, S. M. (1995). PGE2 involvement in experimental infection with Trypanosoma cruzi subpopulations. Prostaglandins 49, 141–153. doi: 10.1016/0090-6980(95)00002-R
Chandrasekharan, J. A., and Sharma-Walia, N. (2015). Lipoxins: nature’s way to resolve inflammation. J. Inflamm. Res. 8, 181–192. doi: 10.2147/JIR.S90380
Chaves, M. M., Marques-da-Silva, C., Monteiro, A. P., Canetti, C., and Coutinho-Silva, R. (2014). Leukotriene B4 modulates P2X7 receptor-mediated Leishmania amazonensis elimination in murine macrophages. J. Immunol. 192, 4765–4773. doi: 10.4049/jimmunol.1301058
Chiang, N., Bermudez, E. A., Ridker, P. M., Hurwitz, S., and Serhan, C. N. (2004). Aspirin triggers antiinflammatory 15-epi-lipoxin A4 and inhibits thromboxane in a randomized human trial. Proc. Natl. Acad. Sci. U.S.A. 101, 15178–15183. doi: 10.1073/pnas.0405445101
Chiang, N., Fredman, G., Backhed, F., Oh, S. F., Vickery, T., Schmidt, B. A., et al. (2012). Infection regulates pro-resolving mediators that lower antibiotic requirements. Nature 484, 524–528. doi: 10.1038/nature11042
Cilloniz, C., Pantin-Jackwood, M. J., Ni, C., Goodman, A. G., Peng, X., Proll, S. C., et al. (2010). Lethal dissemination of H5N1 influenza virus is associated with dysregulation of inflammation and lipoxin signaling in a mouse model of infection. J. Virol. 84, 7613–7624. doi: 10.1128/JVI.00553-10
Colas, R. A., Ashton, A. W., Mukherjee, S., Dalli, J., Akide-Ndunge, O. B., Huang, H., et al. (2018). Trypanosoma cruzi produces the specialized proresolving mediators Resolvin D 1, Resolvin D5 and Resolvin E2. Infect. Immun. 86, e688–e617. doi: 10.1128/IAI.00688-17
Cooray, S. N., Gobbetti, T., Montero-Melendez, T., McArthur, S., Thompson, D., Clark, A. J., et al. (2013). Ligand-specific conformational change of the G-protein-coupled receptor ALX/FPR2 determines proresolving functional responses. Proc. Natl. Acad. Sci. U.S.A. 110, 18232–18237. doi: 10.1073/pnas.1308253110
Cossentini, L. A., Da Silva, R. V., Yamada-Ogatta, S. F., Yamauchi, L. M., De Almeida Araujo, E. J., and Pinge-Filho, P. (2016). Aspirin treatment exacerbates oral infections by Trypanosoma cruzi. Exp. Parasitol. 164, 64–70. doi: 10.1016/j.exppara.2016.01.008
Croasdell, A., Duffney, P. F., Kim, N., Lacy, S. H., Sime, P. J., and Phipps, R. P. (2015). PPARgamma and the innate immune system mediate the resolution of inflammation. PPAR Res. 2015:549691. doi: 10.1155/2015/549691
Dalli, J., Chiang, N., and Serhan, C. N. (2015). Elucidation of novel 13-series resolvins that increase with atorvastatin and clear infections. Nat. Med. 21, 1071–1075. doi: 10.1038/nm.3911
D’Avila, H., Freire-de-Lima, C. G., Roque, N. R., Teixeira, L., Barja-Fidalgo, C., Silva, A. R., et al. (2011). Host cell lipid bodies triggered by Trypanosoma cruzi infection and enhanced by the uptake of apoptotic cells are associated with prostaglandin E(2) generation and increased parasite growth. J. Infect. Dis. 204, 951–961. doi: 10.1093/infdis/jir432
de Vries, H. J., Reedijk, S. H., and Schallig, H. D. (2015). Cutaneous leishmaniasis: recent developments in diagnosis and management. Am. J. Clin. Dermatol. 16, 99–109. doi: 10.1007/s40257-015-0114-z
Delgado, M., and Ganea, D. (2008). Anti-inflammatory neuropeptides: a new class of endogenous immunoregulatory agents. Brain Behav. Immun. 22, 1146–1151. doi: 10.1016/j.bbi.2008.06.001
El Kebir, D., Gjorstrup, P., and Filep, J. G. (2012). Resolvin E1 promotes phagocytosis-induced neutrophil apoptosis and accelerates resolution of pulmonary inflammation. Proc. Natl. Acad. Sci. U.S.A. 109, 14983–14988. doi: 10.1073/pnas.1206641109
Elizondo, G., Rodriguez-Sosa, M., Estrada-Muniz, E., Gonzalez, F. J., and Vega, L. (2011). Deletion of the aryl hydrocarbon receptor enhances the inflammatory response to Leishmania major infection. Int. J. Biol. Sci. 7, 1220–1229. doi: 10.7150/ijbs.7.1220
Farrell, J. P., and Kirkpatrick, C. E. (1987). Experimental cutaneous leishmaniasis. II. A possible role for prostaglandins in exacerbation of disease in Leishmania major-infected BALB/c mice. J. Immunol. 138, 902–907.
Figarella, K., Rawer, M., Uzcategui, N. L., Kubata, B. K., Lauber, K., Madeo, F., et al. (2005). Prostaglandin D2 induces programmed cell death in Trypanosoma brucei bloodstream form. Cell Death Differ. 12, 335–346. doi: 10.1038/sj.cdd.4401564
Figarella, K., Uzcategui, N. L., Beck, A., Schoenfeld, C., Kubata, B. K., Lang, F., et al. (2006). Prostaglandin-induced programmed cell death in Trypanosoma brucei involves oxidative stress. Cell Death Differ. 13, 1802–1814. doi: 10.1038/sj.cdd.4401862
Flitter, B. A., Hvorecny, K. L., Ono, E., Eddens, T., Yang, J., Kwak, D. H., et al. (2017). Pseudomonas aeruginosa sabotages the generation of host proresolving lipid mediators. Proc. Natl. Acad. Sci. U.S.A. 114, 136–141. doi: 10.1073/pnas.1610242114
Franca-Costa, J., Andrade, B. B., Khouri, R., Van Weyenbergh, J., Malta-Santos, H., da Silva, et al. (2016). Differential expression of the eicosanoid pathway in patients with localized or mucosal cutaneous leishmaniasis. J. Infect. Dis. 213, 1143–1147. doi: 10.1093/infdis/jiv548
Fredman, G., Ozcan, L., Spolitu, S., Hellmann, J., Spite, M., Backs, J., et al. (2014). Resolvin D1 limits 5-lipoxygenase nuclear localization and leukotriene B4 synthesis by inhibiting a calcium-activated kinase pathway. Proc. Natl. Acad. Sci. U.S.A. 111, 14530–14535. doi: 10.1073/pnas.1410851111
Freire-de-Lima, C. G., Nascimento, D. O., Soares, M. B., Bozza, P. T., Castro-Faria-Neto, H. C., de Mello, F. G., et al. (2000). Uptake of apoptotic cells drives the growth of a pathogenic trypanosome in macrophages. Nature 403, 199–203. doi: 10.1038/35003208
Frolov, A., Yang, L., Dong, H., Hammock, B. D., and Crofford, L. J. (2013). Anti-inflammatory properties of prostaglandin E2: deletion of microsomal prostaglandin E synthase-1 exacerbates non-immune inflammatory arthritis in mice. Prostaglandins Leukot Essent Fatty Acids 89, 351–358. doi: 10.1016/j.plefa.2013.08.003
Gilroy, D., and De Maeyer, R. (2015). New insights into the resolution of inflammation. Semin. Immunol. 27, 161–168. doi: 10.1016/j.smim.2015.05.003
Golias, C., Tsoutsi, E., Matziridis, A., Makridis, P., Batistatou, A., and Charalabopoulos, K. (2007). Review. Leukocyte and endothelial cell adhesion molecules in inflammation focusing on inflammatory heart disease. In Vivo 21, 757–769.
Gomes, J. A., Molica, A. M., Keesen, T. S., Morato, M. J., de Araujo, F. F., Fares, R. C., et al. (2014). Inflammatory mediators from monocytes down-regulate cellular proliferation and enhance cytokines production in patients with polar clinical forms of Chagas disease. Hum. Immunol. 75, 20–28. doi: 10.1016/j.humimm.2013.09.009
Gonzalez-Herrera, F., Cramer, A., Pimentel, P., Castillo, C., Liempi, A., Kemmerling, U., et al. (2017). Simvastatin attenuates endothelial activation through 15-Epi-lipoxin A4 production in murine chronic chagas cardiomyopathy. Antimicrob. Agents Chemother. 61:e2137-16. doi: 10.1128/AAC.02137-16
Guerrero, N. A., Camacho, M., Vila, L., Iniguez, M. A., Chillon-Marinas, C., Cuervo, H., et al. (2015). Cyclooxygenase-2 and prostaglandin E2 signaling through prostaglandin receptor EP-2 favor the development of myocarditis during acute Trypanosoma cruzi infection. PLoS Negl. Trop. Dis. 9:e0004025. doi: 10.1371/journal.pntd.0004025
Guilliams, M., Movahedi, K., Bosschaerts, T., VandenDriessche, T., Chuah, M. K., Herin, M., et al. (2009). IL-10 dampens TNF/inducible nitric oxide synthase-producing dendritic cell-mediated pathogenicity during parasitic infection. J. Immunol. 182, 1107–1118. doi: 10.4049/jimmunol.182.2.1107
Guimaraes, E. T., Santos, L. A., Ribeiro, dos Santos, R., Teixeira, M. M., dos Santos, W. L., et al. (2006). Role of interleukin-4 and prostaglandin E2 in Leishmania amazonensis infection of BALB/c mice. Microbes Infect. 8, 1219–1226. doi: 10.1016/j.micinf.2005.11.011
Gutierrez-Vazquez, C., and Quintana, F. J. (2018). Regulation of the immune response by the aryl hydrocarbon receptor. Immunity 48, 19–33. doi: 10.1016/j.immuni.2017.12.012
Harris, T. H., Cooney, N. M., Mansfield, J. M., and Paulnock, D. M. (2006). Signal transduction, gene transcription, and cytokine production triggered in macrophages by exposure to trypanosome DNA. Infect. Immun. 74, 4530–4537. doi: 10.1128/IAI.01938-05
Hideko Tatakihara, V. L., Cecchini, R., Borges, C. L., Malvezi, A. D., Graca-de Souza, V. K., Yamada-Ogatta, S. F., et al. (2008). Effects of cyclooxygenase inhibitors on parasite burden, anemia and oxidative stress in murine Trypanosoma cruzi infection. FEMS Immunol. Med. Microbiol. 52, 47–58. doi: 10.1111/j.1574-695X.2007.00340.x
Ilboudo, H., Bras-Gonçalves, R., Camara, M., Flori, L., Camara, O., Sakande, H., et al. (2014). Unravelling human trypanotolerance: IL8 is associated with infection control whereas IL10 and TNFα are associated with subsequent disease development. PLoS Pathog. 10:e1004469. doi: 10.1371/journal.ppat.1004469
Karp, C. L., Flick, L. M., Park, K. W., Softic, S., Greer, T. M., Keledjian, R., et al. (2004). Defective lipoxin-mediated anti-inflammatory activity in the cystic fibrosis airway. Nat. Immunol. 5, 388–392. doi: 10.1038/ni1056
Kato, C. D., Alibu, V. P., Nanteza, A., Mugasa, C. M., and Matovu, E. (2015). Interleukin (IL)-6 and IL-10 are up regulated in late stage Trypanosoma brucei rhodesiense sleeping sickness. PLoS Negl. Trop. Dis. 9:1–13. doi: 10.1371/journal.pntd.0003835
Kim, J. H., Song, J., and Park, K. W. (2015). The multifaceted factor peroxisome proliferator-activated receptor gamma (PPARgamma) in metabolism, immunity, and cancer. Arch. Pharm. Res. 38, 302–312. doi: 10.1007/s12272-015-0559-x
Kim, S. F., Huri, D. A., and Snyder, S. H. (2005). Inducible nitric oxide synthase binds, S-nitrosylates, and activates cyclooxygenase-2. Science 310, 1966–1970. doi: 10.1126/science.1119407
Krishnamoorthy, S., Recchiuti, A., Chiang, N., Fredman, G., and Serhan, C. N. (2012). Resolvin D1 receptor stereoselectivity and regulation of inflammation and proresolving microRNAs. Am. J. Pathol. 180, 2018–2027. doi: 10.1016/j.ajpath.2012.01.028
Kubata, B. K., Duszenko, M., Kabututu, Z., Rawer, M., Szallies, A., Fujimori, K., et al. (2000). Identification of a novel prostaglandin f(2alpha) synthase in Trypanosoma brucei. J. Exp. Med. 192, 1327–1338. doi: 10.1084/jem.192.9.1327
Kubata, B. K., Kabututu, Z., Nozaki, T., Munday, C. J., Fukuzumi, S., Ohkubo, K., et al. (2002). A key role for old yellow enzyme in the metabolism of drugs by Trypanosoma cruzi. J. Exp. Med. 196, 1241–1251. doi: 10.1084/jem.20020885
Lehmann, J. M., Lenhard, J. M., Oliver, B. B., Ringold, G. M., and Kliewer, S. A. (1997). Peroxisome proliferator-activated receptors alpha and gamma are activated by indomethacin and other non-steroidal anti-inflammatory drugs. J. Biol. Chem. 272, 3406–3410. doi: 10.1074/jbc.272.6.3406
Lima, J. B., Araujo-Santos, T., Lazaro-Souza, M., Carneiro, A. B., Ibraim, I. C., Jesus-Santos, F. H., et al. (2017). Leishmania infantum lipophosphoglycan induced-prostaglandin E2 production in association with PPAR-gamma expression via activation of toll like receptors-1 and 2. Sci. Rep. 7:14321. doi: 10.1038/s41598-017-14229-8
Lopez-Munoz, R., Faundez, M., Klein, S., Escanilla, S., Torres, G., Lee-Liu, D., et al. (2010). Trypanosoma cruzi: In vitro effect of aspirin with nifurtimox and benznidazole. Exp. Parasitol. 124, 167–171. doi: 10.1016/j.exppara.2009.09.005
Machado, F. S., Johndrow, J. E., Esper, L., Dias, A., Bafica, A., Serhan, C. N., et al. (2006). Anti-inflammatory actions of lipoxin A4 and aspirin-triggered lipoxin are SOCS-2 dependent. Nat. Med. 12, 330–334. doi: 10.1038/nm1355
Machado, F. S., Mukherjee, S., Weiss, L. M., Tanowitz, H. B., and Ashton, A. W. (2011). Bioactive lipids in Trypanosoma cruzi infection. Adv. Parasitol. 76, 1–31. doi: 10.1016/B978-0-12-385895-5.00001-3
Maclean, L., Chisi, J. E., Odiit, M., Gibson, W. C., Ferris, V., Picozzi, K., et al. (2004). Severity of human African trypanosomiasis in East Africa is associated with geographic location, parasite genotype, and host in ammatory cytokine response pro le. Infect. Immun. 72, 7040–7044. doi: 10.1128/IAI.72.12.7040
Maderna, P., Cottell, D. C., Toivonen, T., Dufton, N., Dalli, J., Perretti, M., et al. (2010). FPR2/ALX receptor expression and internalization are critical for lipoxin A4 and annexin-derived peptide-stimulated phagocytosis. FASEB J. 24, 4240–4249. doi: 10.1096/fj.10-159913
Malta-Santos, H., Andrade, B. B., Zanette, D. L., Costa, J. M., Bozza, P. T., Bandeira-Melo, C., et al. (2017). Resolvin D1 drives establishment of Leishmania amazonensis infection. Sci. Rep. 7:46363. doi: 10.1038/srep46363
Malvezi, A. D., da Silva, R. V., Panis, C., Yamauchi, L. M., Lovo-Martins, M. I., Zanluqui, N. G., et al. (2014a). Aspirin modulates innate inflammatory response and inhibits the entry of Trypanosoma cruzi in mouse peritoneal macrophages. Mediat. Inflamm. 2014:580919. doi: 10.1155/2014/580919
Malvezi, A. D., Panis, C., da Silva, R. V., de Freitas, R. C., Lovo-Martins, M. I., Tatakihara, V. L., et al. (2014b). Inhibition of cyclooxygenase-1 and cyclooxygenase-2 impairs Trypanosoma cruzi entry into cardiac cells and promotes differential modulation of the inflammatory response. Antimicrob. Agents Chemother. 58, 6157–6164. doi: 10.1128/AAC.02752-14
Mansfield, J. M., and Paulnock, D. M. (2005). Regulation of innate and acquired immunity in African trypanosomiasis. Parasite Immunol. 27, 361–371. doi: 10.1111/j.1365-3024.2005.00791.x
Mariani, F., and Roncucci, L. (2015). Chemerin/chemR23 axis in inflammation onset and resolution. Inflamm. Res. 64, 85–95. doi: 10.1007/s00011-014-0792-7
Maspi, N., Abdoli, A., and Ghaffarifar, F. (2016). Pro- and anti-inflammatory cytokines in cutaneous leishmaniasis: a review. Pathog. Glob Health 110,247–260. doi: 10.1080/20477724.2016.1232042
Melo, R. C., D’Avila, H., Fabrino, D. L., Almeida, P. E., and Bozza, P. T. (2003). Macrophage lipid body induction by Chagas disease in vivo: putative intracellular domains for eicosanoid formation during infection. Tissue Cell 35, 59–67. doi: 10.1016/S0040-8166(02)00105-2
Michelin, M. A., Silva, J. S., and Cunha, F. Q. (2005). Inducible cyclooxygenase released prostaglandin mediates immunosuppression in acute phase of experimental Trypanosoma cruzi infection. Exp. Parasitol. 111, 71–79. doi: 10.1016/j.exppara.2005.05.001
Milano, S., Arcoleo, F., Dieli, M., D’Agostino, R., De Nucci, G., D’Agostino, P., et al. (1996). Ex vivo evidence for PGE2 and LTB4 involvement in cutaneous leishmaniasis: relation with infection status and cytokine production. Parasitology 112(Pt 1), 13–19. doi: 10.1017/S0031182000065033
Miyahara, T., Runge, S., Chatterjee, A., Chen, M., Mottola, G., Fitzgerald, J. M., et al. (2013). D-series resolvin attenuates vascular smooth muscle cell activation and neointimal hyperplasia following vascular injury. FASEB J. 27, 2220–2232. doi: 10.1096/fj.12-225615
Molina-Berrios, A., Campos-Estrada, C., Henriquez, N., Faundez, M., Torres, G., Castillo, C., et al. (2013a). Protective role of acetylsalicylic acid in experimental Trypanosoma cruzi infection: evidence of a 15-epi-lipoxin A(4)-mediated effect. PLoS Negl. Trop. Dis. 7:e2173. doi: 10.1371/journal.pntd.0002173
Molina-Berrios, A., Campos-Estrada, C., Lapier, M., Duaso, J., Kemmerling, U., Galanti, N., et al. (2013b). Protection of vascular endothelium by aspirin in a murine model of chronic Chagas’ disease. Parasitol. Res. 112, 2731–2739. doi: 10.1007/s00436-013-3444-x
Morita, M., Kuba, K., Ichikawa, A., Nakayama, M., Katahira, J., Iwamoto, R., et al. (2013). The lipid mediator protectin D1 inhibits influenza virus replication and improves severe influenza. Cell 153, 112–125. doi: 10.1016/j.cell.2013.02.027
Mukherjee, S., Machado, F. S., Huang, H., Oz, H. S., Jelicks, L. A., Prado, C. M., et al. (2011). Aspirin treatment of mice infected with Trypanosoma cruzi and implications for the pathogenesis of Chagas disease. PLoS One 6:e16959. doi: 10.1371/journal.pone.0016959
Musaya, J., Matovu, E., Nyirenda, M., and Chisi, J. (2015). Role of cytokines in Trypanosoma brucei -induced anaemia: a review of the literature. Malawi Med. J. 27, 45–50. doi: 10.4314/mmj.v27i2.3
Ogata, H., Teixeira, M. M., Sousa, R. C., Silva, M. V., Correia, D., Rodrigues Junior, V., et al. (2016). Effects of aspirin-triggered resolvin D1 on peripheral blood mononuclear cells from patients with Chagas’ heart disease. Eur. J. Pharmacol. 777, 26–32. doi: 10.1016/j.ejphar.2016.02.058
Ohira, T., Arita, M., Omori, K., Recchiuti, A., Van Dyke, T. E., and Serhan, C. N. (2010). Resolvin E1 receptor activation signals phosphorylation and phagocytosis. J. Biol. Chem. 285, 3451–3461. doi: 10.1074/jbc.M109.044131
Oliveira, L. G., Souza-Testasicca, M. C., Vago, J. P., Figueiredo, A. B., Canavaci, A. M., Perucci, L. O., et al. (2017). Annexin A1 is involved in the resolution of inflammatory responses during leishmania braziliensis infection. J. Immunol. 198, 3227–3236. doi: 10.4049/jimmunol.1602028
Paiva, C. N., Arras, R. H., Lessa, L. P., Gibaldi, D., Alves, L., Metz, C. N., et al. (2007). Unraveling the lethal synergism between Trypanosoma cruzi infection and LPS: a role for increased macrophage reactivity. Eur. J. Immunol. 37, 1355–1364. doi: 10.1002/eji.200636705
Panaro, M. A., Brandonisio, O., Sisto, M., Acquafredda, A., Leogrande, D., Fumarola, L., et al. (2001). Nitric oxide production by Leishmania-infected macrophages and modulation by prostaglandin E2. Clin. Exp. Med. 1, 137–143. doi: 10.1007/s10238-001-8025-0
Paumi, C. M., Smitherman, P. K., Townsend, A. J., and Morrow, C. S. (2004). Glutathione S-transferases (GSTs) inhibit transcriptional activation by the peroxisomal proliferator-activated receptor gamma (PPAR gamma) ligand, 15-deoxy-delta 12,14prostaglandin J2 (15-d-PGJ2). Biochemistry 43,2345–2352. doi: 10.1021/bi035936
Penas, F., Mirkin, G. A., Hovsepian, E., Cevey, A., Caccuri, R., Sales, M. E., et al. (2013). PPARgamma ligand treatment inhibits cardiac inflammatory mediators induced by infection with different lethality strains of Trypanosoma cruzi. Biochim. Biophys. Acta 1832, 239–248. doi: 10.1016/j.bbadis.2012.08.007
Penas, F. N., Cevey, A. C., Siffo, S., Mirkin, G. A., and Goren, N. B. (2016). Hepatic injury associated with Trypanosoma cruzi infection is attenuated by treatment with 15-deoxy-Delta(12,14) prostaglandin J2. Exp. Parasitol. 170, 100–108. doi: 10.1016/j.exppara.2016.09.015
Penke, L. R., Sudan, R., Sathishkumar, S., and Saha, B. (2013). Prostaglandin E(2) receptors have differential effects on Leishmania major infection. Parasite Immunol. 35, 51–54. doi: 10.1111/pim.12011
Perretti, M., and D’Acquisto, F. (2009). Annexin A1 and glucocorticoids as effectors of the resolution of inflammation. Nat. Rev. Immunol. 9, 62–70. doi: 10.1038/nri2470
Plagge, M., and Laskay, T. (2017). Early production of the neutrophil-derived lipid mediators LTB4 and LXA4 is modulated by intracellular infection with Leishmania major. Biomed. Res. Int. 2017:2014583. doi: 10.1155/2017/2014583
Ponte-Sucre, A. (2016). An overview of trypanosoma brucei infections: an intense host-parasite interaction. Front. Microbiol. 7:1–12. doi: 10.3389/fmicb.2016.02126
Prado, C. M., Jelicks, L. A., Weiss, L. M., Factor, S. M., Tanowitz, H. B., and Rossi, M. A. (2011). The vasculature in chagas disease. Adv. Parasitol. 76, 83–99. doi: 10.1016/B978-0-12-385895-5.00004-9
Qu, Q., Xuan, W., and Fan, G. H. (2015). Roles of resolvins in the resolution of acute inflammation. Cell Biol. Int. 39, 3–22. doi: 10.1002/cbin.10345
Qu, X., Zhang, X., Yao, J., Song, J., Nikolic-Paterson, D. J., and Li, J. (2012). Resolvins E1 and D1 inhibit interstitial fibrosis in the obstructed kidney via inhibition of local fibroblast proliferation. J. Pathol. 228, 506–519. doi: 10.1002/path.4050
Quan, N., Mhlanga, J. D. M., Whiteside, M. B., Kristensson, K., and Herkenham, M. (2000). Chronic sodium salicylate treatment exacerbates brain neurodegeneration in rats infected with Trypanosoma brucei. Neuroscience 96, 181–194. doi: 10.1016/S0306-4522(99)00492-3
Rassi, A. Jr., Rassi, A., Marcondes, and de Rezende, J. (2012). American trypanosomiasis (Chagas disease). Infect. Dis. Clin. North Am. 26, 275–291. doi: 10.1016/j.idc.2012.03.002
Reiner, N. E., and Malemud, C. J. (1984). Arachidonic acid metabolism in murine leishmaniasis (Donovani): ex-vivo evidence for increased cyclooxygenase and 5-lipoxygenase activity in spleen cells. Cell Immunol. 88, 501–510. doi: 10.1016/0008-8749(84)90181-3
Reiner, N. E., and Malemud, C. J. (1985). Arachidonic acid metabolism by murine peritoneal macrophages infected with Leishmania donovani: in vitro evidence for parasite-induced alterations in cyclooxygenase and lipoxygenase pathways. J. Immunol. 134, 556–563.
Ribeiro, A. L., Nunes, M. P., Teixeira, M. M., and Rocha, M. O. (2012). Diagnosis and management of Chagas disease and cardiomyopathy. Nat. Rev. Cardiol. 9, 576–589. doi: 10.1038/nrcardio.2012.109
Rodrigues, W. F., Miguel, C. B., Chica, J. E., and Napimoga, M. H. (2010). 15d-PGJ(2) modulates acute immune responses to Trypanosoma cruzi infection. Mem. Inst. Oswaldo Cruz 105, 137–143. doi: 10.1590/S0074-02762010000200005
Romero, G. A., and Boelaert, M. (2010). Control of visceral leishmaniasis in latin america-a systematic review. PLoS Negl. Trop. Dis. 4:e584. doi: 10.1371/journal.pntd.0000584
Rossi, M. A., Tanowitz, H. B., Malvestio, L. M., Celes, M. R., Campos, E. C., Blefari, V., et al. (2010). Coronary microvascular disease in chronic chagas cardiomyopathy including an overview on history, pathology, and other proposed pathogenic mechanisms. PLoS Negl. Trop. Dis. 4:e674. doi: 10.1371/journal.pntd.0000674
Sacramento, L. A., Cunha, F. Q., de Almeida, R. P., da Silva, J. S., and Carregaro, V. (2014). Protective role of 5-lipoxigenase during Leishmania infantum infection is associated with Th17 subset. Biomed. Res. Int. 2014:264270. doi: 10.1155/2014/264270
Saha, A., Biswas, A., Srivastav, S., Mukherjee, M., Das, P. K., and Ukil, A. (2014). Prostaglandin E2 negatively regulates the production of inflammatory cytokines/chemokines and IL-17 in visceral leishmaniasis. J. Immunol. 193, 2330–2339. doi: 10.4049/jimmunol.1400399
Salmon, D., Vanwalleghem, G., Morias, Y., Denoeud, J., Krumbholz, C., Lhomme, F., et al. (2012). Adenylate cyclases of Trypanosoma brucei inhibit the innate immune response of the host. Science (New York, NY) 337, 463–466. doi: 10.1126/science.1222753
Schleifer, K. W., and Mansfield, J. M. (1993). Suppressor macrophages in African Trypanosomiasis inhibit T cell proliferative responses by nitric oxide and prostoglandins. J. Immunol. 151, 5492–5503.
Serezani, C. H., Perrela, J. H., Russo, M., Peters-Golden, M., and Jancar, S. (2006). Leukotrienes are essential for the control of Leishmania amazonensis infection and contribute to strain variation in susceptibility. J. Immunol. 177, 3201–3208. doi: 10.4049/jimmunol.177.5.3201
Serhan, C. N. (2005). Lipoxins and aspirin-triggered 15-epi-lipoxins are the first lipid mediators of endogenous anti-inflammation and resolution. Prostaglandins Leukot Essent Fatty Acids 73, 141–162. doi: 10.1016/j.plefa.2005.05.002
Serhan, C. N. (2007). Resolution phase of inflammation: novel endogenous anti-inflammatory and proresolving lipid mediators and pathways. Annu. Rev. Immunol. 25, 101–137. doi: 10.1146/annurev.immunol.25.022106.141647
Serhan, C. N. (2014). Pro-resolving lipid mediators are leads for resolution physiology. Nature 510, 92–101. doi: 10.1038/nature13479
Serhan, C. N., and Chiang, N. (2013). Resolution phase lipid mediators of inflammation: agonists of resolution. Curr. Opin. Pharmacol. 13, 632–640. doi: 10.1016/j.coph.2013.05.012
Serhan, C. N., Dalli, J., Colas, R. A., Winkler, J. W., and Chiang, N. (2015). Protectins and maresins: new pro-resolving families of mediators in acute inflammation and resolution bioactive metabolome. Biochim. Biophys. Acta 1851, 397–413. doi: 10.1016/j.bbalip.2014.08.006
Sharma, J., Eickhoff, C. S., Hoft, D. F., Ford, D. A., Gross, R. W., and McHowat, J. (2013). The absence of myocardial calcium-independent phospholipase A2gamma results in impaired prostaglandin E2 production and decreased survival in mice with acute Trypanosoma cruzi infection. Infect. Immun. 81, 2278–2287. doi: 10.1128/IAI.00497-12
Shinohara, M., and Serhan, C. N. (2016). Novel endogenous proresolving molecules: essential fatty acid-derived and gaseous mediators in the resolution of inflammation. J. Atheroscler. Thromb. 23, 655–664. doi: 10.5551/jat.33928
Shirey, K. A., Lai, W., Pletneva, L. M., Karp, C. L., Divanovic, S., Blanco, J. C., et al. (2014). Role of the lipoxygenase pathway in RSV-induced alternatively activated macrophages leading to resolution of lung pathology. Mucosal Immunol. 7, 549–557. doi: 10.1038/mi.2013.71
Shryock, N., McBerry, C., Salazar Gonzalez, R. M., Janes, S., Costa, F. T., and Aliberti, J. (2013). Lipoxin A(4) and 15-epi-lipoxin A(4) protect against experimental cerebral malaria by inhibiting IL-12/IFN-gamma in the brain. PLoS One 8:e61882. doi: 10.1371/journal.pone.0061882
Sileghem, M., Darji, A., Hamers, R., and De Baetselier, P. (1989). Modulation of IL-1 production and IL-1 release during experimental trypanosome infections. Immunology 68, 137–139.
Solca, M. S., Andrade, B. B., Abbehusen, M. M., Teixeira, C. R., Khouri, R., Valenzuela, J. G., et al. (2016). Circulating biomarkers of immune activation, oxidative stress and inflammation characterize severe canine visceral leishmaniasis. Sci. Rep. 6:32619. doi: 10.1038/srep32619
Souza, D. G., Fagundes, C. T., Amaral, F. A., Cisalpino, D., Sousa, L. P., Vieira, A. T., et al. (2007). The required role of endogenously produced lipoxin A4 and annexin-1 for the production of IL-10 and inflammatory hyporesponsiveness in mice. J. Immunol. 179, 8533–8543. doi: 10.4049/jimmunol.179.12.8533
Spite, M., Norling, L. V., Summers, L., Yang, R., Cooper, D., Petasis, N. A., et al. (2009). Resolvin D2 is a potent regulator of leukocytes and controls microbial sepsis. Nature 461, 1287–1291. doi: 10.1038/nature08541
Sternberg, J. M., and MacLean, L. (2010). A spectrum of disease in Human African trypanosomiasis: the host and parasite genetics of virulence. Parasitology 137, 2007–2015. doi: 10.1017/S0031182010000946
Sternberg, J. M., Rodgers, J., Bradley, B., MacLean, L., Murray, M., and Kennedy, P. G. E. (2005). Meningoencephalitic African trypanosomiasis: Brain IL-10 and IL-6 are associated with protection from neuro-inflammatory pathology. J. Neuroimmunol. 167, 81–89. doi: 10.1016/j.jneuroim.2005.06.017
Sugimoto, M. A., Vago, J. P., Teixeira, M. M., and Sousa, L. P. (2016). Annexin A1 and the resolution of inflammation: modulation of neutrophil recruitment, apoptosis, and clearance. J. Immunol. Res. 2016:8239258. doi: 10.1155/2016/8239258
Sundblad, V., Morosi, L. G., Geffner, J. R., and Rabinovich, G. A. (2017). Galectin-1: a jack-of-all-trades in the resolution of acute and chronic inflammation. J. Immunol. 199, 3721–3730. doi: 10.4049/jimmunol.1701172
Tavares, N. M., Araujo-Santos, T., Afonso, L., Nogueira, P. M., Lopes, U. G., Soares, R. P., et al. (2014). Understanding the mechanisms controlling Leishmania amazonensis infection in vitro: the role of LTB4 derived from human neutrophils. J. Infect. Dis. 210, 656–666. doi: 10.1093/infdis/jiu158
Van Dyke, T. E. (2017). Pro-resolving mediators in the regulation of periodontal disease. Mol. Aspects Med. 58, 21–36. doi: 10.1016/j.mam.2017.04.006
Venturin, G. L., Chiku, V. M., Silva, K. L., de Almeida, B. F., and de Lima, V. M. (2016). M1 polarization and the effect of PGE2 on TNF-alpha production by lymph node cells from dogs with visceral leishmaniasis. Parasite Immunol. 38, 698–704. doi: 10.1111/pim.12353
Vishwakarma, P., Parmar, N., Yadav, P. K., Chandrakar, P., and Kar, S. (2016). 15d-Prostaglandin J2 induced reactive oxygen species-mediated apoptosis during experimental visceral leishmaniasis. J. Mol. Med. (Berl.) 94, 695–710. doi: 10.1007/s00109-016-1384-5
Walker, J., Dichter, E., Lacorte, G., Kerner, D., Spur, B., Rodriguez, A., et al. (2011). Lipoxin a4 increases survival by decreasing systemic inflammation and bacterial load in sepsis. Shock 36, 410–416. doi: 10.1097/SHK.0b013e31822798c1
Wallace, J. L., Ianaro, A., Flannigan, K. L., and Cirino, G. (2015). Gaseous mediators in resolution of inflammation. Semin. Immunol. 27, 227–233. doi: 10.1016/j.smim.2015.05.004
Wan, M., Godson, C., Guiry, P. J., Agerberth, B., and Haeggstrom, J. Z. (2011). Leukotriene B4/antimicrobial peptide LL-37 proinflammatory circuits are mediated by BLT1 and FPR2/ALX and are counterregulated by lipoxin A4 and resolvin E1. FASEB J. 25, 1697–1705. doi: 10.1096/fj.10-175687
Wang, C. W., Colas, R. A., Dalli, J., Arnardottir, H. H., Nguyen, D., Hasturk, H., et al. (2015a). Maresin 1 biosynthesis and proresolving anti-infective functions with human-localized aggressive periodontitis leukocytes. Infect. Immun. 84, 658–665. doi: 10.1128/IAI.01131-15
Wang, X., Zhu, M., Hjorth, E., Cortes-Toro, V., Eyjolfsdottir, H., Graff, C., et al. (2015b). Resolution of inflammation is altered in Alzheimer’s disease. Alzheimers Dement. 11, 40.e1-2–50.e1-2. doi: 10.1016/j.jalz.2013.12.024
Wang, H., Anthony, D., Yatmaz, S., Wijburg, O., Satzke, C., Levy, B., et al. (2017). Aspirin-triggered resolvin D1 reduces pneumococcal lung infection and inflammation in a viral and bacterial coinfection pneumonia model. Clin. Sci. (Lond.) 131, 2347–2362. doi: 10.1042/CS20171006
Wenzel, A., and Van Zandbergen, G. (2009). Lipoxin A4 receptor dependent leishmania infection. Autoimmunity 42, 331–333. doi: 10.1080/08916930902828239
Wu, B., Capilato, J., Pham, M. P., Walker, J., Spur, B., Rodriguez, A., et al. (2016). Lipoxin A4 augments host defense in sepsis and reduces Pseudomonas aeruginosa virulence through quorum sensing inhibition. FASEB J. 30,2400–2410. doi: 10.1096/fj.201500029R
Wu, B., Walker, J., Spur, B., Rodriguez, A., and Yin, K. (2015). Effects of lipoxin A4 on antimicrobial actions of neutrophils in sepsis. Prostaglandins Leukot Essent Fatty Acids 94, 55–64. doi: 10.1016/j.plefa.2014.11.005
Ye, Y., Lin, Y., Perez-Polo, J. R., Uretsky, B. F., Ye, Z., Tieu, B. C., et al. (2008). Phosphorylation of 5-lipoxygenase at ser523 by protein kinase A determines whether pioglitazone and atorvastatin induce proinflammatory leukotriene B4 or anti-inflammatory 15-epi-lipoxin a4 production. J. Immunol. 181,3515–3523. doi: 10.4049/jimmunol.181.5.3515
Keywords: resolution of inflammation, prostaglandins, leukotrienes, resolvins, lipoxins, Trypanosoma cruzi, Trypanosoma brucei spp., Leishmania spp.
Citation: López-Muñoz RA, Molina-Berríos A, Campos-Estrada C, Abarca-Sanhueza P, Urrutia-Llancaqueo L, Peña-Espinoza M and Maya JD (2018) Inflammatory and Pro-resolving Lipids in Trypanosomatid Infections: A Key to Understanding Parasite Control. Front. Microbiol. 9:1961. doi: 10.3389/fmicb.2018.01961
Received: 03 April 2018; Accepted: 02 August 2018;
Published: 21 August 2018.
Edited by:
Walderez Ornelas Dutra, Universidade Federal de Minas Gerais, BrazilReviewed by:
Keke Celeste Fairfax, Purdue University, United StatesJyothi Nagajyothi, Rutgers, The State University of New Jersey, Newark, United States
Copyright © 2018 López-Muñoz, Molina-Berríos, Campos-Estrada, Abarca-Sanhueza, Urrutia-Llancaqueo, Peña-Espinoza and Maya. This is an open-access article distributed under the terms of the Creative Commons Attribution License (CC BY). The use, distribution or reproduction in other forums is permitted, provided the original author(s) and the copyright owner(s) are credited and that the original publication in this journal is cited, in accordance with accepted academic practice. No use, distribution or reproduction is permitted which does not comply with these terms.
*Correspondence: Rodrigo A. López-Muñoz, cm9kcmlnby5sb3BlekB1YWNoLmNs Juan D. Maya, amRtYXlhQHVjaGlsZS5jbA==