- 1Faculty of Biosciences and Aquaculture, Nord University, Bodø, Norway
- 2Department of Cell Biology and Histology, Faculty of Biology, Institute of Biomedical Research of Murcia-Arrixaca, University of Murcia, Murcia, Spain
Establishment of the early-life gut microbiota has a large influence on host development and succession of microbial composition in later life stages. The effect of commensal yeasts - which are known to create a conducive environment for beneficial bacteria - on the structure and diversity of fish gut microbiota still remains unexplored. The present study examined the intestinal bacterial community of zebrafish (Danio rerio) larvae exposed to two fish-derived yeasts by sequencing the V4 hypervariable region of bacterial 16S rRNA. The first stage of the experiment (until 7 days post-fertilization) was performed in cell culture flasks under sterile and conventional conditions for germ-free (GF) and conventionally raised (CR) larvae, respectively. The second phase was carried out under standard rearing conditions, for both groups. Exposure of GF and CR zebrafish larvae to one of the yeast species Debaryomyces or Pseudozyma affected the bacterial composition. Exposure to Debaryomyces resulted in a significantly higher abundance of core bacteria. The difference was mainly due to shifts in relative abundance of taxa belonging to the phylum Proteobacteria. In Debaryomyces-exposed CR larvae, the significantly enriched taxa included beneficial bacteria such as Pediococcus and Lactococcus (Firmicutes). Furthermore, most diversity indices of bacterial communities in yeast-exposed CR zebrafish were significantly altered compared to the control group. Such alterations were not evident in GF zebrafish. The water bacterial community was distinct from the intestinal microbiota of zebrafish larvae. Our findings indicate that early exposure to commensal yeast could cause differential bacterial assemblage, including the establishment of potentially beneficial bacteria.
Introduction
Early microbial colonization of the gastrointestinal tract plays a key role in immunological and metabolic development of the host, which influences their health and disease status later in life (Rodríguez et al., 2015; Tanaka and Nakayama, 2017). Host–microbe coevolution has resulted in parasitic, commensal, or mutualistic relationships between the gut microbial community members and their host (Ley et al., 2008). These complex relationships are shaped by the indigenous intestinal microbial communities, which include bacteria, fungi, viruses, and protozoans (Barko et al., 2018). The initial microbial colonizers are essential for the development of neonatal gut epithelial barrier functions, maturation, and modulation of innate and adaptive immune responses (Hooper and Gordon, 2001). In addition, they may provide favorable conditions for subsequent colonization of microbial members (Houghteling and Walker, 2015) and create the platform for establishment of persistent microbial communities (Faith et al., 2013; Houghteling and Walker, 2015). Thus, the microbial composition in adults may reflect the history of early-life events, such as initial encounter with microbes, colonization and establishment of microbes in the gastrointestinal tract (Rodríguez et al., 2015).
Microbial colonization of the fish gut during larval stages is known to occur during either active uptake of water or the time of first feeding (Reitan et al., 1998; Vadstein et al., 2013). Microbes associated with chorion of fish eggs, surrounding water, and early diet influence the composition of the larval gut microbiota (Llewellyn et al., 2014). This community is further shaped by host ontogeny stage, habitat (Stephens et al., 2016) and the gut environmental conditions such as availability of nutrients, pH, and digestive enzymes (Hansen and Olafsen, 1999). The diversity of fish gut microbiota is affected by early-life factors including life stages, diet, and rearing environment on fish gut microbiota composition (Romero et al., 2014; Lokesh et al., 2018). In this context, probiotics are microorganisms that are administered in diets to promote host health and potentially modulate the gut microbiota throughout fish ontogeny from larvae to adult (Montalban-Arques et al., 2015; Ringø et al., 2016). Improving the beneficial microbial community, by altering gut microbial composition through dietary administration of probiotics, will increase feed efficiency and growth, and to maintain the health and wellbeing of aquatic animals (Llewellyn et al., 2014). For instance, lactic acid bacteria (LAB) are considered as probiotic candidates in aquaculture (Llewellyn et al., 2014) because they provide nutritional benefits and protect the host from pathogenic infections (Balcázar et al., 2007; Martínez Cruz et al., 2012; Hai, 2015).
The effect of application of yeast and their derivatives as probiotics, immunostimulants, and feed supplements is also well-documented in different animals including fish (Navarrete and Tovar-Ramírez, 2014; Vohra et al., 2016). For example, European sea bass (Dicentrarchus labrax) larvae fed live yeast Debaryomyces hansenii had improved growth, feed efficiency, survival, and immune competence (Tovar-Ramírez et al., 2004, 2010). Additionally, feeding juvenile European sturgeon (Huso huso) with a live yeast-supplemented diet increased the proportion of LAB in the gut (Hoseinifar et al., 2011), even if their significance for the host remains to be elucidated (Hoseinifar et al., 2011). Nevertheless, the presence of different LAB strains could have a positive effect on host; they exert different competition mechanisms (hydrogen peroxide, lactic acid, bacteriocin-like molecules) to inhibit growth of pathogens and stimulate the host immune system (Ringø and Gatesoupe, 1998; Verschuere et al., 2000; Campana et al., 2017). In a recent study, it was reported that feeding juvenile rainbow trout (Oncorhynchus mykiss) with functional diet incorporated with a probiotic yeast (Saccharomyces cerevisiae) changed the intestinal microbiota (Gonçalves and Gallardo-Escárate, 2017). Rather than allochthonous microorganisms, autochthonous microorganisms are potential functional food ingredients for modulating the composition of microbiota (O’Toole and Cooney, 2008).
To date, there is limited information about the impact of yeast on the establishment of the gut bacterial community in fish, particularly during early developmental stages. Nevertheless, it is known that the gut microbiota in fish larvae is less stable than in adults (Gatesoupe, 1999). Hence, yeast exposure during early ontogeny has the potential to modify the composition and improve the functionality of gut microbiota in fish larvae. We hypothesized that early exposure to yeast may alter the fish gut microbiota. To test this hypothesis, we explored the potential of two yeast species Debaryomyces sp. and Pseudozyma sp., which were originally isolated from Atlantic salmon (Salmo salar) and zebrafish (Danio rerio), respectively. Debaryomyces is frequently associated with fish and it has been considered as an excellent probiotic candidate because of its beneficial and therapeutic properties (Navarrete and Tovar-Ramírez, 2014). On the other hand, though Pseudozyma sp. is not commonly reported in fish, P. fusiformata has been detected in the gut of wild salmonids (Raggi et al., 2014). We investigated the effect of early yeast exposure on the intestinal microbiota composition of zebrafish larvae raised in germ-free (GF) or conventional conditions by sequencing the V4 hypervariable region of the bacterial 16S rRNA gene. Our findings provide the first evidence that fish-derived yeast influence the assembly of the bacterial communities during early life that could lead to a healthy gut environment in fish.
Materials and Methods
Yeast Strains and Culture Conditions
Debaryomyces sp. and Pseudozyma sp. used in this study were originally isolated from the intestine of Atlantic salmon and zebrafish, respectively, at Nord University, Bodø. The isolated yeast colonies were identified by PCR amplification and Sanger sequencing of the internal transcribed spacer 2 (ITS2) region of fungal rDNA. Pure cultures of the yeasts were prepared and stored in 30% glycerol (Sigma-Aldrich St. Louis, MO, United States) at -80°C. Prior to use, the cultures were revived on yeast extract peptone dextrose agar (Sigma-Aldrich) plate and broth supplemented with 0.025% chloramphenicol (Sigma-Aldrich). They were further grown in yeast extract peptone dextrose broth at 28°C, shaking the broth at 180–200 rpm for 24 h. The cultured yeast cells were harvested by centrifugation at 10,000 rpm for 10 min. Subsequently, the harvested cells were washed and resuspended in sterile phosphate-buffered saline (PBS) to obtain a final concentration of 2 × 105 CFU/ml for the following exposure study.
Ethics Statement
The experiments performed comply with the Guidelines of the European Union Council (Directive 2010/63/EU) and the Spanish RD 53/2013. Experiments and procedures were performed as approved by the Bioethical Committee of the University of Murcia (approval numbers #537/2011, #75/2014, and #216/2014).
Zebrafish Husbandry and Preparation of Larvae
The experiments were conducted at the laboratories of the Department of Cell Biology and Histology, University of Murcia. Standard husbandry procedures (Westerfield, 2007) were followed to maintain wild type zebrafish in a re-circulation Fish-box system (Aqua Medic GmBH, Bissendorf, Germany). Fish were offered a commercial diet (GEMMA Micro 300, Skretting, Burgos, Spain) and Artemia salina nauplii, two times a day. Adult zebrafish (1 male: 2 female) were introduced into 1 L breeding tanks with dividers that kept the males and females apart overnight. The following morning the dividers were removed to allow natural spawning of fish. The eggs were evenly split into two groups; one-half of the eggs were used to generate GF embryos as described by Galindo-Villegas et al. (2012). Subsequently, GF embryos were reared in sterile, vented tissue culture flasks containing autoclaved and filtered egg water without antibiotics. The remaining half of the collected eggs were conventionally raised (CR) following same strategy but using regular embryo medium as described elsewhere (Galindo-Villegas et al., 2012). Both groups were carefully monitored daily, and dead eggs, if any, were aseptically removed. In addition, 50% media was daily replaced in each flask according to the respective initial conditions. The sterility of GF fish water media was daily confirmed by visual assessment of inoculated agar plates for microbial growth, as well as by conventional PCR using V4f and V4r primer pairs targeting the V4 region of the bacterial 16S rRNA (Kozich et al., 2013).
Design of the Yeast Exposure Study
The experiments employing GF and CR zebrafish larvae were done in two phases. The first stage (from 0 to 7 days post-fertilization, dpf) was performed in experimental flasks under sterile or conventional conditions for GF and CR larvae, respectively. The second phase was carried out from 7 to 14 dpf using 1.5 L tanks under standard rearing conditions, for both groups. These tanks received water from the zebrafish recirculation system. The larvae did not receive any feed during the first phase. They were offered a commercial larval starter microfeed (NovoTom Artemia, JBL, Neuhofen, Germany) from 7 until 14 dpf, i.e., the second phase. The rearing conditions in the flasks/tanks were: temperature 28.0 ± 0.5°C, pH 7.5 and 12 h light/ 12 h dark cycle.
Zebrafish larvae, both GF and CR, were aseptically divided into three treatment groups (Control, Debaryomyces, and Pseudozyma) (Figure 1). Each group of larvae was maintained in triplicate flasks, prior to immersion-exposure to yeasts. At 2 dpf, GF and CR zebrafish larvae were exposed to 2 × 105 CFU/ml Debaryomyces sp. or Pseudozyma sp. and incubated for 24 h (until 3 dpf) at 28°C. On the other hand, the control larvae were exposed to PBS. After the 24 h exposure, larvae were washed (3×) with regular embryo medium and raised in system water (CR) or gnotobiotic medium (GF) until 7 dpf. Rearing media were aseptically replaced daily with fresh media. At 7 dpf, larvae were transferred to small tanks, where they were raised until 14 dpf.
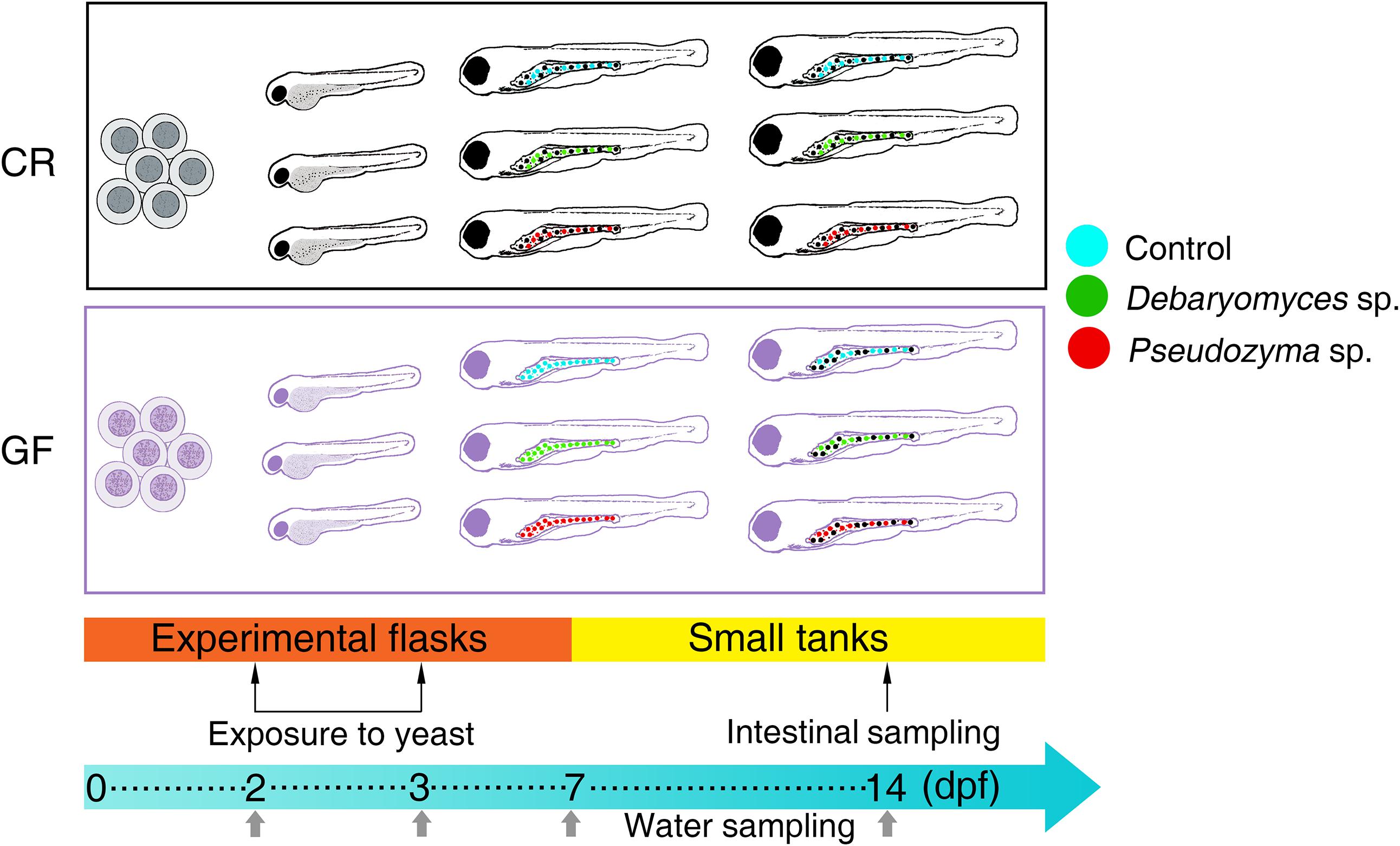
FIGURE 1. Design of the yeast exposure study. The alterations in the intestinal bacterial communities of conventionally raised (CR) and germ-free (GF) zebrafish larvae were examined after exposing them to the yeasts Debaryomyces sp. or Pseudozyma sp. for 24 h. The larvae in the control group were exposed to PBS. The intestine samples were collected on day 14 and water samples from their respective flasks/tanks were collected at days 2, 3, 7, and 14.
The different study groups and their abbreviations are: CRC, conventionally raised control; CRD, conventionally raised Debaryomyces-exposed; CRP, conventionally raised Pseudozyma-exposed; GFC, germ-free control; GFD, germ-free Debaryomyces-exposed; GFP, germ-free Pseudozyma-exposed. For water samples of the different groups, we followed the same nomenclature as above, but inserted the letter “W.” One extra group representing zebrafish system water, ZSW, was also included for comparison.
Collection of Intestine and Water Samples
At 14 dpf, zebrafish larvae were euthanized with an overdose of MS222 (Sigma-Aldrich, Madrid, Spain) and rinsed with sterile water before dissection. The gastrointestinal tract of each laterally placed larva was aseptically excised under a Leica MZ16F fluorescence stereo microscope (Leica Microsystems, Wetzlar, Germany). The intestinal content was gently scraped off with Dumont #5 fine forceps (Fine Science Tools GmbH, Heidelberg, Germany). Each sample was a pool of intestines from 7 larvae; 2 to 3 samples were collected from each tank, making a total of 6 to 9 samples per treatment group. All samples were immediately frozen using dry ice and stored at -80°C until DNA extraction. Water samples from 3 replicate flasks or tanks for each treatment group were pooled and filtered using 0.2 μm pore size filter (Merck, Darmstadt, Germany) and stored at -80°C until use. In addition, we obtained water samples from the zebrafish facilities, which were also filtered and stored at -80°C before DNA extraction.
Isolation of DNA, PCR Amplification and Sequencing
Genomic DNA from the pooled intestinal samples was extracted using the QIAamp Fast DNA Stool Mini Kit (Qiagen, Hilden, Germany) according to the manufacturer’s instructions, with minor modifications as described in our earlier publication (Siriyappagouder et al., 2018). In addition, we used the QIAamp DNA micro spin column instead of QIAamp column to purify and elute the DNA. DNA from water samples was extracted with the Metagenomic DNA isolation kit for water (Epicentre, Madison, WI, United States). The extracted DNA was stored at -20°C until further analyses.
To profile bacterial communities, the V4 hypervariable region (∼250 base pairs) of bacterial 16S rRNA gene was amplified using the primer pair V4f and V4r attached with Illumina barcoded adapters and sample-specific indices (Kozich et al., 2013). PCR reactions were performed in a reaction volume of 20 μl containing 13 μl of KAPA KAPA HiFi HotStart ReadyMix (2X) (KAPA Biosystems, Woburn, MA, United States), 0.3 μM of each primer, 3 μl DNA template and sterile water up to 20 μl. Distilled water and genomic DNA from the bacterial mock community (ZymoBIOMICSTM Microbial Community Standard, Zymo research, Irvine, CA, United States) were used as negative and positive controls, respectively. The DNA from each sample was independently amplified in duplicate reactions in a thermocycler (Bio-Rad Laboratories, Inc., Hercules, CA, United States) with the following thermocycling conditions: initial denaturation at 95°C for 5 min, followed by 35 cycles of 98°C for 30 s, 58°C for 30 s, and 72°C for 45 s and a final elongation at 72°C for 2 min. The resulting amplicon replicates were pooled and run on a 1.2% (w/v) agarose gel and positive amplicons were excised from the gel and further purified using the QIAquick Gel Extraction Kit (Qiagen).
Purified amplicon libraries were quantified with Qubit 3.0 Fluorometer and Qubit® dsDNA HS Assay Kit (Thermo Fisher Scientific – Invitrogen, Waltham, MA, United States). All libraries were pooled in an equimolar ratio to 4 nM. Fragment size and library concentration were further confirmed on the Bioanalyzer 2200 TapeStation system (Agilent Technologies, Santa Clara, CA, United States). The normalized library pool was further diluted to 10 pM, spiked with 20% (10 pM) PhiX control and sequenced on an Illumina Miseq platform (Illumina, San Diego, CA, United States) using the MiSeq® reagent kit V3 to generate 2 × 300 base pair reads.
Sequence Data, Quality Control and Processing
Sequence data were processed and analyzed using USEARCH v9.2.64 (Edgar, 2013) and QIIME v1.9.1 (Caporaso et al., 2010) pipelines. Quality of raw sequencing reads were assessed using FastQC software (Andrews, 2010). The sequence length of the V4 hypervariable region of 16S rRNA gene is approximately 250 base pairs for most microbial species (Kozich et al., 2013), and it was individually covered by both forward and reverse reads. Only forward reads were retained for downstream analysis since their quality was generally better than the corresponding reverse reads. They were trimmed to 260 base pairs; to remove the primer sequence and any subsequent sequences at the 3′ end. Sequences with less than 260 base pairs were removed. A maximum expected error filter strategy with a threshold of 1 was adopted to get rid of the low quality reads (Edgar and Flyvbjerg, 2015). Chimeric sequences were removed using the UCHIME algorithm (Edgar et al., 2011). Then, quality filtered sequences were clustered into operational taxonomic units (OTUs) at 97% sequence similarity threshold. The representative sequences of OTUs were assigned to different taxa using the SINTAX algorithm (Edgar, 2016) employing the 16S rRNA RDP database (v11.5) and choosing a confidence cutoff of 0.5. Subsequently, OTUs with a confidence score <1 at domain level and the 12 OTUs belonging to the phylum Cyanobacteria were removed since they only accounted for 0.0092% of the total reads. All samples were rarefied to 20,000 sequences and rarefaction curves were obtained by plotting the species richness against the number of sequences per sample. The raw 16S sequence data from this study has been deposited at the European Nucleotide Archive database (EBI) under the accession number ERS2484299 to ERS2484358.
Data Visualization and Statistical Analysis
We executed R codes in RStudio v1.1.442 (R Development Core Team, 2018) and employed the undermentioned packages to analyze the data. Data were imported into R using the package ‘xlsx.’ The package ‘phyloseq’ v1.22.3 (McMurdie and Holmes, 2013) was used to make phyloseq objects. The data visualization was done mainly using ‘ggplot2’ v2.2.1 (Wickham, 2010). Packages ‘iNEXT’ v2.0.12 (Hsieh et al., 2016) and microbiome v1.0.2 (Lahti et al., 2017) were used to create the rarefaction curves and boxplots of diversity indices.
Pearson’s chi-squared followed by Benjamin–Hochberg post hoc tests were performed to determine if the proportions of Proteobacteria/Bacteroidetes in the treatment groups of CR and GF fish differed significantly. Significance was set at P < 0.05. To determine the alpha diversity of bacterial communities, species richness, Shannon diversity, and Simpson diversity [effective number of species, Jost (2006)], phylogenetic diversity (PD whole tree, Faith and Baker, 2006), core abundance and rare low abundance [relative proportion of core species and least abundant species (Lahti et al., 2017)] were analyzed. The Kruskal–Wallis test and Dunn’s test with the Benjamini–Hochberg FDR correction were used to identify significant differences in the alpha diversity indices of the groups. To assess the beta diversity, principal coordinate analysis was performed on the matrices generated using weighted UniFrac distances (Lozupone and Knight, 2005). The sample sizes of the experimental groups were different, and we checked for the heterogeneity of dispersions using the function betadisper from vegan v2.4-6 by Oksanen et al. (2018). Analysis of similarity (ANOSIM, employing 999 permutations) and Adonis in the R package ‘vegan’ were employed to understand the differences in clustering and the locations of their centroids. We have examined the differentially abundant bacterial communities of the groups by analyzing the data using linear discriminant analysis effect size (LEfSe) (Segata et al., 2011). An alpha value of 0.05 for the factorial Kruskal–Wallis test and a threshold of 3.5 for the logarithmic LDA score were selected to understand the discriminative features.
Results
Sterility of GF and CR Zebrafish Larvae
Microbial status or sterility of larvae was confirmed before yeast exposure. There was no bacterial growth on TSA agar plate inoculated with samples from GF larvae and media. Furthermore, we did not observe any bands after PCR amplification of the 16S rRNA from larvae samples until 7 dpf and water/media from GF zebrafish. On the other hand, CR larvae and rearing media samples produced positive amplification of 16S rRNA; for samples collected at all the sampling points (2, 3, 7, and 14 dpf).
Sequencing Quality
We analyzed the 16S rRNA V4 amplicon sequences of the bacterial communities of 62 samples. Of these, 25 were intestine samples of CR larvae, 20 were those of GF larvae and remaining 17 were of water from the respective treatment groups and zebrafish facility. We obtained a total of 5,150,530 high-quality reads after adapter and quality trimming of 5,650,637 raw sequences (Supplementary Table S1). They were clustered into 2095 OTUs at 97% identity threshold; the OTUs represented 399 different taxa, of which 308 were assigned to specific genera. The majority of the rarefaction curves reached the saturation point at 20,000, allowing us to capture most of the underlying diversity (Supplementary Figure S1). Hence, 20,000 sequences per sample were considered to be appropriate for further analysis.
Relative Abundance and Core Microbiota in Zebrafish Larvae
Analysis of the intestine samples revealed that Proteobacteria (97.8%) was the dominant phylum, followed by Bacteroidetes (1.5%). Actinobacteria, Firmicutes, Fusobacteria and remaining rare phyla accounted for the residual 0.7% of the reads (Figure 2A).
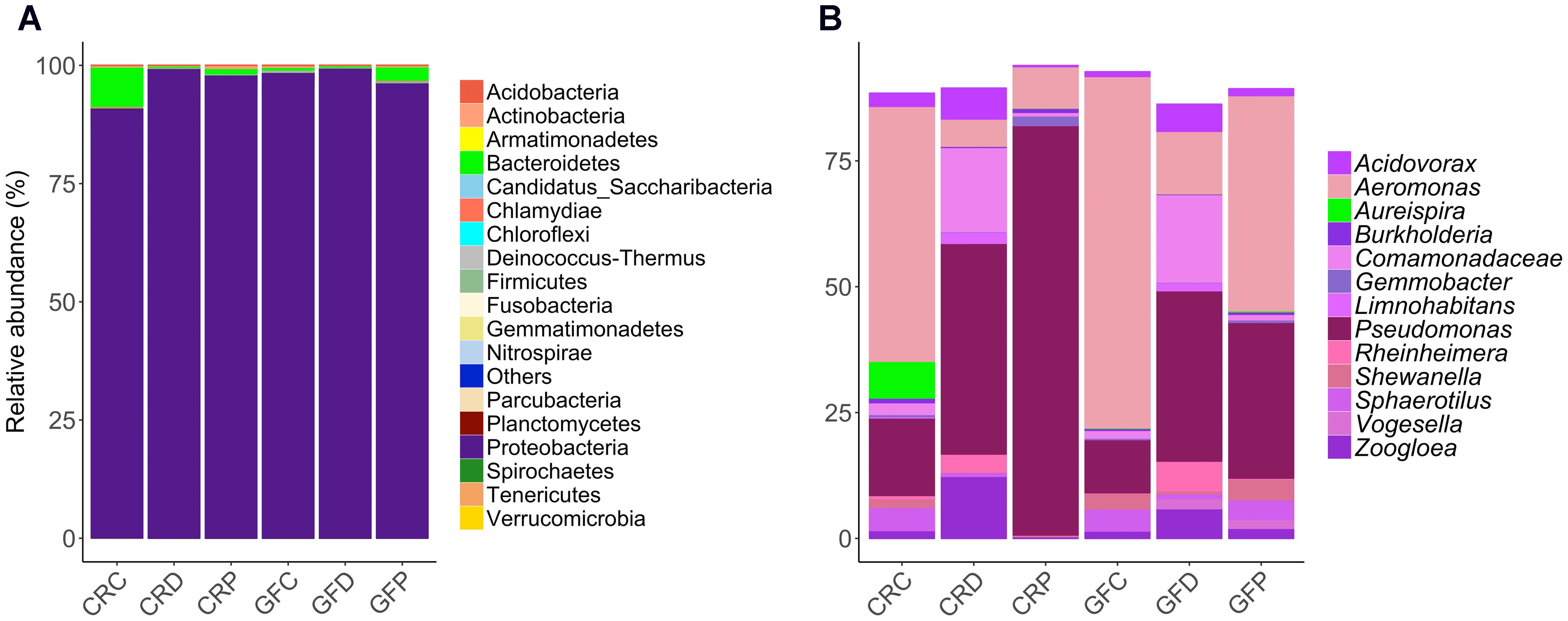
FIGURE 2. Relative abundance of the intestinal bacterial communities of zebrafish larvae, at different taxonomic levels. Phyla (A) and the top abundant genera (B) in CR and GF zebrafish. Each bar segment representing the average relative abundance of a particular bacterial taxon within a group is color coded: Proteobacteria – shades of purple and pink, and Bacteroidetes – green. CRC, conventionally raised control; CRD, conventionally raised Debaryomyces-exposed; CRP, conventionally raised Pseudozyma-exposed; GFC, germ-free control; GFD, germ-free Debaryomyces-exposed; GFP, germ-free Pseudozyma-exposed.
The abundance profile at genus level (Figure 2B) indicated that the most common genera were present in all larvae groups. Differences between bacterial communities in control and yeast-exposed groups were due to different proportions of these core bacteria as well as some rare genera, which are not shown in Figure 2B. The dominant genera, namely Aeromonas, Acidovorax, Pseudomonas, Rheinheimera, Shewanella, Sphaerotilus, Gemmobacter, Zoogloea and unidentified genera of the family Comamonadaceae were found among the core microbiota (shared by 97% of the samples) in the zebrafish larvae (Supplementary Figure S2). In contrast, some low abundant and rare taxa mainly Staphylococcus, Photobacterium, Comamonas, Plesiomonas, Vibrio, and Cetobacterium were unique to some samples, but we did not find any considerable correlation of these genera with yeast exposure.
Yeast-Exposed Larvae Display a Distinct Bacterial Relative Abundance Compared to the Control Larvae
The relative abundance of phylum-level taxa indicates that Proteobacteria is the predominant phylum in all the treatment groups of CR larvae. However, the proportion of Bacteroidetes was higher in CRC (8.8%, P = 0) in comparison to Debaryomyces-exposed (0.3%) and Pseudozyma-exposed (1.1%) CR larvae (Figure 2A). These differences were more evident at lower taxonomic levels (Supplementary Figures S3A–C). Additionally, the relative abundance of the 20 most abundant taxa revealed notable differences between conventionally raised control (CRC) and yeast-exposed groups (CRD/CRP; Figure 2B). At the genus level, Aeromonas and Pseudomonas were the two predominant taxa. The abundance of Aeromonas in yeast-exposed groups decreased, while that of Pseudomonas increased compared to the control group (Figure 2B). A similar pattern was also observed in the GFC compared to the corresponding yeast-exposed groups (GFD, GFP; Figures 2A,B).
The microbial community compositions of CRC and yeast-exposed larvae were significantly different, according to the weighted UniFrac distance-based analysis, which took the bacterial abundance into account rather than presence and absence of taxa. PCoA analysis revealed a clear clustering pattern; samples were largely partitioned based on the yeast exposure (Figure 3A, ANOSIM; R = 0.80, P < 0.001 and Adonis; R2 = 0.55, P < 0.001). Even though axis one captures more variation than axis two, the samples were better separated by axis two. Significant differences between the groups (CRC vs. CRD – P < 0.001, CRC vs. CRP – P < 0.001 and CRD vs. CRP – P < 0.001) were revealed when the groups were considered separately in pairwise comparisons. Samples of the CRC group clustered near the CRD samples, while those of the CRP clustered separately.
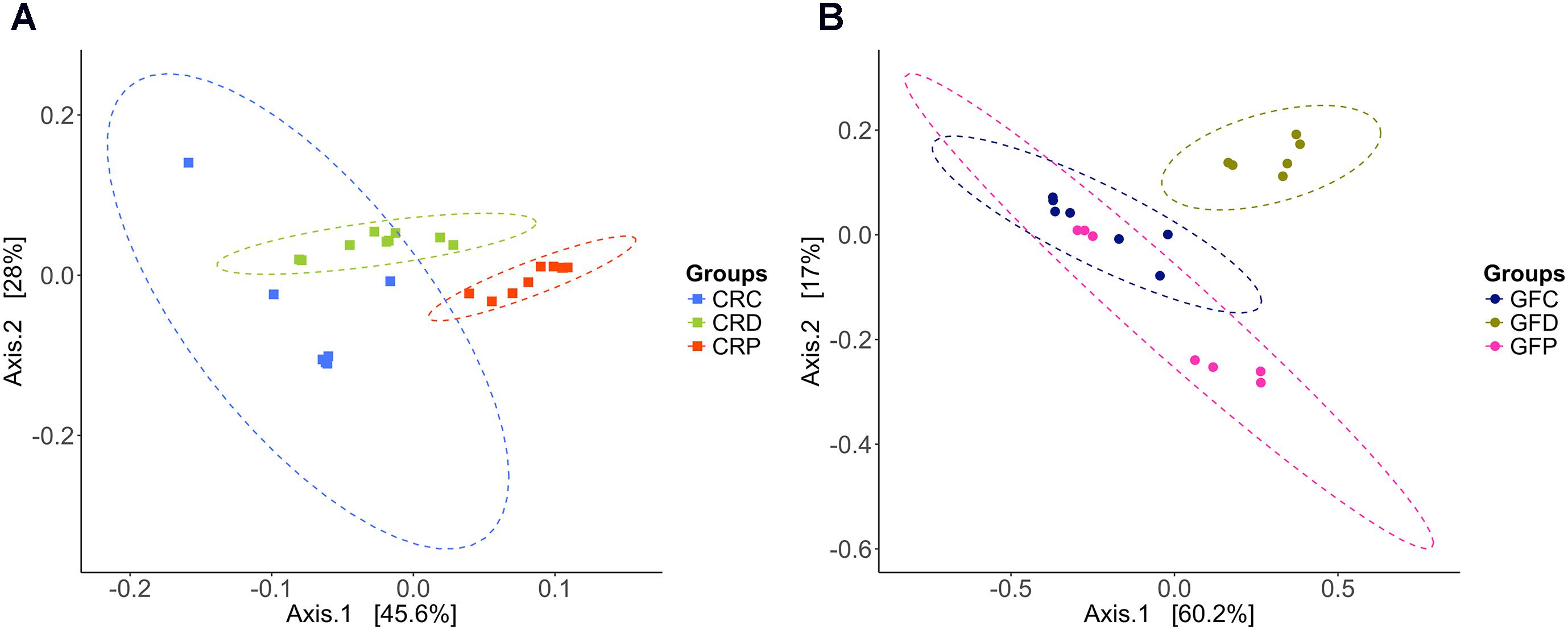
FIGURE 3. Principal coordinate analysis plots based on weighted UniFrac distance metric show the distinct intestinal bacterial communities in CR (A), and GF (B) of zebrafish larvae. Ellipses include 95% of samples from normally distributed data. CRC, conventionally raised control; CRD, conventionally raised Debaryomyces-exposed; CRP, conventionally raised Pseudozyma-exposed; GFC, germ-free control; GFD, germ-free Debaryomyces-exposed; GFP, germ-free Pseudozyma-exposed.
Regarding the GF samples, the cluster of the GFD group was distinct compared to GFC and GFP (ANOSIM; R = 0.57, P < 0.001 and Adonis; R2 = 0.50, P < 0.001, Figure 3B). The GFP group clustered closer to GFC, unlike the CRP group of the CR larvae. Significant difference between groups (GFC vs. GFD – P < 0.01, GFC vs. GFP – P < 0.05 and GFD vs. GFP – P < 0.01) were also observed during pairwise comparisons.
Bacterial Diversity Was Significantly Affected by Exposure to Yeast
We did not observe any significant differences in species richness and Shannon diversity indices (Figures 4A,B) between CRC and CRD groups (P > 0.05) but when we compared Pseudozyma-exposed group (CRP) with CRD significant differences were obtained (P < 0.01) or CRC (P < 0.01). The species richness and evenness of the community was relatively higher (P > 0.05) in the CRD compared to the CRP group. Simpson diversity (dominance) of the CRP group was significantly lower when compared to the CRD (P < 0.001), whereas no significant difference was observed for the CRD vs. CRC comparison (P > 0.05; Figure 4C). Microbial phylogenetic diversity decreased as a result of exposure to Pseudozyma (CRP); the value was higher in the CRC (P < 0.01), but that of the CRD group was not significantly different (P > 0.05; Figure 4D). The core abundance value of the CRP group was significantly different from the CRC group (P < 0.05) but similar to CRD (P > 0.05; Figure 4E). The rare low abundance of the CRP group was significantly lower than that of CRC (P < 0.01) and CRD (P < 0.05; Figure 4F).
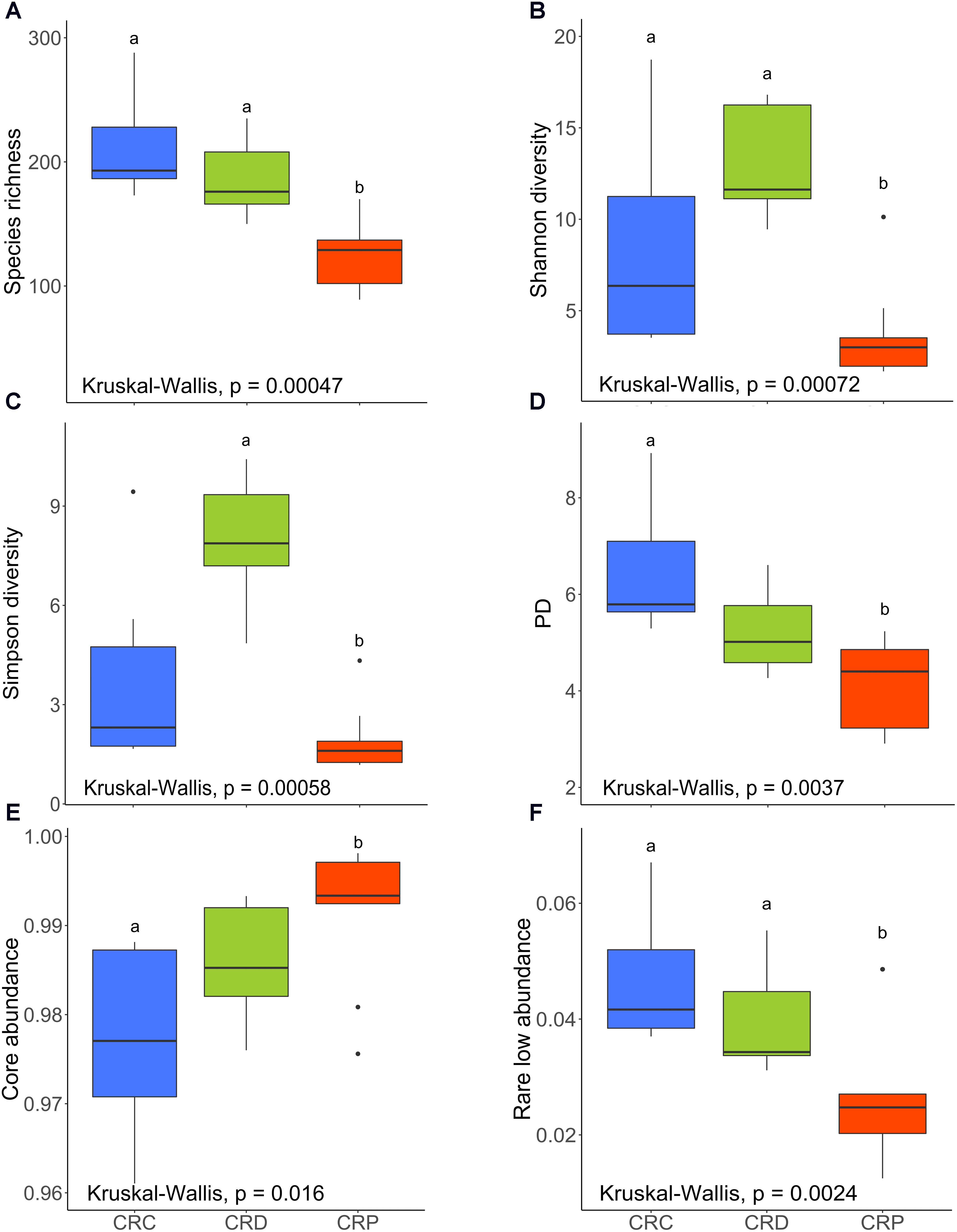
FIGURE 4. Alpha diversity indices of the intestinal bacterial communities of CR zebrafish larvae. Species richness (A), Shannon diversity (B), Simpson diversity (C), phylogenetic diversity (D), core abundance (E), and rare low abundance (F). Different letters above the bars indicate significant differences as determined by Dunn’s tests. CRC, conventionally raised control; CRD, conventionally raised Debaryomyces-exposed; CRP, conventionally raised Pseudozyma-exposed.
In contrast to the results of the CR conditions, Debaryomyces-exposed germ-free group (GFD) had significantly higher Shannon and Simpson diversities when compared to the control group (GFC, P < 0.01; Figures 5B,C). However, Shannon and Simpson diversities of the GFD and GFP groups were not significantly different (P > 0.05; Figures 5B,C). Exposure to yeasts had no significant effect on microbial alpha diversity measures (Figures 5A,D–F), the exception being the abovementioned results of Shannon and Simpson diversity.
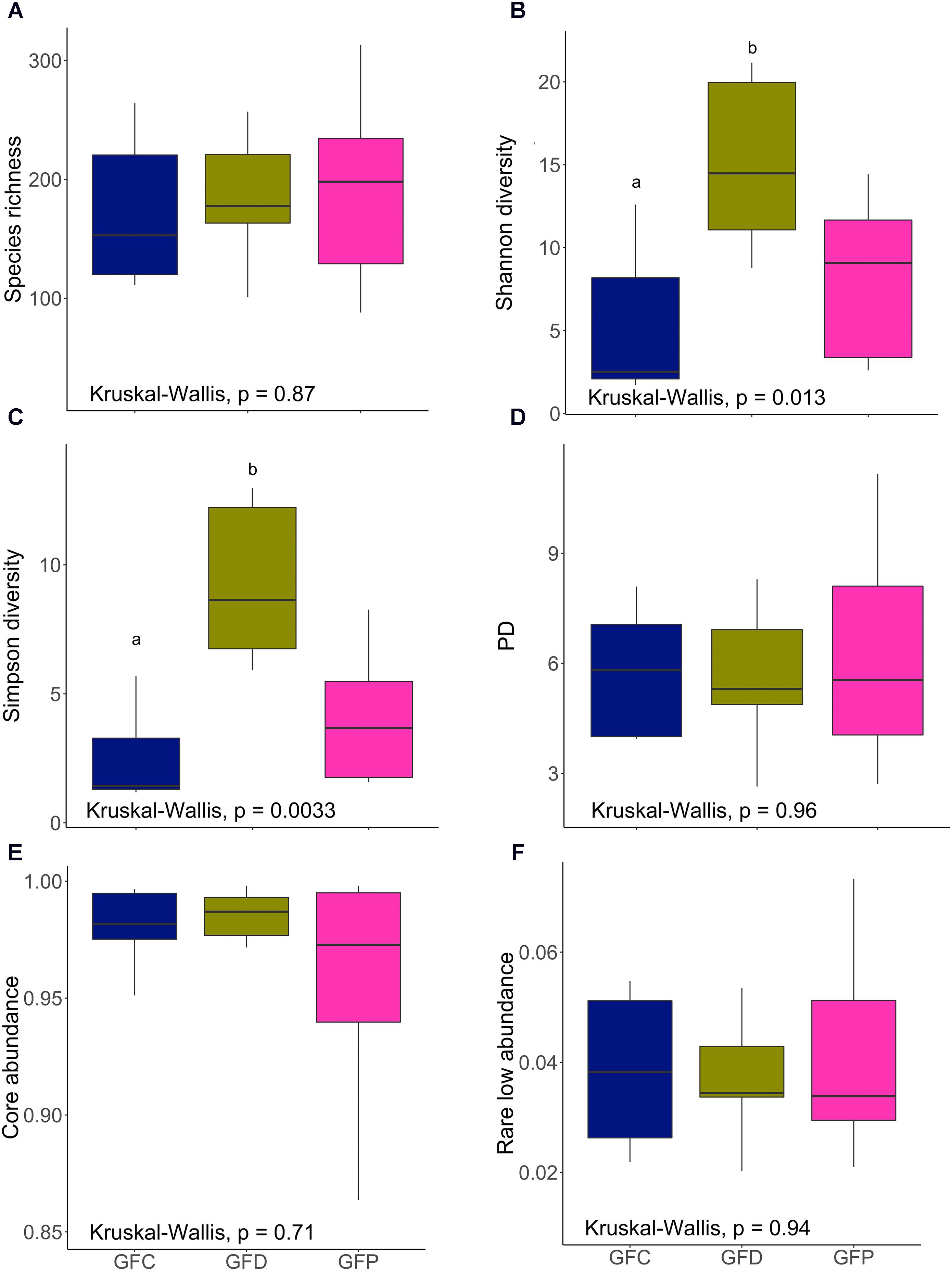
FIGURE 5. Alpha diversity indices of the intestinal bacterial communities of GF zebrafish larvae. Species richness (A), Shannon diversity (B), Simpson diversity (C), phylogenetic diversity (D), core abundance (E), and rare low abundance (F). Different letters above the bars indicate significant differences as determined by Dunn’s tests. GFC, Germ-free control; GFD, germ-free Debaryomyces-exposed; GFP, germ-free Pseudozyma-exposed.
Germ-Free and Conventionally Raised Zebrafish Larvae Not Exposed to Yeast Have Similar Intestinal Microbiota
The compositions of the microbiota in the control larvae reared in GF and CR conditions were almost identical (Figures 2A,B). PCoA also did not reveal any significant differences between GF and CR larvae (Supplementary Figure S4A, ANOSIM; R = 0.01, P > 0.05 and Adonis; R2 = 0.11). Similarly, we have not found any significant differences in the alpha diversity measures of the GF and CR larvae (Supplementary Figures S4B–F). Altogether, microbial composition and diversity of GF and CR larvae were similar.
Differentially Abundant Taxa Differ Between the Treatments
Linear discriminant analysis effect size analysis revealed that the genus Aeromonas was the overrepresented taxon in control zebrafish, regardless of the rearing conditions (Figures 6A,B). On the other hand, Pseudomonas was observed as the significantly abundant type in the yeast-exposed group, i.e., in the Pseudozyma-exposed larvae of the CR group (Figure 6A) and Debaryomyces-exposed larvae in the GF group (Figure 6B), respectively. We also observed that relatively few taxa were differentially abundant in Pseudozyma-exposed larvae (7 and 4 taxa in CR and GF, respectively) in comparison to the control (11 and 5 in CR and GF, respectively) and Debaryomyces-exposed larvae (10 and 15 in CR and GF, respectively). Zoogloe, Acidovorax, Rheinheimera were more abundant in the CRD and GFD groups. Rhizobium was overrepresented only in Pseudozyma-exposed groups of the CR and GF fish larvae. Aureispira, Pedobacter, Chitinophaga, Fluviicola from the second most dominant phylum Bacteroidetes were largely abundant in the CR groups rather than in the GF groups. Bacteria belonging to the phylum Firmicutes, particularly the low abundant Pediococcus and Lactococcus, were predominant in Debaryomyces-exposed CR group. In the GF group, Peptostreptococcus and Vagococcus were the abundant Firmicutes.
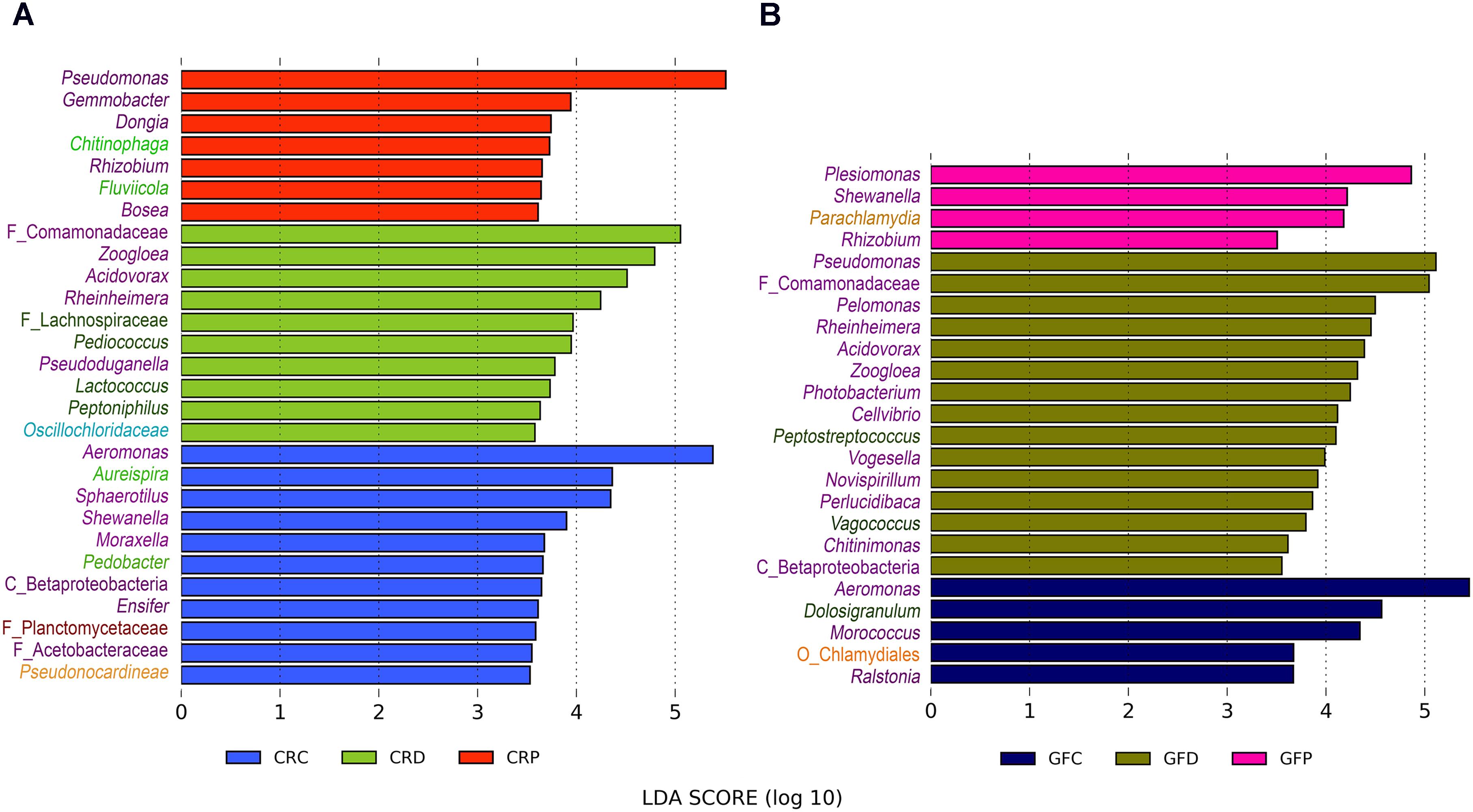
FIGURE 6. Differentially abundant bacterial taxa in CR (A) and GF (B) zebrafish larvae intestine. LEfSe was employed to find the differential abundance using a cut-off of 3.5 and a significance threshold of P < 0.05. Y-axis labels are color coded for different bacterial taxa: Proteobacteria – purple, Bacteroidetes – light green, Firmicutes – dark green, Chlamydiae – coral, Chloroflexi– cyan, Planctomycetes – dark red, and Actinobacteria – light orange. CRC, conventionally raised control; CRD, conventionally raised Debaryomyces-exposed; CRP, conventionally raised Pseudozyma-exposed; GFC, germ-free control; GFD, germ-free Debaryomyces-exposed; GFP, germ-free Pseudozyma-exposed.
Bacterial Communities of Water and Intestine of Zebrafish Larvae Differed
Proteobacteria, Bacteroidetes and Actinobacteria were the dominant groups in water samples from the two rearing conditions (CR and GF). These abundant phyla were also the dominant type in the samples from the water supply source of the zebrafish facility. They also contained other dominant phyla, namely Firmicutes, Fusobacteria, Nitrospirae, Planctomycetes, and Verrucomicrobia (Figure 7A). At a class level, we observed clear differences in microbial communities between water and intestinal samples (Supplementary Figure S5). Similarly, PCoA plot based on weighted UniFrac distance metrics indicated that these groups harbored distinct microbial populations (Figure 7B), despite the dominance of the phylum Proteobacteria in both the water and intestine samples.
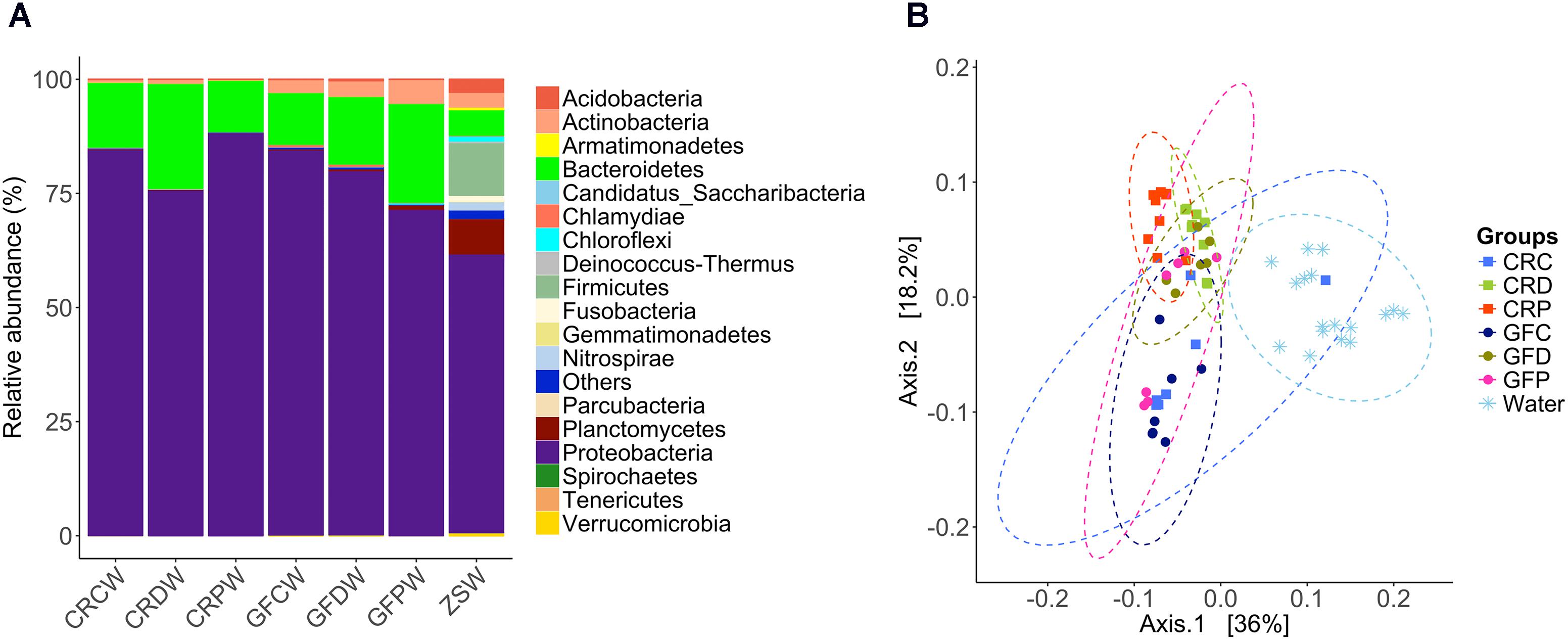
FIGURE 7. Relative abundance of bacterial phyla in the water samples (A) and principal coordinate analysis plot (B). The PCoA plot based on weighted UniFrac distance metric shows the distinction between the bacterial communities in water and zebrafish intestine. Ellipses include 95% of samples from normally distributed data. CRCW, CRDW, and CRPW represent the water samples collected from their respective flasks/tanks on day 2, 3, 7, and 14. GFCW, GFDW, and GFPW represent the water samples collected only on day 14. ZSW represents the water samples collected from the zebrafish facility on days 7 and 14.
Discussion
Early life-associated factors such as diet, environmental conditions, microbial interactions, and exposure to antibiotics or probiotics decide the assemblage and structure of the naïve microbial communities of the hosts (Scholtens et al., 2012; de Muinck et al., 2013). These microbes facilitate the host’s acclimatization to a new environment and subsequent succession of the associated intestinal microbial community. Several studies in most vertebrates, including fish have demonstrated that the selective manipulation through prebiotics and/or probiotics or functional diets modulates the gut microbial community by favoring the growth of beneficial microbes (Montalban-Arques et al., 2015; Ringø et al., 2016; Zorriehzahra et al., 2016). Probiotic administration is a promising approach to maintain the microbial balance, to prevent diseases, and to improve the health status of the host (Kiron, 2015). However, our current knowledge on the influence of yeasts on fish gut microbial composition is based on studies in a few species. We have determined the effect of fish-derived yeasts on the composition of bacteria in the intestine of zebrafish by sequencing the V4 region of bacterial 16S rRNA gene on an Illumina MiSeq platform. Our data demonstrated that early exposure to yeasts shapes the bacterial community and diversity in GF and CR zebrafish larvae and provide evidence that fish derived-yeasts can be used as biological tools to induce alterations in the gut microbial communities.
Proteobacteria Is the Dominant Phylum in Zebrafish Intestine
Bacterial communities in the intestine of zebrafish larvae comprised Proteobacteria (97.8%), Bacteroidetes (1.5%) and other rare phyla (Figure 2A); this profile is similar to other fish gut microbiota, including adult zebrafish (Roeselers et al., 2011; Llewellyn et al., 2014; Stephens et al., 2016). Our result is also consistent with previous reports that zebrafish larval gut harbors a highly constrained set of bacterial phyla (Davis et al., 2016; Phelps et al., 2017; Dahan et al., 2018). At the genus level, Aeromonas, Acidovorax, Pseudomonas, Rheinheimera, Shewanella, Sphaerotilus, Gemmobacter, and Zoogloea, and unidentified genera of the family Comamonadaceae were the dominant bacteria (Figure 2B). Among these, Aeromonas, Pseudomonas, Shewanella and few other rare genera (Staphylococcus, Photobacterium, Comamonas, Plesiomonas, Vibrio, and Cetobacterium) were commonly found and reported as the core gut microbiota of zebrafish (Roeselers et al., 2011; Cantas et al., 2012). The existence of these core communities in the intestine could reflect the significance and functional association of these bacteria with zebrafish. Although we did not find the abovementioned rare genera among the core taxa, this hidden backbone of microbial communities is known to enhance the functionality of abundant microbes (Jousset et al., 2017).
Yeast Exposure Alters the Relative Abundance of Aeromonas and Pseudomonas
Zebrafish larvae that were exposed to yeasts had significantly different microbial composition than their counterparts in the control groups (Figures 3A,B). Bacteroidetes decreased in the yeast-exposed groups compared to the control (Figure 2A). However, the effect of yeasts on the members of Proteobacteria seems to be selective; manifested by a trade-off between the relative abundance of two core genera, Aeromonas and Pseudomonas (Figure 2B). These bacterial genera are natural inhabitants of the aquatic environments and could persist in the fish gut as well (Cantas et al., 2012). Most bacteria belonging to these genera are opportunistic pathogens (Austin and Austin, 2007) but a few species are acknowledged as probiotic candidates in aquaculture (Irianto and Austin, 2002; Pieters et al., 2008; Van Hai and Fotedar, 2009). The intestinal microbiota of the control group was enriched with Aeromonas, whereas yeast-exposed larvae were enriched with Pseudomonas. Fish-derived yeasts or their cellular components seem to have antagonistic activity against the members of Aeromonas but favor the growth of Pseudomonas species. Yeasts and bacteria are common colonizers of gastrointestinal tract of animals, and their interaction, either symbiotic or antagonistic (Arvanitis and Mylonakis, 2015), has been shown to play a major role in the host biological activities (Iliev and Underhill, 2013). Furthermore, yeasts and their cell wall components including β-glucans, mannans and chitin may act as prebiotics or probiotics not only to facilitate the growth of beneficial bacteria but also to suppress the growth of other microbes via the production of various metabolites (Hatoum et al., 2012). Cider yeast-supplemented diet modulated the gut microbiota of pigs and reduced the counts of Lactobacilli (Upadrasta et al., 2013). Similarly, Gonçalves and Gallardo-Escárate (2017) showed that rainbow trout fed S. cerevisiae-supplemented diets had decreased abundance of Gammaproteobacteria of the phylum Proteobacteria and increased abundance of the phyla Firmicutes and Fusobacteria. A higher abundance of Pseudomonas in the yeast-exposed group also suggests that they outcompete Aeromonas. The antifungal properties of Pseudomonas against Candida spp. (fungi) have been reported; these bacteria can produce phenazines (Nishanth Kumar et al., 2014) and quorum-sensing molecules (Hogan et al., 2004). Furthermore, Pseudomonas has developed a mechanism to restrict Candida to its yeast form, so as to take advantage of the yeast fermentation products for its own growth and proliferation. Their complex interaction also involves the attachment of Pseudomonas to existing Candida hyphae to aid its dissemination (Chen et al., 2014). Conversely, Candida is able to down-regulate virulence factors of P. aeruginosa by modulating its quinolone signal (Cugini et al., 2007), which is part of the quorum-sensing system and controls multiple virulence factors (Calfee et al., 2001).
Yeast Exposure Impacts the Intestinal Bacterial Diversity
We have examined the effect of yeast exposure on the bacterial diversity in the intestine of zebrafish larvae. Regarding the CR larvae, Pseudozyma had a significant impact on both species richness and diversity indices (Figures 4A,B). However, such an effect was not observed for Debaryomyces. The lower diversity (richness, common and dominant species) of bacteria in the CRP group compared to CRC and CRD groups may be related to the predominance of Pseudomonas in CRP larvae (Figure 2B). The intestinal bacteria in the CRP group were phylogenetically more homogenous or closely related than those of the CRC and CRD groups (Figure 4D). We also observed a significant difference in the abundance of core community in CRP compared to CRC but not between CRP and CRD (Figure 4E). Again, this can be due to higher relative proportion of Pseudomonas, which is part of the zebrafish core community and could explain the significantly lower proportion of the low abundant species (Figure 4F). As for the germ-free larvae, the GFD group had significantly higher Shannon and Simpson diversity compared to the GFC group, suggesting that exposure to yeast can facilitate the establishment of diverse bacteria in the intestine of germ-free larvae. On the other hand, we did not observe any significant differences among other alpha diversity indices of the GF larvae. In CR zebrafish, the effect of yeast-exposure on the different diversity indices of intestinal bacteria contrasted with previous observations in rainbow trout (Waché et al., 2006; Gonçalves and Gallardo-Escárate, 2017). These authors reported that feeding rainbow trout fry and juveniles with diet containing S. cerevisiae increases their gut microbial diversity. Similarly, feeding dietary mannan-oligosaccharides to juvenile rainbow trout and gilthead seabream (Sparus aurata) increased the diversity of gut microbiota (Dimitroglou et al., 2010; Gonçalves and Gallardo-Escárate, 2017). Our results are in agreement with a previous report on the reduction of microbial diversity of zebrafish larvae after immersion exposure to probiotic Lactobacillus rhamnosus GG (Falcinelli et al., 2016). Interestingly, we have observed increased microbial diversity only in germ-free larvae exposed to Debaryomyces (GFD). Tapia-Paniagua et al. (2011) observed lower Shannon and Simpson diversity of the gut microbiota of S. aurata after the fish consumed diets incorporated with D. hansenii L2 for 4 weeks. The result of immersion versus feeding exposure to probiotics on gut microbiota depends on several factors, including the probiotic strain in question, dosage, viability and ability to cope with alimentary tract conditions in the host (Hai, 2015). In some cases, a similar colonization success is achieved through live feed and rearing water. However, three probiotic strains of Ruegeria, Pseudoalteromonas, and Vibrio were unable to colonize the gut of Atlantic cod (Gadus morhua) larvae either by immersion or feeding exposure (Skjermo et al., 2015). Nevertheless, the influence of yeast exposure methods on gut microbiota in fish warrants further investigation.
Microbial composition, diversity and abundance are positively affected by favorable conditions related to space, nutrients and competition (Hibbing et al., 2010). Debaryomyces-exposed CR and GF zebrafish larvae had relatively higher alpha diversity indices and we speculate that it might provide favorable intestinal conditions due to its inherent probiotic properties. For instance, juvenile leopard grouper (Mycteroperca rosacea) and gilthead seabream (S. aurata) offered live D. hansenii CBS8339 and strain L2, respectively, had enhanced immune responses and were resistant to pathogenic A. hydrophila (Reyes-Becerril et al., 2011, 2012). In addition, cell wall components of yeast can influence the host immune responses to regulate the diversity of gut microbiota (Charlet et al., 2018). The prebiotic/probiotic efficacy of a yeast is dependent on many factors including the type of species. In our study, larvae exposed to zebrafish-derived Pseudozyma had more abundance of Pseudomonas and lower microbial diversity compared to Debaryomyces, which was isolated from Atlantic salmon. Unlike Pseudozyma, Debaryomyces might have positive effect on host by increasing the microbial diversity. Thus, the species of yeast impacts the diversity of bacterial species which in turn influence the bacterial assemblage. Several studies have shown that the presence of diverse microbiota indicate a healthy ecosystem, which increases microbial functional stability and adaptive capacity (De Roy et al., 2013). However, its beneficial effects depend on the presence or enrichment of certain genera/species in the community rather than diversity per se. For example, it has been demonstrated that functional characteristics of a small number of bacterial species (low richness) have a positive impact on feed efficiency and energy requirements in ruminants (Shabat et al., 2016).
Exposure to Debaryomyces Favors Beneficial Bacteria
Linear discriminant analysis has indicated that taxa belonging to Proteobacteria were differentially abundant in all the groups (Figures 6A,B), irrespective of treatment. However, taxa from the Bacteroidetes were only enriched in CR zebrafish larvae (Figure 6A). Debaryomyces-exposed groups (GF and CR) were characterized by several significantly abundant bacterial taxa that included beneficial bacteria. Particularly, species from the low occurring phylum Firmicutes including Pediococcus and Lactococcus were enriched in CRD. Increasing abundance of LAB after yeast supplementation has also been reported in studies of juvenile European sturgeon (Hoseinifar et al., 2011) and Nile tilapia (Ran et al., 2015). Exposure to Debaryomyces may improve and maintain homeostasis in the gut ecosystem by modulating the abundance and diversity of bacteria, including those of LAB.
Variation in the Intestinal Bacterial Assemblage of Zebrafish Larvae Is Not Determined by Their Rearing Conditions
The CR larvae in control group were colonized with commensal bacteria as soon as they hatched from their protective chorions. On the other hand, GF larvae in the control group keep the GF conditions up to 7 dpf. Surprisingly, the microbial composition and diversity in the intestinal tract of both the GF and CR larvae were similar after they were reared in the system tanks (Supplementary Figure S4A). The intestinal bacterial composition of zebrafish larvae was distinct from that of the rearing water (Figure 7B). Similar results have been observed in other fish studies (Lokesh et al., 2018; Sevellec et al., 2018; Zhang et al., 2018). The intestinal microbiota of zebrafish larvae at 4 and 8 dpf had more abundance of Gammaproteobacteria, whereas the corresponding water samples had predominance of Betaproteobacteria (Stephens et al., 2016). We also observed more abundance of Gammaproteobacteria in the intestine of zebrafish larvae than their rearing water, which was mostly comprised of Betaproteobacteria (Supplementary Figure S5).
Our results suggest that exposure to yeast during the early developmental stage of zebrafish could influence the microbiota, and the differences in the established communities were evident after they were maintained in similar rearing conditions. In other words, variation in microbial composition is driven by specific yeast species, rather than the rearing condition of zebrafish larvae. Thus, our observations are in agreement with those of Yan et al. (2012) who proposed that bacterial community assembly and dynamics are shaped by both deterministic and stochastic factors.
Conclusion
The present study demonstrates that exposure to fish-derived yeast can alter the composition and diversity of the intestinal bacteria of zebrafish larvae. Noticeably, even a transient early-life exposure to yeast could induce remarkable alterations in the bacterial assemblage. If persistent, this early modulation of microbiota could influence host physiology later in life.
Author Contributions
PS, VK, JF, VM, and JG-V designed the study. PS and JG-V conducted the experiments. VM, JF, and VK contributed reagents and materials for the experiments. PS and JL performed the laboratory work. JL, PS, VK, and JF analyzed the data. PS, VK, and JF wrote the manuscript. All authors read, revised, and approved the manuscript.
Funding
PS was supported by ICAR International fellowship from the Indian Council of Agricultural Research, India. The study was part of the project “Bioteknologi – en framtidsrettet næring” (FR-274/16) from the Nordland County Council, Norway led by VK with additional support from the project “NorMur” (13011) awarded to JF and VK by Nord University and the Spanish Ministry of Science, Innovation and Universities: grants BIO2014-52655-R and BIO2017-84702-R to VM co-funded with Fondos Europeos de Desarrollo Regional (FEDER).
Conflict of Interest Statement
The authors declare that the research was conducted in the absence of any commercial or financial relationships that could be construed as a potential conflict of interest.
Acknowledgments
We thank Pedro J. Martínez and Inma Fuentes, University of Murcia, for their technical support while conducting the experiments. We are grateful to Ms. Bisa Saraswathy, Nord University, for her support in data analysis and for reviewing the manuscript.
Supplementary Material
The Supplementary Material for this article can be found online at: https://www.frontiersin.org/articles/10.3389/fmicb.2018.01868/full#supplementary-material
References
Andrews, S. (2010). FastQC: a Quality Control Tool for High Throughput Sequence Data. Available: http://www.bioinformatics.babraham.ac.uk/projects/fastqc/
Arvanitis, M., and Mylonakis, E. (2015). Fungal-bacterial interactions and their relevance in health. Cell. Microbiol. 17, 1442–1446. doi: 10.1111/cmi.12493
Austin, B., and Austin, D. A. (2007). Bacterial Fish Pathogens: Diseases of Farmed and Wild Fish. Dordrecht: Praxis Publishing Ltd.
Balcázar, J. L., De Blas, I., Ruiz-Zarzuela, I., Vendrell, D., Gironés, O., and Muzquiz, J. L. (2007). Enhancement of the immune response and protection induced by probiotic lactic acid bacteria against furunculosis in rainbow trout (Oncorhynchus mykiss). FEMS Immunol. Med. Microbiol. 51, 185–193. doi: 10.1111/j.1574-695X.2007.00294.x
Barko, P. C., Mcmichael, M. A., Swanson, K. S., and Williams, D. A. (2018). The gastrointestinal microbiome: a review. J. Vet. Intern. Med. 32, 9–25. doi: 10.1111/jvim.14875
Calfee, M. W., Coleman, J. P., and Pesci, E. C. (2001). Interference with Pseudomonas quinolone signal synthesis inhibits virulence factor expression by Pseudomonas aeruginosa. Proc. Natl. Acad. Sci. U.S.A. 98, 11633–11637. doi: 10.1073/pnas.201328498
Campana, R., Van Hemert, S., and Baffone, W. (2017). Strain-specific probiotic properties of lactic acid bacteria and their interference with human intestinal pathogens invasion. Gut. Pathog. 9:12. doi: 10.1186/s13099-017-0162-4
Cantas, L., Sorby, J. R., Alestrom, P., and Sorum, H. (2012). Culturable gut microbiota diversity in zebrafish. Zebrafish 9, 26–37. doi: 10.1089/zeb.2011.0712
Caporaso, J. G., Kuczynski, J., Stombaugh, J., Bittinger, K., Bushman, F. D., Costello, E. K., et al. (2010). QIIME allows analysis of high-throughput community sequencing data. Nat. Methods 7, 335–336. doi: 10.1038/nmeth.f.303
Charlet, R., Pruvost, Y., Tumba, G., Istel, F., Poulain, D., Kuchler, K., et al. (2018). Remodeling of the Candida glabrata cell wall in the gastrointestinal tract affects the gut microbiota and the immune response. Sci. Rep. 8:3316. doi: 10.1038/s41598-018-21422-w
Chen, A. I., Dolben, E. F., Okegbe, C., Harty, C. E., Golub, Y., Thao, S., et al. (2014). Candida albicans ethanol stimulates Pseudomonas aeruginosa WspR-controlled biofilm formation as part of a cyclic relationship involving phenazines. PLoS Pathog. 10:e1004480. doi: 10.1371/journal.ppat.1004480
Cugini, C., Calfee, M. W., Farrow, J. M., Morales, D. K., Pesci, E. C., and Hogan, D. A. (2007). Farnesol, a common sesquiterpene, inhibits PQS production in Pseudomonas aeruginosa. Mol. Microbiol. 65, 896–906. doi: 10.1111/j.1365-2958.2007.05840.x
Dahan, D., Jude, B. A., Lamendella, R., Keesing, F., and Perron, G. G. (2018). Exposure to arsenic alters the microbiome of larval zebrafish. Front. Microbiol. 9:1323. doi: 10.3389/fmicb.2018.01323
Davis, D. J., Bryda, E. C., Gillespie, C. H., and Ericsson, A. C. (2016). Microbial modulation of behavior and stress responses in zebrafish larvae. Behav. Brain Res. 311, 219–227. doi: 10.1016/j.bbr.2016.05.040
de Muinck, E. J., Stenseth, N. C., Sachse, D., Vander Roost, J., Rønningen, K. S., Rudi, K., et al. (2013). Context-dependent competition in a model gut bacterial community. PLoS One 8:e67210. doi: 10.1371/journal.pone.0067210
De Roy, K., Marzorati, M., Negroni, A., Thas, O., Balloi, A., Fava, F., et al. (2013). Environmental conditions and community evenness determine the outcome of biological invasion. Nat. Commun. 4:1383. doi: 10.1038/ncomms2392
Dimitroglou, A., Merrifield, D. L., Spring, P., Sweetman, J., Moate, R., and Davies, S. J. (2010). Effects of mannan oligosaccharide (MOS) supplementation on growth performance, feed utilisation, intestinal histology and gut microbiota of gilthead sea bream (Sparus aurata). Aquaculture 300, 182–188. doi: 10.1016/j.aquaculture.2010.01.015
Edgar, R. (2016). SINTAX: a simple non-Bayesian taxonomy classifier for 16S and ITS sequences. bioRxiv [Preprint]. doi: 10.1101/074161
Edgar, R. C. (2013). UPARSE: highly accurate OTU sequences from microbial amplicon reads. Nat. Methods 10, 996–998. doi: 10.1038/nmeth.2604
Edgar, R. C., and Flyvbjerg, H. (2015). Error filtering, pair assembly and error correction for next-generation sequencing reads. Bioinformatics 31, 3476–3482. doi: 10.1093/bioinformatics/btv401
Edgar, R. C., Haas, B. J., Clemente, J. C., Quince, C., and Knight, R. (2011). UCHIME improves sensitivity and speed of chimera detection. Bioinformatics 27, 2194–2200. doi: 10.1093/bioinformatics/btr381
Faith, D. P., and Baker, A. M. (2006). Phylogenetic diversity (PD) and biodiversity conservation: some bioinformatics challenges. Evol. Bioinform. Online. 2, 121–128. doi: 10.1177/117693430600200007
Faith, J. J., Guruge, J. L., Charbonneau, M., Subramanian, S., Seedorf, H., Goodman, A. L., et al. (2013). The long-term stability of the human gut microbiota. Science 341, 1237439. doi: 10.1126/science.1237439
Falcinelli, S., Rodiles, A., Unniappan, S., Picchietti, S., Gioacchini, G., Merrifield, D. L., et al. (2016). Probiotic treatment reduces appetite and glucose level in the zebrafish model. Sci. Rep. 6:18061. doi: 10.1038/srep18061
Galindo-Villegas, J., García-Moreno, D., De Oliveira, S., Meseguer, J., and Mulero, V. (2012). Regulation of immunity and disease resistance by commensal microbes and chromatin modifications during zebrafish development. PNAS 109, E2605–E2614. doi: 10.1073/pnas.1209920109
Gatesoupe, F. J. (1999). The use of probiotics in aquaculture. Aquaculture 180, 147–165. doi: 10.1016/S0044-8486(99)00187-8
Gonçalves, A. T., and Gallardo-Escárate, C. (2017). Microbiome dynamic modulation through functional diets based on pre- and probiotics (mannan-oligosaccharides and Saccharomyces cerevisiae) in juvenile rainbow trout (Oncorhynchus mykiss). J. Appl. Microbiol. 122, 1333–1347. doi: 10.1111/jam.13437
Hai, N. V. (2015). The use of probiotics in aquaculture. J. Appl. Microbiol. 119, 917–935. doi: 10.1111/jam.12886
Hansen, G. H., and Olafsen, J. A. (1999). Bacterial interactions in early life stages of marine cold water fish. Microb. Ecol. 38, 1–26. doi: 10.1007/s002489900158
Hatoum, R., Labrie, S., and Fliss, I. (2012). Antimicrobial and probiotic properties of yeasts: from fundamental to novel applications. Front. Microbiol. 3:421. doi: 10.3389/fmicb.2012.00421
Hibbing, M. E., Fuqua, C., Parsek, M. R., and Peterson, S. B. (2010). Bacterial competition: surviving and thriving in the microbial jungle. Nat. Rev. Microbiol. 8, 15–25. doi: 10.1038/nrmicro2259
Hogan, D. A., Vik, A., and Kolter, R. (2004). A Pseudomonas aeruginosa quorum-sensing molecule influences Candida albicans morphology. Mol. Microbiol. 54, 1212–1223. doi: 10.1111/j.1365-2958.2004.04349.x
Hooper, L. V., and Gordon, J. I. (2001). Commensal host-bacterial relationships in the gut. Science 292, 1115–1118. doi: 10.1126/science.1058709
Hoseinifar, S. H., Mirvaghefi, A., and Merrifield, D. L. (2011). The effects of dietary inactive brewer’s yeast Saccharomyces cerevisiae var. ellipsoideus on the growth, physiological responses and gut microbiota of juvenile beluga (Huso huso). Aquaculture 318, 90–94. doi: 10.1016/j.aquaculture.2011.04.043
Houghteling, P. D., and Walker, W. A. (2015). Why is initial bacterial colonization of the intestine important to infants’ and children’s health? J. Pediatr. Gastroenterol. Nutr. 60, 294–307. doi: 10.1097/mpg.0000000000000597
Hsieh, T. C., Ma, K. H., and Chao, A. (2016). iNEXT: an R package for rarefaction and extrapolation of species diversity (Hill numbers). Methods Ecol. Evol. 7, 1451–1456. doi: 10.1111/2041-210X.12613
Iliev, I. D., and Underhill, D. M. (2013). Striking a balance: fungal commensalism versus pathogenesis. Curr. Opin. Microbiol. 16, 366–373. doi: 10.1016/j.mib.2013.05.004
Irianto, A., and Austin, B. (2002). Use of probiotics to control furunculosis in rainbow trout, Oncorhynchus mykiss (Walbaum). J. Fish Dis. 25, 333–342. doi: 10.1046/j.1365-2761.2002.00375.x
Jousset, A., Bienhold, C., Chatzinotas, A., Gallien, L., Gobet, A., Kurm, V., et al. (2017). Where less may be more: how the rare biosphere pulls ecosystems strings. ISME J. 11, 853–862. doi: 10.1038/ismej.2016.174
Kiron, V. (2015). “Gastrointestinal microorganisms of fish and probiotics,” in Dietary Nutrients, Additives, and Fish Health, eds L. Cheng-Sheng Lee, Chhorn lim, M. Delbert, I. I. I. Gatlin, and C. D. Webster (Hoboken, NJ: Wiley-Blackwell), 283–304. doi: 10.1002/9781119005568.ch14
Kozich, J. J., Westcott, S. L., Baxter, N. T., Highlander, S. K., and Schloss, P. D. (2013). Development of a dual-index sequencing strategy and curation pipeline for analyzing amplicon sequence data on the MiSeq Illumina sequencing platform. Appl. Environ. Microbiol. 79, 5112–5120. doi: 10.1128/AEM.01043-13
Lahti, L., Shetty, S., Blake, T., and Salojarvi, J. (2017). Tools for Microbiome Analysis in R. Version 1.1.2. Available at: http://microbiome.github.com/microbiome
Ley, R. E., Hamady, M., Lozupone, C., Turnbaugh, P. J., Ramey, R. R., Bircher, J. S., et al. (2008). Evolution of mammals and their gut microbes. Science 320, 1647–1651. doi: 10.1126/science.1155725
Llewellyn, M. S., Boutin, S., Hoseinifar, S. H., and Derome, N. (2014). Teleost microbiomes: the state of the art in their characterization, manipulation and importance in aquaculture and fisheries. Front. Microbiol. 5:207. doi: 10.3389/fmicb.2014.00207
Lokesh, J., Kiron, V., Sipkema, D., Fernandes, J. M. O., and Moum, T. (2018). Succession of embryonic and the intestinal bacterial communities of Atlantic salmon (Salmo salar) reveals stage-specific microbial signatures. Microbiologyopen doi: 10.1002/mbo3.672 [Epub ahead of print].
Lozupone, C., and Knight, R. (2005). UniFrac: a new phylogenetic method for comparing microbial communities. Appl. Environ. Microbiol. 71, 8228–8235. doi: 10.1128/aem.71.12.8228-8235.2005
Martínez Cruz, P., Ibáñez, A. L., Monroy Hermosillo, O. A., and Ramírez Saad, H. C. (2012). Use of probiotics in aquaculture. ISRN Microbiol. 2012:916845. doi: 10.5402/2012/916845
McMurdie, P. J., and Holmes, S. (2013). phyloseq: an R package for reproducible interactive analysis and graphics of microbiome census data. PLoS One 8:e61217. doi: 10.1371/journal.pone.0061217
Montalban-Arques, A., De Schryver, P., Bossier, P., Gorkiewicz, G., Mulero, V., Gatlin, D. M., et al. (2015). Selective manipulation of the gut microbiota improves immune status in vertebrates. Front. Immunol. 6:512. doi: 10.3389/fimmu.2015.00512
Navarrete, P., and Tovar-Ramírez, D. (2014). “Use of yeasts as probiotics in fish aquaculture,” in Sustainable Aquaculture Techniques, ed. M. H. Vergara (London: InTechOpen), 135–172. doi: 10.5772/57196
Nishanth Kumar, S., Nisha, G. V., Sudaresan, A., Venugopal, V. V., Sree Kumar, M. M., Lankalapalli, R. S., et al. (2014). Synergistic activity of phenazines isolated from Pseudomonas aeruginosa in combination with azoles against Candida species. Med. Mycol. 52, 482–490. doi: 10.1093/mmy/myu012
Oksanen, J., Blanchet, F., Kindt, R., Legendre, P., Minchin, P., O’hara, R., et al. (2018). Community Ecology Package “Vegan”. R Package Version 2.4–6.
O’Toole, P. W., and Cooney, J. C. (2008). Probiotic bacteria influence the composition and function of the intestinal microbiota. Interdiscip. Perspect. Infect. Dis. 2008:175285. doi: 10.1155/2008/175285
Phelps, D., Brinkman, N. E., Keely, S. P., Anneken, E. M., Catron, T. R., Betancourt, D., et al. (2017). Microbial colonization is required for normal neurobehavioral development in zebrafish. Sci. Rep. 7:11244. doi: 10.1038/s41598-017-10517-5
Pieters, N., Brunt, J., Austin, B., and Lyndon, A. R. (2008). Efficacy of in-feed probiotics against Aeromonas bestiarum and Ichthyophthirius multifiliis skin infections in rainbow trout (Oncorhynchus mykiss, Walbaum). J. Appl. Microbiol. 105, 723–732. doi: 10.1111/j.1365-2672.2008.03817.x
R Development Core Team (2018). R: A Language and Environment for Statistical Computing. Vienna: R Foundation for Statistical Computing.
Raggi, P., Lopez, P., Diaz, A., Carrasco, D., Silva, A., Velez, A., et al. (2014). Debaryomyces hansenii and Rhodotorula mucilaginosa comprised the yeast core gut microbiota of wild and reared carnivorous salmonids, croaker and yellowtail. Environ. Microbiol. 16, 2791–2803. doi: 10.1111/1462-2920.12397
Ran, C., Huang, L., Liu, Z., Xu, L., Yang, Y., Tacon, P., et al. (2015). A comparison of the beneficial effects of live and heat-inactivated baker’s yeast on Nile tilapia: suggestions on the role and function of the secretory metabolites released from the yeast. PLoS One 10:e0145448. doi: 10.1371/journal.pone.0145448
Reitan, K. I., Natvik, C. M., and Vadstein, O. (1998). Drinking rate, uptake of bacteria and microalgae in turbot larvae. J. Fish Biol. 53, 1145–1154. doi: 10.1111/j.1095-8649.1998.tb00238.x
Reyes-Becerril, M., Tovar-Ramirez, D., Ascencio-Valle, F., Civera-Cerecedo, R., Gracia-Lopez, V., and Barbosa-Solomieu, V. (2011). Effects of dietary supplementation with probiotic live yeast Debaryomyces hansenii on the immune and antioxidant systems of leopard grouper Mycteroperca rosacea infected with Aeromonas hydrophila. Aquacult. Res. 42, 1676–1686. doi: 10.1111/j.1365-2109.2010.02762.x
Reyes-Becerril, M., Ascencio-Valle, F., José, M., Tapia-Paniagua, S., and Morinigo, M. A. (2012). Debaryomyces hansenii L2-enriched diet enhances the immunity status, gene expression and intestine functionality in gilthead seabream (Sparus aurata L.). Aquacult. Res. 43, 1107–1118. doi: 10.1111/j.1365-2109.2011.02914.x
Ringø, E., and Gatesoupe, F.-J. (1998). Lactic acid bacteria in fish: a review. Aquaculture 160, 177–203. doi: 10.1016/S0044-8486(97)00299-8
Ringø, E., Zhou, Z., Vecino, J. L. G., Wadsworth, S., Romero, J., Krogdahl, Å, et al. (2016). Effect of dietary components on the gut microbiota of aquatic animals. A never-ending story? Aquacult. Nutr. 22, 219–282. doi: 10.1111/anu.12346
Rodríguez, J. M., Murphy, K., Stanton, C., Ross, R. P., Kober, O. I., Juge, N., et al. (2015). The composition of the gut microbiota throughout life, with an emphasis on early life. Microb. Ecol. Health Dis. 26:26050. doi: 10.3402/mehd.v26.26050
Roeselers, G., Mittge, E. K., Stephens, W. Z., Parichy, D. M., Cavanaugh, C. M., Guillemin, K., et al. (2011). Evidence for a core gut microbiota in the zebrafish. ISME J. 5, 1595–1608. doi: 10.1038/ismej.2011.38
Romero, J., Ringø, E., and Merrifield, D. L. (2014). “The gut microbiota of fish,” in Aquaculture Nutrition:Gut Health, Probiotics and Prebiotics, eds D. Merrifield and E. Ringø (John Wiley & Sons), 75–100.
Scholtens, P. A., Oozeer, R., Martin, R., Amor, K. B., and Knol, J. (2012). The early settlers: intestinal microbiology in early life. Annu Rev Food Sci Technol. 3, 425–447. doi: 10.1146/annurev-food-022811-101120
Segata, N., Izard, J., Waldron, L., Gevers, D., Miropolsky, L., Garrett, W. S., et al. (2011). Metagenomic biomarker discovery and explanation. Genome Biol. 12:R60. doi: 10.1186/gb-2011-12-6-r60
Sevellec, M., Derome, N., and Bernatchez, L. (2018). Holobionts and ecological speciation: the intestinal microbiota of lake whitefish species pairs. Microbiome 6:47. doi: 10.1186/s40168-018-0427-2
Shabat, S. K., Sasson, G., Doron-Faigenboim, A., Durman, T., Yaacoby, S., Berg Miller, M. E., et al. (2016). Specific microbiome-dependent mechanisms underlie the energy harvest efficiency of ruminants. ISME J. 10, 2958–2972. doi: 10.1038/ismej.2016.62
Siriyappagouder, P., Kiron, V., Lokesh, J., Rajeish, M., Kopp, M., and Fernandes, J. (2018). The intestinal mycobiota in wild zebrafish comprises mainly Dothideomycetes while Saccharomycetes predominate in their laboratory-reared counterparts. Front. Microbiol. 9:387. doi: 10.3389/fmicb.2018.00387
Skjermo, J., Bakke, I., Dahle, S. W., and Vadstein, O. (2015). Probiotic strains introduced through live feed and rearing water have low colonizing success in developing Atlantic cod larvae. Aquaculture 438, 17–23. doi: 10.1016/j.aquaculture.2014.12.027
Stephens, W. Z., Burns, A. R., Stagaman, K., Wong, S., Rawls, J. F., Guillemin, K., et al. (2016). The composition of the zebrafish intestinal microbial community varies across development. ISME J. 10, 644–654. doi: 10.1038/ismej.2015.140
Tanaka, M., and Nakayama, J. (2017). Development of the gut microbiota in infancy and its impact on health in later life. Allergol. Intern. 66, 515–522. doi: 10.1016/j.alit.2017.07.010
Tapia-Paniagua, S., Reyes-Becerril, M., Ascencio-Valle, F., Esteban, M., Clavijo, E., Balebona, M., et al. (2011). Modulation of the intestinal microbiota and immune system of farmed Sparus aurata by the administration of the yeast Debaryomyces hansenii L2 in conjunction with inulin. J Aquac. Res. Dev. 1:012. doi: 10.4172/2155-9546.S1-012
Tovar-Ramírez, D., Mazurais, D., Gatesoupe, J. F., Quazuguel, P., Cahu, C. L., and Zambonino-Infante, J. L. (2010). Dietary probiotic live yeast modulates antioxidant enzyme activities and gene expression of sea bass (Dicentrarchus labrax) larvae. Aquaculture 300, 142–147. doi: 10.1016/j.aquaculture.2009.12.015
Tovar-Ramírez, D., Zambonino Infante, J., Cahu, C., Gatesoupe, F. J., and Vázquez-Juárez, R. (2004). Influence of dietary live yeast on European sea bass (Dicentrarchus labrax) larval development. Aquaculture 234, 415–427. doi: 10.1016/j.aquaculture.2004.01.028
Upadrasta, A., O’sullivan, L., O’sullivan, O., Sexton, N., Lawlor, P. G., Hill, C., et al. (2013). The effect of dietary supplementation with spent cider yeast on the swine distal gut microbiome. PLoS One 8:e75714. doi: 10.1371/journal.pone.0075714
Vadstein, O., Bergh,Ø, Gatesoupe, F. J., Galindo-Villegas, J., Mulero, V., Picchietti, S., et al. (2013). Microbiology and immunology of fish larvae. Rev. Aquacult. 5, S1–S25. doi: 10.1111/j.1753-5131.2012.01082.x
Van Hai, N., and Fotedar, R. (2009). Comparison of the effects of the prebiotics (Bio-Mos® and β-1,3-D-glucan) and the customised probiotics (Pseudomonas synxantha and P. aeruginosa) on the culture of juvenile western king prawns (Penaeus latisulcatus Kishinouye, 1896). Aquaculture 289, 310–316. doi: 10.1016/j.aquaculture.2009.02.001
Verschuere, L., Rombaut, G., Sorgeloos, P., and Verstraete, W. (2000). Probiotic bacteria as biological control agents in aquaculture. Microbiol. Mol. Biol. Rev. 64, 655–671. doi: 10.1128/MMBR.64.4.655-671.2000
Vohra, A., Syal, P., and Madan, A. (2016). Probiotic yeasts in livestock sector. Anim. Feed Sci. Tech. 219, 31–47. doi: 10.1016/j.anifeedsci.2016.05.019
Waché, Y., Auffray, F., Gatesoupe, F.-J., Zambonino, J., Gayet, V., Labbé, L., et al. (2006). Cross effects of the strain of dietary Saccharomyces cerevisiae and rearing conditions on the onset of intestinal microbiota and digestive enzymes in rainbow trout, Onchorhynchus mykiss, fry. Aquaculture 258, 470–478. doi: 10.1016/j.aquaculture.2006.04.002
Westerfield, M. (2007). The Zebrafish Book. A Guide for the Laboratory Use of Zebrafish (Danio Rerio), 5th Edn. Eugene, OR: The University of Oregon.
Wickham, H. (2010). Ggplot2: elegant graphics for data analysis. J. Stat. Softw. 35, 65–88. doi: 10.18637/jss.v077.b02
Yan, Q., Van Der Gast, C. J., and Yu, Y. (2012). Bacterial community assembly and turnover within the intestines of developing zebrafish. PLoS One 7:e30603. doi: 10.1371/journal.pone.0030603
Zhang, Z., Li, D., Refaey, M. M., Xu, W., Tang, R., and Li, L. (2018). Host age affects the development of southern catfish gut bacterial community divergent from that in the food and rearing water. Front. Microbiol. 9:495. doi: 10.3389/fmicb.2018.00495
Keywords: yeast, microbiota, zebrafish, germ-free, 16S rRNA, amplicon sequencing, Debaryomyces, Pseudozyma
Citation: Siriyappagouder P, Galindo-Villegas J, Lokesh J, Mulero V, Fernandes JMO and Kiron V (2018) Exposure to Yeast Shapes the Intestinal Bacterial Community Assembly in Zebrafish Larvae. Front. Microbiol. 9:1868. doi: 10.3389/fmicb.2018.01868
Received: 18 May 2018; Accepted: 25 July 2018;
Published: 14 August 2018.
Edited by:
Jaime Romero, Universidad de Chile, ChileReviewed by:
Sylvia Brugman, Wageningen University & Research, NetherlandsMarie Filteau, Laval University, Canada
Copyright © 2018 Siriyappagouder, Galindo-Villegas, Lokesh, Mulero, Fernandes and Kiron. This is an open-access article distributed under the terms of the Creative Commons Attribution License (CC BY). The use, distribution or reproduction in other forums is permitted, provided the original author(s) and the copyright owner(s) are credited and that the original publication in this journal is cited, in accordance with accepted academic practice. No use, distribution or reproduction is permitted which does not comply with these terms.
*Correspondence: Jorge M. O. Fernandes, am9yZ2UubS5mZXJuYW5kZXNAbm9yZC5ubw== Viswanath Kiron, a2lyb24udmlzd2FuYXRoQG5vcmQubm8=