- 1Hubei Collaborative Innovation Center for Grain Industry, School of Agriculture, Yangtze University, Jingzhou, China
- 2College of Horticulture and Gardening, Yangtze University, Jingzhou, China
- 3Matthias-Schleiden-Institute, Plant Physiology, Friedrich-Schiller-University Jena, Jena, Germany
Phytohormones play vital roles in the growth and development of plants as well as in interactions of plants with microbes such as endophytic fungi. The endophytic root-colonizing fungus Piriformospora indica promotes plant growth and performance, increases resistance of colonized plants to pathogens, insects and abiotic stress. Here, we discuss the roles of the phytohormones (auxins, cytokinin, gibberellins, abscisic acid, ethylene, salicylic acid, jasmonates, and brassinosteroids) in the interaction of P. indica with higher plant species, and compare available data with those from other (beneficial) microorganisms interacting with roots. Crosstalks between different hormones in balancing the plant responses to microbial signals is an emerging topic in current research. Furthermore, phytohormones play crucial roles in systemic signal propagation as well as interplant communication. P. indica interferes with plant hormone synthesis and signaling to stimulate growth, flowering time, differentiation and local and systemic immune responses. Plants adjust their hormone levels in the roots in response to the microbes to control colonization and fungal propagation. The available information on the roles of phytohormones in beneficial root–microbe interactions opens new questions of how P. indica manipulates the plant hormone metabolism to promote the benefits for both partners in the symbiosis.
Introduction
Phytohormones, such as auxins (e.g., IAA, the most common auxin), CK, GAs, ABA, ET, SA, and jasmonates play important roles in growth and development of plants as well as in plant resistance/tolerance to abiotic stresses and in plant interactions with various mutualistic fungi (Cakmak and Marschner, 1992; Sirrenberg et al., 2007; Vadassery et al., 2008; Schäfer et al., 2009a,b; Camehl et al., 2010; Lee et al., 2011; Gutjahr and Parniske, 2013; Johnson et al., 2013; Miransari et al., 2014; Zhang Y. et al., 2015; Kopittke, 2016; Naser and Shani, 2016; Tian et al., 2017; Inahashi et al., 2018; Mathur et al., 2018). Endo- and ectomycorrhizal fungi, N2-fixing rhizobacteria and a large number of fungal and bacterial endophytes colonize the roots and confer benefits to the roots in addition to its effects to the leaves. The spectrum of benefits for their host plants includes growth promotion, higher yields, and enhanced resistance/tolerance to several biotic (e.g., pathogens, pests, or nematodes) and abiotic (e.g., drought, salinity and low temperature) stresses. On the other hand, defense-related phytohormones control roots colonization which is essential for stabilizing a symbiotic interaction. Piriformospora indica (family Sebacinaceae, order Sebacinales) is a well-known endophytic root-colonizing fungus which was originally discovered in association with xerophytic plants of the Thar Desert in India (Verma et al., 1998). Similar to arbuscular mycorrhizal fungi, P. indica promotes growth of the host plants and enhances resistance to microbial pathogens/insects and tolerance to abiotic stress (Harman, 2011). In the past decade, more information has become available regarding the roles of phytohormones in fungus–plant interactions. Growth and yield benefits are largely attributed to the production of the phytohormones IAA, CK, and GA, whereas enhanced stress tolerance is often explained by a systemic resistance response involving ABA, ET, SA, and jasmonates, and their interactions with P. indica have been reported recently (Varma et al., 2012; Gill et al., 2016). However, none of these hormones can be considered individually, since there is an enormous crosstalk between them. The crosstalk between JA, SA, (and ET) is long known (cf. Caarls et al., 2015; Yang et al., 2015; Zhang P.J. et al., 2015); only recently, it became clear that two ABA-responsive plastid lipases are involved in JA biosynthesis (Avramova, 2017; Wang et al., 2018). Many other processes in symbiotic interactions of roots with microbes require intensive crosstalk between phytohormones (e.g., Pozo et al., 2015; Gamas et al., 2017; Hu et al., 2017; Liu et al., 2017; Tognetti et al., 2017; Shen et al., 2018, to mention a few). Here, we discuss the importance of phytohormones and their crosstalks in the interaction of P. indica with a wide range of higher plants. Based on studies of beneficial root symbioses and pathosystems, we highlight open questions which can be experimentally addressed with an endophyte like P. indica during its interaction with model plants and agriculturally important crops.
Auxin
Auxins (such as IAA, the most abundant auxin in nature) coordinate many key processes in plant development and adaptive growth (Naser and Shani, 2016), and therefore, it is not surprising that root-colonizing microbes including P. indica interfere with the plant auxin metabolism or signaling for stimulating growth and developmental processes in their hosts. Besides the involvement of auxin in cell division, cell enlargement and organ development, it also participates in defense and stress responses (Kopittke, 2016; Egamberdieva et al., 2017), which makes this hormone an interesting target for root-interacting microbes. How P. indica targets auxin to the responsive cells in the roots, and how it establishes new and/or controls auxin maxima have not yet been investigated. It is long known that many root-colonizing beneficial and pathogenic bacteria produce IAA (e.g., Agrobacterium tumefaciens, A. rhizogenes, Pseudomonas syringae and other sp., Erwinia herbicola, species of Azospirillum, Azotobacter, Rhizobium, Bacillus, and Enterobacter (Glick, 1995; Patten and Glick, 2002; Ouzari et al., 2008; Abd El-Hadi Nadia et al., 2009; Khan and Doty, 2009; Ahmed and Hasnain, 2010; Merzaeva and Shirokikh, 2010; Roy et al., 2010; Fu and Wang, 2011; Patil, 2011; Celloto et al., 2012). Also root-colonizing beneficial and pathogenic fungi produce auxins (Reineke et al., 2008; Felten et al., 2009, 2010; Splivallo et al., 2009; Fu et al., 2011; Robert-Seilaniantz et al., 2011; Sukumar et al., 2013; Fusconi, 2014; Gutjahr, 2014; Raudaskoski and Kothe, 2015). For instance, the IAA producing Metarhizium robertsii promotes lateral root growth and root hair development in Arabidopsis and auxin is involved in enhancing virulence to insects (Liao et al., 2017). M. robertsii-colonized plants withstand attacks by herbivores in part by emitting volatiles that attract insect predators and bolster resistance to future threats. IAA is particularly important in regulating plant defensive responses and it is likely to accumulate at the site of insect feeding (Peck and Kende, 1998; Howe and Jander, 2008), which could make it a useful host-related signal triggering infection-related processes in M. robertsii. However, the exact mechanisms remain to be confirmed. Also auxin transport can be affected by beneficial microbes (Felten et al., 2009; Ng et al., 2015). The hormone may quantitatively influence AM colonization and perform an additional cell-autonomous function in the promotion of arbuscule development (Gutjahr, 2014). Several reports demonstrate that P. indica interferes with auxin production and signaling in the hosts and is responsible for, or at least contribute to, root growth (Sirrenberg et al., 2007; Vadassery et al., 2008; Lee et al., 2011; Hilbert et al., 2012, 2013; Dong et al., 2013; Ye et al., 2014; Kao et al., 2016; Hua et al., 2017). Sirrenberg et al. (2007) first demonstrated that P. indica produced IAA in liquid culture and that fungal auxin production affects root growth. The auxin levels vary substantially in different plant species colonized by P. indica, which has been measured either directly or deduced from the expression of auxin-responsive genes. For example, large-scale microarray analysis of P. indica-colonized Arabidopsis roots showed that auxin-related gene expression and the auxin content remained unchanged in the entire root in response to P. indica (Vadassery et al., 2008). Lee et al. (2011) showed that growth promotion of Chinese cabbage (Brassica campestris) and Arabidopsis by P. indica is not stimulated by mycelium-synthesized auxin. Also exogenous application of auxins to Arabidopsis seedlings could not mimic the growth promotion effect induced by the fungus. However, P. indica rescued the dwarf phenotype of the Arabidopsis auxin overproducer sur1-1 by converting free auxin into conjugates, resulting in down-regulation of the auxin-induced IAA6 gene (Vadassery et al., 2008). Apparently, high concentrations of active auxin, which inhibit growth in the roots and aerial parts of plants, are negated by the presence of P. indica. Additionally, Arabidopsis mutants with reduced auxin levels exhibit a normal colonization response to P. indica, suggesting that P. indica-promoted Arabidopsis growth results from alterations in auxin homeostasis (Vadassery et al., 2008). Promotion of growth and development in P. indica-colonized Chinese cabbage and barley seedlings have been attributed to increased levels of auxin in roots, while the auxin level in leaves remained unaffected (Lee et al., 2011; Hilbert et al., 2012). Auxin transport-related and signaling proteins such as AUX1 and PINs in Chinese cabbage were up-regulated at the mRNA level by P. indica inoculation, suggesting a positive role of the fungus on the plant auxin metabolism (Lee et al., 2011). These analyses are based on hormone levels in entire organs (roots or shoots), and local auxin maxima which are required for root growth in the maturation zone or for the initiation of lateral root formation were not considered in these studies.
In Chinese cabbage roots, P. indica mainly stimulated growth promotion in the maturation zone and this involves the up-regulation of auxin-responsive genes (Dong et al., 2013). By using high-throughput gas-chromatography-based mass spectrometry, Hua et al. (2017) demonstrated that auxin and its intermediates were induced and de novo synthesized in colonized Chinese cabbage roots. Ye et al. (2014) performed a genome-wide expression profiling of small RNAs in Oncidium orchid roots either colonized or not-colonized by P. indica. The predicted target genes of these miRNAs are mainly involved in auxin signal perception and transduction, transcription, development and plant defense. The mRNA level for the auxin receptor protein TIR1 showed a gradual downregulation within the first 8 weeks after P. indica inoculation of Oncidium orchid roots, while the mRNAs for auxin response factors (ARFs) were up-regulated during the first week followed by down-regulation during later phases of the symbiosis (Ye et al., 2014). Although these results clearly demonstrate the involvement of auxin in the symbiotic interaction of various plant species with P. indica, the source of the phytohormone and how it is generated and active remained unclear, until Hilbert et al. (2012) showed that indole derivative production by P. indica is not required for growth promotion but for biotrophic colonization of barley roots. They showed that P. indica produce IAA, but also indole-3-lactate (ILA) which is synthesized through the intermediate indole-3-pyruvic acid (IPA). Time course transcriptional analyses after exposure to tryptophan designated the P. indica piTam1 gene as a key player. A green fluorescence protein reporter study and transcriptional analysis of colonized barley roots showed that piTam1 is induced during the biotrophic phase. P. indica strains in which the piTam1 gene was silenced via an RNA interference approach were compromised in IAA and ILA production and displayed reduced colonization of barley roots in the biotrophic phase, but the elicitation of growth promotion was not affected compared with the wild-type situation. Their results suggest that IAA is involved in the establishment of biotrophy in P. indica-barley symbiosis and might represent a compatibility factor in this system (Hilbert et al., 2012).
Many soil-borne fungi directly target the plant root system and auxin production, as also shown for lateral root production in mycorrhized maize (Kaldorf et al., 2005). In soybean (Glycine max), exogenous auxin induces phosphate uptake, demonstrating that plant growth promotion by P. indica might also be the result of improved soil exploitation achieved by auxin-induced root branching (Shen et al., 2006). The bushy root hair phenotype of Chinese cabbage roots conferred by the enhanced auxin content resulting from P. indica colonization promotes acquisition of water and minerals (Lee et al., 2011). The described differences of P. indica in auxin levels and functions in different plant species might be caused by different sensitivities of the species to growth stimulating signals. For example, the Chinese cabbage cultivars used for the co-cultivation analyses (which are mainly used for vegetable production) are optimized to produce large biomasses and might therefore be more susceptible to growth stimulating signals than Arabidopsis which was not under such a selection pressure.
The addition, low concentrations of IAA in barley also led also to suppression of the oxidative burst (Hilbert et al., 2012), suggesting that the IAA produced by the fungus also interferes with host plant defense. The involvement of auxin in balancing growth and defense responses has been often postulated (cf. Egamberdieva et al., 2017). Stringlis et al. (2017) showed that Pseudomonas simiae WCS417 promotes Arabidopsis growth and induces systemic resistance without being warded off by local root immune responses. Using the auxin response mutant tir1afb2afb3, they demonstrated a dual role for auxin signaling in finely balancing growth-promoting and defense eliciting activities of beneficial microbes in plant roots. This requires cross-talks between auxin and defense-related phytohormones. Considering the central role of auxin in beneficial root–microbe interactions, it will be an important task to understand how the symbionts manage to regulate the trait-off between growth and defense in their hosts. Future studies should focus on specific tissues or cells which are targeted by beneficial fungi, and how biologically active auxin maxima are established in response to root colonization, especially, the roles of small secreted proteins from P. indica in auxin synthesis and signaling in the specific tissues or cells. Combinations of –omics approaches, phytohormone analyses and tissue/cell-specific responses in wild-type and auxin mutants will help to unravel the complex roles of auxin in the multiple responses elicited by the beneficial fungi. Studies on the role of auxin in pathogenic plant/microbe organisms (Kunkel and Harper, 2018) or under stress (Kazan, 2013; Naser and Shani, 2016) will provide us with additional tools to understand how auxin is involved in balancing beneficial and non-beneficial traits in symbiotic interactions.
Cytokinin (Ck)
Although CKs are involved in many basic processes including photosynthesis and growth regulation, maintenance of cell proliferation, cell differentiation and retardation of senescence, their role in symbiotic interactions is less clear (Boivin et al., 2016). Besides regulation of the plant CK level, metabolism and signaling in response to root-colonizing microbes, CKs are also produced by rhizospheral microorganisms themselves (Podlešáková et al., 2013; Fusconi, 2014; Kudoyarova et al., 2014). Furthermore, CKs interact with other phytohormones or control defense processes in symbiotic roots (e.g., Bishopp et al., 2011; Jiang et al., 2013). CKs travel from shoot to root and vice versa thereby distributing information within the entire plant. Shoot CK has a positive impact on arbuscular mycorrhizal fungal development in roots and on the root transcript level of the AM-responsive phosphate transporter gene NtPT4 in tobacco. Reduced CK content in roots caused shoot and root growth depression of AM-colonized plants (Cosme et al., 2016b). Systemic shoot-to-root signaling also negatively regulated nodulation in legumes (Sasaki et al., 2014). Moreover, symbiotic root-to-shoot information transfer is necessary for the benefits in the aerial parts of root-colonized plants. Ko et al. (2014) provide molecular evidence for the long-distance transport of CK and showed that AtABCG14, an ABC transporter, is crucial for the translocation of CK from the roots to the shoot, and this is required for normal shoot development. Trans-zeatin-riboside, a CK precursor, is a major long-distance root-to-shoot signaling molecule in xylem vessels and its action depends on metabolic conversion via the LONELY GUY enzyme in proximity to the site of action. An additional long-distance signaling molecule is trans-zeatin, an active CK form. Trans-zeatin, a minor component of xylem CK, controls leaf size but not meristem activity-related traits, whereas trans-zeatin riboside is sufficient for regulating both traits (Osugi et al., 2017, and ref. therein). These observations raise the question whether CKs are involved in microbe-induced systemic signaling, and – if so – how this is related to other components which also distribute information within the plant body.
The information on the involvement of CK in the colonization of the roots by P. indica as well as systemic signal transfer is mainly descriptive. P. indica targeted CK-responsive genes in local root tissues and systemic leaves of colonized plants have been identified in several plant species (e.g., Vadassery et al., 2008; Johnson et al., 2014; Zhang et al., 2018). Vadassery et al. (2008) demonstrated that trans-zeatin biosynthesis and the receptor combination CERK1/AHK2 are important for P. indica-mediated growth promotion in Arabidopsis seedlings, while mutants lacking cis-zeatin showed a wild-type response to the fungus. Since trans-zeatin and its precursor controls the leaf size but not meristem activity-related traits whereas trans-zeatin riboside is sufficient to control both traits (Osugi et al., 2017) via systemic root-to-shoot signaling, it is conceivable that the information transfer from colonized roots to the leaves requires or is dependent on trans-zeatin.
Besides their involvement in systemic signaling, CKs play different roles in the entry of mirco-symbionts into the cortex: they inhibit entry of rhizobacteria and promote colonization of mycorrhizal fungi (Jones et al., 2015). A similar function has been attributed to ABA (López-Ráez, 2016). It would be interesting to understand whether CKs have the same or similar function on root colonization or hyphal entry into the root cell by endophytes, such as P. indica.
A quite interesting study by Hérivaux et al. (2017) showed that histidine kinases in early diverging fungi share a high degree of similarity with phytohormone receptors including those for CK and ET perception. These phytohormone receptor homologs are found in plant root symbionts but also in species that colonize decaying plant material. Hérivaux et al. (2017) hypothesize that these sensing proteins promote the fungal interaction with plants, which might have led to the conquest of land by ancestral fungi. The hypothesis that plant-derived CKs might be perceived by fungal perception systems, is appealing considering that P. indica shows similar symbiotic and saprophytic features as the diverging fungi analyzed early.
Furthermore, there is increasing evidence that volatiles from microbes in the rhizosphere contribute to the performance of plants in ecosystems. Sánchez-López et al. (2016) showed that volatiles emitted by phylogenetically diverse rhizosphere and non-rhizosphere bacteria and fungi (both pathogens and beneficial microbes) promote growth and flowering of various plant species, including crops and Arabidopsis. CKs play essential roles in this phenomenon, because growth and flowering responses to the volatiles were reduced in mutants with CK-deficiency (e.g., 35S:AtCKX1) or low receptor sensitivity (e.g., ahk2/3 mutants). They suggested that plants react to microbial volatiles through highly conserved regulatory mechanisms. Although the role of volatiles in the interaction of P. indica with various plant species is not well understood, their potential involvement in growth responses can be analyzed in the CK mutants.
Taken together, CK may participate in P. indica-induced physiological responses in host plants which have not yet been analyzed or for which an involvement of CK has not yet been investigated.
Gibberellin (GA)
Gibberellin has become a novel player in orchestrating symbiotic interactions of plants with microorganisms (Foo et al., 2014). Many investigations demonstrated that GAs crosstalk with other phytohormones and are involved in stress resistance, in particular salt stress (reviewed in Egamberdieva et al., 2017). DELLA proteins are master negative regulators of GA signaling, and integrate quite diverse hormone signaling pathways and pathways from developmental processes. They also control and coordinate many steps in root development, including the formation of endosymbiotic interactions with rhizobial bacteria and mycorrhizal fungi (Yu et al., 2014; Fonouni-Farde et al., 2016). GA signaling inhibits AM formation (Gutjahr, 2014; Martín-Rodríguez et al., 2015, cf. also Ortu et al., 2012; Takeda et al., 2015a,b), and GA is controlled by ABA (Martín-Rodríguez et al., 2016). Reduced Arbuscular Mycorrhiza1 (RAM1), a GRAS protein, regulates arbuscule development, and a Lotus japonicas mutant impaired in RAM1 function is arrested in arbuscule branching. Symbiotic and GA signals control RAM1 expression and arbuscule development (Pimprikar et al., 2016). In pea roots, AM colonization was increased in GA-deficient mutants while a mutant which lacks DELLA proteins showed reduced colonization (Foo et al., 2013, 2014, 2016).
In contrast, quite little is known about the role of GA in interactions of endophytes with roots, and the available studies on P. indica mainly focused on the activation of innate immune responses. Cosme et al. (2016a) showed that P. indica helps rice plants to tolerate root herbivory, and GA functions as a signal component of inducible plant tolerance against the biotic stress. Barley mutants impaired in GA synthesis as well as perception showed reduced colonization by P. indica which implicates that GA functions as a modulator of the root’s basal defense (Schäfer et al., 2009b). A quintuple-DELLA mutant displayed constitutive GA responses and the GA biosynthesis mutant ga1-6 (for GA requiring 1) showed higher and lower degrees of colonization in Arabidopsis roots, respectively, suggesting that P. indica recruits GA signaling to establish root cell colonization (Jacobs et al., 2011). Ent-kaurene synthases and ent-kaurene-like synthases are involved in the biosynthesis of phytoalexins and/or GAs. Li et al. (2016) demonstrated that kaurene synthase activity is required for successful root colonization of P. indica in barley. Finally, P. indica-induced growth promotion of Chinese cabbage and barley seedlings correlated to an increased GA level in the colonized roots (Schäfer et al., 2009b; Lee et al., 2011), and the GA2ox gene involved in inactivation of GA was down-regulated in P. indica-colonized barley roots (Hedden, 2008; Schäfer et al., 2009b). In most of these publications, a crosstalk to or involvement in other phytohormones is proposed to induce the described effects. Whether all these observations are based on a common GA mechanism, requires more investigations.
Gibberellin is involved in early flowering phenotype, and P. indica promotes early flowering and plant growth in the medicinal plant Coleus forskohlii and Arabidopsis. To determine the impact of P. indica on flowering time in Arabidopsis, Kim et al. (2017) co-cultivated Arabidopsis plants with the fungus under long day condition, and these plants displayed an early flowering phenotype. The same results were reported by Pan et al. (2017). Colonized plants had higher transcript levels of the flowering regulatory genes FLOWERING LOCUS T, LEAFY, and APETALA1, as well as of the GA biosynthetic genes Gibberellin 20-Oxidase 2, Gibberellin 3-Oxidase 1 and Gibberellin Requiring 1, while the flowering-repressing gene FLOWERING LOCUS C was down-regulated. Quantification of GA contents showed that the colonization by P. indica caused an increase in GA4 content. Compared to wild-type, inoculation of the Arabidopsis ga5 mutant defective in an GA biosynthetic gene led to less pronounced changes in the expression of genes regulating flowering and to a lower increase in GA4 content. Taken together, the two studies demonstrated that P. indica promotes early flowering in Arabidopsis likely by increasing the GA content.
Abscisic Acid (ABA)
Abscisic acid is a classical stress hormone and involved in many osmotic responses including salt and drought stress. A classical ABA response is the closure of the stomata, which prevents or restricts water loss and pathogen entry into the host plants. ABA often represses plant immune responses, and pathogens also utilize or synthesize ABA for suppression or manipulation of the immunity of their hosts (Mbengue et al., 2016; Lievens et al., 2017). The importance of ABA for beneficial symbiotic interactions has recently become an interesting research field (Lievens et al., 2017). In general, ABA promotes AM symbiosis although the effect of the hormone is strongly dependent on the developmental stage of the interaction and stress conditions (López-Ráez, 2016). In contrast, its role in the legume–rhizobia interaction is mainly inhibitory (Lievens et al., 2017). ABA comes into play when plants and their associated symbionts are exposed to stresses, in particular osmotic stress. Unstressed plants with sufficient nutrient supply do not require help from symbionts. Vahabi et al. (2015) showed that the benefits for the plants in the symbiotic interaction between P. indica and Arabidopsis increase with increasing moderate stress, because the plants have to decide whether they invest in either stress tolerance responses or symbiotic features. Stec et al. (2016) proposed that the fluctuation in the ABA levels may work as an alert system that calculates the ratio between costs and incomes. The ABA level may function as a monitor for the decision whether an investment in a particular symbiotic interaction is higher than the profit coming out of it. In the light of such a hypothesis, ABA regulation in response to P. indica colonization might be interesting since the growth and stress conditions can be experimentally manipulated.
A strong up-regulation of the ABA concentration during P. indica colonization was observed in Arabidopsis roots and shoots during the early recognition phase and before a physical contact between the two symbiotic partners (Vahabi et al., 2015). Apparently, mobile signaling molecules from the fungus, such as cellotriose (Johnson et al., 2018) or small secreted proteins (Akum et al., 2015), inform the plants about the presence of the microbe, and activate ABA-dependent stress responses. The plants also close the stomata. Interestingly, the ABA levels decreased in both roots and shoots when a physical contact between the two organisms was established (Vahabi et al., 2015). It appears that the microbe is no longer recognized as a threat. ABA is also involved in plant innate immune responses, and may thereby interfere with or even control root colonization. Peskan-Berghöfer et al. (2015) showed that treatment of Arabidopsis seedlings with exogenous ABA or the ABA analog pyrabactin increased fungal colonization efficiency without impairment of plant fitness. Concomitantly, the ABA-deficient mutants aba1 and aba2 were less colonized, while plants exposed to moderate stress were more colonized than corresponding controls. Sustained exposure to ABA attenuated expression of transcription factors MYB51, MYB122, and WRKY33 in roots upon P. indica challenge. Thus, ABA strengthens the interaction of Arabidopsis roots with P. indica as a consequence of its impact on plant innate immunity. This will also have a strong impact on the establishment and outcome of the symbiosis under stress conditions. Similar results have been obtained with arbuscular mycorrhizal fungi, where ABA promotes the infection process and establishment of the compatible interaction (Herrera-Medina et al., 2007; Charpentier et al., 2014; Fracetto et al., 2017; Lievens et al., 2017). Salomon et al. (2014) also demonstrated that root-colonizing bacteria were able to interfere with their host plants by producing ABA. In summary, the available data support a concept in which ABA plays an important role in the control of beneficial traits in the symbiosis, in particular under stress, and may even function as sensor.
Ethylene (ET)
The plant hormone ET controls flowering, ripening, seed dormancy, seed germination, senescence, root formation, plant growth, and adaptation under abiotic and biotic stress (Kende, 2003; Abeles et al., 2012). The gaseous hormone is synthesized from the precursor ACC, which derives from methionine and ACC synthase and ACC oxidase are key enzymes in this biosynthetic pathway.
The role of ET in mutualistic interactions is complicated and not clear, and numerous reports demonstrated its involvement in quite different steps. Correlations of ET emissions with P. indica-induced responses in the hosts have not yet been performed and the participation of this hormone in P. indica–host interactions is mainly based on mutant analyses or altered expression of ET-responsive genes. Some plant-growth promoting bacteria, such as Rhizobacteria spp., degrade the ET precursor ACC with an ACC deaminase, which presumably promotes growth of the microbes by repressing hosts’ defense system which is activated by ET (cf. Shaharoona et al., 2006). A gene encoding such an enzyme has not been found in the P. indica genome. In many studies, colonization of roots by mycorrhizal fungi or rhizobacteria is inhibited by ET, however, there are also reports showing opposite effects (cf. Khatabi and Schäfer, 2012). In the root–P. indica interaction, the fungus positively modulates expression of ACC synthase (Khatabi et al., 2012; Ansari et al., 2013), and ET has been implicated as a positive modulator of the symbiosis in Arabidopsis and barley roots (Khatabi et al., 2012). Moreover, DNA microarray-based gene expression analysis of barley roots colonized by P. indica showed differential expression of genes related to ET synthesis and signaling (Khatabi et al., 2012). P. indica induces ET synthesis in barley and Arabidopsis during colonization and Arabidopsis mutants impaired in ET signaling were less colonized by the fungus and mutants with constitutive ET signaling, ET synthesis or ET-related defenses were hyper-susceptible to P. indica. The fungus also induces methionine synthase which may provide more substrates for ET synthesis (Peskan-Berghöfer et al., 2004). The observation that ET supports root colonization is consistent with the requirement of JA for root colonization (cf. below, Verma et al., 1998), and the two hormones function synergistically in the host in response to P. indica colonization. ET induces innate immune responses against pathogenic microbes, but also mildly in response to beneficial root-colonizing fungi, for example before recognition of the microbe as a friend, for restriction of root colonization or secondary colonization of distal root parts by the same microorganism. Thus, ET is probably one of many factors which plays role in balancing beneficial and non-beneficial colonization traits through signaling components in P. indica symbiosis (Camehl et al., 2010).
The growth of Arabidopsis mutants impaired in ET biosynthesis and activation of ET response (etr1, ein2, and ein3/eil1), were not promoted or even inhibited by P. indica (Camehl et al., 2010), suggesting that these ET signaling components are required for P. indica-induced growth promotion in Arabidopsis. In older plants, which are already colonized by the fungus, ETR1, EIN2, and EIN3/EIL1 participate in restricting root colonization and repressing defense responses. Consequently, ET perception and signaling, as well as ET-targeted transcription factors, are crucial for P. indica-induced growth promotion in Arabidopsis (Camehl et al., 2010). The available data suggest that ET might have different effects during different stages of the symbiosis in balancing beneficial and non-beneficial traits in the symbiosis. This is not surprising considering the huge amount of ET targets and ET-targeted transcription factors. More than 100 ET RESPONSE FACTORS (ERFs) affect quite different downstream responses both positively and negatively ranging from development, metabolic processes to defense gene activation. Camehl and Oelmüller (2010) showed that two ERFs, ERF9 and ERF14, are required for the growth promoting response of P. indica in Arabidopsis. Finally, P. indica confers salt-stress tolerance in barley (Ghaffari et al., 2016). The fungus-induced reprogramming during salt stress affects major metabolic and transcriptomic processes including the ET biosynthesis pathway. The involvement of the gaseous hormone in major developmental steps and its crosstalk with other hormones makes it difficult to define specific effects in the stabilization of a beneficial symbiosis under changing environmental and developmental conditions. ET also participates in rhizobacteria-induced induced systemic resistance (ISR), where the microbes activate processes in roots which induce systemic resistance in the aerial parts of the plants against microbial pathogens or insects (Verhagen et al., 2004). P. indica has been shown to induce ISR in various plant species, and ET and jasmonates have been proposed to be involved in the signal transfer and/or realization (cf. Molitor and Kogel, 2009). ET as a gaseous hormone, can easily stimulate these responses systemically in distant tissues, but also ACC travels from the roots to the aerial parts via the xylem (Bradford and Yang, 1980; Finlayson et al., 1991; Tudela and Primo-Millo, 1992, and ref. therein). Finally, ET might also play a role in interplant communication, by informing adjacent plants about upcoming threat in a plant community. Understanding of the role of ET in plant–microbe interaction requires probably broader experimental approaches which should consider also other players and hormones in the communication networks.
A recent study by de Zélicourt et al. (2018) shows a novel role of ET in stress tolerance. The desert endophyte Enterobacter sp. SA187 enhances yield of the crop plant alfalfa under field conditions as well as growth of Arabidopsis in vitro. Among the different mechanisms related to the beneficial association of SA187 with plants, the bacterium induces salt stress tolerance by production of 2-keto-4-methylthiobutyric acid (KMBA) which is known to be converted to ET. Using this novel chemical compounds, the authors demonstrated that the Arabidopsis ET signaling pathway, but not ET production is required for KMBA-induced plant salt stress tolerance. Since ET has been proposed to be involved in numerous plant resistance responses in symbiotic interactions, it remains to be seen, whether the novel chemical mediator KMBA is also produced by other endophytes.
SA and JA, Two Phytohormones Involved in Quite Different Defense Strategies
The two phytohormones SA and JA mediate defense responses and systemic signal propagation in plant–microbe interactions, whereas SA is mainly involved in the response to biotrophic microbes and JA (and ET) to necrotrophic microbes. Studies have also revealed the dependency of P. indica on JA-mediated suppression of early immune responses (e.g., root oxidative burst) as well as SA- and glucosinolate-related defense pathways (Jacobs et al., 2011; Gill et al., 2016). To ensure that plants establish a proper response to attacking pathogens, there is a massive crosstalk between the two hormone signaling pathways, in which one hormone represses the action of the other. In many aspects, initial steps in establishing beneficial plant–microbe interactions resemble those of biotrophic interactions, therefore, up-regulation of SA levels and intensified SA signaling is likely, in particular since SA is also known to participate in ISR responses, which are often induced by root-colonizing beneficial microbes. However, this appears to be too simplified considering the literature published on P. indica–host interactions.
Mutualistic interactions are characterized by several phases: during early phases of recognition, the host has not yet physically contacted the microbe and recognized it as a friend. This results in an activation of a mild defense response which involves SA and/or jasmonates. Vahabi et al. (2015) showed that both SA and jasmonate levels increased when Arabidopsis roots were growing next to P. indica before a physical contact has been established. This suggests that the growing hyphae release chemical mediators which activate SA and jasmonate biosynthesis genes in the roots. During a second phase, the two symbionts have physical contact and root colonization is initiated, which results in a local repression of the host’s defense response and lower SA and jasmonate levels (Vahabi et al., 2015). This can be caused by numerous factors: effector proteins released by the microbes can actively repress plant immune responses, but progression of root colonization can also “convince” the plant that the microbe is friendly. Down-regulation of the defense machinery in the host may be a consequence of the beginning nutrient exchange. Ultimately, a balance between growth of the microbes in the host and the profit of both symbiotic partners from the symbiosis has to be established, and this balance requires plant defense strategies to restrict the progression of root colonization. Analyses of phytohormone mutants demonstrated that SA, JA, and ET are crucial players in establishing and maintaining the balance, and the participation of the individual hormones depends on the developmental stage and growth conditions. Apparently, both SA and jasmonates are involved in controlling root colonization at the infected sites and the colonization success essentially depends on the evolution of strategies for immunosuppression (Jacobs et al., 2011).
In contrast, when Arabidopsis roots are directly exposed to P. indica hyphae, the JA and JA-Ile levels are up-regulated, although the benefits for the plants were not lost (Vahabi et al., 2013). Apparently, under these conditions, the microbe is recognized as threat. Similarly, Lahrmann et al. (2015) found that P. indica as well as Sebacina vermifera stimulated the SA catabolism and the accumulation of jasmonates and glucosinolates in Arabidopsis roots. In the context of the saprophytic traits of the microbes they concluded that the symbiotic interaction of these microbes requires non-compromised plant innate immune responses. Vahabi et al. (2016) investigated beneficial and non-beneficial traits in the symbiotic interaction between P. indica and Arabidopsis roots and found that mild stress (limited access to nutrients, exposure to heavy metals or salt, light and osmotic stress, pathogen infections) promotes the benefits for the plant in the symbiosis. A physical contact was necessary, suggesting that the endophyte helps the plant after recognition of P. indica as a friend. Furthermore, the level of active jasmonates increased under increasing stress conditions. A testable hypothesis could be that shifting the symbiosis from beneficial to less beneficial conditions might be associated with a shift from SA to jasmonate usage as the defense-regulating plant hormone.
Akum et al. (2015) investigated the P. indica effector protein PIIN_08944, which promotes the mutualistic symbiosis and root colonization. When ectopically expressed in Arabidopsis and barley, the effector protein contributed to root colonization by interfering with the SA-mediated basal immune response. Interestingly, the transgenic Arabidopsis plants supported the growth of a biotrophic oomycete while growth of necrotrophic fungi on Arabidopsis or barley was not affected. This result supports the important role of SA in root colonization and shows that the microbe has specific strategies to interfere with the plant SA pathway.
P. indica controls secondary colonization in distal root areas by stimulating JA-dependent defense responses, which demonstrates systemic signaling. While ISR is normally considered as SA dependent, Stein et al. (2008) demonstrated that JA signaling and the cytoplasmic, but not nuclear localization of NPR1 are required for P. indica-induced resistance against powdery mildew infection. Two jasmonate signaling mutants were non-responsive to P. indica and JA-responsive vegetative storage protein expression was primed in response to the pathogen infection. The resistant phenotype was independent of SA and SA biosynthesis and signaling (Molitor and Kogel, 2009). How the information travels to the aerial parts is not known, but several reports suggested that, like in roots, altered phytohormone levels in the leaves suppress host immunity (cf. Jacobs et al., 2011) or prime the aerial parts for better protection against pathogen attack (e.g., Sun et al., 2014). Stimulation of jasmonates and JA-responsive genes by A. brassicae infection was strongly inhibited when the plants are colonized by P. indica (Vahabi et al., 2013, 2018), suggesting that the colonized plants suppress the host immunity because these plants are stronger and healthier and require less JA-dependent defense for protection. In maize seedlings, the drought stress responses were also diminished by P. indica, but in this study, the fungus stimulated the expression of SA-related genes in roots (Zhang et al., 2018). Altered phytohormone levels can also result in a secondary effect: e.g., P. indica stimulated the expression of a tau-type glutathione S-transferase in Chinese cabbage. When the gene encoding the enzyme was expressed in Arabidopsis, the plants performed better and stimulated the expression of auxin-, SA- and JA-responsive genes (Kao et al., 2016). Thus, the fungus-induced reprogramming of the host’s gene expression might alter phytohormone levels.
Brassinosteroids (BRs)
Other phytohormones synthesized or manipulated by the root endophytes include brassinosteroids (BRs). Direct measurements of these steroid hormones in response to root colonization by P. indica have not yet been performed, however, the gene encoding cycloartenol synthase, which contributes to the synthesis of BR precursors, and BLE2 encoding a BR-responsive nine-transmembrane protein, are up-regulated after P. indica inoculation. In addition, two BAK1 (brassinosteroid insensitive 1-associated receptor kinase 1) genes involved in BR signaling are induced (Schäfer et al., 2009a,b).
Conclusion
The symbiotic relationship with P. indica promotes growth and enhances stress tolerance in plants, which involves two possible mechanisms. (1) The first is stimulating the action of host stress-responsive systems under a particular stress, which directs the plants to either keep away from or mitigate the stress. The mechanism of drought and salt tolerance mediated by fungi in plants involves the action of CK (Miller, 1974), ABA (Nishiyama et al., 2011), and fungal ACC deaminase to utilize ACC (Viterbo et al., 2010). Additionally, SA and JA are likely involved in ISR conferred by P. indica inoculation. (2) Another possible mechanism involves the synthesis of anti-stress biochemicals by endophytes (Singh et al., 2011). Some microbes clearly synthesize auxins or CK, which influence plant metabolism. Fungi also stimulate lateral root formation and increase the root surface area by producing auxins and GA, which help plants take up more water and minerals during stresses, thereby enhancing survival and yield. The role of phytohormones in P. indica–plants interactions has been simply summarized in Figure 1. In recent years melatonin has emerged as a research highlight in plant studies. Melatonin plays important roles in plant growth and development (Chen et al., 2009), and is related to abiotic stresses such as drought, radiation, extreme temperature, and other harsh environmental conditions (Zhang et al., 2012; Byeon and Back, 2014; Wang et al., 2014; Li et al., 2017). Exposure of plants to environmental stress can increase the level of endogenous melatonin (Zhao et al., 2013). Overexpression of the melatonin biosynthetic genes elevates melatonin levels and enhanced tolerance to abiotic stresses (Zhang et al., 2012). More importantly, the antioxidant system is one of the targets of melatonin in plants stress tolerance, and preliminary data suggest that the melatonin targets are similar to those of root-colonizing microorganisms including P. indica (Rodriguez et al., 2010; Narayan et al., 2017). Though melatonin distinguishes from a classic hormone such as its direct, non-receptor-mediated free radical scavenging activity, it could cross talk with stress or toher hormones, like IAA, ABA, JA, SA, and ET (Zhang N. et al., 2015). How the beneficial effects induced by P. indica are related to melatonin needs to be elucidated.
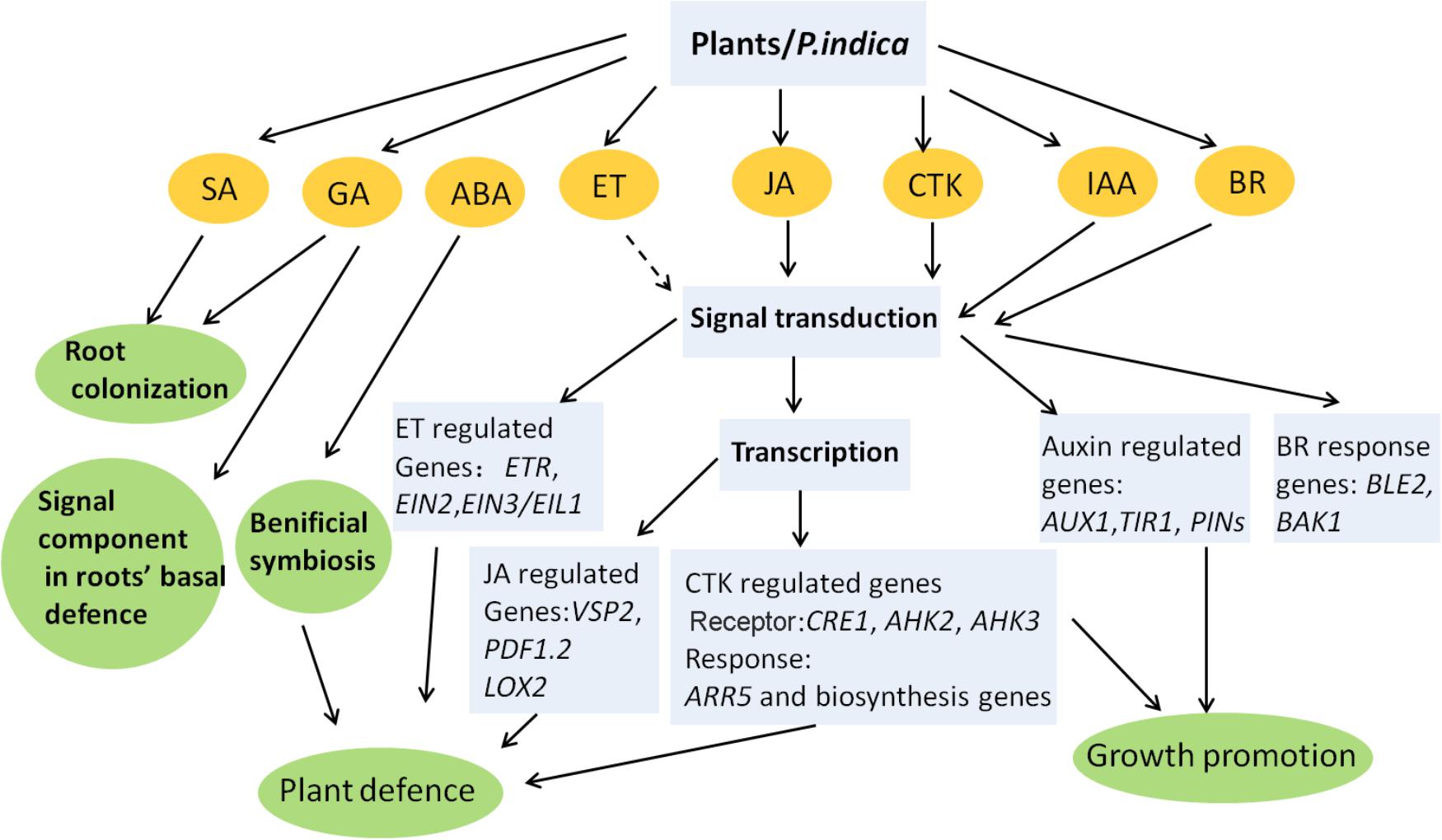
FIGURE 1. Phytohormone-related processes influenced by P. indica in plants. Most of the conclusions are obtained from Arabidopsis, barley, and Chinese cabbage. Dashed line represents undefined role. Interactions between different phytohormones are not indicated. The model is adapted from: Gill et al. (2016) and Varma et al. (2012) and enriched by adding new findings.
Thus, the fungus is ideal to study many of the open questions of the role of phytohormones in plant–microbe interaction. Since P. indica colonizes the roots of many host plants, similarities and differences between different host species can be investigated, which ultimately is also relevant for agriculture. Agricultural productivity suffers a heavy loss due to plant pathogens, insect pests and various abiotic stresses. Many endophytic fungi like P. indica are able to produce antimicrobial compounds, plant growth hormones and various agrochemical bioactive metabolites and play an important role in plant growth promotion, higher seed yield and plants resistance to various biotic, abiotic stresses, and diseases. Biotechnological applications of the endophytic fungi could be an ideal strategy for sustainable agriculture (Oelmüller et al., 2009). Moreover, endophytic fungi can be applied for advancement of chemical synthesis using genetic engineering tools for the generation of new organisms. Further, growing endophytes in large scale, modifying culture conditions like changing pH, changing growth media and supplying some stimulants might help in getting better production of the particular bioactive compound for agricultural purpose (Varma et al., 2012; Rai et al., 2014).
Chemical mediators released by microbes into the rhizosphere may be crucial players in the rhizosphere and could stabilize symbiotic interactions. Very little is known about chemical compounds which participate in the communication of the community members in the ecosystem. Recently, it was demonstrated that P. indica releases cellotriose in nmol concentrations which is perceived by roots and activates a surveillance system that informs the host about the integrity of its cell wall (Johnson et al., 2018). The role of phytohormones in the plant response to such chemical mediators need to be investigated. Cellotriose is one of million chemical mediators which are synthesized and released by microorganisms in extreme low concentrations to facilitate communication among community members. Phytohormones also play important roles in shaping the rhizosphere community by participating in transferring information systemically from the roots to the shoots, but also by transferring information to neighboring plants. Again, the interaction of P. indica with Arabidopsis roots served as a model system to demonstrate that threat induced by Alternaria brassicae in one leaf of a plant induces systemic JA responses in the entire infected plants including the roots. This information travels to neighboring plants via a P. indica hyphal network and activates ABA responses (Vahabi et al., 2018). In the context of the ideas by Stec et al. (2016) who proposed that the fluctuation in the ABA levels may work as an alert system that calculates the ratio between costs and incomes for an individual plant, a broader concept for the entire plant/microbe community in an ecosystem could be developed. Well-established model systems also demonstrate that phytohormone-based threat information applied to one plant travels to neighboring plants via mycelial hyphal networks which connect different plants and even plant species in an ecosystem. Threat information about herbivore attacks or pathogen infections can also be distributed in a plant community by volatiles, which induces appropriate phytohormone-based defense responses in non-threatened neighboring plants (see references in Hettenhausen et al., 2017; Vahabi et al., 2018). These examples demonstrate that phytohormones play important roles in shaping community structures. A better understanding of the role of phytohormones in rhizosphere communities will contribute substantially to improve agricultural applications.
Author Contributions
WZ and RO conceived the topic and outline and critically revised the manuscript. LX prepared the manuscript draft. CW contributed critical components to the draft. All authors reviewed the manuscript.
Conflict of Interest Statement
The authors declare that the research was conducted in the absence of any commercial or financial relationships that could be construed as a potential conflict of interest.
Acknowledgments
RO was supported by the CRC1127.
Abbreviations
ABA, abscisic acid; ACC, 1-aminocyclopropane-1-carboxylic acid; AM, arbuscular mycorrhiza; AMF, arbuscular mycorrhizal fungus; CK, cytokinin; ET, ethylene; GA, gibberellin; IAA, indole-3-acetic acid; JA, jasmonic acid; JA-Ile, JA-isoleucine; MAMPs, microbe-associated molecular patterns; MTI, MAMP-triggered immunity; OPDA, 12-oxo-phytodienoic acid; SA, salicylic acid.
References
Abd El-Hadi Nadia, I. M., Abo El-Ala, H. K., and Abd El-Azim, W. M. (2009). Response of some Mentha species to plant growth promoting bacteria isolated from soil rhizosphere. Aust. J. Basic Appl. Sci. 3, 4437–4448.
Abeles, F. B., Morgan, P. W., Mikal, E., and Saltveit, J. (2012). Ethylene in Plant Biology, 2nd Edn. London: Academic Press, Inc.
Ahmed, A., and Hasnain, S. (2010). Auxin producing Bacillussp.: auxin quantification and effect on the growth Solanum tuberosum. Pure Appl. Chem. 82, 313–319. doi: 10.1351/PAC-CON-09-02-06
Akum, F. N., Steinbrenner, J., Biedenkopf, D., Imani, J., and Kogel, K. H. (2015). The Piriformospora indica effector PIIN_08944 promotes the mutualistic sebacinalean symbiosis. Front. Plant Sci. 6:906. doi: 10.3389/fpls.2015.00906
Ansari, M. W., Trivedi, D. K., Sahoo, R. K., Gill, S. S., and Tuteja, N. (2013). A critical review on fungi mediated plant responses with special emphasis to Piriformospora indica on improved production and protection of crops. Plant Physiol. Biochem. 70, 403–410. doi: 10.1016/j.plaphy.2013.06.005
Avramova, Z. (2017). The jasmonic acid-signalling and abscisic acid-signalling pathways cross talk during one, but not repeated, dehydration stress: a non-specific ‘panicky’ or a meaningful response? Plant Cell Environ. 40, 1704–1710. doi: 10.1111/pce.12967
Bishopp, A., Lehesranta, S., Vatén, A., Help, H., El-Showk, S., Scheres, B., et al. (2011). Phloem-transported cytokinin regulates polar auxin transport and maintains vascular pattern in the root meristem. Curr. Biol. 21, 927–932. doi: 10.1016/j.cub.2011.04.049
Boivin, S., Fonouni-Farde, C., and Frugier, F. (2016). How auxin and cytokinin phytohormones modulate root microbe interactions. Front. Plant Sci. 7:1240. doi: 10.3389/fpls.2016.01240
Bradford, K. J., and Yang, S. F. (1980). Xylem transport of 1-Aminocyclopropane-1-carboxylic acid, an ethylene precursor, in waterlogged tomato plants. Plant Physiol. 65, 322–326. doi: 10.1104/pp.65.2.322
Byeon, Y., and Back, K. (2014). Melatonin synthesis in rice seedlings in vivo is enhanced at high temperatures and under dark conditions due to increased serotonin N “cetyltransferase and N”cetylserotonin methyltransferase activities. J. Pineal Res. 56, 189–195. doi: 10.1111/jpi.12111
Caarls, L., Pieterse, C. M., and Van Wees, S. C. (2015). How salicylic acid takes transcriptional control over jasmonic acid signaling. Front. Plant Sci. 6:170. doi: 10.3389/fpls.2015.00170
Cakmak, I., and Marschner, H. (1992). Magnesium deficiency and high light intensity enhance activities of superoxide dismutase, ascorbate peroxidase, and glutathione reductase in bean leaves. Plant Physiol. 98, 1222–1227. doi: 10.1104/pp.98.4.1222
Camehl, I., and Oelmüller, R. (2010). Do ethylene response factorS9 and -14 repress PR gene expression in the interaction between Piriformospora indica and Arabidopsis? Plant Signal. Behav. 5, 932–936.
Camehl, I., Sherameti, I., Venus, Y., Bethke, G., Varma, A., Lee, J., et al. (2010). Ethylene signalling and ethylene-targeted transcription factors are required to balance beneficial and nonbeneficial traits in the symbiosis between the endophytic fungus Piriformospora indica and Arabidopsis thaliana. New Phytol. 185, 1062–1073. doi: 10.1111/j.1469-8137.2009.03149.x
Celloto, V. R., Oliveira, A. J. B., Gonçalves, J. E., Watanabe, C. S. F., Matioli, G., and Gonçalves, R. A. C. (2012). Biosynthesis of indole-3-acetic acid by new Klebsiella oxytoca free and immobilized cells on inorganic matrices. Sci. World J. 2012:495970. doi: 10.1100/2012/495970
Charpentier, M., Sun, J., Wen, J., Mysore, K. S., and Oldroyd, G. E. (2014). Abscisic acid promotion of arbuscular mycorrhizal colonization requires a component of the protein phosphatase 2A complex. Plant Physiol. 166, 2077–2090. doi: 10.1104/pp.114.246371
Chen, Q., Qi, W. B., Reiter, R. J., Wei, W., and Wang, B. M. (2009). Exogenously applied melatonin stimulates root growth and raises endogenous indoleacetic acid in roots of etiolated seedlings of Brassica juncea. J. Plant Physiol. 166, 324–328. doi: 10.1016/j.jplph.2008.06.002
Cosme, M., Lu, J., Erb, M., Stout, M. J., Franken, P., and Wurst, S. (2016a). A fungal endophyte helps plants to tolerate root herbivory through changes in gibberellin and jasmonate signaling. New Phytol. 211, 1065–1076. doi: 10.1111/nph.13957
Cosme, M., Ramireddy, E., Franken, P., Schmülling, T., and Wurst, S. (2016b). Shoot- and root-borne cytokinin influences arbuscular mycorrhizal symbiosis. Mycorrhiza 26, 709–720. doi: 10.1007/s00572-016-0706-3
de Zélicourt, A., Synek, L., Saad, M. M., Alzubaidy, H., Jalal, R., Xie, Y., et al. (2018). Ethylene induced plant stress tolerance by Enterobacter sp. SA187 is mediated by 2-keto-4-methylthiobutryric acid production. PLoS Genet. 14:e1007273. doi: 10.1371/journal.pgen.1007273
Dong, S., Tian, Z., Chen, P. J., Senthil Kumar, R., Shen, C. H., Cai, D., et al. (2013). The maturation zone is an important target of Piriformospora indica in Chinese cabbage roots. J. Exp. Bot. 64, 4529–4540. doi: 10.1093/jxb/ert265
Egamberdieva, D., Wirth, S. J., Alqarawi, A. A., Abd Allah, E. F., and Hashem, A. (2017). Phytohormones and beneficial microbes: essential components for plants to balance stress and fitness. Front. Microbiol. 8:2104. doi: 10.3389/fmicb.2017.02104
Felten, J., Kohler, A., Morin, E., Bhalerao, R. P., Palme, K., Martin, F., et al. (2009). The ectomycorrhizal fungus Laccaria bicolor stimulates lateral root formation in poplar and Arabidopsis through auxin transport and signaling. Plant Physiol. 151, 1991–2005. doi: 10.1104/pp.109.147231
Felten, J., Legué, V., and Ditengou, F. A. (2010). Lateral root stimulation in the early interaction between Arabidopsis thaliana and the ectomycorrhizal fungus Laccaria bicolor: is fungal auxin the trigger? Plant Signal. Behav. 5, 864–867. doi: 10.1104/pp.109.147231
Finlayson, S. A., Foster, K. R., and Reid, D. M. (1991). Transport and metabolism of 1-Aminocyclopropane-1-carboxylic acid in sunflower (Helianthus annuus L.) seedlings. Plant Physiol. 96, 1360–1367. doi: 10.1104/pp.96.4.1360
Fonouni-Farde, C., Diet, A., and Frugier, F. (2016). Root development and endosymbioses: DELLAs lead the orchestra. Trends Plant Sci. 21, 898–900. doi: 10.1016/j.tplants.2016.08.012
Foo, E., Ferguson, B. J., and Reid, J. B. (2014). Common and divergent roles of plant hormones in nodulation and arbuscular mycorrhizal symbioses. Plant Signal. Behav. 9:e29593. doi: 10.4161/psb.29593
Foo, E., McAdam, E. L., Weller, J. L., and Reid, J. B. (2016). Interactions between ethylene, gibberellins, and brassinosteroids in the development of rhizobial and mycorrhizal symbioses of pea. J. Exp. Bot. 67, 2413–2424. doi: 10.1093/jxb/erw047
Foo, E., Ross, J. J., Jones, W. T., and Reid, J. B. (2013). Plant hormones in arbuscular mycorrhizal symbioses: an emerging role for gibberellins. Ann. Bot. 111, 769–779. doi: 10.1093/aob/mct041
Fracetto, G. G., Peres, L. E., and Lambais, M. R. (2017). Gene expression analyses in tomato near isogenic lines provide evidence for ethylene and abscisic acid biosynthesis fine-tuning during arbuscular mycorrhiza development. Arch. Microbiol. 199, 787–798. doi: 10.1007/s00203-017-1354-5
Fu, J., Liu, H., Li, Y., Yu, H., Li, X., Xiao, J., et al. (2011). Manipulating broad-spectrum disease resistance by suppressing pathogen-induced auxin accumulation in rice. Plant Physiol. 155, 589–602. doi: 10.1104/pp.110.163774
Fu, J., and Wang, S. (2011). Insights into auxin signaling in plant-pathogen interactions. Front. Plant Sci. 2:74. doi: 10.3389/fpls.2011.00074
Fusconi, A. (2014). Regulation of root morphogenesis in arbuscular mycorrhizae: what role do fungal exudates, phosphate, sugars and hormones play in lateral root formation? Ann Bot. 113, 19–33. doi: 10.1093/aob/mct258
Gamas, P., Brault, M., Jardinaud, M. F., and Frugier, F. (2017). Cytokinins in symbiotic nodulation: when, where, what for? Trends Plant Sci. 22, 792–802. doi: 10.1016/j.tplants.2017.06.012
Ghaffari, M. R., Ghabooli, M., Khatabi, B., Hajirezaei, M. R., Schweizer, P., and Salekdeh, G. H. (2016). Metabolic and transcriptional response of central metabolism affected by root endophytic fungus Piriformospora indica under salinity in barley. Plant Mol. Biol. 90, 699–717. doi: 10.1007/s11103-016-0461-z
Gill, S. S., Gill, R., Trivedi, D. K., Anjum, N. A., Sharma, K. K., Ansari, M. W., et al. (2016). Piriformospora indica: potential and significance in plant stress tolerance. Front. Microbiol. 7:332. doi: 10.3389/fmicb.2016.00332
Glick, B. R. (1995). The enhancement of plant growth by free living bacteria. Can. J. Microbiol. 41, 109–117. doi: 10.1139/m95-015
Gutjahr, C. (2014). Phytohormone signaling in arbuscular mycorhiza development. Curr. Opin. Plant Biol. 20, 26–34. doi: 10.1016/j.pbi.2014.04.003
Gutjahr, C., and Parniske, M. (2013). Cell and developmental biology of arbuscular mycorrhiza symbiosis. Annu. Rev. Cell Dev. Biol. 29, 593–617. doi: 10.1146/annurev-cellbio-101512-122413
Harman, G. E. (2011). Multifunctional fungal plant symbionts: new tools to enhance plant growth and productivity. New Phytol. 189, 647–649. doi: 10.1111/j.1469-8137.2010.03614.x
Hérivaux, A., Dugé de Bernonville, T., Roux, C., Clastre, M., Courdavault, V., Gastebois, A., et al. (2017). The identification of phytohormone receptor homologs in early diverging fungi suggests a role for plant sensing in land colonization by fungi. mBio 8:e1739-16. doi: 10.1128/mBio.01739-16
Herrera-Medina, M. J., Steinkellner, S., Vierheilig, H., Ocampo Bote, J. A., and García Garrido, J. M. (2007). Abscisic acid determines arbuscule development and functionality in the tomato arbuscular mycorrhiza. New Phytol. 175, 554–564. doi: 10.1111/j.1469-8137.2007.02107.x
Hettenhausen, C., Li, J., Zhuang, H., Sun, H., Xu, Y., Qi, J., et al. (2017). Stem parasitic plant Cuscuta australis (dodder) transfers herbivory-induced signals among plants. Proc. Natl. Acad. Sci. U.S.A. 114, E6703–E6709. doi: 10.1073/pnas.1704536114
Hilbert, M., Nostadt, R., and Zuccaro, A. (2013). Exogenous auxin affects the oxidative burst in barley roots colonized by Piriformospora indica. Plant Signal. Behav. 8:e23572. doi: 10.4161/psb.23572
Hilbert, M., Voll, L. M., Ding, Y., Hofmann, J., Sharma, M., and Zuccaro, A. (2012). Indole derivative production by the root endophyte Piriformospora indica is not required for growth promotion but for biotrophic colonization of barley roots. New Phytol. 196, 520–534. doi: 10.1111/j.1469-8137.2012.04275.x
Howe, G. A., and Jander, G. (2008). Plant immunity to insect herbivores. Annu. Rev. Plant Biol. 59, 41–66. doi: 10.1146/annurev.arplant.59.032607.092825
Hu, Y., Jiang, Y., Han, X., Wang, H., Pan, J., and Yu, D. (2017). Jasmonate regulates leaf senescence and tolerance to cold stress: crosstalk with other phytohormones. J. Exp. Bot. 68, 1361–1369. doi: 10.1093/jxb/erx004
Hua, M. D., Senthi Kumar, R., Shyur, L. F., Cheng, Y. B., Tian, Z., Oelmüller, R., et al. (2017). Metabolomic compounds identified in Piriformospora indica-colonized Chinese cabbage roots delineate symbiotic functions of the interaction. Sci. Rep. 7:9291. doi: 10.1038/s41598-017-08715-2
Inahashi, H., Shelley, I. J., Yamauchi, T., Nishiuchi, S., Takahashi-Nosaka, M., Matsunami, M., et al. (2018). OsPIN2, which encodes a member of the auxin efflux carrier proteins, is involved in root elongation growth and lateral root formation patterns via the regulation of auxin distribution in rice. Physiol. Plant. doi: 10.1111/ppl.12707 [Epub ahead of print].
Jacobs, S., Zechmann, B., Molitor, A., Trujillo, M., Petutschnig, E., Lipka, V., et al. (2011). Broad-spectrum suppression of innate immunity is required for colonization of Arabidopsis roots by the fungus Piriformospora indica. Plant Physiol. 156, 726–740. doi: 10.1104/pp.111.176446
Jiang, C. J., Shimono, M., Sugano, S., Kojima, M., Liu, X., Inoue, H., et al. (2013). Cytokinins act synergistically with salicylic acid to activate defense gene expression in rice. Mol. Plant Microbe Interact. 26, 287–296. doi: 10.1094/MPMI-06-12-0152-R
Johnson, J. M., Reichelt, M., Vadassery, J., Gershenzon, J., and Oelmüller, R. (2014). An Arabidopsis mutant impaired in intracellular calcium elevation is sensitive to biotic and abiotic stress. BMC Plant Biol. 14:162. doi: 10.1186/1471-2229-14-162
Johnson, J. M., Sherameti, I., Nongbri, P. L., and Oelmüller, R. (2013). “Standardized conditions to study beneficial and nonbeneficial traits in the Piriformospora indica/Arabidopsis thaliana interaction,” in Piriformospora∗∗ indica. Soil Biology, Vol. 33, eds A. Varma, G. Kost, and R. Oelmüller (Heidelberg: Springer), 83–101. doi: 10.1007/978-3-642-33802-1_20
Johnson, J. M., Thürich, J., Petutschnig, E. K., Altschmied, L., Meichsner, D., Sherameti, I., et al. (2018). A poly(A) ribonuclease controls the cellotriose-based interaction between Piriformospora indica and its host Arabidopsis. Plant Physiol. 176, 2496–2514. doi: 10.1104/pp.17.01423
Jones, J. M., Clairmont, L., Macdonald, E. S., Weiner, C. A., Emery, R. J., and Guinel, F. C. (2015). E151 (sym15), a pleiotropic mutant of pea (Pisum sativum L.), displays low nodule number, enhanced mycorrhizae, delayed lateral root emergence, and high root cytokinin levels. J. Exp. Bot. 66, 4047–4059. doi: 10.1093/jxb/erv201
Kaldorf, M., Koch, B., Rexer, K. H., Kost, G., and Varma, A. (2005). Patterns of interaction between populus esch5 and Piriformospora indica: a transition from mutualism to antagonism. Plant Biol. 7, 210–218. doi: 10.1055/s-2005-837470
Kao, C. W., Bakshi, M., Sherameti, I., Dong, S., Reichelt, M., Oelmüller, R., et al. (2016). A Chinese cabbage (Brassica campetris subsp. Chinensis) τ-type glutathione-S-transferase stimulates Arabidopsis development and primes against abiotic and biotic stress. Plant Mol. Biol. 92, 643–659. doi: 10.1007/s11103-016-0531-2
Kazan, K. (2013). Auxin and the integration of environmental signals into plant root development. Ann. Bot. 112, 1655–1665. doi: 10.1093/aob/mct229
Khan, Z., and Doty, S. L. (2009). Characterization of bacterial endophytes of sweet potato plants. Plant Soil 322, 197–207. doi: 10.1007/s11104-009-9908-1
Khatabi, B., Molitor, A., Lindermayr, C., Pfiffi, S., Durner, J., von Wettstein, D., et al. (2012). Ethylene supports colonization of plant roots by the mutualistic fungus Piriformospora indica. PLoS One 7:e35502. doi: 10.1371/journal.pone.0035502
Khatabi, B., and Schäfer, P. (2012). Ethylene in mutualistic symbioses. Plant Signal. Behav. 7, 1634–1638. doi: 10.4161/psb.22471
Kim, D., Abdelaziz, M. E., Ntui, V. O., Guo, X., and Al-Babili, S. (2017). Colonization by the endophyte Piriformospora indica leads to early flowering in Arabidopsis thaliana likely by triggering gibberellin biosynthesis. Biochem. Biophys. Res. Commun. 490, 1162–1167. doi: 10.1016/j.bbrc.2017.06.169
Ko, D., Kang, J., Kiba, T., Park, J., Kojima, M., Do, J., et al. (2014). Arabidopsis ABCG14 is essential for the root-to-shoot translocation of cytokinin. Proc. Natl. Acad. Sci. U.S.A. 111, 7150–7155. doi: 10.1073/pnas.1321519111
Kopittke, P. M. (2016). Role of phytohormones in aluminium rhizotoxicity. Plant Cell Environ. 33, 2319–2328. doi: 10.1111/pce.12786
Kudoyarova, G. R., Melentiev, A. I., Martynenko, E. V., Timergalina, L. N., Arkhipova, T. N., Shendel, G. V., et al. (2014). Cytokinin producing bacteria stimulate amino acid deposition by wheat roots. Plant Physiol. Biochem. 83, 285–291. doi: 10.1016/j.plaphy.2014.08.015
Kunkel, B. N., and Harper, C. P. (2018). The roles of auxin during interactions between bacterial plant pathogens and their hosts. J. Exp. Bot. 69, 245–254. doi: 10.1093/jxb/erx447
Lahrmann, U., Strehmel, N., Langen, G., Frerigmann, H., Leson, L., Ding, Y., et al. (2015). Mutualistic root endophytism is not associated with the reduction of saprotrophic traits and requires a noncompromised plant innate immunity. New Phytol. 207, 841–857. doi: 10.1111/nph.13411
Lee, Y. C., Johnson, J. M., Chien, C. T., Sun, C., Cai, D., Lou, B., et al. (2011). Growth promotion of Chinese cabbage and Arabidopsis by Piriformospora indica is not stimulated by mycelium-synthesized auxin. Mol. Plant Microbe Interact. 24, 421–431. doi: 10.1094/MPMI-05-10-0110
Li, L., Chen, X., Ma, C., Wu, H., and Qi, S. (2016). Piriformospora indica requires kaurene synthase activity for successful plant colonization. Plant Physiol. Biochem. 102, 151–160. doi: 10.1016/j.plaphy.2016.02.017
Li, X., Brestic, M., Tan, D. X., Zivcak, M., Zhu, X., Liu, S., et al. (2017). Melatonin alleviates low PS I-limited carbon assimilation under elevated CO2 and enhances the cold tolerance of offspring in chlorophyll b-deficient mutant wheat. J. Pineal Res. 64, doi: 10.1111/jpi.12453
Liao, X., Lovett, B., Fang, W., and St Leger, R. J. (2017). Metarhizium robertsii produces indole-3-acetic acid, which promotes root growth in Arabidopsis and enhances virulence to insects. Microbiology 163, 980–991. doi: 10.1099/mic.0.000494
Lievens, L., Pollier, J., Goossens, A., Beyaert, R., and Staal, J. (2017). Abscisic acid as pathogen effector and immune regulator. Front. Plant Sci. 8:587. doi: 10.3389/fpls.2017.00587
Liu, J., Moore, S., Chen, C., and Lindsey, K. (2017). Crosstalk Complexities between auxin, cytokinin, and ethylene in Arabidopsis root development: from experiments to systems modeling, and back again. Mol. Plant 10, 1480–1496. doi: 10.1016/j.molp.2017.11.002
López-Ráez, J. A. (2016). How drought and salinity affect arbuscular mycorrhizal symbiosis and strigolactone biosynthesis? Planta 243, 1375–1385. doi: 10.1007/s00425-015-2435-9
Martín-Rodríguez, J. A., Huertas, R., Ho-Plágaro, T., Ocampo, J. A., Turečková, V., Tarkowská, D., et al. (2016). Gibberellin-abscisic acid balances during arbuscular mycorrhiza formation in tomato. Front. Plant Sci. 7:1273. doi: 10.3389/fpls.2016.01273
Martín-Rodríguez, J. Á., Ocampo, J. A., Molinero-Rosales, N., Tarkowská, D., Ruíz-Rivero, O., and García-Garrido, J. M. (2015). Role of gibberellins during arbuscular mycorrhizal formation in tomato: new insights revealed by endogenous quantification and genetic analysis of their metabolism in mycorrhizal roots. Physiol. Plant. 154, 66–81. doi: 10.1111/ppl.12274
Mathur, A., Koul, A., and Hattewar, J. (2018). Root colonization and fungicidal activities of the bacterial strains isolated from rhizospheric region of Zea mays: plant growth promotion activity, fungicidal activity and nematicidal activity of PGPRs extracts. J. Photochem. Photobiol. 180, 149–154.
Mbengue, M., Navaud, O., Peyraud, R., Barascud, M., Badet, T., Vincent, R., et al. (2016). Emerging trends in molecular interactions between plants and the broad host range fungal pathogens Botrytis cinerea and Sclerotinia sclerotiorum. Front. Plant Sci. 7:422. doi: 10.3389/fpls.2016.00422
Merzaeva, D. V., and Shirokikh, I. G. (2010). The production of auxins by the endophytic bacteria of winter rye. Appl. Biochem. Microbiol. 46, 44–50. doi: 10.1134/S0003683810010072
Miller, C. O. (1974). Detection and identification of cytokinins produced by Mycorrhizal Fungi. Plant Physiol. 54, 586–588. doi: 10.1104/pp.54.4.586
Miransari, M., Abrishamchi, A., Khoshbakht, K., and Niknam, V. (2014). Plant hormones as signals in arbuscular mycorrhizal symbiosis. Crit. Rev. Biotechnol. 34, 123–133. doi: 10.3109/07388551.2012.731684
Molitor, A., and Kogel, K. H. (2009). Induced resistance triggered by Piriformospora indica. Plant Signal. Behav. 4, 215–216. doi: 10.4161/psb.4.3.7814
Narayan, O. P., Verma, N., Singh, A. K., Oelmüller, R., Kumar, M., Prasad, D., et al. (2017). Antioxidant enzymes in chickpea colonized by Piriformospora indica participate in defense against the pathogen Botrytis cinerea. Sci. Rep. 7, 13553. doi: 10.1038/s41598-017-12944-w
Naser, V., and Shani, E. (2016). Auxin response under osmotic stress. Plant Mol. Biol. 91, 661–672. doi: 10.1007/s11103-016-0476-5
Ng, J. L., Perrine-Walker, F., Wasson, A. P., and Mathesius, U. (2015). The control of auxin transport in parasitic and symbiotic root–microbe interactions. Plants 4, 606–643. doi: 10.3390/plants4030606
Nishiyama, R., Watanabe, Y., Fujita, Y., Le, D. T., Kojima, M., Werner, T., et al. (2011). Analysis of cytokinin mutants and regulation of cytokinin metabolic genes reveals important regulatory roles of cytokinins in drought, salt and abscisic acid responses, and abscisic acid biosynthesis. Plant Cell 23, 2169–2183. doi: 10.1105/tpc.111.087395
Oelmüller, R., Sherameti, I., Tripathi, S., and Varma, A. (2009). Piriformospora indica: a novel multifunctional symbiotic fungus. Symbiosis 49, 1–18. doi: 10.1007/s13199-009-0009-y
Ortu, G., Balestrini, R., Pereira, P. A., Becker, J. D., Küster, H., and Bonfante, P. (2012). Plant genes related to gibberellin biosynthesis and signaling are differentially regulated during the early stages of AM fungal interactions. Mol. Plant 5, 951–954. doi: 10.1093/mp/sss027
Osugi, A., Kojima, M., Takebayashi, Y., Ueda, N., Kiba, T., and Sakakibara, H. (2017). Systemic transport of trans-zeatin and its precursor have differing roles in Arabidopsis shoots. Nat. Plants 3, 17112. doi: 10.1038/nplants.2017.112
Ouzari, H., Khsairi, A., Raddadi, N., Jaoua, L., Hassen, A., Zarrouk, M., et al. (2008). Diversity of auxin-producing bacteria associated to Pseudomonas savastanoi- induced olive knots. J. Basic Microbiol. 48, 370–377. doi: 10.1002/jobm.200800036
Pan, R., Xu, L., Wei, Q., Wu, C., Tang, W., Oelmüller, R., et al. (2017). Piriformospora indica promotes early flowering in Arabidopsis through regulation of the photoperiod and gibberellin pathways. PLoS One 12:e0189791. doi: 10.1371/journal.pone.0189791
Patil, V. (2011). Production of indole acetic acid by Azotobacter sp. Recent Res. Sci. Technol. 3, 14–16.
Patten, C. L., and Glick, B. R. (2002). Role of Pseudomonas putida indoleacetic acid in development of the host plant root system. Appl. Environ. Microbiol. 68, 3795–3801. doi: 10.1128/AEM.68.8.3795-3801.2002
Peck, S. C., and Kende, H. (1998). Differential regulation of genes encoding 1-aminocyclopropane-1-carboxylate (ACC) synthase in etiolated pea seedlings: effects of indole-3-acetic acid, wounding, and ethylene. Plant Mol. Biol. 38, 977–982. doi: 10.1023/A:1006033030081
Peskan-Berghöfer, T., Shahollari, B., Giong, P. H., Hehl, S., Markert, C., Blanke, V., et al. (2004). Association of Piriformospora indica with Arabidopsis thaliana roots represents a novel system to study beneficial plant-microbe interactions and involves early plant protein modifications in the endoplasmic reticulum and at the plasma membrane. Physiol. Plant. 122, 465–477. doi: 10.1111/j.1399-3054.2004.00424.x
Peskan-Berghöfer, T., Vilches-Barro, A., Müller, T. M., Glawischnig, E., Reichelt, M., Gershenzon, J., et al. (2015). Sustained exposure to abscisic acid enhances the colonization potential of the mutualist fungus Piriformospora indica on Arabidopsis thaliana roots. New Phytol. 208, 873–886. doi: 10.1111/nph.13504
Pimprikar, P., Carbonnel, S., Paries, M., Katzer, K., Klingl, V., Bohmer, M. J., et al. (2016). CCaMK-CYCLOPS-DELLA complex activates transcription of RAM1 to regulate arbuscule branching. Curr. Biol. 26, 987–998. doi: 10.1016/j.cub.2016.01.069
Podlešáková, K., Fardoux, J., Patrel, D., Bonaldi, K., Novák, O., Strnad, M., et al. (2013). Rhizobial synthesized cytokinins contribute to but are not essential for the symbiotic interaction between photosynthetic Bradyrhizobia and Aeschynomene legumes. Mol. Plant Microbe Interact. 205, 1431–1436. doi: 10.1111/nph.13252
Pozo, M. J., López-Ráez, J. A., Azcón-Aguilar, C., and García-Garrido, J. M. (2015). Phytohormones as integrators of environmental signals in the regulation of mycorrhizal symbioses. Mol. Plant Microbe Interact. 26, 1232–1238. doi: 10.1094/MPMI-03-13-0076-R
Rai, M., Rathod, D., Agarkar, G., Dar, M., Brestic, M., Pastore, G. M., et al. (2014). Fungal growth promotor endophytes, a pragmatic approach towards sustainable food and agriculture. Symbiosis 62, 63–79. doi: 10.1007/s13199-014-0273-3
Raudaskoski, M., and Kothe, E. (2015). Novel findings on the role of signal exchange in arbuscular and ectomycorrhizal symbioses. Mycorrhiza 25, 243–252. doi: 10.1007/s00572-014-0607-2
Reineke, G., Heinze, B., Schirawski, J., Buettner, H., Kahmann, R., and Basse, C. W. (2008). Indole-3-acetic acid (IAA) biosynthesis in the smut fungus Ustilago maydis and its relevance for increased IAA levels in infected tissue and host tumour formation. Mol. Plant Pathol. 9, 339–355. doi: 10.1111/j.1364-3703.2008.00470.x
Robert-Seilaniantz, A., Grant, M., and Jones, J. D. (2011). Hormone crosstalk in plant disease and defense: more than just jasmonate-salicylate antagonism. Annu. Rev. Phytopathol. 49, 317–343. doi: 10.1146/annurev-phyto-073009-114447
Rodriguez, C., Mayo, J. C., Sainz, R. M., Antolín, I., Herrera, F., Martín, V., et al. (2010). Regulation of antioxidant enzymes, a significant role for melatonin. J. Pineal Res. 36, 1–9. doi: 10.1046/j.1600-079X.2003.00092.x∗∗ doi: 10.1046/j.1600-079X.2003.00092.x
Roy, B. D., Deb, B., and Sharma, G. D. (2010). Role of acetic acid bacteria in biological nitrogen fixation. Biofrontiers 1, 83–98.
Salomon, M. V., Bottini, R., de Souza Filho, G. A., Cohen, A. C., Moreno, D., Gil, M., et al. (2014). Bacteria isolated from roots and rhizosphere of Vitis vinifera retard water losses, induce abscisic acid accumulation and synthesis of defense-related terpenes in in vitro cultured grapevine. Physiol. Plant. 151, 359–374. doi: 10.1111/ppl.12117
Sánchez-López,Á. M., Baslam, M., De Diego, N., Muñoz, F. J., Bahaji, A., Almagro, G., et al. (2016). Volatile compounds emitted by diverse phytopathogenic microorganisms promote plant growth and flowering through cytokinin action. Plant Cell Environ. 39, 2592–2608. doi: 10.1111/pce.12759
Sasaki, T., Suzaki, T., Soyano, T., Kojima, M., Sakakibara, H., and Kawaguchi, M. (2014). Shoot-derived cytokinins systemically regulate root nodulation. Nat. Commun. 5, 4983. doi: 10.1038/ncomms5983
Schäfer, P., Pfiffi, S., Voll, L. M., Zajic, D., Chandler, P. M., Waller, F., et al. (2009a). Manipulation of plant innate immunity and gibberellin as factor of compatibility in the mutualistic association of barley roots with Piriformospora indica. Plant J. 59, 461–474. doi: 10.1111/j.1365-313X.2009.03887.x
Schäfer, P., Pfiffi, S., Voll, L. M., Zajic, D., Chandler, P. M., Waller, F., et al. (2009b). Phytohormones in plant root-Piriformospora indica mutualism. Plant Signal. Behav. 4, 669–671.
Shaharoona, B., Arshad, M., and Zahir, Z. A. (2006). Effect of plant growth promoting rhizobacteria containing ACC-deaminase on maize (Zea mays L.) growth under axenic conditions and on nodulation in mung bean (Vigna radiata L.). Lett. Appl. Microbiol. 42, 155–159. doi: 10.1111/j.1472-765X.2005.01827.x
Shen, H., Chen, J., Wang, Z., Yang, C., Sasaki, T., Yamamoto, Y., et al. (2006). Root plasma membrane H+-ATPase is involved in the adaptation of soybean to phosphorus starvation. J. Exp. Bot. 57, 1353. doi: 10.1093/jxb/erj111
Shen, Q., Liu, Y., and Naqvi, N. I. (2018). Fungal effectors at the crossroads of phytohormone signaling. Curr. Opin. Microbiol. 46, 1–6. doi: 10.1016/j.mib.2018.01.006
Singh, L. P., Gill, S. S., and Tuteja, N. (2011). Unraveling the role of fungal symbionts in plant abiotic stress tolerance. Plant Signal. Behav. 6, 175–191. doi: 10.4161/psb.6.2.14146
Sirrenberg, A., Göbel, C., Grond, S., Czempinski, N., Ratzinger, A., Karlovsky, P., et al. (2007). Piriformospora indica affects plant growth by auxin production. Physiol. Plant. 131, 581–589. doi: 10.1111/j.1399-3054.2007.00983.x
Splivallo, R., Fischer, U., Göbel, C., Feussner, I., and Karlovsky, P. (2009). Truffles regulate plant root morphogenesis via the production of auxin and ethylene. Plant Physiol. 150, 2018–2029. doi: 10.1104/pp.109.141325
Stec, N., Banasiak, J., and Jasiński, M. (2016). Abscisic acid - an overlooked player in plant-microbe symbioses formation? Acta Biochim. Pol. 63, 53–58. doi: 10.18388/abp.2015-1210
Stein, E., Molitor, A., Kogel, K. H., and Waller, F. (2008). Systemic resistance in Arabidopsis conferred by the mycorrhizal fungus Piriformospora indica requires jasmonic acid signaling and the cytoplasmic function of NPR1. Plant Cell Physiol. 49, 1747–1751. doi: 10.1093/pcp/pcn147
Stringlis, I. A., Proietti, S., Hickman, R., Van Verk, M. C., Zamioudis, C., and Pieterse, C. M. J. (2017). Root transcriptional dynamics induced by beneficial rhizobacteria and microbial immune elicitors reveal signatures of adaptation to mutualists. Plant J. 93, 166–180. doi: 10.1111/tpj.13741
Sukumar, P., Legué, V., Vayssières, A., Martin, F., Tuskan, G. A., and Kalluri, U. C. (2013). Involvement of auxin pathways in modulating root architecture during beneficial plant-microorganism interactions. Plant Cell Environ. 36, 909–919. doi: 10.1111/pce.12036
Sun, C., Shao, Y., Vahabi, K., Lu, J., Bhattacharya, S., Dong, S., et al. (2014). The beneficial fungus Piriformospora indica protects Arabidopsis from Verticillium dahliae infection by downregulation plant defense responses. BMC Plant Biol. 14:268. doi: 10.1186/s12870-014-0268-5
Takeda, N., Handa, Y., Tsuzuki, S., Kojima, M., Sakakibara, H., and Kawaguchi, M. (2015a). Gibberellins interfere with symbiosis signaling and gene expression and alter colonization by arbuscular mycorrhizal fungi in Lotus japonicus. Plant Physiol. 167, 545–557. doi: 10.1104/pp.114.247700
Takeda, N., Handa, Y., Tsuzuki, S., Kojima, M., Sakakibara, H., and Kawaguchi, M. (2015b). Gibberellin regulates infection and colonization of host roots by arbuscular mycorrhizal fungi. Plant Signal. Behav. 10, e1028706. doi: 10.1080/15592324.2015.1028706
Tian, H., Lv, B., Ding, T., Bai, M., and Ding, Z. (2017). Auxin-BR interaction regulates plant growth and development. Front. Plant Sci. 8:2256. doi: 10.3389/fpls.2017.02256
Tognetti, V. B., Bielach, A., and Hrtyan, M. (2017). Redox regulation at the site of primary growth: auxin, cytokinin and ROS crosstalk. Plant Cell Environ. 40, 2586–2605. doi: 10.1111/pce.13021
Tudela, D., and Primo-Millo, E. (1992). 1-Aminocyclopropane-1-Carboxylic acid transported from roots to shoots promotes leaf abscission in Cleopatra Mandarin (Citrus reshni Hort. ex Tan.) seedlings rehydrated after water stress. Plant Physiol. 100, 131–137. doi: 10.1104/pp.100.1.131
Vadassery, J., Ritter, C., Venus, Y., Camehl, I., Varma, A., Shahollari, B., et al. (2008). The role of auxins and cytokinins in the mutualistic interaction between Arabidopsis and Piriformospora indica. Mol. Plant Microbe Interact. 21, 1371–1383. doi: 10.1094/MPMI-21-10-1371
Vahabi, K., Camehl, I., Sherameti, I., and Oelmüller, R. (2013). Growth of Arabidopsis seedlings on high fungal doses of Piriformospora indica has little effect on plant performance, stress, and defense gene expression in spite of elevated jasmonic acid and jasmonic acid-isoleucine levels in the roots. Plant Signal. Behav. 8:e26301. doi: 10.4161/psb.26301
Vahabi, K., Dorcheh, S. K., Monajembashi, S., Westermann, M., Reichelt, M., Falkenberg, D., et al. (2016). Stress promotes Arabidopsis - Piriformospora indica interaction. Plant Signal. Behav. 11:e1136763. doi: 10.1080/15592324.2015.1136763
Vahabi, K., Reichelt, M., Scholz, S. S., Furch, A. C. U., Matsuo, M., Johnson, J. M., et al. (2018). Alternaria brassicae induces systemic jasmonate responses in Arabidopsis which travel to neighboring plants via a Piriformsopora indica hyphal network after conversion to an absicsic acid response. Front. Plant Biol. 8:626. doi: 10.3389/fpls.2018.00626
Vahabi, K., Sherameti, I., Bakshi, M., Mrozinska, A., Ludwig, A., and Reichelt, M. (2015). The interaction of Arabidopsis with Piriformospora indica shifts from initial transient stress induced by fungus-released chemical mediators to a mutualistic interaction after physical contact of the two symbionts. BMC Plant Biol. 15:58. doi: 10.1186/s12870-015-0419-3
Varma, A., Sherameti, I., Tripathi, S., Prasad, R., Das, A., Sharma, M., et al. (2012). The symbiotic fungus Piriformospora indica: review. Mycota Fungal Assoc. 9, 231–254. doi: 10.1007/978-3-642-30826-0-13
Verhagen, B. W., Glazebrook, J., Zhu, T., Chang, H. S., van Loon, L. C., and Pieterse, C. M. (2004). The transcriptome of rhizobacteria-induced systemic resistance in arabidopsis. Mol. Plant Microbe Interact. 17, 895–908. doi: 10.1094/MPMI.2004.17.8.895
Verma, S., Varma, A., Rexer, K. H., Hassel, A., Kost, G., Sarbhoy, A., et al. (1998). Piriformospora indica, gen. et sp. nov., a new root-colonizing fungus. Mycologia 5, 896–903. doi: 10.2307/3761331
Viterbo, A., Landau, U., Kim, S., Chernin, L., and Chet, I. (2010). Characterization of ACC deaminase from the biocontrol and plant growth-promoting agent Trichoderma asperellum T203. Fems Microbiol. Lett. 305, 42–48. doi: 10.1111/j.1574-6968.2010.01910.x
Wang, K., Guo, Q., Froehlich, J. E., Hersh, H. L., Zienkiewicz, A., Howe, G. A., et al. (2018). Two abscisic acid-responsive plastid lipase genes involved in jasmonic acid biosynthesis in Arabidopsis thaliana. Plant Cell 30, 1006–1022. doi: 10.1105/tpc.18.00250
Wang, L., Zhao, Y., Reiter, R. J., He, C., Liu, G., Lei, Q., et al. (2014). Changes in melatonin levels in transgenic ’Micro-Tom’ tomato overexpressing ovine AANAT and ovine HIOMT genes. J. Pineal Res. 56, 134–142. doi: 10.1111/jpi.12105
Yang, Y. X., Ahammed, G. J., Wu, C., Fan, S. Y., and Zhou, Y. H. (2015). Crosstalk among jasmonate, salicylate and ethylene signaling pathways in plant disease and immune responses. Curr. Protein Pept. Sci. 16, 450–461. doi: 10.2174/1389203716666150330141638
Ye, W., Shen, C. H., Lin, Y., Chen, P. J., Xu, X., Oelmüller, R., et al. (2014). Growth promotion-related miRNAs in Oncidium orchid roots colonized by the endophytic fungus Piriformospora indica. PLoS One 9:e84920. doi: 10.1371/journal.pone.0084920
Yu, N., Luo, D., Zhang, X., Liu, J., Wang, W., Jin, Y., et al. (2014). A DELLA protein complex controls the arbuscular mycorrhizal symbiosis in plants. Cell Res. 24, 130–133. doi: 10.1038/cr.2013.167
Zhang, L. J., Jia, J. F., Xu, Y., Wang, Y., Hao, J., Li, T., et al. (2012). Production of transgenic Nicotiana sylvestris plants expressing melatonin synthetase genes and their effect on UV-B-induced DNA damage. Vitro Cell. Dev. Plant 48, 275–282. doi: 10.1007/s11627-011-9413-0
Zhang, N., Sun, Q., Zhang, H., Cao, Y., Weeda, S., Ren, S., et al. (2015). Roles of melatonin in abiotic stress resistance in plants. J. Exp. Bot. 66, 647. doi: 10.1093/jxb/eru336
Zhang, P. J., Huang, F., Zhang, J. M., Wei, J. N., and Lu, Y. B. (2015). The mealybug Phenacoccus solenopsis suppresses plant defense responses by manipulating JA-SA crosstalk. Sci. Rep. 5:9354. doi: 10.1038/srep09354
Zhang, W., Wang, J., Xu, L., Wang, A., Huang, L., Du, H., et al. (2018). Drought stress responses in maize are diminished by Piriformospora indica. Plant Signal. Behav. 13:e1414121. doi: 10.1080/15592324.2017.1414121
Zhang, Y., von Behrens, I., Zimmermann, R., Ludwig, Y., Hey, S., and Hochholdinger, F. (2015). LATERAL ROOT PRIMORDIA 1 of maize acts as a transcriptional activator in auxin signalling downstream of the Aux/IAA gene rootless with undetectable meristem 1. J. Exp. Bot. 66, 3855–3863. doi: 10.1093/jxb/erv187
Keywords: phytohormones, Piriformospora indica, growth promotion, stress tolerance, root–microbe interaction, signal transduction
Citation: Xu L, Wu C, Oelmüller R and Zhang W (2018) Role of Phytohormones in Piriformospora indica-Induced Growth Promotion and Stress Tolerance in Plants: More Questions Than Answers. Front. Microbiol. 9:1646. doi: 10.3389/fmicb.2018.01646
Received: 26 February 2018; Accepted: 02 July 2018;
Published: 31 July 2018.
Edited by:
Brigitte Mauch-Mani, University of Neuchâtel, SwitzerlandReviewed by:
Marian Brestic, Slovak University of Agriculture, SlovakiaJoy Michal Johnson, Kerala Agricultural University, India
Copyright © 2018 Xu, Wu, Oelmüller and Zhang. This is an open-access article distributed under the terms of the Creative Commons Attribution License (CC BY). The use, distribution or reproduction in other forums is permitted, provided the original author(s) and the copyright owner(s) are credited and that the original publication in this journal is cited, in accordance with accepted academic practice. No use, distribution or reproduction is permitted which does not comply with these terms.
*Correspondence: Wenying Zhang, d3l6aGFuZ0B5YW5ndHpldS5lZHUuY24= Ralf Oelmüller, cmFsZi5vZWxtdWVsbGVyQHVuaS1qZW5hLmRl