- 1College of Bioengineering, Chongqing University, Chongqing, China
- 2National Engineering Research Center of Immunological Products, Department of Microbiology and Biochemical Pharmacy, College of Pharmacy, Third Military Medical University, Chongqing, China
- 3Department of Critical Care Medicine, Children’s Hospital of Chongqing Medical University, Chongqing, China
Pseudomonas aeruginosa is a formidable pathogen that causes infections with high mortality rates. Because of its ability to form biofilms and rapidly acquire resistance to many first-line antibiotics, P. aeruginosa-related infections are typically difficult to cure by traditional antibiotic treatment regimes. Thus, new strategies to prevent and treat such infections are urgently required. PA0833 is a newly identified protective antigen of P. aeruginosa that was identified in a screen using a reverse vaccine strategy in our laboratory. In this study, we further confirmed its protective efficacy in murine sepsis and pneumonia models. Immunization with PA0833 induced strong immune responses and resulted in reduced bacterial loads; decreased pathology, inflammatory cytokine expression and inflammatory cell infiltration; and improved survival. Furthermore, PA0833 was identified as an OmpA C-like protein by bioinformatics analysis and biochemical characterization and shown to contribute to bacterial environmental stress resistance and virulence. These results demonstrate that PA0833 is an OmpA C-like protein that induces a protective immune response in mice, indicating that PA0833 is a promising antigen for vaccine development.
Introduction
Pseudomonas aeruginosa (P. aeruginosa) is a leading cause of hospital-acquired infections (Kalil et al., 2016), especially in patients with cystic fibrosis or compromised host defense mechanisms (Nathwani et al., 2014; Kizny Gordon et al., 2017). P. aeruginosa can infect any part of the body, and serious P. aeruginosa infections, such as infections of the blood, pneumonia, and infections following surgery, are typically complicated and can be life-threatening (Gellatly and Hancock, 2013). These infections are generally treated with antibiotics (Curran et al., 2017). Unfortunately, because of increasing antibiotic resistance and the ability of P. aeruginosa to form biofilms, such infections are becoming more difficult to treat, imposing a high and increasing burden on health care resources (Melsen et al., 2013; Ramirez-Estrada et al., 2016).
Vaccination strategies to prevent P. aeruginosa infection have attracted a great deal of attention. In the past two decades, a number of different vaccines and several monoclonal antibodies have been developed for active and passive vaccination against P. aeruginosa infection (Pier, 2005; Priebe and Goldberg, 2014; Vincent, 2014; Grimwood et al., 2015; Le Moigne et al., 2016). The antigens used in these studies include lipopolysaccharides (Pier, 2007), surface polysaccharides (Pier, 2000), outer membrane proteins (Gao et al., 2017; Rello et al., 2017), secreted proteins (Hamaoka et al., 2017; Yang et al., 2017), flagella (Hassan et al., 2017), and pili (Ohama et al., 2006; Banadkoki et al., 2016). Several vaccine candidates even entered phase II/III clinical trials (Baumann et al., 2004; Pier, 2005; Sharma et al., 2011; Le Moigne et al., 2016; Rello et al., 2017). However, these efforts have not resulted in a marketable product to date, which can be attributed to two primary reasons. First, P. aeruginosa utilizes multiple virulence factors and pathways to establish a successful infection, and immunization with a single antigen is able to provide only partial protection (Gellatly and Hancock, 2013; Curran et al., 2017). Second, it is unclear what type of immune response should be induced by an effective P. aeruginosa vaccine to clear an infection (Worgall, 2012). Thus, more effort should be focused on studying the pathogenic mechanisms of P. aeruginosa and identifying new candidate antigens.
Our lab has been working on developing a P. aeruginosa vaccine since 2011, and we have identified several novel candidate antigens, such as PA0833, PA5505, PA4110, and PA0807. PA0833 is a hypothetical, uncharacterized protein that is 237 amino acids in length and has an unclear structure and function. Only one study (Choi et al., 2011) has demonstrated that PA0833 is a component of outer membrane vesicles secreted by P. aeruginosa. In the present study, we observed that PA0833 is a promising candidate antigen for vaccine development. It exhibited strong immunogenicity in mice and was able to protect mice against a P. aeruginosa challenge in both sepsis and acute lung infection models. Further bioinformatics prediction and biochemical studies indicated that PA0833 has characteristics of the C-terminal domain of OmpA family proteins and that it may be an OmpA family protein. Moreover, this protein may be involved in the pathogenesis of the bacterium by affecting the synthesis of alginate. These results will be helpful for understanding the biological function of PA0833 and enabling future studies of the pathogenic mechanism of this protein.
Materials and Methods
Ethics Statement
All animal care and use protocols in this study were performed in accordance with the Regulations for the Administration of Affairs Concerning Experimental Animals approved by the State Council of the People’s Republic of China. All animal experiments in this study were approved by the Animal Ethical and Experimental Committee of the Third Military Medical University (Chongqing, Permit No. 2011-04) in accordance with their rules and regulations. All surgery was performed under sodium pentobarbital anesthesia, and all efforts were made to minimize suffering.
Bacterial Strains and Culture Methods
The P. aeruginosa standard strain PAO1 was purchased from ATCC (Manassas, VA, United States). Bacterial strains were cultured in Luria Bertani (LB) broth (Difco Laboratories, United States), washed and diluted with sterile phosphate buffer solution (PBS) to an appropriate cell concentration determined spectrophotometrically at 600 nm (OD600).
Animals
The 8–12-week-old female BALB/c mice used in this study were purchased from Beijing HFK Bioscience Limited Company (Beijing, People’s Republic of China). Mice were matched for age and sex and were housed under specific pathogen-free (SPF) conditions. All mice were randomly assigned to treatment groups using a randomization tool implemented in MS Excel. Female New Zealand white rabbits (2.00 ± 0.20 kg) were provided by TengXin Company (Chongqing, China).
Bioinformatics Prediction of PA0833
SignalP 4.0 (Petersen et al., 2011), TMHMM 2.0 (Chen et al., 2003), CFSSP, Phyre2 (Kelley et al., 2015), and TBBprep (Natt et al., 2004) were used to predict the possible signal peptide, trans-membrane domain, secondary structure and homologous proteins of PA0833, respectively. The structure of PA0833 was modeled by the automated protein homology-modeling server SWISS-MODEL (Arnold et al., 2006). Sequence alignment was performed using the online software ClusterW at http://www.ebi.ac.uk/Tools/msa/clustalw2/.
Cloning and Expression of Recombinant PA0833
Two constructs were made to produce truncated PA0833 proteins comprising residues 26–237 and 86–237. In brief, the coding sequences of the two constructs were amplified by PCR from P. aeruginosa strain PAO1 genomic DNA and cloned into the pGEX6p-2 vector (Novagen, Italy) via the BamH1/XhoI restriction sites. The inserts were sequenced and determined to be in complete agreement with the expected sequences. The resulting proteins harbor an N-terminal glutathione S-transferases (GST) tag to facilitate the purification of the proteins. Escherichia coli strain BL21 (DE3) competent cells (Tiangen Biotech, China) were transformed with the recombinant plasmids, and isopropyl-β-d-thiogalactopyranoside (IPTG) was then added to a final concentration of 0.05 mM to induce the expression of recombinant protein at 16°C overnight.
Purification and Characterization of Recombinant PA0833
GST-tagged proteins were harvested from cleared lysates with glutathione-Sepharose, and the GST tag was cleaved using PreScission Protease (GE Healthcare, United States). Next, the recombinant proteins were purified by hydrophobic chromatography using a 5 ml phenyl HP column. Finally, the proteins were desalted with PBS and loaded onto a 5 ml Q HP column to remove endotoxins. The purity and concentration of the proteins was determined by sodium dodecyl sulfate-polyacrylamide gel electrophoresis (SDS–PAGE) and the bicinchoninic acid (BCA) Protein Assay Kit (Thermo scientific, United States), respectively. The endotoxin content after the proteins were purified was detected using the kinetic turbidimetric tachypleus amoebocyte lysate assay (Houshiji cod Inc., Xiamen, China), and the endotoxin of the two recombinant proteins were at acceptable levels (<2.5 pg/μg).
The oligomeric states of PA083386-237 were analyzed by using a SuperdexTM 200 10/300GL column as described previously (Zhang et al., 2013). Gel Filtration Calibration Kits (GE Healthcare, United States) was used to generate the calibration curve, 200 μl purified PA083386-237 was loaded onto the column, and the elution volume of the corresponding peak was used to calculate the molecular weight, thereby determining the oligomeric state of the protein.
PA083386-237 were further analyzed by chemical cross-linking analysis following the protocol described by Fadouloglou (Fadouloglou et al., 2008). In brief, PA083386-237 or bovine serum albumin (BSA) was diluted to 1 mg/ml and incubated with glutaraldehyde at 37°C for 30 min. The final concentration of glutaraldehyde in each reaction was 0.01, 0.05, 0.1, 0.2, 0.3, 0.4, and 0.5%. The cross-linking reaction was then terminated by adding loading buffer containing SDS and glycine. The protein samples were then analyzed by SDS–PAGE.
Peptidoglycan Binding Assay
The peptidoglycan binding assay was performed as described previously with minimal modifications (Wang et al., 2016). First, 50, 100, 150 or 200 μg of commercial insoluble peptidoglycan (InvivoGen, United States) was incubated with PA083386-237 (0.5 mg/ml) or BSA (0.5 mg/ml) in 10 mM sodium phosphate buffer with 50 mM NaCl and pH 7.5 for 1 h at room temperature. After the mixtures had been centrifuged for 20 min 12,000 g, the supernatants were collected, and the precipitates were washed three times with 10 mM sodium phosphate buffer (with 500 mM NaCl, pH 7.5). Finally, the precipitates were resuspended in sodium phosphate buffer. The supernatant and precipitate fractions were analyzed by western blotting using rabbit anti-PA083386-237 or anti-BSA polyclonal antibodies as the primary antibodies. The anti-PA083386-237 polyclonal antibodies (pcAb) were generated in rabbits based on a previously published method (Zhang et al., 2015).
Immunization of Mice
Purified PA083326-237 in Histidine (His) buffer (10 mM His, 150 mM NaCl, pH 6.0) was emulsified 1:1 (v/v) in Al(OH)3 (Pierce, United States). Mice were intramuscularly injected with 200 μl of the emulsion containing 30 μg protein, His buffer plus adjuvant, or His buffer alone as the control on days 0, 14, and 21, and the mice were infected on day 35.
ELISA
On day 28 after primary immunization, mice were exsanguinated, and serum samples were collected for an enzyme-linked immunosorbent assay (ELISA). Microtiter plate wells (Corning Incorporated, United States) were coated with PA083326-237 (200 ng per well) in 0.05 M carbonate buffer (pH 9.5) overnight at 4°C. Diluted serum samples were used as the primary antibodies, and the secondary antibodies were horseradish peroxidase (HRP)-conjugated goat anti-mouse IgG, anti-mouse IgG1, anti-mouse IgG2a or anti-mouse IgG2b (Sigma). The optical density at 450 nm was measured, and the titers were defined as the highest dilution that yielded an absorbance value of more than twice the value of the pre-immune serum.
P. aeruginosa Sepsis Mouse Model
To measure the survival rates of mice in the P. aeruginosa sepsis model, immunized BALB/c mice were intravenously infected with PAO1 [7.0 × 107 colony-forming units (CFUs)] on day 35 and monitored for survival for 14 days after infection. For bacterial burdens in the sepsis model, a sub-lethal dose (3.5 × 107 CFUs) of PAO1 was intravenously administered to each mouse. Livers and spleens from all immunized mice were removed, weighed, and homogenized in 1 ml of PBS 1 or 3 days after infection. The peripheral blood was collected in heparin anticoagulant tubes. All samples were then plated on LB plates at a 10-fold serial dilution and cultured at 37°C for 20 h. The number of CFUs per gram of tissue (CFUs/g) was calculated from each plate.
P. aeruginosa Pneumonia Mouse Model
In the acute pneumonia model, mice in each group were anesthetized with pentobarbital sodium followed by intratracheal injection with a lethal dose of PAO1 (1.0 × 107 CFUs) to measure the survival rates. The number of deaths in each group was recorded every 12 h over a 7-day observation period post challenge. For bacterial burdens, histopathology, inflammatory cells and cytokine analyses, mice were infected by intratracheal injection with 5.0 × 106 CFUs of PAO1. Next, the lung tissues were collected, weighed, and homogenized in 1 ml of sterilized PBS buffer 24 h after infection to determine CFUs.
Histological Analysis
Lung tissues were collected from the pneumonia model mice 24 h post-infection, inflated, and fixed in 10% neutral buffered formalin. All lung samples were embedded in paraffin, sectioned, and stained with hematoxylin and eosin (HE). The sections were then viewed at 200 × magnifications by a single pathologist who was blinded to the study groups. Each lung section was given a score of 0–4 (no abnormality to most severe) according to established criteria based on hyperemia, edema, hemorrhage, and neutrophil infiltration (Zuo et al., 2013).
Evaluation of Inflammation
To quantify the neutrophils’ infiltration of lungs in the pneumonia model, cells in bronchoalveolar lavage fluid (BALF) from mice 24 h post challenge were collected and stained using the following antibodies: PE/Cy7 anti-mouse CD45 and APC/cy7 anti-mouse Ly-6G (Biolegend Inc., United States). Samples were then analyzed using BD FACSArray software on a BD FACS Array flow cytometer (BD Biosciences).
To quantify proinflammatory responses, cytokines such as TNF-α, IL-1β, and IL-6 in BALF were collected 24 h after infection. The concentrations of proinflammatory cytokines were determined using a Mouse Quantikine ELISA kit for TNF-α, IL-1β or IL-6 (R&D Systems, United States) according to the manufacturer’s instructions.
Construction of PAO1 Isogenic Mutants
The PA0833 knockout strain (PAO1/ΔPA0833) was constructed using the suicide vector pCVD442 (Liu et al., 2009). Briefly, the primers were designed to amplify the gentamicin resistance (GmR) gene from the plasmid pJQ200SK (primers P1 and P2 in Table 1, PCR conditions in Supplementary Table S1) and amplify the 5′- and 3′-regions of PA0833, including flanking sequences (primers P3/P4 and P5/P6 in Table 1, PCR conditions in Supplementary Table S2). The three fragments were joined (primers P3 and P6 in Table 1, PCR conditions in Supplementary Table S3) and cloned into the suicide plasmid pCVD442 to yield the plasmid pCVD442-ΔPA0833::GmR, which was then transformed into E. coli β2155 (Sangon Biotech, China), and then positive clones were selected on LB agar containing ampicillin (100 μg/ml), gentamicin (25 μg/ml) and diaminopimelic acid (DAP, 0.5 mM). Next, conjugation between the recipient PAO1 and the donor β2155/pCVD442-ΔPA0833::GmR was performed to transfer the recombinant suicide plasmid pCVD442-ΔPA0833::GmR from β2155 to PAO1. Gentamicin- and sucrose-resistant colonies were selected and screened by PCR using primers P7 and P8, as shown in Table 1. These primers yielded fragments of 2490 and 2695 bp for the intact and mutated GmR gene, respectively. To further characterize the GmR gene in one of the selected mutant strains, primers P9 and P10 (shown in Table 1) were used for its amplification and confirmation by DNA sequencing (Sangon Biotech, China).
To construct PAO1/ΔPA0833 strain complemented with PA0833 (PAO1/CPA0833), plasmid pDN18-PA0833 was constructed by cloning PA0833 gene into the EcoRI/ HindIII site of the broad-host-range vector pDN18 (Bai et al., 2007). This PA0833 gene was amplified by PCR using primers P11 and P12 (Table 1) from the chromosomal DNA of PAO1. Resulting plasmid with the PA0833 gene was electroporated into the PAO1/ΔPA0833 strain to generate the strain PAO1/CPA0833. The expression of PA0833 was detected by indirect immunofluorescence, which was carried out based on a method established by us previously (Zhang et al., 2015).
Analysis of PAO1 Mutant Resistance to Environmental Stress
The growth rate of wild-type PAO1 (PAO1/WT), PAO1/ΔPA0833 and PAO1/CPA0833 was recorded prior to the initiation of experiments. For the acid survival analysis (Wang et al., 2016), bacteria in the exponential growth phase were harvested and adjusted to 3.0 × 107 CFUs/ml in PBS. Next, 1/10th of the bacterial suspension was mixed with LB containing 60 mM acetic acid (pH 4.0) and incubated for 150 min at 37°C, with samples taken bacterial enumeration every 30 min. The relative growth index is the ratio of the number of bacteria after growing in acidic conditions relative to their starting point.
For the SDS resistance assay (Wang et al., 2016), bacteria in the exponential growth phase were harvested and adjusted to 1.0 × 109 CFUs/ml in fresh LB. Next, 1/100th of the bacterial suspension was inoculated in LB containing 9% SDS and incubated for 8 h at 37°C. Samples were taken for determining the absorbance at 600 nm every hour.
Analysis of the Expression of PA0833 in the GEO Profiles Database
The GEO database stores gene expression profiles derived from curated GEO DataSets. Each profile is presented as a chart that displays the expression level of one gene across all samples within a DataSet. To gain insight into differential expression patterns of PA0833 across different experimental conditions, the PA0833 gene was analyzed using the GEO profiles database1.
Statistical Analysis
Data are presented as the means ± standard deviation (SD) or the means ± standard error of the mean (SEM). Scoring experiments were performed in a blind manner. Survival data were analyzed using Kaplan-Meier survival curves. To calculate P-values, non-parametric Mann–Whitney tests, log-rank tests, Student’s t-tests, or one-way ANOVA with Bonferroni correction were used depending on sample distribution and variation as described in the figure legends. SPSS 13.0 (SPSS Inc., United States) and GraphPad Prism 6.0 (GraphPad Software, Inc., United States) were used to perform statistical analyses. Significance was accepted at P < 0.05.
Results
PA0833 Stimulates Protective Immunity in a P. aeruginosa Sepsis Model
We first tested the immunogenicity of PA0833 in mice. After the last booster immunization, the levels of total IgG and IgG subgroups (IgG1, IgG2a, and IgG2b) in the mice immunized with PA0833 were determined by ELISA. As shown in Figure 1A, the geometric mean titer of total IgG was approximately 216, which confirmed that PA0833 is a powerful immunogen. Meanwhile, the level of antigen-specific IgG1 was approximately 32 times higher than that of IgG2a (Figure 1A). Since IgG1 and IgG2a are markers for Th2 and Th1 responses, respectively, this result suggested that a Th2-biased response was induced upon immunization with PA0833. We next tested the protective efficacy of PA0833 in a P. aeruginosa sepsis model. Two weeks after the last immunization, mice were challenged with a lethal dose of PAO1. As shown in Figure 1B, at the end of the observation period, 7 out of 10 mice in the PA0833 formulated with Al(OH)3 group survived the PAO1 challenge, which was significantly higher than that in the His buffer control group (P = 0.0095) and adjuvant control group (P = 0.0215), indicating that PA0833 stimulated protective immunity in mice.
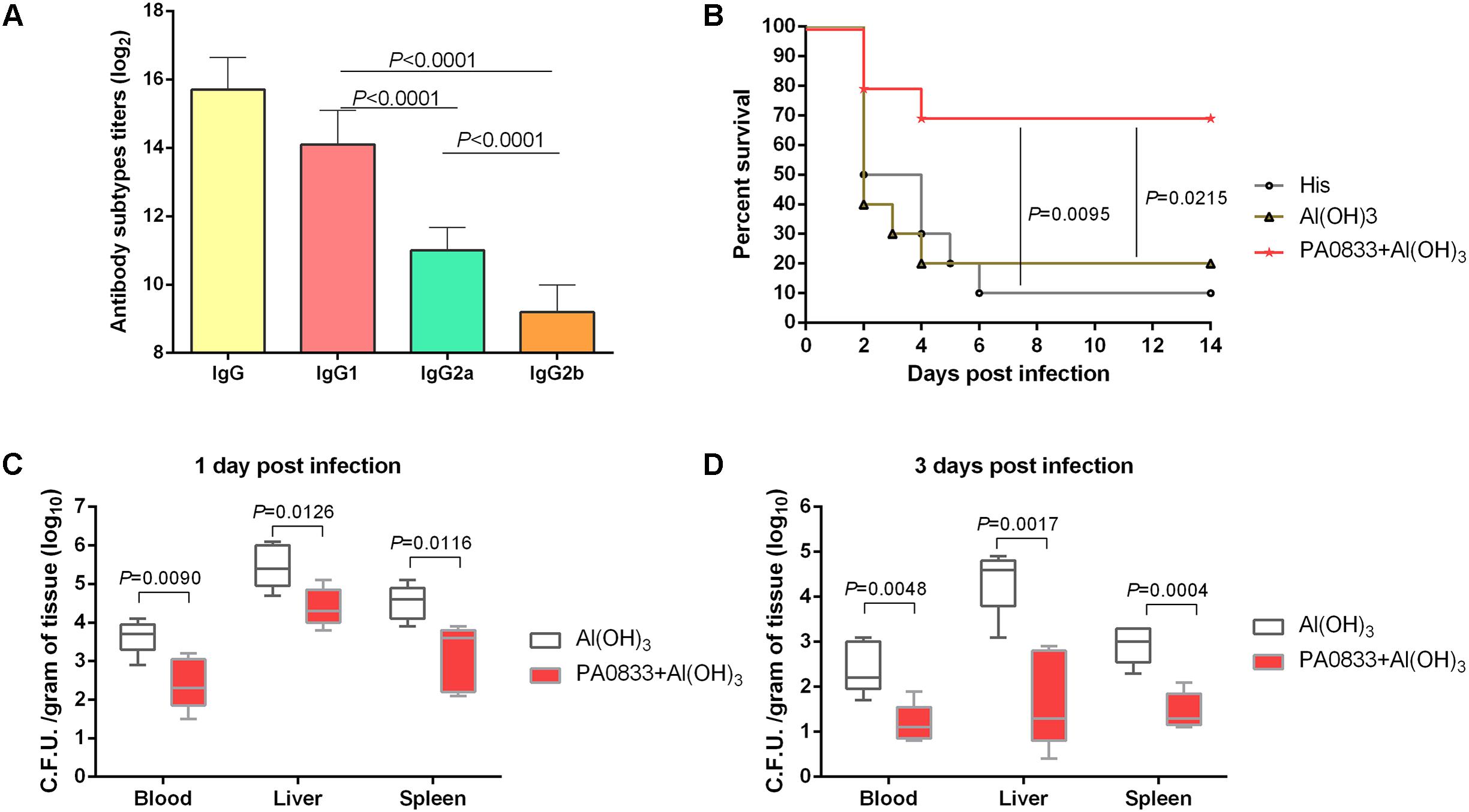
FIGURE 1. PA0833 stimulates protective immunity in a P. aeruginosa sepsis model. (A) Comparison of total IgG and IgG subgroups (IgG1, IgG2a, and IgG2b) in the mice (n = 5) immunized with PA0833. Serum was obtained at 7 days after the final immunization, and the levels of total IgG and IgG subgroups were expressed as the means of log2 titers. Multiple comparisons among different groups were analyzed using one-way ANOVA. Data are shown as the means ± SD. (B) BALB/c mice (n = 10) were immunized with PA0833 plus an Al(OH)3 adjuvant and challenged with PAO1 at 7.0 × 107 CFUs/mouse by intravenous injection. The survival rate was monitored for 14 days. The P-values were calculated using the Mantel–Cox log-rank test. (C,D) Efficacy of immunization with PA0833 on the spread of P. aeruginosa. The number of viable bacteria in the blood, liver, and spleen of mice (n = 10) at 1 and 3 days post-infection are shown. Data are presented in box and whisker plots, and the medians are shown. Differences were compared to determine their significance using Student’s t-test.
Next, the PA0833-immunized mice were challenged with a sub-lethal dose of P. aeruginosa to investigate the bacterial burden in the organs. The results showed that the bacterial burden in the blood, livers, and spleens were much lower in the PA0833 group 1 day post-infection mice than in the Al(OH)3 control group (Pblood = 0.0090, Pliver = 0.0126, and Pspleen = 0.0116, Figure 1C). Furthermore, the reduction of P. aeruginosa was enhanced in the PA0833 group 3 days post-infection compared to that in the Al(OH)3 group (Pblood = 0.0048, Pliver = 0.0017, and Pspleen = 0.0004, Figure 1D). These results showed that immunization with PA0833 protected mice against P. aeruginosa infection by reducing the ability of the bacteria to colonize and directly attack organs, improving the survival of the mice.
PA0833 Vaccination Protects Mice From Pneumonia by Reducing Local Bacterial Burden and Inflammation
PA0833 also showed protective efficacy in a lethal P. aeruginosa pneumonia model. Mice were immunized with 30 μg of PA0833 at day 0, 14, and 21 and then intratracheally infected with a lethal dose of PAO1. Similar to the sepsis model, mice exhibited survival rates after being immunized with PA0833 (50%, Figure 2A) that were significantly higher than those in the His buffer control group (P = 0.0014) and the Al(OH)3 adjuvant control group (P = 0.0281, Figure 2A).
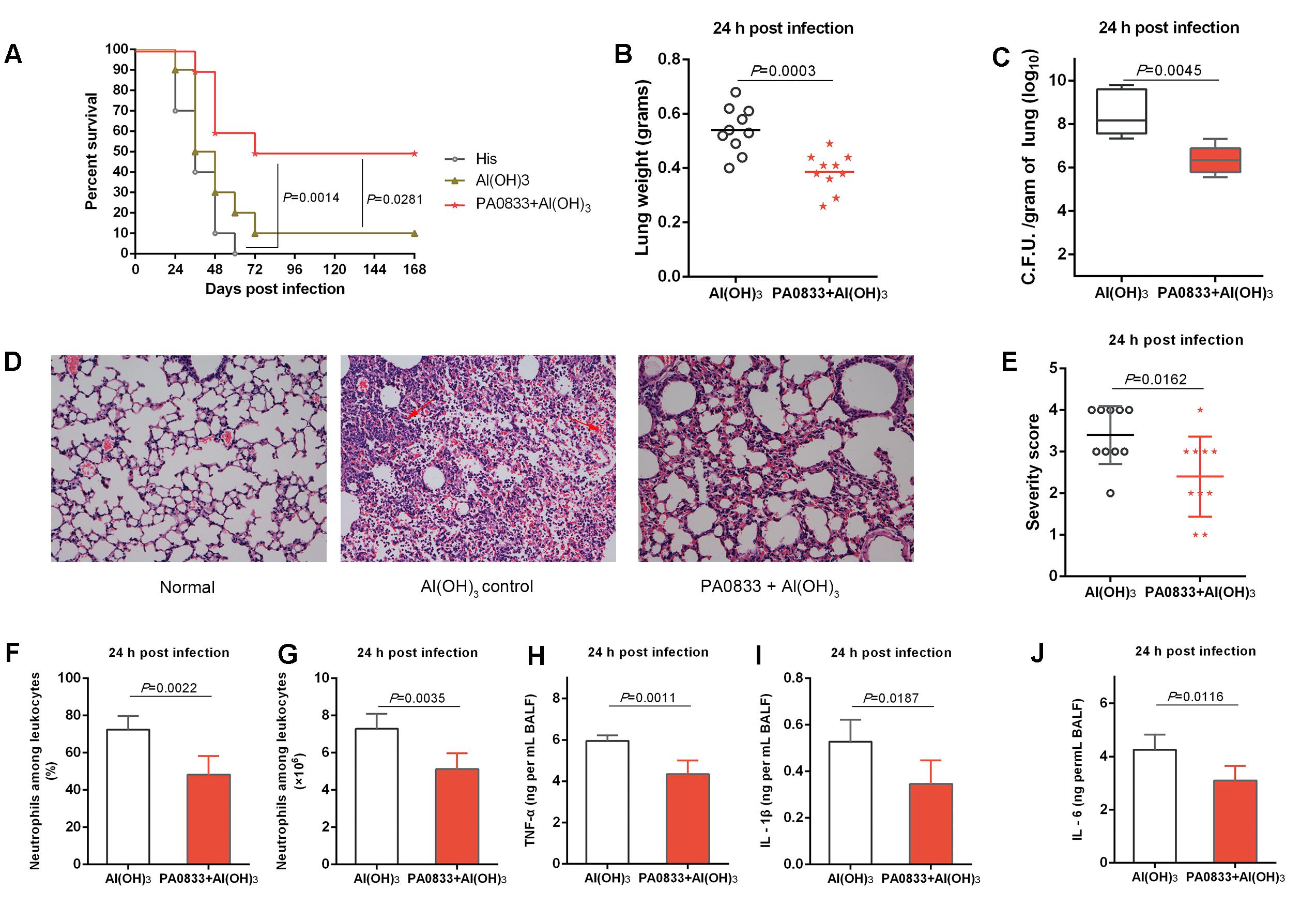
FIGURE 2. Protective efficacy of PA0833 in a murine P. aeruginosa pneumonia model. (A) BALB/c mice (n = 10) were immunized with PA0833 plus an Al(OH)3 adjuvant and challenged with PAO1 at 1.0 × 107 CFUs/mouse by intratracheal injection. The survival rate was monitored for 1 week. (B–J) The immunized mice and control mice were infected intratracheally with 5.0 × 106 CFUs/mouse of PAO1. (B) Lung weight in infected mice (n = 10) immunized with PA0833 plus an Al(OH)3 adjuvant. The data are presented as scatter plots. (C) The number of viable bacteria in the lungs of mice (n = 10) at 24 h post-infection are shown. Data are presented in box and whisker plots, and the medians are shown. (D) Hematoxylin-eosin staining of lungs from immunized mice and control mice 24 h after infection. Representative histopathological sections from 10 mice per group are shown (magnification = 200 × ). The arrows point to hemorrhage and neutrophil infiltration in lungs. (E) Semi-quantification of lung inflammation in infected mice. Severity scores of lungs (n = 10) from immunized mice and control mice 24 h post-infection are shown. The data are presented as scatter plots. (F,G) Evaluation of neutrophil infiltration in infected mice (n = 10). The bar represents the percentage (F) and the number (G) of neutrophils in the BALF of immunized mice at 24 h post challenge. (H–J) Quantitative detection of proinflammatory cytokines TNF-α, IL-1β and IL-6 in infected mice (n = 10). The data (F–J) are shown as the means ± SD. The P-value (A) was calculated using the Mantel–Cox log-rank test. The differences (B,C,E–J) were compared to determine their statistical significance using Student’s t-test.
Next, the PA0833-immunized mice were challenged with a sub-lethal dose of P. aeruginosa to investigate the mechanism of PA0833-induced protection. First, the lungs from immunized and control mice were harvested 24 h post-infection. Mice that were immunized with PA0833 exhibited decreased pulmonary edema post-infection, as measured by lung weight, compared to the Al(OH)3 immunized mice (P = 0.0003, Figure 2B). In addition, the PA0833 vaccinated group showed significantly lower bacterial loads than the Al(OH)3 group 24 h post-infection (P = 0.0045, Figure 2C).
Histological analysis showed that the lungs from the mice in the PA0833 immunized groups exhibited reduced alveolar disruption, vascular leakage and deposition of bacterial microcolonies in the alveoli after infection compared with the Al(OH)3 group (Figure 2D). In addition, typical pathological changes were observed in mice immunized with Al(OH)3 (Figures 2D,E). However, mice immunized with PA0833 exhibited reduced inflammatory cell infiltration, bleeding, and tissue damage compared to the Al(OH)3 group (Figures 2D,E).
Markers of inflammation, including neutrophil infiltration and proinflammatory cytokine production in the BALF, such as TNF-α, IL-1β and IL-6, were determined 24 h post-infection. Consistent with the lung histopathology results described above, neutrophil number and percentage relative to other leukocytes were significantly reduced in the BALF of mice immunized with PA0833 (Figures 2F,G). Similar results were observed for proinflammatory cytokine secretion (Figure 2H–J). Taken together, these results confirmed the protective efficacy of PA0833 vaccination, which was attributed to reduced pulmonary edema, bacterial burden, pathology and proinflammatory cytokine production.
Bioinformatics Prediction Indicates PA0833 Is an OmpA C-Like Protein
Our results clearly showed that PA0833 conferred protection against P. aeruginosa infection in animal models, but the biochemical and structural properties of this protein have not been characterized to date. We systematically predicted the bioinformatics features of this protein based on its sequence. According to our results, the C-terminus of PA0833 is similar to that of OmpA family proteins. First, the subcellular localization of PA0833 is predicted to be similar to that of OmpA family proteins. PA0833 consists of two domains, the N-terminal domain contains a signal peptide (amino acids 1 to 25) and two trans-membrane helixes (Supplementary Figure S1A), which may anchor the protein to the membrane of the bacteria. Whereas the C-terminal domain is located in the periplasm. Second, the predicted overall structure of the C-terminal domain of PA0833 (PA083386-237) modeled by SWISS-MODEL contains 3 α-helixes and 3 β-sheets, which share the same folding pattern as the crystal structure of the C-terminal domain of OmpA from Acinetobacter baumannii (Park et al., 2012) (Supplementary Figure S1B). Third, when the sequence of the C-terminal region of PA0833 was aligned with homologous proteins, the similarity and identity was approximately 50 and 20%, respectively (Supplementary Figure S1C). Finally, the structure of the C-terminal domain of OmpA from A. baumannii revealed that two residues (Asp271 and Arg286) were involved in binding diaminopimelate (DAP), a unique bacterial amino acid present in peptidoglycan, and these residues were completely conserved in PA0833 (Supplementary Figure S1C). These results strongly indicate that PA0833 is an OmpA C-like protein.
PA0833 Exists as a Dimer in Solution and Binds to Peptidoglycan in Vitro
As shown in Figure 3A, PA083326-237 and PA083386-237 expressed in E. coli were soluble, and the purity was up to 95% as determined by SDS–PAGE after two rounds of chromatography. The molecular weights of the two proteins were in accordance with their predicted molecular masses (22.6 and 16.4 kDa for PA083326-237 and PA083386-237, respectively). The C-terminal domain of OmpA family proteins typically form dimers and are able to bind to peptidoglycan in vitro. Therefore, we determined the oligomeric states of PA083386-237 by gel-filtration and cross-linking analyses. As shown in Figure 3C, the elution volume for PA083386-237 was 17.33 ml, and the molecular weight of PA0833 was calculated as 33.2 kDa according to the standard curve (Figure 3B). The theoretical molecular weight of PA083386-237 is 16.8 kDa, and thus it appears as a dimer in solution. This result was further confirmed by the cross-linking assay, where a band corresponding to dimerized PA083386-237 was detected in the gel in the presence of glutaraldehyde, and the amount of the linked PA0833 dimer increased in a glutaraldehyde dose-dependent manner (Figure 3D). As a negative control, BSA did not form cross-links in the presence of glutaraldehyde (Supplementary Figure S2).
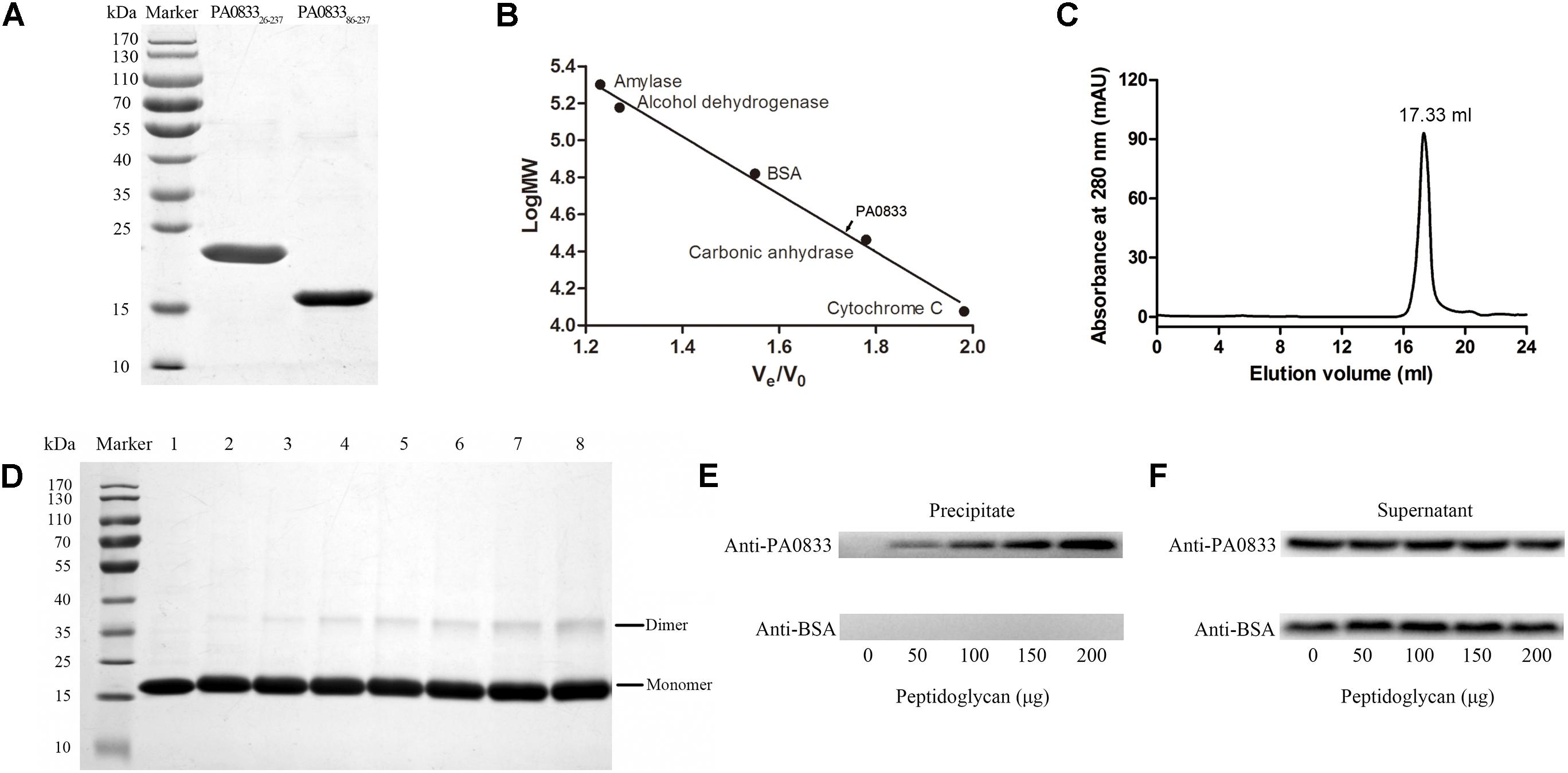
FIGURE 3. Preparation and characterization of PA0833. (A) PA083326-237 and PA083386-237 were purified and analyzed by SDS–PAGE. (B,C) Gel-filtration analysis of PA083386-237. The standard curve was built by protein standards (amylase, alcohol dehydrogenase, BSA, carbonic anhydrase, and cytochrome (C). The elution volume of PA083386-237 was 17.33 ml. (D) SDS–PAGE analysis of PA083386-237 after treatment with different concentrations of glutaraldehyde. Lane 1 shows the native PA083386-237. Lanes 2–8 show the formation of dimers or aggregates with an increasing concentration of glutaraldehyde (0.01, 0.05, 0.1, 0.2, 0.3, 0.4, and 0.5%). (E,F) BSA was used as a negative control. (E) Detection of PA083386-237 and BSA from the insoluble precipitates by western blot. (F) Detection of free proteins in the supernatant.
We next assessed the binding capacity between soluble purified PA083386-237 to insoluble peptidoglycan. As shown in Figure 3E, PA083386-237 was detected in the precipitate fraction, whereas the BSA negative control was not observed. In addition, the density of PA083386-237, which binds to insoluble peptidoglycan, increased when the amount of peptidoglycan increased, suggesting that PA083386-237 binds peptidoglycan in a dose-dependent manner. Furthermore, PA083386-237 was also observed in the supernatant fractions, indicating that PA083386-237 was present in excess in the binding assay (Figure 3F).
PA0833 Facilitates Bacteria Survival in a Stressful Environment
To test the function of PA0833 in vitro, we first knocked out the PA0833 gene in PAO1 using the suicide vector pCVD442 to generate PAO1/ΔPA0833. And then we constructed complementary plasmid pDN18-PA0833, and electroporated them into the PA0833 mutant strain to generate PAO1/CPA0833, which was confirmed by the results of indirect immunofluorescence (Supplementary Figure S3). No significant difference in the growth rate between PAO1/WT and these two mutants was observed under normal culturing conditions (Supplementary Figure S4). However, in acidic culture medium (pH 4.0), PAO1/WT grew significantly better than PAO1/ΔPA0833, its growth index did not decline until 60 min after incubation (Figure 4A). The PAO1/ΔPA0833 strain was sensitive to the acidic environment, as its growth index decreased shortly after incubation. The complement of pDN18-PA0833 in this strain significantly changed the acid resistance ability, which was similar to that of the PAO1/WT, as no significant difference was found between PAO1/CPA0833 and PAO1/WT (Figure 4A). This result suggested that PA0833 is partly involved in the acid resistance.
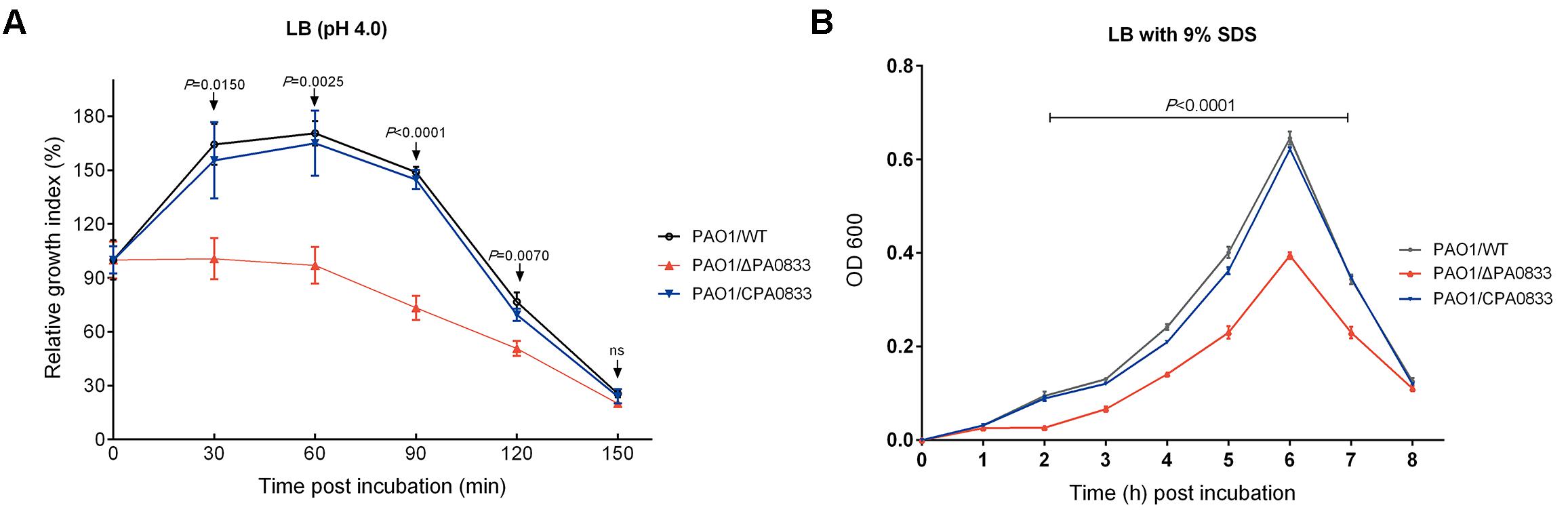
FIGURE 4. Analysis of the contribution of PA0833 to bacterial resistance to stress. (A) Acid resistance of PAO1/ WT, PAO1/ΔPA0833, and PAO1/CPA0833. The three strains of PAO1 were grown in LB (pH 4.0) for 30, 60, 90, 120, and 150 min, respectively. (B) The SDS resistance of PAO1/WT, PAO1/ΔPA0833, and PAO1/CPA0833. Bacteria were grown in LB culture that contained 9% SDS for 8 h. The absorbency at 600 nm of the three strains of PAO1 was recorded every hour. The data are shown as the mean ± SD. The differences were compared to determine their statistical significance using one-way ANOVA (ns = no significance). The P-values shown in the figure were the statistical difference between PAO1/WT and PAO1/ΔPA0833, and there was no statistical difference between PAO1/WT and PAO1/CPA0833.
A similar pattern was observed in the presence of the detergent SDS (Figure 4B). When the PA0833 mutants were grown in LB with 9% SDS, significant differences were observed from 2 to 7 h after incubation (Figure 4B). The PAO1/WT and PAO1/CPA0833 strain grew significantly better than the PAO1/ΔPA0833 mutant, and there was no significant difference in the growth rate between the two strains (Figure 4B). This result suggested that PA0833 is responsible for bacterial detergent resistance.
PA0833 Is Involved in the P. aeruginosa Virulence
Since PA0833 provided protective immunity against P. aeruginosa infection, we speculated that this protein may be involved in the pathogenicity of the bacterium. With this in mind, we infected mice intratracheally with different doses [LD50 (5 × 106) CFU/mice, 2 × LD50 (1 × 107) CFU/mice] of the PAO1/WT or PAO1/ΔPA0833 strains, and the survival of the mice was monitored for 1 week. As shown in Figure 5, at the end of the observation period, mice challenged with the PAO1/ΔPA0833 mutant exhibited higher survival rates (90 and 40%) than the PAO1/WT group (50 and 0%, PLD50 = 0.0063, P2 × LD50 = 0.0416, respectively). These data demonstrated that the virulence of PAO1 was decreased in the PA0833 knock out strain, indicating that PA0833 is involved in P. aeruginosa virulence.
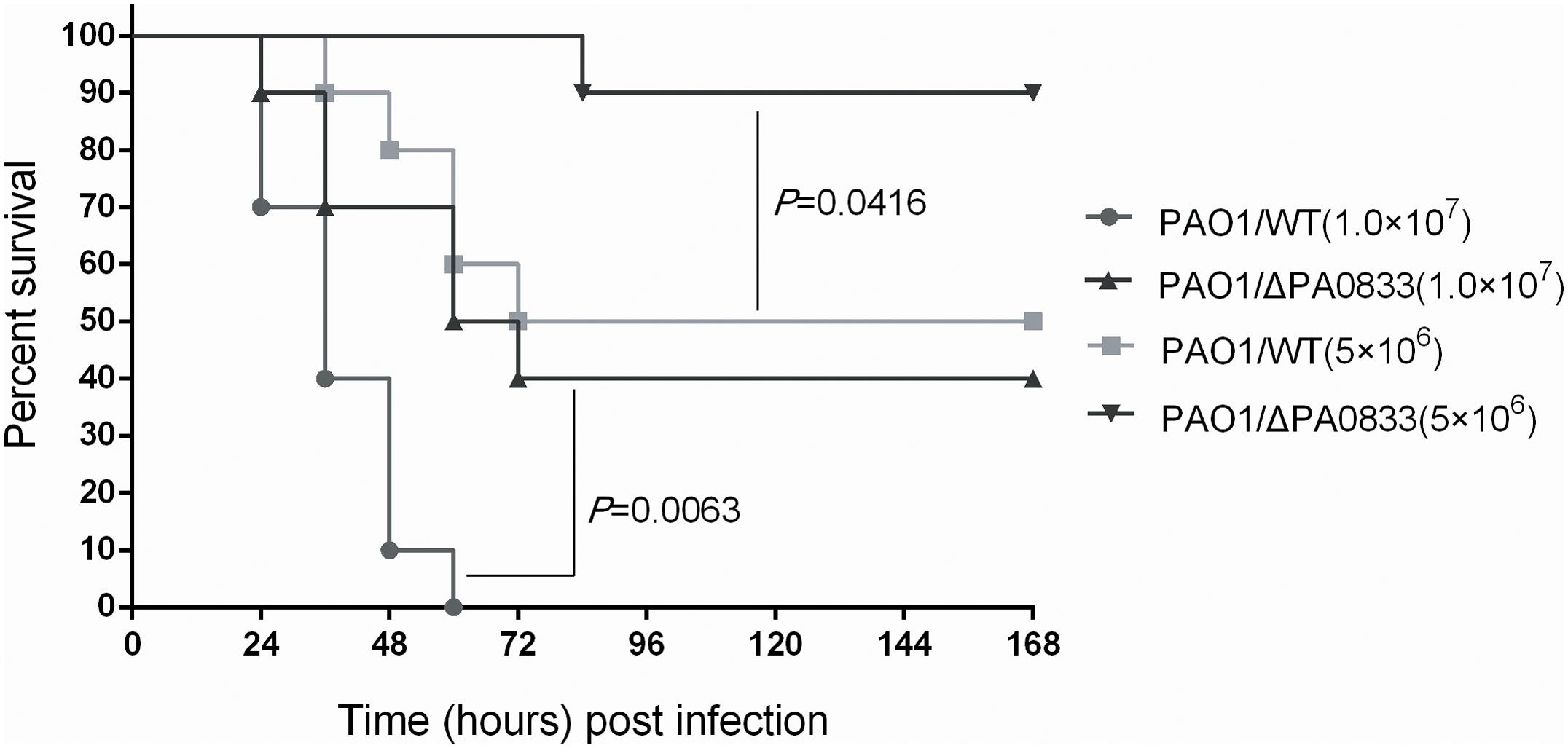
FIGURE 5. Analysis of the contribution of PA0833 to bacteria virulence. BALB/c mice (n = 10) were challenged with PAO1/WT or PAO1/ΔPA0833 at 1.0 × 107 or 5.0 × 106 CFUs/mouse by intratracheal injection, respectively. The survival rate was monitored for 1 week. The P-value was calculated using the Mantel–Cox log-rank test.
Pseudomonas aeruginosa strains that overproduce alginate, also known as mucoid strains, are associated with chronic endobronchial infections in cystic fibrosis patients (Damron et al., 2012). Through GEO database analyses, we observed that the expression of PA0833 is increased in P. aeruginosa strains overexpressing alginate. As shown in Supplementary Figure S5A, in an alginate overproduction mutant strain of PAO1, the expression of PA0833 was significantly higher than that in a PAO1 mutant strain that produces low amounts of alginate [P = 0.0163, GEO accession: GSE35248 (Damron et al., 2012)]. Furthermore, the expression of PA0833 in a mucoid strain from a cystic fibrosis (CF) patient was 7.3-fold higher than that in a non-mucoid P. aeruginosa strain [Supplementary Figure S5B, GEO accession: GSE9621 (Rao et al., 2008)]. Moreover, the expression of PA0833 in P. aeruginosa strains isolated from cystic fibrosis lungs was significantly higher than that in PAO1 [Supplementary Figure S5C, P = 0.0147, GEO accession: GSE7704 (Son et al., 2007)]. These results indicated that the expression of PA0833 may be associated with the expression of alginate. The ability of P. aeruginosa to produce chronic infection is based in part on its ability to form biofilms, in which alginate is the major polysaccharide. Thus, conversion to the mucoid phenotype in P. aeruginosa is associated with a significant increase in morbidity and mortality (Alkawash et al., 2006). These studies further suggested that PA0833 may be participated in the establishment of chronic infection by P. aeruginosa.
Discussion
The OmpA family of outer membrane proteins is characterized as a group of genetically related, surface-exposed porin proteins (Confer and Ayalew, 2013). Typically, these proteins have a high copy number and are highly conserved among different species of Gram-negative bacteria (Confer and Ayalew, 2013). The N-terminal domain of OmpA family proteins typically forms an eight-stranded, anti-parallel β barrel that is embedded in the outer membrane, whereas the C-terminal domain is globular, forms a dimer and is associated with peptidoglycan (Reusch, 2012b). In this study, PA0833 was observed to exist as a dimer in solution and was able to bind to peptidoglycan. The sequence and structure of the C-terminal domain of PA0833 (PA083386-237) was observed to be highly conserved with OmpA family proteins, and two conserved peptidoglycan-interacting residues were also observed in PA0833. In contrast, sequence alignment indicated that the N-terminal domain of PA0833 was not conserved among OmpA family proteins, several deletions were observed in PA0833 when compared with other proteins (Supplementary Figure S1C). Meanwhile, there was no beta barrel domains as predicted by TBBpred, only two trans-membrane domain were predicted by TMHMM, which embedded the protein in the outer membrane of the bacterial. Therefore, we speculated that PA0833 is an OmpA C-like protein.
Because of the high abundance and surface exposure properties of OmpA family proteins in the bacterial outer membrane, these proteins have been reported to participate in a variety of pathogenic pathways and play a key role during bacterial infection (Hancock and Brinkman, 2002; Krishnan and Prasadarao, 2012; Reusch, 2012a). For example, they are involved in bacterial virulence, adhesion and invasion, and in interactions with surface receptors on host cells (Smith et al., 2007; Fito-Boncompte et al., 2011; Sugawara et al., 2012). In addition, these proteins are important for maintaining the integrity of the outer membrane and can stimulate strong antibody responses, with anti-OmpA family proteins antibodies reported to be bactericidal, opsonic, and protective (Zhang et al., 2016; Kazemi Moghaddam et al., 2017; Maccarini et al., 2017; Tang et al., 2017). As a result, OmpA family proteins are promising candidates for vaccine development, and these proteins from a number of bacteria have been reported to be able to induce protective immunity against bacterial infection, some vaccines contain OmpA family proteins even entered phase III clinical trials, such as OprF from P. aeruginosa (Price et al., 2001; Oldfield et al., 2008; Peluso et al., 2010; Ayalew et al., 2011; Hounsome et al., 2011; Westritschnig et al., 2014; Zhang et al., 2016; Hassan et al., 2017).
According to our results, PA0833 may function as a novel virulence factor in P. aeruginosa for the following reasons. First, PA0833 knockout led to an impaired ability of P. aeruginosa cells to resist environment stress, suggesting that PA0833 may be essential for maintaining the integrity of the bacterial cell wall to enhance its adaptability to complex environments and improve the survival of bacteria. Second, the virulence of PAO1 was decreased after PA0833 knockout. Third, the expression of PA0833 was higher in P. aeruginosa strains isolated from cystic fibrosis lungs, indicating PA0833 may be associated with the biosynthesis of biofilms and the establishment of chronic infections by P. aeruginosa.
As an outer membrane protein, we speculate that PA0833 may interact with the receptors on host cells, such as alveolar epithelial cells and macrophages, to activate substantial signaling pathways to exert its biological effects. We are currently screening for possible receptors of PA0833 in different cell lines and have identified several significantly up-regulated genes in the NOD-like receptor (NLR) signaling pathway, such as NAIP, BIRC3 and HSP90AA1 (Supplementary Figure S6). These results indicate that the Nod-like family of pattern recognition receptors are involved in the innate immune response to P. aeruginosa. Further studies will focus on the pathogenic mechanism of PA0833 and the immune response mechanism to PA0833.
Our findings also confirmed that immunization with PA0833 induced strong immune responses and resulted in reduced bacterial loads as well as decreased pathology, inflammatory cytokine expression and inflammatory cell infiltration after P. aeruginosa infection. PA0833 was able to induce a protective efficacy against P. aeruginosa lethal sepsis and pneumonia murine models. Therefore, as an OmpA C-like protein, PA0833 is a potentially promising vaccine candidate for combating P. aeruginosa infection.
In this study, two different truncated forms of PA0833, termed PA083326-237 and PA083386-237, were constructed. The former was used as a vaccine candidate to evaluate the protective efficacy of this protein, primarily because this construct contained the outer membrane domains of this protein, which may be the key domain that induces protective immunity since it is surface exposed. The latter domain was used to evaluate the homology of PA0833 with OmpA proteins because it contains a unique and intact domain that functions similarly to the OmpA family proteins.
Conclusion
PA0833 is an OmpA C-like protein that was observed to exist as a dimer in solution and was able to bind to peptidoglycan in vitro. In addition, PA0833 contributed to bacterial environment stress resistance and virulence. Furthermore, immunization with PA0833 significantly reduced acute systemic infection and pneumonia due to P. aeruginosa in mice. Therefore, PA0833 can be regarded as a novel P. aeruginosa vaccine candidate. Further studies on PA0833 will focus on its involvement in crosstalk between P. aeruginosa and host cells to explain its pathogenic and immune protection mechanisms.
Author Contributions
JyZ, FL, and QZ designed the research. FY, JG, and JtZ conducted the experiments, analyzed the data, wrote the main manuscript text, and prepared the figures and tables, LL, HJ, and JZ helped to conduct the experiments, HZ and QZ contributed to writing the manuscript and supervised the project. FL and JyZ helped with the discussion of results and manuscript refinement. All authors reviewed the manuscript.
Funding
This work was supported by the National Natural Science Foundation of China (Grant No. 81771778) and the Key Project of Innovative Drug Development (Grant No. 2016ZX09J16102-002). None of the funding agencies had a role in study design, data collection or interpretation, or the decision to submit the work for publication.
Conflict of Interest Statement
The authors declare that the research was conducted in the absence of any commercial or financial relationships that could be construed as a potential conflict of interest.
Supplementary Material
The Supplementary Material for this article can be found online at: https://www.frontiersin.org/articles/10.3389/fmicb.2018.01062/full#supplementary-material
Footnotes
References
Alkawash, M. A., Soothill, J. S., and Schiller, N. L. (2006). Alginate lyase enhances antibiotic killing of mucoid Pseudomonas aeruginosa in biofilms. APMIS 114, 131–138. doi: 10.1111/j.1600-0463.2006.apm_356.x
Arnold, K., Bordoli, L., Kopp, J., and Schwede, T. (2006). The SWISS-MODEL workspace: a web-based environment for protein structure homology modelling. Bioinformatics 22, 195–201. doi: 10.1093/bioinformatics/bti770
Ayalew, S., Shrestha, B., Montelongo, M., Wilson, A. E., and Confer, A. W. (2011). Immunogenicity of Mannheimia haemolytica recombinant outer membrane proteins serotype 1-specific antigen, OmpA, OmpP2, and OmpD15. Clin. Vaccine Immunol. 18, 2067–2074. doi: 10.1128/CVI.05332-11
Bai, F., Li, Y., Xu, H., Xia, H., Yin, T., Yao, H., et al. (2007). Identification and functional characterization of pfm, a novel gene involved in swimming motility of Pseudomonas aeruginosa. Gene 401, 19–27. doi: 10.1016/j.gene.2007.06.019
Banadkoki, A. Z., Keshavarzmehr, M., Afshar, Z., Aleyasin, N., Fatemi, M. J., Behrouz, B., et al. (2016). Protective effect of pilin protein with alum+naloxone adjuvant against acute pulmonary Pseudomonas aeruginosa infection. Biologicals 44, 367–373. doi: 10.1016/j.biologicals.2016.06.009
Baumann, U., Mansouri, E., and Von Specht, B. U. (2004). Recombinant OprF-OprI as a vaccine against Pseudomonas aeruginosa infections. Vaccine 22, 840–847. doi: 10.1016/j.vaccine.2003.11.029
Chen, Y., Yu, P., Luo, J., and Jiang, Y. (2003). Secreted protein prediction system combining CJ-SPHMM, TMHMM, and PSORT. Mamm. Genome 14, 859–865. doi: 10.1007/s00335-003-2296-6
Choi, D. S., Kim, D. K., Choi, S. J., Lee, J., Choi, J. P., Rho, S., et al. (2011). Proteomic analysis of outer membrane vesicles derived from Pseudomonas aeruginosa. Proteomics 11, 3424–3429. doi: 10.1002/pmic.201000212
Confer, A. W., and Ayalew, S. (2013). The OmpA family of proteins: roles in bacterial pathogenesis and immunity. Vet. Microbiol. 163, 207–222. doi: 10.1016/j.vetmic.2012.08.019
Curran, C. S., Bolig, T., and Torabi-Parizi, P. (2017). Mechanisms and targeted therapies for Pseudomonas aeruginosa lung infection. Am. J. Respir. Crit. Care Med. 197, 708–727. doi: 10.1164/rccm.201705-1043SO
Damron, F. H., Owings, J. P., Okkotsu, Y., Varga, J. J., Schurr, J. R., Goldberg, J. B., et al. (2012). Analysis of the Pseudomonas aeruginosa regulon controlled by the sensor kinase KinB and sigma factor RpoN. J. Bacteriol. 194, 1317–1330. doi: 10.1128/JB.06105-11
Fadouloglou, V. E., Kokkinidis, M., and Glykos, N. M. (2008). Determination of protein oligomerization state: two approaches based on glutaraldehyde crosslinking. Anal. Biochem. 373, 404–406. doi: 10.1016/j.ab.2007.10.027
Fito-Boncompte, L., Chapalain, A., Bouffartigues, E., Chaker, H., Lesouhaitier, O., Gicquel, G., et al. (2011). Full virulence of Pseudomonas aeruginosa requires OprF. Infect. Immun. 79, 1176–1186. doi: 10.1128/IAI.00850-10
Gao, C., Yang, F., Wang, Y., Liao, Y., Zhang, J., Zeng, H., et al. (2017). Vaccination with a recombinant OprL fragment induces a Th17 response and confers serotype-independent protection against Pseudomonas aeruginosa infection in mice. Clin. Immunol. 183, 354–363. doi: 10.1016/j.clim.2017.09.022
Gellatly, S. L., and Hancock, R. E. (2013). Pseudomonas aeruginosa: new insights into pathogenesis and host defenses. Pathog. Dis. 67, 159–173. doi: 10.1111/2049-632X.12033
Grimwood, K., Kyd, J. M., Owen, S. J., Massa, H. M., and Cripps, A. W. (2015). Vaccination against respiratory Pseudomonas aeruginosa infection. Hum. Vaccin. Immunother. 11, 14–20. doi: 10.4161/hv.34296
Hamaoka, S., Naito, Y., Katoh, H., Shimizu, M., Kinoshita, M., Akiyama, K., et al. (2017). Efficacy comparison of adjuvants in PcrV vaccine against Pseudomonas aeruginosa pneumonia. Microbiol. Immunol. 61, 64–74. doi: 10.1111/1348-0421.12467
Hancock, R. E., and Brinkman, F. S. (2002). Function of Pseudomonas porins in uptake and efflux. Annu. Rev. Microbiol. 56, 17–38. doi: 10.1146/annurev.micro.56.012302.160310
Hassan, R., El-Naggar, W., Abd El-Aziz, A. M., Shaaban, M., Kenawy, H. I., and Ali, Y. M. (2017). Immunization with outer membrane proteins (OprF and OprI) and flagellin B protects mice from pulmonary infection with mucoid and nonmucoid Pseudomonas aeruginosa. J. Microbiol. Immunol. Infect. doi: 10.1016/j.jmii.2016.08.014 [Epub ahead of print].
Hounsome, J. D., Baillie, S., Noofeli, M., Riboldi-Tunnicliffe, A., Burchmore, R. J., Isaacs, N. W., et al. (2011). Outer membrane protein A of bovine and ovine isolates of Mannheimia haemolytica is surface exposed and contains host species-specific epitopes. Infect. Immun. 79, 4332–4341. doi: 10.1128/IAI.05469-11
Kalil, A. C., Metersky, M. L., Klompas, M., Muscedere, J., Sweeney, D. A., Palmer, L. B., et al. (2016). Management of adults with hospital-acquired and ventilator-associated pneumonia: 2016 clinical practice guidelines by the infectious diseases society of America and the American thoracic society. Clin. Infect. Dis. 63, e61–e111. doi: 10.1093/cid/ciw353
Kazemi Moghaddam, E., Owlia, P., Jahangiri, A., Rasooli, I., Rahbar, M. R., and Aghajani, M. (2017). Conserved OprF as a selective immunogen against Pseudomonas aeruginosa. Iran. J. Pathol. 12, 165–170.
Kelley, L. A., Mezulis, S., Yates, C. M., Wass, M. N., and Sternberg, M. J. (2015). The Phyre2 web portal for protein modeling, prediction and analysis. Nat. Protoc. 10, 845–858. doi: 10.1038/nprot.2015.053
Kizny Gordon, A. E., Mathers, A. J., Cheong, E. Y. L., Gottlieb, T., Kotay, S., Walker, A. S., et al. (2017). The hospital water environment as a reservoir for carbapenem-resistant organisms causing hospital-acquired infections-A systematic review of the literature. Clin. Infect. Dis. 64, 1435–1444. doi: 10.1093/cid/cix132
Krishnan, S., and Prasadarao, N. V. (2012). Outer membrane protein A and OprF: versatile roles in Gram-negative bacterial infections. FEBS J. 279, 919–931. doi: 10.1111/j.1742-4658.2012.08482.x
Le Moigne, V., Gaillard, J. L., and Herrmann, J. L. (2016). Vaccine strategies against bacterial pathogens in cystic fibrosis patients. Med. Mal. Infect. 46, 4–9. doi: 10.1016/j.medmal.2015.11.013
Liu, J., Sun, Y., Feng, S., Zhu, L., Guo, X., and Qi, C. (2009). Towards an attenuated enterohemorrhagic Escherichia coli O157:H7 vaccine characterized by a deleted ler gene and containing apathogenic Shiga toxins. Vaccine 27, 5929–5935. doi: 10.1016/j.vaccine.2009.07.097
Maccarini, M., Gayet, L., Alcaraz, J. P., Liguori, L., Stidder, B., Watkins, E. B., et al. (2017). Functional characterization of cell-free expressed OprF porin from Pseudomonas aeruginosa stably incorporated in tethered lipid bilayers. Langmuir 33, 9988–9996. doi: 10.1021/acs.langmuir.7b01731
Melsen, W. G., Rovers, M. M., Groenwold, R. H., Bergmans, D. C., Camus, C., Bauer, T. T., et al. (2013). Attributable mortality of ventilator-associated pneumonia: a meta-analysis of individual patient data from randomised prevention studies. Lancet Infect. Dis. 13, 665–671. doi: 10.1016/S1473-3099(13)70081-1
Nathwani, D., Raman, G., Sulham, K., Gavaghan, M., and Menon, V. (2014). Clinical and economic consequences of hospital-acquired resistant and multidrug-resistant Pseudomonas aeruginosa infections: a systematic review and meta-analysis. Antimicrob. Resist. Infect. Control 3:32. doi: 10.1186/2047-2994-3-32
Natt, N. K., Kaur, H., and Raghava, G. P. (2004). Prediction of transmembrane regions of beta-barrel proteins using ANN- and SVM-based methods. Proteins 56, 11–18. doi: 10.1002/prot.20092
Ohama, M., Hiramatsu, K., Miyajima, Y., Kishi, K., Nasu, M., and Kadota, J. (2006). Intratracheal immunization with pili protein protects against mortality associated with Pseudomonas aeruginosa pneumonia in mice. FEMS Immunol. Med. Microbiol. 47, 107–115. doi: 10.1111/j.1574-695X.2006.00069.x
Oldfield, N. J., Donovan, E. A., Worrall, K. E., Wooldridge, K. G., Langford, P. R., Rycroft, A. N., et al. (2008). Identification and characterization of novel antigenic vaccine candidates of Actinobacillus pleuropneumoniae. Vaccine 26, 1942–1954. doi: 10.1016/j.vaccine.2008.02.022
Park, J. S., Lee, W. C., Yeo, K. J., Ryu, K. S., Kumarasiri, M., Hesek, D., et al. (2012). Mechanism of anchoring of OmpA protein to the cell wall peptidoglycan of the gram-negative bacterial outer membrane. FASEB J. 26, 219–228. doi: 10.1096/fj.11-188425
Peluso, L., De Luca, C., Bozza, S., Leonardi, A., Giovannini, G., Lavorgna, A., et al. (2010). Protection against Pseudomonas aeruginosa lung infection in mice by recombinant OprF-pulsed dendritic cell immunization. BMC Microbiol. 10:9. doi: 10.1186/1471-2180-10-9
Petersen, T. N., Brunak, S., Von Heijne, G., and Nielsen, H. (2011). SignalP 4.0: discriminating signal peptides from transmembrane regions. Nat. Methods 8, 785–786. doi: 10.1038/nmeth.1701
Pier, G. (2005). Application of vaccine technology to prevention of Pseudomonas aeruginosa infections. Expert Rev. Vaccines 4, 645–656. doi: 10.1586/14760584.4.5.645
Pier, G. B. (2000). Peptides, Pseudomonas aeruginosa, polysaccharides and lipopolysaccharides–players in the predicament of cystic fibrosis patients. Trends Microbiol. 8, 247–250; discussion 250–251. doi: 10.1016/S0966-842X(00)01743-1
Pier, G. B. (2007). Pseudomonas aeruginosa lipopolysaccharide: a major virulence factor, initiator of inflammation and target for effective immunity. Int. J. Med. Microbiol. 297, 277–295. doi: 10.1016/j.ijmm.2007.03.012
Price, B. M., Galloway, D. R., Baker, N. R., Gilleland, L. B., Staczek, J., and Gilleland, H. E. Jr. (2001). Protection against Pseudomonas aeruginosa chronic lung infection in mice by genetic immunization against outer membrane protein F (OprF) of P. aeruginosa. Infect. Immun. 69, 3510–3515. doi: 10.1128/IAI.69.5.3510-3515.2001
Priebe, G. P., and Goldberg, J. B. (2014). Vaccines for Pseudomonas aeruginosa: a long and winding road. Expert Rev. Vaccines 13, 507–519. doi: 10.1586/14760584.2014.890053
Ramirez-Estrada, S., Borgatta, B., and Rello, J. (2016). Pseudomonas aeruginosa ventilator-associated pneumonia management. Infect. Drug Resist. 9, 7–18. doi: 10.2147/IDR.S50669
Rao, J., Digiandomenico, A., Unger, J., Bao, Y., Polanowska-Grabowska, R. K., and Goldberg, J. B. (2008). A novel oxidized low-density lipoprotein-binding protein from Pseudomonas aeruginosa. Microbiology 154, 654–665. doi: 10.1099/mic.0.2007/011429-0
Rello, J., Krenn, C. G., Locker, G., Pilger, E., Madl, C., Balica, L., et al. (2017). A randomized placebo-controlled phase II study of a Pseudomonas vaccine in ventilated ICU patients. Crit. Care 21:22. doi: 10.1186/s13054-017-1601-9
Reusch, R. N. (2012a). Biogenesis and functions of model integral outer membrane proteins: Escherichia coli OmpA and Pseudomonas aeruginosa OprF. FEBS J. 279:893. doi: 10.1111/j.1742-4658.2012.08486.x
Reusch, R. N. (2012b). Insights into the structure and assembly of Escherichia coli outer membrane protein A. FEBS J. 279, 894–909. doi: 10.1111/j.1742-4658.2012.08484.x
Sharma, A., Krause, A., and Worgall, S. (2011). Recent developments for Pseudomonas vaccines. Hum. Vaccin. 7, 999–1011. doi: 10.4161/hv.7.10.16369
Smith, S. G., Mahon, V., Lambert, M. A., and Fagan, R. P. (2007). A molecular Swiss army knife: OmpA structure, function and expression. FEMS Microbiol. Lett. 273, 1–11. doi: 10.1111/j.1574-6968.2007.00778.x
Son, M. S., Matthews, W. J. Jr., Kang, Y., Nguyen, D. T., and Hoang, T. T. (2007). In vivo evidence of Pseudomonas aeruginosa nutrient acquisition and pathogenesis in the lungs of cystic fibrosis patients. Infect. Immun. 75, 5313–5324. doi: 10.1128/IAI.01807-06
Sugawara, E., Nagano, K., and Nikaido, H. (2012). Alternative folding pathways of the major porin OprF of Pseudomonas aeruginosa. FEBS J. 279, 910–918. doi: 10.1111/j.1742-4658.2012.08481.x
Tang, X., Wang, H., Liu, F., Sheng, X., Xing, J., and Zhan, W. (2017). Outer membrane protein A: an immunogenic protein induces highly protective efficacy against Vibrio ichthyoenteri. Microb. Pathog. 113, 152–159. doi: 10.1016/j.micpath.2017.10.043
Vincent, J. L. (2014). Vaccine development and passive immunization for Pseudomonas aeruginosa in critically ill patients: a clinical update. Future Microbiol. 9, 457–463. doi: 10.2217/fmb.14.10
Wang, H., Li, Q., Fang, Y., Yu, S., Tang, B., Na, L., et al. (2016). Biochemical and functional characterization of the periplasmic domain of the outer membrane protein A from enterohemorrhagic Escherichia coli. Microbiol. Res. 182, 109–115. doi: 10.1016/j.micres.2015.10.004
Westritschnig, K., Hochreiter, R., Wallner, G., Firbas, C., Schwameis, M., and Jilma, B. (2014). A randomized, placebo-controlled phase I study assessing the safety and immunogenicity of a Pseudomonas aeruginosa hybrid outer membrane protein OprF/I vaccine (IC43) in healthy volunteers. Hum. Vaccin. Immunother. 10, 170–183. doi: 10.4161/hv.26565
Worgall, S. (2012). 40 years on: have we finally got a vaccine for Pseudomonas aeruginosa? Future Microbiol. 7, 1333–1335. doi: 10.2217/fmb.12.106
Yang, F., Gu, J., Yang, L., Gao, C., Jing, H., Wang, Y., et al. (2017). Protective efficacy of the trivalent Pseudomonas aeruginosa vaccine candidate PcrV-OprI-Hcp1 in murine pneumonia and burn models. Sci. Rep. 7:3957. doi: 10.1038/s41598-017-04029-5
Zhang, J., Yang, F., Zhang, X., Jing, H., Ren, C., Cai, C., et al. (2015). Protective efficacy and mechanism of passive immunization with polyclonal antibodies in a sepsis model of Staphylococcus aureus infection. Sci. Rep. 5:15553. doi: 10.1038/srep15553
Zhang, X., Yang, T., Cao, J., Sun, J., Dai, W., and Zhang, L. (2016). Mucosal immunization with purified OmpA elicited protective immunity against infections caused by multidrug-resistant Acinetobacter baumannii. Microb. Pathog. 96, 20–25. doi: 10.1016/j.micpath.2016.04.019
Zhang, X., Zhang, J., Zhang, R., Guo, Y., Wu, C., Mao, X., et al. (2013). Structural, enzymatic and biochemical studies on Helicobacter pylori arginase. Int. J. Biochem. Cell Biol. 45, 995–1002. doi: 10.1016/j.biocel.2013.02.008
Keywords: Pseudomonas aeruginosa, PA0833, OmpA, pneumonia, sepsis
Citation: Yang F, Gu J, Zou J, Lei L, Jing H, Zhang J, Zeng H, Zou Q, Lv F and Zhang J (2018) PA0833 Is an OmpA C-Like Protein That Confers Protection Against Pseudomonas aeruginosa Infection. Front. Microbiol. 9:1062. doi: 10.3389/fmicb.2018.01062
Received: 05 March 2018; Accepted: 04 May 2018;
Published: 23 May 2018.
Edited by:
Yuji Morita, Meiji Pharmaceutical University, JapanReviewed by:
Younes Smani, Instituto de Biomedicina de Sevilla (IBIS), SpainSylvie Chevalier, Université de Rouen, France
Graham Stafford, University of Sheffield, United Kingdom
Copyright © 2018 Yang, Gu, Zou, Lei, Jing, Zhang, Zeng, Zou, Lv and Zhang. This is an open-access article distributed under the terms of the Creative Commons Attribution License (CC BY). The use, distribution or reproduction in other forums is permitted, provided the original author(s) and the copyright owner are credited and that the original publication in this journal is cited, in accordance with accepted academic practice. No use, distribution or reproduction is permitted which does not comply with these terms.
*Correspondence: Fenglin Lv, bHl1ZmVuZ2xpbkBjcXUuZWR1LmNu Jinyong Zhang, emhhbmdqeTE5ODIxN0AxMjYuY29t