- 1Department of Gastroenterology and Hepatology, Sichuan University-University of Oxford Huaxi Joint Centre for Gastrointestinal Cancer, Frontiers Science Center for Disease-Related Molecular Network, West China Hospital, Sichuan University, Chengdu, China
- 2Laboratory of Liver Surgery, State Key Laboratory of Biotherapy/Collaborative Innovation Center for Biotherapy, West China Hospital, Sichuan University, Chengdu, China
Exosomes are small discoid extracellular vesicles (EVs) originating from endosomes that are 30–150 nm in diameter and have a double lipid layer. They participate in the immune response, cell migration, cell differentiation, and tumor invasion and mediate intercellular communication, regulating the biological activity of receptor cells through the proteins, nucleic acids, and lipids that they carry. Exosomes also play vital roles in the diagnosis and treatment of liver diseases. Macrophages, which show unique phenotypes and functions in complex microenvironments, can be divided into M1 and M2 subtypes. M1 macrophages function in immune surveillance, and M2 macrophages downregulate the immune response. Recent studies have shown that macrophages are involved in non-alcoholic fatty liver disease, liver fibrosis, and hepatocellular carcinoma. Moreover, several studies have demonstrated that liver diseases are associated with exosomes derived from or transferred to macrophages. This review focuses on the participation of macrophages and exosomes in liver diseases.
Introduction
Exosomes are small discoid extracellular vesicles (EVs) originating from endosomes that are 30–150 nm in diameter and have a double lipid layer (1). The exosome formation process involves invagination of the cell membrane to form an endosome, which then develops into a multivesicular body (MVB) that subsequently fuses with the cell membrane, releases the particles outside of the cell, and forms the exosome (2). A variety of cells can secrete exosomes under normal and pathological conditions (3). In addition, exosomes are also widely found in bodily fluids, including blood, saliva, urine, ascites, and cerebrospinal fluid (4, 5). The function of an exosome depends on the type of cell from which it originates. In general, exosomes can participate in processes such as immune response, cell migration, cell differentiation, and tumor invasion (6). Exosomes mediate intercellular communication, regulating the biological activity of receptor cells through the proteins, nucleic acids, and lipids they carry (7, 8). Exosomes also play a vital role in the diagnosis and treatment of liver diseases (9).
Macrophages are a heterogeneous population of cells that exhibit a unique phenotype and function in the complex microenvironment in vivo. According to differences in their activation state and function, macrophages can be divided into classically activated macrophages (CAMs or M1) and alternatively activated macrophages (AAMs or M2). M1 macrophages participate in the immune response and in immune surveillance by presenting antigens and secreting pro-inflammatory cytokines such as IL-6 and tumor necrosis factor-α (TNF-α). M2 macrophages have a weak antigen presentation ability and play an important role in immune regulation by downregulating the immune response via the secretion of the inhibitory cytokines IL-10, transforming growth factor-β (TGF-β) and mannose receptor (Mrc) (10–12). It has been suggested that macrophages have a series of continuous functional states, and M1 and M2 macrophages are the two extremes of this continuous state (13). Moreover, recent studies have found that macrophages are involved in non-alcoholic fatty liver disease (NAFLD) (14, 15), liver fibrosis (16), and hepatocellular carcinoma (HCC) (17).
Exosomes secreted by hepatocytes exposed to alcohol can be ingested by macrophages, thereby promoting the secretion of cytokines (18). In cholestatic liver disease, exosomal long non-coding RNA (lncRNA) H19 from bile duct cells promotes the M1 polarization of Kupffer cells and the production of chemokine ligand 2 and interleukin 6 (19). In melatonin-treated HCC cells, exosomes change the immunosuppression status of macrophages (20). In this review, we summarize the effects and interaction of macrophages and exosomes in liver diseases (Table 1).
Characteristics of Exosomes
Cells release bilayer membranous vesicles called EVs, which can be divided into exosomes, microvesicles (MVs), ectosomes, migrasomes, apoptotic bodies, and oncosomes according to their size and origin (37). Exosomes are the smallest EVs, with a diameter of 30–150 nm. Further, exosomes can be divided into small exosomes (60–80 nm) and large exosomes (90–120 nm). Proteomic analyses have shown that small exosomes carry proteins that are associated with endosomes, MVBs, and phagocytic vesicles, indicating that small exosomes are classical exosomes from the endosomal compartment. In contrast, large exosomes include plasma membrane proteins, cellular connexins, and late endosomal proteins and may be atypical exosomes from plasma membrane germination (38). Medium-sized EVs, 100–1,000 nm in diameter, include MVs, ectosomes, and microparticles (39). Ectosomes depend on the plasma membrane, while exosomes depend on endocytic membranes. These two distinct types of EVs differ in size, composition, and release regulation mechanisms. For ectosomes and exosomes, the goods on the surface and in the lumen differ when EVs are released by different cell types or individual cells in different functional conditions. After release, the two types of EVs move through the extracellular fluid at different times and for different distances (40). Migrasomes, apoptotic bodies, and oncosomes are large EVs (a few thousand nanometers) that have been found to be associated with migration, phagocytosis, and cancer, respectively. Migrasomes are newly identified organelles that depend on migration, leaving long retractable fibers upon cell migration, and vesicles grow atop the tips and intersections of fibers. Eventually, the fibers that connect the vesicles to the main cell body break apart, and the vesicles are released into the extracellular space or absorbed directly by the surrounding cells (41). Apoptotic bodies, small bodies released by programmed cell death, can be formed in two ways: the sprouting and shedding mechanism and the autophagosome mechanism (42). The term “oncosomes” was originally used to describe abnormally large EVs, although it is often used to refer to EVs released by cancer cells (43). Oncosomes derived from prostate cancer cells strongly promote the establishment of a tumor-supporting environment by inducing new interstitial reprogramming (44). In fact, EVs should not be classified into subtypes according to their sizes because their diameters overlap; for example, some MVs, whose size range is very large (100–1,000 nm), can be easily confused with large exosomes (45). At present, the origin is the only basis for distinguishing exosomes from other EVs. Other EVs are formed by the protrusion and shedding of cell membranes, whereas exosomes are derived from intracellular endosomes, which can form MVBs that are then degraded by lysosomes or fused with the cytoplasmic membrane, released and enter the receiving cell through fusion, endocytosis or receptors (46). According to the MISEV2018 guidelines, exploring the biogenesis of EVs remains a challenge without the use of live imaging techniques. Therefore, operational terms are still recommended for the description of EV subtypes according to their size, density, biochemical composition, and cell or organ origin (47).
The exosome formation process involves invagination of the cell membrane to form an endosome, which then develops into MVBs. Some of these MVBs directly fuse with lysosomes and degrade, some are transported to the Golgi for recovery, and some fuse with the cell membrane to release small vesicles outside of the cell and form exosomes. Regarding the mechanisms associated with exosome biogenesis and abscission, many molecules play an important role. First, the endosomal sorting complex required for transport (ESCRT) and other proteins, such as tumor susceptibility gene 101 protein (TSG101) and ALG-2 interacting protein X (ALIX), are involved in cargo sorting into exosomes (3). Apart from ESCRT, other ESCRT-independent mechanisms, including lipid rafts and tetraspanins CD63 and CD81, are conducive to exosome biogenesis (48). Finally, the Rab-GTPase family contributes to the intracellular trafficking and fusion of MVBs with the cell membrane to release exosomes. Some studies clarified that sphingomyelinase, protein kinase D family, and argonaute-2 are involved in the formation of exosomes (49) (Figure 1).
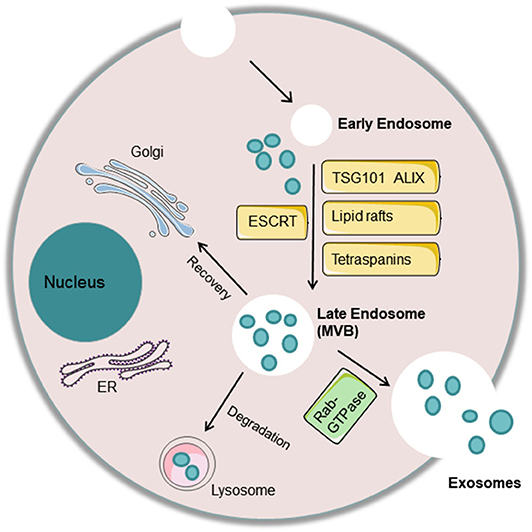
Figure 1. Exosome biogenesis and abscission. The cell membrane invaginates to form an endosome, which then develops into a late endosome or multivesicular body (MVB) with processing of the rough endoplasmic reticulum and Golgi. Some of these MVBs directly fuse with lysosomes and degrade, some are transported to the Golgi for recovery, and some fuse with the cell membrane to release small vesicles outside of the cell and form exosomes. Many molecules play an important role in exosome biogenesis and abscission. First, the endosomal sorting complex required for transport (ESCRT) and other proteins, such as tumor susceptibility gene 101 protein (TSG101) and ALG-2 interacting protein X (ALIX), are involved in cargo sorting into exosomes. In addition, other ESCRT-independent mechanisms, including lipid rafts and tetraspanins CD63 and CD81, are conducive to exosome biogenesis. Finally, the Rab-GTPase family contributes to the intracellular trafficking and fusion of MVBs with the cell membrane to release exosomes.
Exosomes are composed of nucleic acids (including DNA and RNA), proteins, and lipids. Exosomal RNAs mainly play key roles in the target cell and mainly include mRNAs, microRNAs, lncRNAs, circRNAs, etc. (50). MicroRNAs are now the most widely and deeply studied type of RNA in exosomes, often due to their relationship with the occurrence and development of diseases (51). Exosomal proteins can be divided into membrane proteins and intramembrane proteins. Membrane proteins, including tetraspanins (CD63, CD81, and CD9) and some cell-specific proteins, such as A33 (colon epithelial cell source), MHC-II, and CD86 (antigen-presenting cell sources), participate in exosome transport. Intracellular exosomal proteins include the heat shock protein family (HSP60, HSP70, HSP90, HSPA5, and CCT2), a variety of metabolic enzymes (GAPDH, PKM2, PGK1, PDIA3, antioxidant proteins), ribosomal proteins (RPS3), signal transduction factors (melanoma differentiation-related factors, ARF1, CDC42), adhesion factors (MFGE8, integrin), cytoskeletal proteins, and ubiquitin (52, 53). Lipids are important components of the exosomal membrane, and exosomes contain more specific lipids than parent cells. Several studies have found that the percentages of different lipid categories in cells and exosomes vary among several cell types, such as human B cells and dendritic cells. Specifically, in human B cells, cholesterol, and sphingomyelins have been found to be enriched from cells to exosomes (54) (Figure 2).
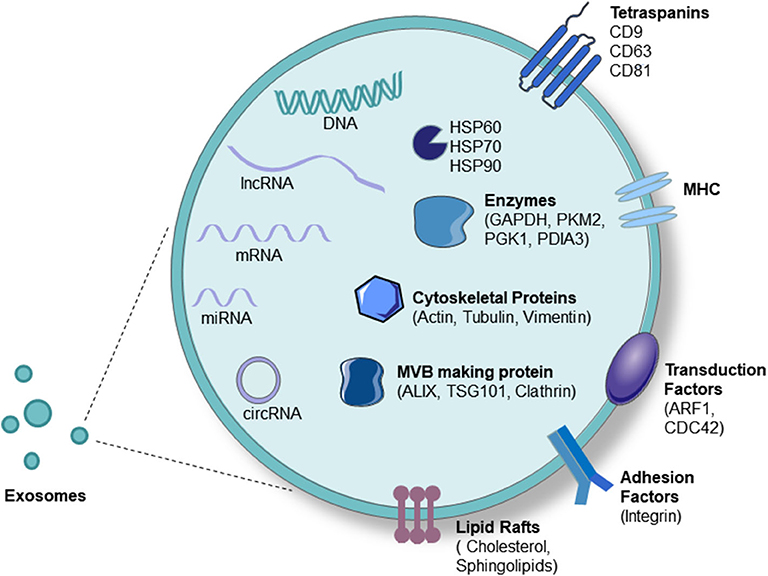
Figure 2. Exosome components. Exosomes carry proteins, nucleic acids, and lipids. Proteins include tetraspanins (CD63, CD81, and CD9), some cell-specific proteins such as MHC-II (antigen-presenting cell source), heat-shock protein family (HSP60, HSP70, HSP90), a variety of metabolic enzymes (GAPDH, PKM2, PGK1, PDIA3, antioxidant proteins), signal transduction factors (ARF1, CDC42), adhesion factors (MFGE8, integrin), cytoskeletal proteins (Actin, Tubulin, Vimentin), and MVB-producing proteins (Alixs, Tsg101, Clathrin). Nucleic acids include DNA, mRNA, microRNA, lncRNA, and circRNA.
Exosomes have several functions. First, they function as a shuttle bus between cells, mediate cell-cell communication and play a role in immunity. Exosomes have been identified as important mediators of intercellular communication through the transfer of encapsulated cargo, such as bioactive lipids, non-coding RNAs, mRNAs, and proteins (55). These bioactive molecules are stable because they are covered with a biofilm capable of avoiding degradation. In addition, due to the characteristics of their surface proteins, exosomes also show relatively high target specificity to receptor cells. All these characteristics make exosomes important mediators of communication between cells, especially between organs. Second, the occurrence and progression of diseases [e.g., tumor metastasis (56–58), cardiovascular disease risk (59, 60), neurological diseases (61, 62)] can be predicted by studying the relationship among the exosome type, number, size, and content. Finally, several recent studies have reported on targeted drugs for exosomes. Exosomes can be used as carriers to deliver drugs to target areas, providing hope for the treatment of many diseases (63, 64).
Hepatitis Virus
Hepatitis B virus (HBV) infection is characterized by long-term chronic infection accompanied by hepatocyte injury due to the complicated interaction between HBV and the immune system. In addition, according to the World Health Organization, more than 185 million people are infected with hepatitis C virus (HCV) (65). In the process of HCV infection, the interaction between macrophages and hepatocytes is an important part of liver innate immunity.
HBV encodes a microRNA (HBV-miR-3) that inhibits HBV replication by impeding transcription. Type I interferons (IFNs) constitute important immune responses to viral infection and can thus be used to treat some infectious viruses, including HBV and HCV. IFN-I interacts with its receptor to activate the Janus kinase (JAK)/STAT pathway, and STAT1/2 is then phosphorylated and transferred to the nucleus to bind to the IFN-stimulating response element, initiating the transcription of IFN-stimulated genes. SOCS5, an E3 ubiquitin ligase, negatively regulates the mechanism described above; specifically, it inhibits JAK kinase activity by interacting with JAKs through its JAK interaction region. Exosomal HBV-miR-3 promotes macrophage differentiation into the M1 phenotype and IL-6 secretion through the SOCS5/STAT1 pathway (21). Macrophage exosomes rely on T cell immunoglobulin and the hepatitis A virus receptor mucin receptor 1 to enter liver cells and then promote anti-HBV activity induced by IFN-α. In addition, the two main endocytic pathways for viral infection, namely, reticular protein-mediated endocytosis and macrophage phagocytosis, cooperate to allow exosome entry into liver cells and transfer of this activity (66). HBV-infected hepatocyte exosomes carry viral nucleic acids and prompt the expression of NKG2D ligands in macrophages. Compared to normal hepatocytes, HBV-infected hepatocyte exosomes show higher expression levels of immunoregulatory microRNAs, which are transported to macrophages and then restrict IL-12p35 mRNA expression in macrophages, leading to resistance to the host's inherent immune response (22).
Exosomes derived from macrophages play a key role in inhibiting the replication of HCV. TLR3-activated macrophages release exosomes containing anti-HCV microRNA (miRNA)-29 family members (23). Further studies show that interferon-stimulated macrophage-derived EVs inhibit HCV replication and participate in antiviral immune responses, while polyunsaturated fatty acids weaken this process (67). On the other hand, exosomes can also affect macrophages. Concretely, monocytes tend to differentiate into macrophages that show high expression of M2 surface markers and produce pro- and anti-inflammatory cytokines when cocultured with exosome-packaged HCV, which is mediated by TLR7/8 (24).
Alcoholic Liver Disease
Alcoholic liver disease (ALD) or alcoholic hepatitis (AH) is a liver disease caused by long-term heavy drinking. The effects of alcohol, alcohol metabolites, and gut-derived endotoxins cause liver damage in patients with ALD (68, 69). The initial manifestation is usually fatty liver, which can develop into AH, liver fibrosis, and liver cirrhosis (70). Approximately 3.3 million people die each year from excessive drinking, accounting for almost 5.9% of all global deaths. According to the World Health Organization, Europe has the highest amount of alcohol consumption per adult. In EU countries, 41% of all liver deaths are attributed to alcohol. Since ALD patients have not shown any clinical symptoms or abnormal laboratory indicators in the past, screening should be carried out in high-risk groups (71).
A recent study focused on the potential correlation between autophagy and exosomes since autophagosome and exosome biogenesis involve the same components. The researchers found autophagy damage in ALD and AH mouse models and in the livers of patients with ALD. Moreover, this autophagy occurs at the lysosome level by reducing the expression of lysosome-associated membrane protein 1 (LAMP1) and lysosome-associated membrane protein 2 (LAMP2). The expression of microRNA 155 (miR-155) is increased by alcohol, and its action targets are LAMP1, LAMP2, mechanistic target of rapamycin, and Ras homolog enriched in the brain. In line with this, miR-155 gene-deficient mice exhibited less alcohol-induced autophagic damage and less exosome production than control mice. Downregulation of LAMP1 or LAMP2 increases the number of exosomes released by hepatocytes and macrophages. These results reveal that the increased exosome content induced by alcohol is related to the destruction of autophagy and the impaired function of autophagosomes and lysosomes (25). Another study clarified that atypical exosomes can eliminate lysosomal waste to combat lysosomal dysfunction, thus maintaining dynamic equilibrium (72). In addition, researchers have found that EVs in patients with ALD carry a unique protein cargo and induce macrophage activation by heat shock protein 90 (73). Another study found that alcohol increases the EV (mainly exosomes) production of primary human monocytes and THP-1 monocytes, and monocytes exposed to alcohol communicate with primitive monocytes through EVs. Furthermore, miR-27A in exosomes polarizes primitive monocytes into M2 macrophages (26). Similarly, patients with AH and alcohol-fed mice produced more EVs than normal controls. Exosomal miRNA-192, miRNA-122, and miRNA-30a secreted into the blood could be used as diagnostic biomarkers of ALD (74). In addition, a previous study in mice demonstrated that ethanol promotes the secretion of EVs via CYP2E1 and revealed for the first time that the caspase-3 pathway is involved in this process. EVs contain CD40L (TNFSF5) and can activate pro-inflammatory macrophages (27). Liver cells exposed to alcohol secrete exosomes containing increased concentrations of miR-122, which is absorbed by macrophages and makes them sensitive to lipopolysaccharide (LPS), thereby enhancing cytokine secretion (18). In addition, mitochondria have also attracted much attention. Ethanol exposure can activate toll-like receptor 3 in Kupffer cells by hepatic mitochondrial double-stranded RNA (MtdsRNA) through exosomal delivery, resulting in increased IL-1β levels, which promotes the production of IL-17A. MtdsRNA and TLR3 can be used as therapeutic targets for ALD (28, 75). Hence, blocking these pathways may protect against alcohol-induced liver injury.
Non-Alcoholic Fatty Liver Disease
NAFLD is characterized by the excessive accumulation of liver fat and insulin resistance, which is defined by histological analysis as >5% hepatocyte steatosis or by proton density as a fat content of >5.6%. NAFLD includes two kinds of pathological diagnoses with different prognoses: non-alcoholic fatty liver (NAFL) and non-alcoholic steatohepatitis (NASH). The latter is more severe than the former and includes fibrosis, liver cirrhosis, and HCC (76). High-calorie diets, excessive intake of saturated fats, refined carbohydrates, sugary drinks, and fructose and Western diets are all associated with increased body mass, obesity, and especially NAFLD (77). High-fructose intake increases the risk of NASH and advanced liver fibrosis (78, 79). In addition, it is generally recognized that monocyte-derived macrophages recruited in the liver are involved in the inflammatory response of NASH.
The pathological features of NASH are lipid-induced hepatocyte apoptosis (apoptosis induced by toxic lipid mediators) and infiltration by inflammatory cells, some of which are activated macrophages (80). The latest research indicates that the number and miR-192-5p level of serum exosomes in NASH patients, and NASH model rats are significantly higher than those in their respective control groups. Furthermore, the exosomes released by lipotoxic hepatocytes can be ingested by macrophages, resulting in activation of M1 macrophages and hepatic inflammation by regulating the Rictor/Akt/FoxO1 signaling pathway (29). Another study showed that in a mouse model of NASH, EVs derived from lipotoxic hepatocytes are rich in active integrin β1 (ITGβ1), mediating the adhesion of monocytes to hepatic sinusoidal endothelial cells, which is a necessary step in hepatic inflammation. ITGβ1 inhibition reduces liver injury (31). In addition, it has been reported that exosomes isolated from melatonin-treated adipocytes significantly attenuate liver steatosis induced by a high-fat diet and resistin-mediated ER stress. Further research has shown that melatonin reduces the level of exosomal resistin derived from adipocytes through Bmal1 transcription inhibition and M6A RNA demethylation in adipocytes (81). Several studies have observed that macrophage-derived exosomes contribute to insulin resistance through paracrine or endocrine mechanisms (55, 82, 83). Another study found elevated levels of exosomes derived from natural killer T cells and macrophages among patients with NAFLD or NASH (84). Moreover, lipids have been shown to stimulate death receptor 5, promoting the release of EVs from hepatocytes; subsequently, these EVs activate the inflammatory phenotype in macrophages, which ultimately causes NASH (30). Cholesterol damages the lysosomal function of hepatocytes, leading to the secretion of hepatocyte-derived exosomal miR122-5p, which enters macrophages to promote M1 polarization and the occurrence of inflammation (32). Hepatocytes treated with ezetimibe can inhibit inflammasome formation in macrophages and IL-1 secretion as well as alleviate NASH liver inflammation through exosomes (85).
Acute Liver Failure
Acute liver failure (ALF), a clinical syndrome characterized by jaundice, ascites, hepatic encephalopathy, and coagulation dysfunction, refers to the extensive necrosis of hepatocytes or severe liver function damage caused by various factors, such as viruses, drugs, and toxins. The treatments for ALF are liver transplantation and artificial liver therapy. However, there are limitations associated with liver transplantation due to a lack of appropriate donor livers and a variety of complications. Additionally, the efficacy of artificial liver therapy is relatively limited (86).
The transplantation of mesenchymal stem cells (MSCs) might become a potential approach for treating liver disease (87). Researchers administered human umbilical cord MSC-derived exosomes (hucMSC-Ex) to mice via their tail vein or oral gavage. The hucMSC-Exs exhibited antioxidant functions and antiapoptotic effects and rescued the mice from liver failure induced by CCl4 (88). Another study further explored the role of macrophages in this process. The researchers treated mice with LPS and D-galactosamine (LPS/GalN) and immediately injected adipose MSC (AMSC)-derived exosomes (AMSC-Exos) intravenously. AMSC-Exos colocalized with hepatic macrophages and reduced the secretion of inflammatory factors by inhibiting the activation of inflammatory factors in macrophages. Exosome-encapsulated miR-17 plays an important role in the treatment of ALF by targeting TXNIP and inhibiting the activation of inflammatory factors in hepatic macrophages (33). Exosomes secreted by MSCs may improve the therapeutic efficacy of MSCs by mediating intercellular communication and transporting paracrine factors (89).
HCC
The incidence of liver cancer is on the rise worldwide, with the number of newly diagnosed cases increasing by 75% between 1990 and 2015 (90). It is predicted that liver cancer will be the sixth most common cancer in the world and the fourth-largest cause of cancer-related death. According to statistics by the International Agency for Research on Cancer, there were approximately 842,080 new cases of liver cancer and 781,631 deaths in 2018. Liver cancer includes HCC (75–85% of cases), intrahepatic cholangiocarcinoma (10–15% of cases) and other rare types (91). Because patients with early HCC exhibit no obvious clinical symptoms, early diagnosis is quite difficult. Currently, screening methods for HCC rely on mainly serum tumor markers and imaging tests. Clinical serological tests include α-fetoprotein (AFP), des-γ-carboxy prothrombin, and the AFP-L3 fraction. Imaging-based diagnostic methods include computed tomography and magnetic resonance imaging. If necessary, pathological examination may be used, but this method is not ideal in early HCC monitoring (92–94). Surgical resection is suggested as the first choice for the treatment of HCC patients with non-cirrhosis. However, those who undergo surgery have a recurrence rate of 70% (95). Therefore, a need exists for improved diagnostic and treatment methods for liver cancer.
Recent studies have shown that tumor-derived exosomes can be absorbed by fibroblasts and macrophages in the tumor microenvironment, change their phenotype, and ultimately promote tumor progression and metastasis (96). Several studies have noted that HCC-derived exosomes can be ingested by macrophages and thereby promote tumor progression. A recent study showed that exosomes derived from HCC contain a large amount of the lncRNA TUC339, which is taken up along with exosomes by macrophages in the tumor microenvironment, reducing the secretion of pro-inflammatory cytokines from these macrophages, increasing the secretion of anti-inflammatory cytokines, and causing the phenotypic conversion of macrophages. These phenotypically transformed macrophages can inhibit immune-mediated tumor cell death and promote tumor immune escape, thus facilitating rapid tumor growth progression (34). The exosomes secreted by hepatoma cells and released by melatonin-induced hepatoma cells can be phagocytosed and ingested by macrophages. The immune response is affected by regulating the expression of PD-L1 and the inflammatory factors IL-6, IL-10, and TNF-α. The melatonin-induced release of exosomes from HCC cells downregulates the expression of PD-L1 in macrophages by downregulating the protein expression of STAT3 (20). Another HCC study showed that endoplasmic reticulum (ER)-stressed HCC cells release exosomes, upregulate PD-L1 expression in macrophages, and then inhibit T cell function through the exosomal miR-23a-PTEN-AKT pathway. These results provide new insights into how tumor cells escape antitumor immunity (35). Hepatoma cells transmit miRNA-21 to hepatic stellate cells and activate the tumor suppressor gene PTEN through exosomes to activate the transition of hepatic stellate cells into cancer-associated fibroblasts (CAFs) via the PDK1/AKT signaling pathway. Activated CAFs further secrete angiogenic cytokines, including vascular endothelial growth factor (VEGF), MMP2, and MMP9, increasing the number of blood vessels and promoting the development of HCC (97). In contrast, the expression of miR-122 in serum or circulating exosomes is lower in HCC patients than in healthy subjects (98, 99). In tumor-bearing mice, propofol inhibits the invasion of HCC cells by stimulating the transfer of microvesicular miR-142-3p from tumor-associated macrophages to HCC cells (36). ER stress induces the release of exosomes from HCC cells, and by regulating the expression of programmed death ligand 1 in macrophages, the mir-23a-PTEN-AKT pathway inhibits T cell function and weakens antitumor immunity (35). Macrophages and exosomes also play an important role in tumor metastasis. Some scholars have found that in pancreatic ductal adenocarcinoma cells, tumor-derived exosomes can recruit bone marrow-derived macrophages to form a preliver metastatic environment and promote tumor metastasis (100).
Exosome Roles in Prognosis and Treatment
In the process of HCV infection, the interaction between retained macrophages and hepatocytes is an important part of liver innate immunity. Exosomes derived from macrophages play a key role in inhibiting the replication of HCV. Further study shows that TLR3-activated macrophages release exosomes containing anti-HCV miRNA-29 family members (23). Virus entry mechanisms and pathways have also been applied to study the exosome-mediated transfer of antiviral activity between cells. In HBV infection, macrophage-derived exosomes can use hepatitis A virus receptors to enter liver cells. Subsequently, exosomes utilize clathrin-mediated endocytosis and macrophage phagocytosis and then fuse with endosomes to effectively transmit the anti-HBV activity induced by IFN-α (66). Together, these studies suggest that exosomes have great potential as delivery vehicles for disease treatment. Exosomes can also be used for prognostic analyses. Circulating EV concentrations and sphingolipid carrier characteristics can be used not only for the diagnosis and differentiation of AH, decompensated alcoholic cirrhosis, and other end-stage liver diseases but also for the prediction of the 90-day survival time (101).
Conclusions and Perspectives
Macrophage activation is an important force driving liver injury. Exosomes are important vesicles that are released by almost all cell types and play an important role in intercellular communication. Increasing evidence indicates that exosomes have outstanding functions, suggesting their potential use for future applications. In all liver diseases, studies on the effects and connections between macrophages and exosomes have concentrated on ALD, NAFLD, and HCC areas and have provided ideas for the non-invasive diagnosis and treatment of these diseases (Table 1). Generally, exosomes from damaged hepatocytes or tumors can promote the activation and differentiation of macrophages, thereby promoting inflammation. On the other hand, macrophage-derived exosomes also play a role in target hepatocytes (Figure 3). Nevertheless, the identification of novel specific biomarkers is required. In addition, it is worth investigating macrophages and exosomes in other liver diseases.
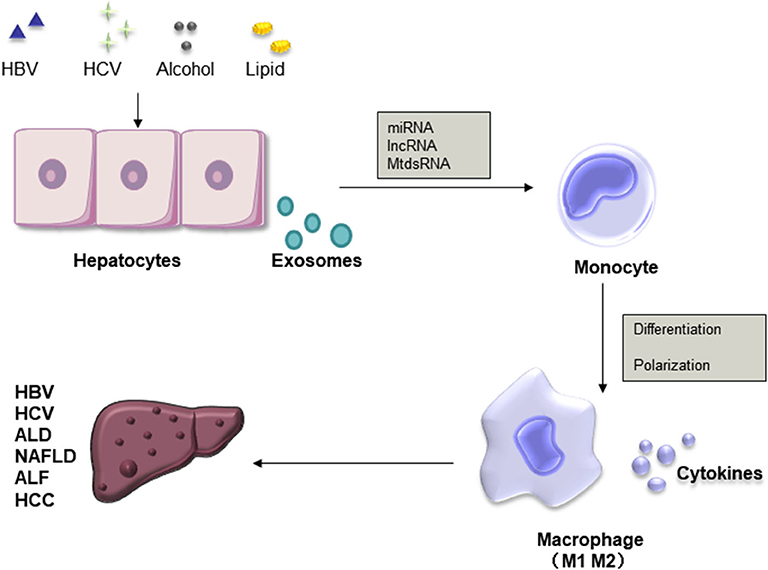
Figure 3. Roles of exosomes and macrophages in liver diseases. Exosomal miRNAs, lncRNAs, and MtdsRNAs released from injured hepatocytes promote the differentiation of macrophages into the M1 or M2 phenotype and the secretion of cytokines, thereby promoting inflammation.
Author Contributions
MS and YS drafted the manuscript. LY and TY conceived the idea. XF and RM provided critical feedback. All authors read and approved the final version. All authors contributed to the article and approved the submitted version.
Funding
This manuscript was funded by the National Natural Science Foundation of China (No. 81770568 to LY), the 1.3.5 project for disciplines of excellence, West China Hospital, Sichuan University (No. ZYGD20012 to LY), and the 1.3.5 project for disciplines of excellence, West China Hospital, Sichuan University (No. ZYJC18008 to LY).
Conflict of Interest
The authors declare that the research was conducted in the absence of any commercial or financial relationships that could be construed as a potential conflict of interest.
References
1. Colombo M, Raposo G, Thery C. Biogenesis, secretion, and intercellular interactions of exosomes and other extracellular vesicles. Annu Rev Cell Dev Biol. (2014) 30:255–89. doi: 10.1146/annurev-cellbio-101512-122326
2. Thery C, Zitvogel L, Amigorena S. Exosomes: composition, biogenesis and function. Nat Rev Immunol. (2002) 2:569–79. doi: 10.1038/nri855
3. Andaloussi SEL, Mager I, Breakefield XO, Wood MJ. Extracellular vesicles: biology and emerging therapeutic opportunities. Nat Rev Drug Discov. (2013) 12:347–57. doi: 10.1038/nrd3978
4. Bang C, Thum T. Exosomes: new players in cell-cell communication. Int J Biochem Cell Biol. (2012) 44:2060–4. doi: 10.1016/j.biocel.2012.08.007
5. Vlassov AV, Magdaleno S, Setterquist R, Conrad R. Exosomes: current knowledge of their composition, biological functions, and diagnostic and therapeutic potentials. Biochim Biophys Acta. (2012) 1820:940–8. doi: 10.1016/j.bbagen.2012.03.017
6. Raposo G, Stoorvogel W. Extracellular vesicles: exosomes, microvesicles, and friends. J Cell Biol. (2013) 200:373–83. doi: 10.1083/jcb.201211138
7. Tkach M, Thery C. Communication by extracellular vesicles: where we are and where we need to go. Cell. (2016) 164:1226–32. doi: 10.1016/j.cell.2016.01.043
8. Jabbari N, Akbariazar E, Feqhhi M, Rahbarghazi R, Rezaie J. Breast cancer-derived exosomes: tumor progression and therapeutic agents. J Cell Physiol. (2020) 235:6345–56. doi: 10.1002/jcp.29668
9. Sato K, Meng F, Glaser S, Alpini G. Exosomes in liver pathology. J Hepatol. (2016) 65:213–21. doi: 10.1016/j.jhep.2016.03.004
10. Tacke F, Zimmermann HW. Macrophage heterogeneity in liver injury and fibrosis. J Hepatol. (2014) 60:1090–6. doi: 10.1016/j.jhep.2013.12.025
11. Wynn TA, Vannella KM. Macrophages in tissue repair, regeneration, and fibrosis. Immunity. (2016) 44:450–62. doi: 10.1016/j.immuni.2016.02.015
12. Lawrence T, Natoli G. Transcriptional regulation of macrophage polarization: enabling diversity with identity. Nat Rev Immunol. (2011) 11:750–61. doi: 10.1038/nri3088
13. Mantovani A, Sica A, Sozzani S, Allavena P, Vecchi A, Locati M. The chemokine system in diverse forms of macrophage activation and polarization. Trends Immunol. (2004) 25:677–86. doi: 10.1016/j.it.2004.09.015
14. Bala S, Csak T, Saha B, Zatsiorsky J, Kodys K, Catalano D, et al. The pro-inflammatory effects of miR-155 promote liver fibrosis and alcohol-induced steatohepatitis. J Hepatol. (2016) 64:1378–87. doi: 10.1016/j.jhep.2016.01.035
15. Kazankov K, Jorgensen SMD, Thomsen KL, Moller HJ, Vilstrup H, George J, et al. The role of macrophages in nonalcoholic fatty liver disease and nonalcoholic steatohepatitis. Nat Rev Gastroenterol Hepatol. (2019) 16:145–59. doi: 10.1038/s41575-018-0082-x
16. Weiskirchen R, Tacke F. Liver fibrosis: from pathogenesis to novel therapies. Dig Dis. (2016) 34:410–22. doi: 10.1159/000444556
17. Yin Z, Huang J, Ma T, Li D, Wu Z, Hou B, et al. Macrophages activating chemokine (C-X-C motif) ligand 8/miR-17 cluster modulate hepatocellular carcinoma cell growth and metastasis. Am J Transl Res. (2017) 9:2403–11.
18. Momen-Heravi F, Bala S, Kodys K, Szabo G. Exosomes derived from alcohol-treated hepatocytes horizontally transfer liver specific miRNA-122 and sensitize monocytes to LPS. Sci Rep. (2015) 5:9991. doi: 10.1038/srep09991
19. Li X, Liu R, Wang Y, Zhu W, Zhao D, Wang X, et al. Cholangiocyte-derived exosomal lncRNA H19 promotes macrophage activation and hepatic inflammation under cholestatic conditions. Cells. (2020) 9:190. doi: 10.3390/cells9010190
20. Cheng L, Liu J, Liu Q, Liu Y, Fan L, Wang F, et al. Exosomes from melatonin treated hepatocellularcarcinoma cells alter the immunosupression status through STAT3 pathway in macrophages. Int J Biol Sci. (2017) 13:723–34. doi: 10.7150/ijbs.19642
21. Zhao X, Sun L, Mu T, Yi J, Ma C, Xie H, et al. An HBV-encoded miRNA activates innate immunity to restrict HBV replication. J Mol Cell Biol. (2020) 12:263–76. doi: 10.1093/jmcb/mjz104
22. Kouwaki T, Fukushima Y, Daito T, Sanada T, Yamamoto N, Mifsud EJ, et al. Extracellular vesicles including exosomes regulate innate immune responses to hepatitis B virus infection. Front Immunol. (2016) 7:335. doi: 10.3389/fimmu.2016.00335
23. Zhou Y, Wang X, Sun L, Zhou L, Ma TC, Song L, et al. Toll-like receptor 3-activated macrophages confer anti-HCV activity to hepatocytes through exosomes. FASEB J. (2016) 30:4132–40. doi: 10.1096/fj.201600696R
24. Saha B, Kodys K, Adejumo A, Szabo G. Circulating and exosome-packaged hepatitis c single-stranded RNA induce monocyte differentiation via TLR7/8 to polarized macrophages and fibrocytes. J Immunol. (2017) 198:1974–84. doi: 10.4049/jimmunol.1600797
25. Babuta M, Furi I, Bala S, Bukong TN, Lowe P, Catalano D, et al. Dysregulated autophagy and lysosome function are linked to exosome production by micro-RNA 155 in alcoholic liver disease. Hepatology. (2019) 70:2123–41. doi: 10.1002/hep.30766
26. Saha B, Momen-Heravi F, Kodys K, Szabo G. MicroRNA cargo of extracellular vesicles from alcohol-exposed monocytes signals naive monocytes to differentiate into M2 macrophages. J Biol Chem. (2016) 291:149–59. doi: 10.1074/jbc.M115.694133
27. Verma VK, Li H, Wang R, Hirsova P, Mushref M, Liu Y, et al. Alcohol stimulates macrophage activation through caspase-dependent hepatocyte derived release of CD40L containing extracellular vesicles. J Hepatol. (2016) 64:651–60. doi: 10.1016/j.jhep.2015.11.020
28. Lee JH, Shim YR, Seo W, Kim MH, Choi WM, Kim HH, et al. Mitochondrial double-stranded RNA in exosome promotes interleukin-17 production through toll-like receptor 3 in alcoholic liver injury. Hepatology. (2019) 72:609–25. doi: 10.1002/hep.31041
29. Liu XL, Pan Q, Cao HX, Xin FZ, Zhao ZH, Yang RX, et al. Lipotoxic hepatocyte-derived exosomal miR-192-5p activates macrophages via Rictor/Akt/FoxO1 signaling in NAFLD. Hepatology. (2019) 72:454–69. doi: 10.1002/hep.31050
30. Hirsova P, Ibrahim SH, Krishnan A, Verma VK, Bronk SF, Werneburg NW, et al. Lipid-Induced signaling causes release of inflammatory extracellular vesicles from hepatocytes. Gastroenterology. (2016) 150:956–67. doi: 10.1053/j.gastro.2015.12.037
31. Guo Q, Furuta K, Lucien F, Gutierrez Sanchez LH, Hirsova P, Krishnan A, et al. Integrin beta1-enriched extracellular vesicles mediate monocyte adhesion and promote liver inflammation in murine NASH. J Hepatol. (2019) 71:1193–205. doi: 10.1016/j.jhep.2019.07.019
32. Zhao Z, Zhong L, Li P, He K, Qiu C, Zhao L, et al. Cholesterol impairs hepatocyte lysosomal function causing M1 polarization of macrophages via exosomal miR-122-5p. Exp Cell Res. (2020) 387:111738. doi: 10.1016/j.yexcr.2019.111738
33. Liu Y, Lou G, Li A, Zhang T, Qi J, Ye D, et al. AMSC-derived exosomes alleviate lipopolysaccharide/d-galactosamine-induced acute liver failure by miR-17-mediated reduction of TXNIP/NLRP3 inflammasome activation in macrophages. EBioMedicine. (2018) 36:140–50. doi: 10.1016/j.ebiom.2018.08.054
34. Li X, Lei Y, Wu M, Li N. Regulation of macrophage activation and polarization by HCC-derived exosomal lncRNA TUC339. Int J Mol Sci. (2018) 19:2958. doi: 10.3390/ijms19102958
35. Liu J, Fan L, Yu H, Zhang J, He Y, Feng D, et al. Endoplasmic reticulum stress causes liver cancer cells to release exosomal miR-23a-3p and up-regulate programmed death ligand 1 expression in macrophages. Hepatology. (2019) 70:241–58. doi: 10.1002/hep.30607
36. Zhang J, Shan WF, Jin TT, Wu GQ, Xiong XX, Jin HY, et al. Propofol exerts anti-hepatocellular carcinoma by microvesicle-mediated transfer of miR-142-3p from macrophage to cancer cells. J Transl Med. (2014) 12:279. doi: 10.1186/s12967-014-0279-x
37. Maas SLN, Breakefield XO, Weaver AM. Extracellular vesicles: unique intercellular delivery vehicles. Trends Cell Biol. (2017) 27:172–88. doi: 10.1016/j.tcb.2016.11.003
38. Zhang H, Freitas D, Kim HS, Fabijanic K, Li Z, Chen H, et al. Identification of distinct nanoparticles and subsets of extracellular vesicles by asymmetric flow field-flow fractionation. Nat Cell Biol. (2018) 20:332–43. doi: 10.1038/s41556-018-0040-4
39. Wu AY, Ueda K, Lai CP. Proteomic analysis of extracellular vesicles for cancer diagnostics. Proteomics. (2019) 19:e1800162. doi: 10.1002/pmic.201800162
40. Meldolesi J. Exosomes and ectosomes in intercellular communication. Curr Biol. (2018) 28:R435–44. doi: 10.1016/j.cub.2018.01.059
41. Ma L, Li Y, Peng J, Wu D, Zhao X, Cui Y, et al. Discovery of the migrasome, an organelle mediating release of cytoplasmic contents during cell migration. Cell Res. (2015) 25:24–38. doi: 10.1038/cr.2014.135
42. Crescitelli R, Lasser C, Szabo TG, Kittel A, Eldh M, Dianzani I, et al. Distinct RNA profiles in subpopulations of extracellular vesicles: apoptotic bodies, microvesicles and exosomes. J Extracell Vesicles. (2013) 2:20677. doi: 10.3402/jev.v2i0.20677
43. Meehan B, Rak J, Di Vizio D. Oncosomes - large and small: what are they, where they came from? J Extracell Vesicles. (2016) 5:33109. doi: 10.3402/jev.v5.33109
44. Minciacchi VR, Spinelli C, Reis-Sobreiro M, Cavallini L, You S, Zandian M, et al. MYC mediates large oncosome-induced fibroblast reprogramming in prostate cancer. Cancer Res. (2017) 77:2306–17. doi: 10.1158/0008-5472.CAN-16-2942
45. Zaborowski MP, Balaj L, Breakefield XO, Lai CP. Extracellular vesicles: composition, biological relevance, and methods of study. Bioscience. (2015) 65:783–97. doi: 10.1093/biosci/biv084
46. Genschmer KR, Russell DW, Lal C, Szul T, Bratcher PE, Noerager BD, et al. Activated PMN exosomes: pathogenic entities causing matrix destruction and disease in the lung. Cell. (2019) 176:113–26.e15. doi: 10.1016/j.cell.2018.12.002
47. Thery C, Witwer KW, Aikawa E, Alcaraz MJ, Anderson JD, Andriantsitohaina R, et al. Minimal information for studies of extracellular vesicles 2018. (MISEV2018): a position statement of the International Society for Extracellular Vesicles and update of the MISEV2014 guidelines. J Extracell Vesicles. (2018) 7:1535750. doi: 10.1080/20013078.2018.1461450
48. Rezaie J, Nejati V, Khaksar M, Oryan A, Aghamohamadzadeh N, Shariatzadeh MA, et al. Diabetic sera disrupted the normal exosome signaling pathway in human mesenchymal stem cells in vitro. Cell Tissue Res. (2018) 374:555–65. doi: 10.1007/s00441-018-2895-x
49. Rezaie J, Rahbarghazi R, Pezeshki M, Mazhar M, Yekani F, Khaksar M, et al. Cardioprotective role of extracellular vesicles: a highlight on exosome beneficial effects in cardiovascular diseases. J Cell Physiol. (2019) 234:21732–45. doi: 10.1002/jcp.28894
50. Lloret-Llinares M, Karadoulama E, Chen Y, Wojenski LA, Villafano GJ, Bornholdt J, et al. The RNA exosome contributes to gene expression regulation during stem cell differentiation. Nucleic Acids Res. (2018) 46:11502–13. doi: 10.1093/nar/gky817
51. Neviani P, Fabbri M. Exosomic microRNAs in the tumor microenvironment. Front Med. (2015) 2:47. doi: 10.3389/fmed.2015.00047
52. Dorayappan KDP, Gardner ML, Hisey CL, Zingarelli RA, Smith BQ, Lightfoot MDS, et al. A microfluidic chip enables isolation of exosomes and establishment of their protein profiles and associated signaling pathways in ovarian cancer. Cancer Res. (2019) 79:3503–13. doi: 10.1158/0008-5472.CAN-18-3538
53. Jabbari N, Karimipour M, Khaksar M, Akbariazar E, Heidarzadeh M, Mojarad B, et al. Tumor-derived extracellular vesicles: insights into bystander effects of exosomes after irradiation. Lasers Med Sci. (2020) 35:531–45. doi: 10.1007/s10103-019-02880-8
54. Skotland T, Sandvig K, Llorente A. Lipids in exosomes: current knowledge and the way forward. Prog Lipid Res. (2017) 66:30–41. doi: 10.1016/j.plipres.2017.03.001
55. Ying W, Riopel M, Bandyopadhyay G, Dong Y, Birmingham A, Seo JB, et al. Adipose tissue macrophage-derived exosomal miRNAs can modulate in vivo and in vitro insulin sensitivity. Cell. (2017) 171:372–84.e12. doi: 10.1016/j.cell.2017.08.035
56. Hoshino A, Costa-Silva B, Shen TL, Rodrigues G, Hashimoto A, Tesic Mark M, et al. Tumour exosome integrins determine organotropic metastasis. Nature. (2015) 527:329–35. doi: 10.1038/nature15756
57. Lan J, Sun L, Xu F, Liu L, Hu F, Song D, et al. M2 macrophage-derived exosomes promote cell migration and invasion in colon cancer. Cancer Res. (2019) 1:146–58. doi: 10.1158/0008-5472.CAN-18-0014
58. Jabbari N, Nawaz M, Rezaie J. Ionizing radiation increases the activity of exosomal secretory pathway in MCF-7 human breast cancer cells: a possible way to communicate resistance against radiotherapy. Int J Mol Sci. (2019) 20:3649. doi: 10.3390/ijms20153649
59. Eleftheriou D, Moraitis E, Hong Y, Turmaine M, Venturini C, Ganesan V, et al. Microparticle-mediated VZV propagation and endothelial activation: Mechanism of VZV vasculopathy. Neurology. (2020) 94:e474–80. doi: 10.1212/WNL.0000000000008885
60. Tian C, Gao L, Zimmerman MC, Zucker IH. Myocardial infarction-induced microRNA-enriched exosomes contribute to cardiac Nrf2 dysregulation in chronic heart failure. Am J Physiol Heart Circ Physiol. (2018) 314:H928–39. doi: 10.1152/ajpheart.00602.2017
61. Xu B, Zhang Y, Du XF, Li J, Zi HX, Bu JW, et al. Neurons secrete miR-132-containing exosomes to regulate brain vascular integrity. Cell Res. (2017) 27:882–97. doi: 10.1038/cr.2017.62
62. Yu Z, Shi M, Stewart T, Fernagut PO, Huang Y, Tian C, et al. Reduced oligodendrocyte exosome secretion in multiple system atrophy involves SNARE dysfunction. Brain. (2020) 143:1780–97. doi: 10.1093/brain/awaa110
63. Li S, Wu Y, Ding F, Yang J, Li J, Gao X, et al. Engineering macrophage-derived exosomes for targeted chemotherapy of triple-negative breast cancer. Nanoscale. (2020) 12:10854–62. doi: 10.1039/D0NR00523A
64. Elsharkasy OM, Nordin JZ, Hagey DW, de Jong OG, Schiffelers RM, Andaloussi SE, et al. Extracellular vesicles as drug delivery systems: why and how? Adv Drug Deliv Rev. (2020). doi: 10.1016/j.addr.2020.04.004. [Epub ahead of print].
65. Messina JP, Humphreys I, Flaxman A, Brown A, Cooke GS, Pybus OG, et al. Global distribution and prevalence of hepatitis C virus genotypes. Hepatology. (2015) 2:77–87. doi: 10.1002/hep.27259
66. Yao Z, Qiao Y, Li X, Chen J, Ding J, Bai L, et al. Exosomes exploit the virus entry machinery and pathway to transmit alpha interferon-induced antiviral activity. J Virol. (2018) 92:e01578-18. doi: 10.1128/JVI.01578-18
67. Cai C, Koch B, Morikawa K, Suda G, Sakamoto N, Rueschenbaum S, et al. Macrophage-derived extracellular vesicles induce long-lasting immunity against hepatitis c virus which is blunted by polyunsaturated fatty acids. Front Immunol. (2018) 9:723. doi: 10.3389/fimmu.2018.00723
68. Szabo G, Bala S. Alcoholic liver disease and the gut-liver axis. World J Gastroenterol. (2010) 16:1321–9. doi: 10.3748/wjg.v16.i11.1321
70. O'Shea RS, Dasarathy S, McCullough AJ, Practice Guideline Committee of the American Association for the Study of Liver D, Practice Parameters Committee of the American College of G. Alcoholic liver disease. Hepatology. (2010) 1:307–28. doi: 10.1002/hep.23258
71. European Association for the Study of the Liver. Electronic address EEE, European Association for the Study of the L. EASL clinical practice guidelines: management of alcohol-related liver disease. J Hepatol. (2018) 57:154–81. doi: 10.1016/j.jhep.2018.03.018
72. Miranda AM, Lasiecka ZM, Xu Y, Neufeld J, Shahriar S, Simoes S, et al. Neuronal lysosomal dysfunction releases exosomes harboring APP C-terminal fragments and unique lipid signatures. Nat Commun. (2018) 9:291. doi: 10.1038/s41467-017-02533-w
73. Saha B, Momen-Heravi F, Furi I, Kodys K, Catalano D, Gangopadhyay A, et al. Extracellular vesicles from mice with alcoholic liver disease carry a distinct protein cargo and induce macrophage activation through heat shock protein 90. Hepatology. (2018) 67:1986–2000. doi: 10.1002/hep.29732
74. Momen-Heravi F, Saha B, Kodys K, Catalano D, Satishchandran A, Szabo G. Increased number of circulating exosomes and their microRNA cargos are potential novel biomarkers in alcoholic hepatitis. J Transl Med. (2015) 13:261. doi: 10.1186/s12967-015-0623-9
75. Cai Y, Xu MJ, Koritzinsky EH, Zhou Z, Wang W, Cao H, et al. Mitochondrial DNA-enriched microparticles promote acute-on-chronic alcoholic neutrophilia and hepatotoxicity. JCI Insight. (2017) 2:e92634. doi: 10.1172/jci.insight.92634
76. European Association for the Study of the L, European Association for the Study of D, European Association for the Study of O. EASL-EASD-EASO Clinical Practice Guidelines for the management of non-alcoholic fatty liver disease. J Hepatol. (2016) 60:1388–402. doi: 10.1007/s00125-016-3902-y
77. Barrera F, George J. The role of diet and nutritional intervention for the management of patients with NAFLD. Clin Liver Dis. (2014) 18:91–112. doi: 10.1016/j.cld.2013.09.009
78. Chiu S, Sievenpiper JL, de Souza RJ, Cozma AI, Mirrahimi A, Carleton AJ, et al. Effect of fructose on markers of non-alcoholic fatty liver disease (NAFLD): a systematic review and meta-analysis of controlled feeding trials. Eur J Clin Nutr. (2014) 68:416–23. doi: 10.1038/ejcn.2014.8
79. Gerber L, Otgonsuren M, Mishra A, Escheik C, Birerdinc A, Stepanova M, et al. Non-alcoholic fatty liver disease (NAFLD) is associated with low level of physical activity: a population-based study. Aliment Pharmacol Ther. (2012) 36:772–81. doi: 10.1111/apt.12038
80. Hirsova P, Gores GJ. Death receptor-mediated cell death and proinflammatory signaling in nonalcoholic steatohepatitis. Cell Mol Gastroenterol Hepatol. (2015) 1:17–27. doi: 10.1016/j.jcmgh.2014.11.005
81. Rong B, Feng R, Liu C, Wu Q, Sun C. Reduced delivery of epididymal adipocyte-derived exosomal resistin is essential for melatonin ameliorating hepatic steatosis in mice. J Pineal Res. (2019) 66:e12561. doi: 10.1111/jpi.12561
82. Antonyak MA, Lukey MJ, Cerione RA. Lipid-filled vesicles modulate macrophages. Science. (2019) 363:931–2. doi: 10.1126/science.aaw6765
83. Flaherty SE III, Grijalva A, Xu X, Ables E, Nomani A, Ferrante AW Jr. A lipase-independent pathway of lipid release and immune modulation by adipocytes. Science. (2019) 363:989–93. doi: 10.1126/science.aaw2586
84. Kornek M, Lynch M, Mehta SH, Lai M, Exley M, Afdhal NH, et al. Circulating microparticles as disease-specific biomarkers of severity of inflammation in patients with hepatitis C or nonalcoholic steatohepatitis. Gastroenterology. (2012) 143:448–58. doi: 10.1053/j.gastro.2012.04.031
85. Kim SH, Kim G, Han DH, Lee M, Kim I, Kim B, et al. Ezetimibe ameliorates steatohepatitis via AMP activated protein kinase-TFEB-mediated activation of autophagy and NLRP3 inflammasome inhibition. Autophagy. (2017) 13:1767–81. doi: 10.1080/15548627.2017.1356977
86. Patel P, Okoronkwo N, Pyrsopoulos NT. Future approaches and therapeutic modalities for acute liver failure. Clin Liver Dis. (2018) 22:419–27. doi: 10.1016/j.cld.2018.01.011
87. Volarevic V, Nurkovic J, Arsenijevic N, Stojkovic M. Concise review: therapeutic potential of mesenchymal stem cells for the treatment of acute liver failure and cirrhosis. Stem Cells. (2014) 32:2818–23. doi: 10.1002/stem.1818
88. Yan Y, Jiang W, Tan Y, Zou S, Zhang H, Mao F, et al. hucMSC exosome-derived GPX1 is required for the recovery of hepatic oxidant injury. Mol Ther. (2017) 25:465–79. doi: 10.1016/j.ymthe.2016.11.019
89. Lou G, Chen Z, Zheng M, Liu Y. Mesenchymal stem cell-derived exosomes as a new therapeutic strategy for liver diseases. Exp Mol Med. (2017) 6:e346. doi: 10.1038/emm.2017.63
90. Global Burden of Disease Liver Cancer C, Akinyemiju T, Abera S, Ahmed M, Alam N, Alemayohu MA, et al. The burden of primary liver cancer and underlying etiologies from 1990 to 2015 at the global, regional, and national level: results from the global burden of disease study 2015. JAMA Oncol. (2017) 12:1683–91. doi: 10.1001/jamaoncol.2017.3055
91. Bray F, Ferlay J, Soerjomataram I, Siegel RL, Torre LA, Jemal A. Global cancer statistics 2018: GLOBOCAN estimates of incidence and mortality worldwide for 36 cancers in 185 countries. CA Cancer J Clin. (2018) 68:394–424. doi: 10.3322/caac.21492
92. European Association for the Study of the Liver. Electronic address EEE, European Association for the Study of the L. EASL clinical practice guidelines: management of hepatocellular carcinoma. J Hepatol. (2018) 69:182–236. doi: 10.1016/j.jhep.2018.03.019
93. Lok AS, Sterling RK, Everhart JE, Wright EC, Hoefs JC, Di Bisceglie AM, et al. Des-gamma-carboxy prothrombin and alpha-fetoprotein as biomarkers for the early detection of hepatocellular carcinoma. Gastroenterology. (2010) 138:493–502. doi: 10.1053/j.gastro.2009.10.031
94. Marrero JA, Feng Z, Wang Y, Nguyen MH, Befeler AS, Roberts LR, et al. Alpha-fetoprotein, des-gamma carboxyprothrombin, and lectin-bound alpha-fetoprotein in early hepatocellular carcinoma. Gastroenterology. (2009) 137:110–8. doi: 10.1053/j.gastro.2009.04.005
95. Lee WC, Lee CF, Cheng CH, Wu TJ, Chou HS, Wu TH, et al. Outcomes of liver resection for hepatocellular carcinoma in liver transplantation era. Eur J Surg Oncol. (2015) 14:1144–52. doi: 10.1016/j.ejso.2015.05.024
96. Hui L, Chen Y. Tumor microenvironment: sanctuary of the devil. Cancer Lett. (2015) 368:7–13. doi: 10.1016/j.canlet.2015.07.039
97. Zhou Y, Ren H, Dai B, Li J, Shang L, Huang J, et al. Hepatocellular carcinoma-derived exosomal miRNA-21 contributes to tumor progression by converting hepatocyte stellate cells to cancer-associated fibroblasts. J Exp Clin Cancer Res. (2018) 37:324. doi: 10.1186/s13046-018-0965-2
98. Sohn W, Kim J, Kang SH, Yang SR, Cho JY, Cho HC, et al. Serum exosomal microRNAs as novel biomarkers for hepatocellular carcinoma. Exp Mol Med. (2015) 47:e184. doi: 10.1038/emm.2015.68
99. Wu X, Wu S, Tong L, Luan T, Lin L, Lu S, et al. miR-122 affects the viability and apoptosis of hepatocellular carcinoma cells. Scand J Gastroenterol. (2009) 44:1332–9. doi: 10.3109/00365520903215305
100. Costa-Silva B, Aiello NM, Ocean AJ, Singh S, Zhang H, Thakur BK, et al. Pancreatic cancer exosomes initiate pre-metastatic niche formation in the liver. Nat Cell Biol. (2015) 17:816–26. doi: 10.1038/ncb3169
Keywords: macrophages, exosomes, hepatitis virus, alcoholic liver disease, non-alcoholic fatty liver disease, acute liver failure, hepatocellular carcinoma
Citation: Shen M, Shen Y, Fan X, Men R, Ye T and Yang L (2020) Roles of Macrophages and Exosomes in Liver Diseases. Front. Med. 7:583691. doi: 10.3389/fmed.2020.583691
Received: 15 July 2020; Accepted: 13 August 2020;
Published: 24 September 2020.
Edited by:
Jianpeng Sheng, Nanyang Technological University, SingaporeReviewed by:
Jafar Rezaie, Urmia University of Medical Sciences, IranReza Rahbarghazi, Tabriz University of Medical Sciences, Iran
Copyright © 2020 Shen, Shen, Fan, Men, Ye and Yang. This is an open-access article distributed under the terms of the Creative Commons Attribution License (CC BY). The use, distribution or reproduction in other forums is permitted, provided the original author(s) and the copyright owner(s) are credited and that the original publication in this journal is cited, in accordance with accepted academic practice. No use, distribution or reproduction is permitted which does not comply with these terms.
*Correspondence: Tinghong Ye, yeth1309@scu.edu.cn; Li Yang, yangli_hx@scu.edu.cn
†These authors have contributed equally to this work