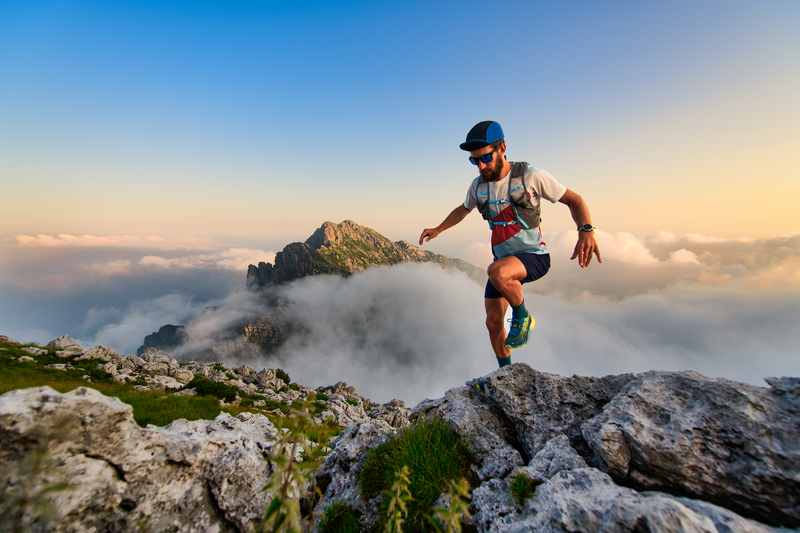
94% of researchers rate our articles as excellent or good
Learn more about the work of our research integrity team to safeguard the quality of each article we publish.
Find out more
MINI REVIEW article
Front. Med. , 30 January 2019
Sec. Translational Medicine
Volume 6 - 2019 | https://doi.org/10.3389/fmed.2019.00010
This article is part of the Research Topic Recent Advances in Endothelial Progenitor Cells Toward Their use in Clinical Translation View all 10 articles
Endoglin (ENG) is a transmembrane glycoprotein expressed on endothelial cells that functions as a co-receptor for several ligands of the transforming growth factor beta (TGF-β) family. ENG is also a recognized marker of angiogenesis and mutations in the endoglin gene are responsible for Hereditary Hemorrhagic Telangiectasia (HHT) type 1, a vascular disease characterized by defective angiogenesis, arteriovenous malformations, telangiectasia, and epistaxis. In addition to its involvement in the TGF-β family signaling pathways, several lines of evidence suggest that the extracellular domain of ENG has a role in integrin-mediated cell adhesion via its RGD motif. Indeed, we have described a role for endothelial ENG in leukocyte trafficking and extravasation via its binding to leukocyte integrins. We have also found that ENG is involved in vasculogenic properties of endothelial progenitor cells known as endothelial colony forming cells (ECFCs). Moreover, the binding of endothelial ENG to platelet integrins regulate the resistance to shear during platelet-endothelium interactions under inflammatory conditions. Because of the need for more effective treatments in HHT and the involvement of ENG in angiogenesis, current studies are aimed at identifying novel biological functions of ENG which could serve as a therapeutic target. This review focuses on the interaction between ENG and integrins with the aim to better understand the role of this protein in blood vessel formation driven by progenitor and mature endothelial cells.
Endoglin (ENG; also known as CD105) is a type I transmembrane glycoprotein predominantly expressed in endothelial cells (ECs) (1, 2) and endothelial colony-forming cells (ECFCs) (3). It is known as an essential co-receptor for the transforming growth factor β (TGF-β) family, playing an important role in angiogenesis. At the cell surface, ENG associates with TGF-β type I receptors, including the ECs-specific ALK1 (activin receptor-like kinase 1) and the ubiquitous ALK5, as well as to a TGF-β type II receptor. In this receptor complex, ENG and ALK1 are able to bind to bone morphogenetic protein 9 (BMP9; also known as growth differentiation factor 2 [GDF2]), a member of the TGF-β family, with much higher affinity than to TGF-β1 and mediate its proliferation signal (4–7).
Upon ligand binding, the TGF-β signaling receptor complex phosphorylates members of the Smad family of transcription factors which, in turn, undergo nuclear translocation to regulate gene expression (Figure 1A).
Figure 1. Hypothetical model of Endoglin and its role as TGF-β co-receptor and adhesion molecule in different physiological contexts. (A) Canonical pathway that implicates Eng as a co-receptor of TGF-β in ECs. Bone morphogenetic protein 9 (BMP9), and other members of the TGF-β family, bind to an EC receptor complex composed by the type I (R-I) receptor named ALK1 and the type II (R-II) receptor, both exhibiting serine/threonine kinase activity, as well as the auxiliary receptor endoglin. Upon ligand binding, the R-II transphosphorylates ALK1, which subsequently propagates the signal by phosphorylating the receptor-regulated Smad (R-Smad) family of proteins Smad1/5/8. Phosphorylated R-Smads form heteromeric complexes with Smad4, translocating into the nucleus to regulate the transcriptional activity of different target genes. BMP9, Endoglin, ALK1 and Smad4 proteins are encoded by GDF2, ENG, ACVRL1 and MADH4 genes, whose pathogenic mutations give rise to HHT5, HHT1, HHT2, and JPHT, respectively (30). (B) Endothelial endoglin as an adhesion molecule involving its RGD sequence when binding to cell surface integrins from leukocytes, VMCs or platelets. (C) Leukocyte transmigration through the vessel endothelium. Under inflammatory conditions, different soluble factors are released leading to leukocyte adhesion and transmigration through ECs. This process is mediated, at least, by the interaction between leukocyte integrin α5β1 and endothelial endoglin involving the specific recognition of the RGD motif in Eng (22). (D) Adhesion between endothelial cells and vascular mural cells (VMCs). This intercellular association involves, at least, the interaction between integrin α5β1 in VMCs and endothelial endoglin via the specific recognition of the RGD motif in Eng (23). (E) Platelet-dependent hemostasis. Endothelial endoglin via its RGD binds to platelet integrins αIIbβ3 and α5β1, contributing to stabilize platelets adhesion to endothelium (24).
In addition to the membrane bound form of endoglin, a circulating form of the ENG ectodomain, was found to be released upon a C-terminal cleavage of ENG by matrix metalloproteinase 14 (MMP-14) (8, 9). Increased levels of circulating endoglin have been detected at early stages of preeclampsia (5, 10) in hypertensive or diabetic patients (11) and in certain cancer patients (12), suggesting its role as a predictive biomarker in these pathologies. Interestingly, a detailed characterization of plasma from women with preeclampsia has revealed that circulating endoglin can be complexed within exosomes, rather than as individual soluble endoglin (13).
A pioneer article on human ENG described that this glycoprotein is present on ECs as a dimer (two subunits of ~90-kDa), each displaying the tripeptide arginine-glycine-aspartic acid (RGD) in the extracellular region of the protein (1). The RGD motif and homologous sequences in mouse and pig are recognition motifs for cell surface integrins present in extracellular matrix proteins such as fibronectin, tenascin, thrombospondin, vitronectin, von Willebrand factor as well as fibrinogen and prothrombin (14–16). Integrin-mediated adhesion is involved in hemostasis, thrombosis, and inflammation, processes in which the endothelium plays a critical role. Therefore, the presence of the RGD motif within a hydrophilic environment (1) and its accessibility in the 3D structure of ENG (7), clearly suggest the involvement of ENG in integrin binding, as postulated in early studies (17, 18). In fact, endothelial endoglin binds to leukocyte integrins, allowing leukocyte extravasation (19) suggesting a possible novel function for ENG independent of TGF-β signaling (Figures 1B,C). Several lines of evidence support the role of ENG in leukocyte trafficking: (i) ENG expression is markedly up regulated in endothelial cells of inflamed tissues with an associated inflammatory cell infiltrate (19); (ii) ENG is up-regulated in the post-ischemic kidney and ENG-haploinsufficient mice are protected from renal ischemia-reperfusion injury, due to a reduction of cellular inflammatory responses (20); (iii) Arterial, venous, and capillary endothelia in lymphoid organs are highly reactive with anti-ENG antibodies and a marked staining pattern is observed in high endothelial venules (21); and (iv) Although ENG is present throughout the vascular endothelium, its expression, as compared to veins or arteries, is stronger in capillaries, where most leukocyte infiltration to organs occurs.
In addition to leukocytes, other cell types present in the circulatory system have also revealed an integrin-mediated adhesion activity toward endothelial endoglin (22–24) (Figures 1D,E). Thus, endoglin plays a role in integrin-mediated adhesion of vascular mural cells to endothelium (23). Also, a new role for endoglin in αIIbβ3 integrin-mediated adhesion of platelets to the endothelium, conferring resistance of adherent platelets to detachment has been described (24) (Figure 1E).
Hereditary Hemorrhagic Telangiectasia (HHT) is an autosomal dominant disease with a prevalence of one case per 5,000–8,000 individuals (25, 26). HHT patients develop muco-cutaneous lesions known as telangiectasia in the nose, mouth, and gastrointestinal tract, as well as larger AVMs in major organs such as the lung, liver, and brain (25). The telangiectasia are comprised of fragile vessels that are susceptible to rupture and hemorrhage, which means that HHT patients can suffer recurrent anemia following frequent and severe bleeding episodes. Mutations in the endoglin gene (ENG) are responsible for HHT type 1 (HHT1) (27), while mutations in activing receptor-like kinase (ACVRL1 alias ALK1) are responsible for HHT2 (2, 27). Taken together, HHT1 and HHT2 account for more than 80% of all HHT cases, whereas a small number of patients show mutations in SMAD4 (MADH4) or in BMP9 gene (GDF2), which are responsible for less common HHT variants such as a combined syndrome with juvenile polyposis (JP-HHT) and HHT5, respectively (28, 29). Remarkably, all of these genes code for proteins involved in the TGF-β family signaling pathways (30). Nowadays, the most widely accepted hypotheses for AVMs formation in HHT1 and HHT2 involves a “first hit” (gene haploinsufficiency) based on the loss of one functional allele Eng+/− (HHT1) or ALK1+/− (HHT2), which causes a 50% reduction in protein expression, followed by a “second hit” based on an angiogenic trigger such as inflammation, hypoxia or vascular injury (31). Together, these events may induce a deficient endothelial function of ENG that could result in HHT vascular lesions. The relevant role of endoglin in the pathophysiology of the vascular system is illustrated by the phenotype of different HHT1 animal models (32). While endoglin heterozygous mice are viable and reproduce the vascular phenotype of HHT patients, total loss of ENG expression leads in mice to cardiovascular defects and embryonic death by mid-gestation (33–35). A conditional knock out mouse model for HHT1 has revealed that the arteriovenous malformations (AVMs), induced upon ENG loss, appear to be the result of delayed vascular remodeling and inappropriate ECs proliferation responses (36). In ENG null mice, vasculogenesis does not seem affected, suggesting that ENG is not required for initial differentiation of ECs or formation of a primitive vascular plexus. The yolk sac of Eng−/− embryos has enlarged fragile vessels, while the embryo proper shows cardiac cushion defects and delayed maturation of major vessels (34). The reason why the total loss of ENG expression leads to cardiovascular defects and embryonic death is not completely understood. Some authors have postulated that the primary defect is in the heart (37), while others suggest that the loss of smooth muscle cells (SMCs) coverage of the endothelium could play a decisive role in the lethality of Eng−/− animals (34).
Previous studies reported that endothelial ENG is associated with several proteins critically involved in endothelial cell adhesion, proliferation, migration, angiogenesis, and vascular permeability, including integrins (38), zyxin (39), zyxin-related protein 1 (ZRP-1) (40), VEGF receptor type 2 (VEGFR-2) (41), beta-arrestin 2 (42), and vascular endothelial-cadherin (VE-cadherin) (43). It has been shown that VE-cadherin interacts with several components of the TGF-β receptor complex, including ENG, ALK1, and the TGF-β type II receptor TGFBR2 in adherents junctions. Of note, ENG, TGFBR2, and ALK1 also interact with p21-activated kinase (PAK-1), GTP-binding proteins, and Ras-related C3 botulinum toxin substrate 2 (Rac-2), involved in endothelial barrier maintenance, cytoskeletal remodeling and cell migration (44). Using in vitro ECs lines derived from ENG homozygous null, heterozygous, and control mouse embryos, Jerkic and Letarte have reported that ENG-deficient mouse embryonic ECs are hyper-permeable and unresponsive to stimulation by VEGF and TGF-β1, factors that regulate vascular permeability (45). Interestingly, VE-cadherin is barely detected in ENG-deficient ECs, a finding that could explain the EC junction destabilization and impairment of TGF-β signaling. Furthermore, permeability alterations in Eng+/− mice are in line with findings in patients with Hereditary Hemorrhagic Telangiectasia type 1 (HHT1). Thus, ECs from HHT1 patients show a disorganized actin cytoskeleton prone to cell breaking with changes in shear stress and blood pressure (46). This reorganization of actin filaments in HHT1 might lead to vessel hemorrhages and eventual disappearance of the capillary network, as reported in this disorder. In line with the pathogenic hypothesis of an endothelial dysfunction in HHT1, the involvement of ENG in integrin-mediated trans-endothelial leukocyte trafficking was proposed (31). Accordingly, following inflammation triggering, the extracellular region of ENG binds to leukocyte integrin α5β1 and promotes leukocyte transmigration (31). In contrast, a different experimental approach showed an increased gut inflammation and myeloid infiltration in colitis Eng+/− vs. control mice (47). Overall, this novel role of ENG may contribute to a better understanding of the inflammation process and the high incidence of infections in HHT patients (48). Because pericytes embrace the endothelium to regulate the blood retinal barrier, the role of endothelial endoglin in this context has also been analyzed. Thus, vascular permeability studies carried out in vivo showed that retinas of Eng+/− heterozygous mice displayed higher retinal permeability than that of Eng+/+ mice, suggesting a destabilization of the endothelial barrier function due to ENG haploinsufficiency (23). The importance of the interaction between ECs and vascular mural cells is also underlined by studies showing that thalidomide stabilizes the vasculature in a mouse model of HHT1 (49). Indeed, pericyte recruitment and stabilization appears as a therapeutic strategy to reduce the severity of epistaxis in HHT (50). Thus, ENG, directly, or as a component of the TGF-β receptor complex may regulate ECs integrity, whereas its absence may result in vascular hyper-permeability, thus contributing to the fetal lethality associated with the homozygous null genotype (39, 40).
Although this review focuses on the role of endoglin in ECs, it is worth mentioning that endoglin is also expressed at lower levels in other cell types, some of which present in the vasculature as vascular SMCs, activated monocytes or mesenchymal cells (18, 51–53). However, a review on the endoglin role in these cell types deserves an independent study.
The formation of blood vessels from mesoderm is driven by the recruitment of undifferentiated cells which can differentiate into endothelial lineage. In the adult, populations of bone marrow-derived endothelial progenitor cells (EPCs) are mobilized into the circulation by stimuli such as estrogen and VEGF (54). Interestingly, human EPCs could derive from very small embryonic like stem cells (55) that can also give rise to hematopoietic cells (56). Two populations of EPCs have been differentially characterized from circulating endothelial progenitors: (i) early EPCs that exhibit a hematopoietic profile with a genomic fingerprint similar to monocytes; and (ii) ECFCs, also known as blood outgrowth endothelial cells (BOECs), which are considered late EPCs with an endothelial-like genomic profile (57). ECFCs display an intrinsic tube forming capacity in vitro and in vivo and they are involved in blood vessel formation and vascular repair (58, 59). For example, upon vascular injury, tissue perfusion of blood flow involves not only angiogenic sprouting of ECs from nearby intact vessels, but also the recruitment circulating ECFCs allowing the formation of new blood vessels (60). Acting at the interface between blood and tissues, ECFCs have a remarkable ability for migration and proliferation, being key cells in angiogenesis and vascular remodeling. It is therefore not surprising that perturbations in these critical ECFCs functions contribute to several vascular pathologies. Accordingly, ECFCs have opened a new area for treatment of cardiovascular diseases using cell therapy, advancing in the knowledge of vessel reconstruction ability in postnatal life. In this line, much effort has been recently devoted to identify novel molecular targets within ECFCs able to enhance their angiogenic potential. ECFCs are positive for endoglin (CD105), VE-cadherin (CD144), CD31, VEGFR2 (KDR), EGFL7, and CD146, and negative for CD45 and CD14 (58, 59). Among these, ENG is emerging as an interesting therapeutic target based on its involvement in angiogenesis and vascular remodeling (46, 61).
Many strategies of therapeutic revascularization, based on the administration of growth factors or stem/progenitor cells from diverse sources including EPCs, have been proposed and are currently being tested in patients with peripheral arterial disease or cardiac diseases (62). Some pretreatment of ECFCs with erythropoietin (63), fucoidan (64), soluble CD146 (65), tumor necrosis factor-α (TNF-α) (66), or platelet lysates (67, 68) have also been proposed to improve the therapeutic efficacy of in vivo administered ECFCs. Interestingly, integrins represent a major molecular determinant of EPCs function. More specifically, integrin α4β1 is a key regulator of EPCs retention and/or mobilization from the bone marrow, while integrins α5β1, α6β1, αvβ3, and αvβ5 are involved in EPCs homing, invasion, differentiation and paracrine factor production (69). The involvement of ECFCs in angiogenesis has been shown to be essential for ischemic disease recovery (70, 71). Furthermore, the differentiation of mesenchymal stem cells (MSCs) into mural cells appears to be stimulated by their co-culture with ECFCs, whereas overexpression of the Notch ligand Jagged1 in ECs further enhanced the differentiation of MSC into pericytes (72). This cooperation prompted some authors to postulate an improvement of ECFCs efficiency by co-injecting ECFCs and SMC progenitors (73). Therefore, a combined treatment of ECFCs with mesenchymal stem/progenitor cells (MSCs/MPCs) appears to operate synergistically, enhancing neovascularization compared to either individual cell population (74, 75). Recently, we proposed a role for ENG in the ECFCs-MSCs interplay involved in the revascularization process (61). Indeed, ENG silencing in ECFCs markedly inhibited ECFCs adhesion to MSCs in vitro, without affecting MSCs differentiation into perivascular cells or other mesenchymal lineages. Mesenchymal stem cells (MSCs) increase muscle recovery of ECFC in HLI model and we found that ENG-silenced ECFCs co-injected with MSCs in mice abolish beneficial effects of MSCs leading to decreased vessel density and foot perfusion upon ischemia. Our results suggest ENG involvement in the crosstalk between ECFCs and MSCs, leading to vessel formation and stabilization (51) (Figures 2A,B).
Figure 2. Endoglin in ECFCs. (A) Role of endoglin matrigel vascularization in vivo. Matrigel plugs were mixed with either ECFCs treated with control siRNA plus mesenchymal stem cells (MSCs) (left) or with ECFCs treated with endoglin specific siRNA plus MSCs (right). The Matrigel mixture was injected into nude mice and the number of vessels was analyzed after 1 week. Control plugs display a marked vascularization with functional vessels (presence of erythrocytes). Plugs with endoglin-silenced ECFCs display less vascularization than controls, suggesting an important role for this protein in cell adhesion (23). (B) MSCs combined with ECFCs, accelerate muscle recovery in a mouse model of hind limb ischemia, through an endoglin-dependent mechanism. After femoral ligature and retro-orbital injection of ECFC+MSC Doppler analysis shows a revascularization induced by co-injection of ECFCs plus MSCs (left). This synergistic effect is abolished when endoglin is previously silenced in ECFCs (right) (61). (C) Mechanisms involved in the synergy between ECFCs and MSCs. (i) Mutual paracrine effect between ECFCs and MSCs involving growth factors like (76), VEGF and PDGFBB (77). (ii) ECFCs-induced differentiation of MSCs into perivascular cells via Notch-Jagged1 (72) (iii) Adhesion between ECFCs and MSCs involving endoglin (61).
As postulated in Figure 2C, three steps may be involved during the formation of stable vessels by ECFC and MSC and/or perivascular cells: (i) Mutual paracrine effects by VEGF and/or PDGF-BB (76, 77). (ii) ECFCs-driven perivascular maturation of MSC and/or SMC progenitors via the notch-dependent pathway; and (iii) Adhesion between ECFCs and perivascular cells in an ENG-dependent manner. The relevance of endothelial ENG adhesive properties in mature vessel formation in mice (61) is in agreement with a previous report on the active role of human ENG in the adhesion between mature ECs and vascular mural cells (23).
Relevance of ENG in ECFC has been demonstrated also in ECFCs isolated directly from HHT1 patients. Indeed, these ECFCs have disorganized and depolymerized actin fibers and impaired tube formation, as compared to healthy ECFCs (46). The molecular basis for the abnormal behavior of HHT1 ECFCs appears to be mediated, at least in part, by the regulatory role of endoglin and we can speculate via its interaction with zyxin family members involved in the actin cytoskeletal organization as it was proposed for endothelial cells (39, 40). Thus, the ENG cytoplasmic domain binds to zyxin and ZRP1, which concentrate at focal adhesions within actin polymerization points. Consequently, the decrease of ENG levels in HHT1 ECFCs could be related to the cytoskeleton alteration.
Blood vessel formation and remodeling are essential processes in the maintenance of tissue homeostasis and function, and therefore, their alteration causes a variety of pathologic conditions. ENG involvement in the function of mature and progenitor endothelial cells is a hot topic not completely understood and developed yet. Recent findings underline the importance of ENG not only as a co-receptor for several ligands of the TGF-β family, but also as a molecule involved in cell adhesion, which can regulate ECFCs behavior in the context of angiogenic processes. It is recognized that in mature ECs, ENG plays a key role in angiogenesis and blood vessel homeostasis, becoming a potential therapeutic target for pro- and anti-angiogenic approaches in the treatment of diseases such as HHT, cancer, preeclampsia, diabetes complications or post-ischemic disease. While ENG expression is relatively low in quiescent ECs, it is upregulated in the active endothelium involved in angiogenesis and vessel remodeling and permeability. In these processes, endothelial ENG regulates ECs proliferation, migration, and actin cytoskeletal organization, leukocyte extravasation, and interaction with mural cells. In addition, a role for ENG in platelet-endothelium interaction, including the resistance of adherent platelets to shear under inflammatory conditions, was also recently proposed, opening new perspectives on ENG functions.
This review highlights the emerging roles of endothelial ENG as a cell adhesion molecule in mature and progenitor endothelial cells interacting with vascular mural cells, leukocytes, and platelets, independently or in parallel to its TGF-β co-receptor role.
While evidence-based therapeutics are coming into play in the traditionally empirical base for endoglin related protein derivatives, future studies of ENG in endothelial progenitor cells may pave the way for a better understanding of its function in the vascular system and for the development and understanding of the use of these immature cells as a cell therapy product.
ER wrote the manuscript. CB provided helpful suggestions and contributed to writing the manuscript. DS wrote the manuscript and provided funding support.
The authors declare that the research was conducted in the absence of any commercial or financial relationships that could be construed as a potential conflict of interest.
1. Gougos A, Letarte M. Primary structure of endoglin, an RGD-containing glycoprotein of human endothelial cells. J Biol Chem. (1990) 265:8361–4.
2. López-Novoa JM, Bernabeu C. The physiological role of endoglin in the cardiovascular system. Am J Physiol Heart Circ Physiol. (2010) 299:H959–74. doi: 10.1152/ajpheart.01251.2009
3. Campioni D, Zauli G, Gambetti S, Campo G, Cuneo A, Ferrari R, et al. In vitro characterization of circulating endothelial progenitor cells isolated from patients with acute coronary syndrome. PLoS ONE (2013) 8:e56377. doi: 10.1371/journal.pone.0056377
4. Alt A, Miguel-Romero L, Donderis J, Aristorena M, Blanco FJ, Round A, et al. Structural and functional insights into endoglin ligand recognition and binding. PLoS ONE (2012) 7:e29948. doi: 10.1371/journal.pone.0029948
5. Gregory AL, Xu G, Sotov V, Letarte M. Review: the enigmatic role of endoglin in the placenta. Placenta (2014) 35:S93–9. doi: 10.1016/j.placenta.2013.10.020
6. Li W, Salmon RM, Jiang H, Morrell NW. Regulation of the ALK1 ligands, BMP9 and BMP10. Biochem Soc Trans. (2016) 44:1135–41. doi: 10.1042/BST20160083
7. Saito T, Bokhove M, Croci R, Zamora-Caballero S, Han L, Letarte M, et al. Structural basis of the human endoglin-BMP9 interaction: insights into BMP signaling and HHT1. Cell Rep. (2017) 19:1917–28. doi: 10.1016/j.celrep.2017.05.011
8. Hawinkels LJAC, Kuiper P, Wiercinska E, Verspaget HW, Liu Z, Pardali E, et al. Matrix metalloproteinase-14 (MT1-MMP)-mediated endoglin shedding inhibits tumor angiogenesis. Cancer Res. (2010) 70:4141–50. doi: 10.1158/0008-5472.CAN-09-4466
9. Valbuena-Diez AC, Blanco FJ, Oujo B, Langa C, Gonzalez-Nuñez M, Llano E, et al. Oxysterol-induced soluble endoglin release and its involvement in hypertension clinical perspective. Circulation (2012) 126:2612–24. doi: 10.1161/CIRCULATIONAHA.112.101261
10. Venkatesha S, Toporsian M, Lam C, Hanai J, Mammoto T, Kim YM, et al. Soluble endoglin contributes to the pathogenesis of preeclampsia. Nat Med. (2006) 12:642–9. doi: 10.1038/nm1429
11. Blázquez-Medela AM, García-Ortiz L, Gómez-Marcos MA, Recio-Rodríguez JI, Sánchez-Rodríguez A, López-Novoa JM, et al. Increased plasma soluble endoglin levels as an indicator of cardiovascular alterations in hypertensive and diabetic patients. BMC Med. (2010) 8:86. doi: 10.1186/1741-7015-8-86
12. Bernabeu C, Lopez-Novoa JM, Quintanilla M. The emerging role of TGF-β superfamily coreceptors in cancer. Biochim Biophys Acta BBA (2009) 1792:954–73. doi: 10.1016/j.bbadis.2009.07.003
13. Ermini L, Ausman J, Melland-Smith M, Yeganeh B, Rolfo A, Litvack ML, et al. A single sphingomyelin species promotes exosomal release of endoglin into the maternal circulation in preeclampsia. Sci Rep. (2017) 7:12172. doi: 10.1038/s41598-017-12491-4
14. Ruoslahti E. RGD and other recognition sequences for integrins. Annu Rev Cell Dev Biol. (1996) 12:697–715. doi: 10.1146/annurev.cellbio.12.1.697
15. Luo B-H, Carman CV, Springer TA. Structural basis of integrin regulation and signaling. Annu Rev Immunol. (2007) 25:619–47. doi: 10.1146/annurev.immunol.25.022106.141618
16. Eto K, Puzon-McLaughlin W, Sheppard D, Sehara-Fujisawa A, Zhang X-P, Takada Y. RGD-independent binding of integrin α9β1 to the ADAM-12 and−15 disintegrin domains mediates cell-cell interaction. J Biol Chem. (2000) 275:34922–30. doi: 10.1074/jbc.M001953200
17. Gougos A, St Jacques S, Greaves A, O'Connell PJ, d'Apice AJ, Bühring HJ, et al. Identification of distinct epitopes of endoglin, an RGD-containing glycoprotein of endothelial cells, leukemic cells, and syncytiotrophoblasts. Int Immunol. (1992) 4:83–92.
18. Lastres P, Bellon T, Cabañas C, Sanchez-Madrid F, Acevedo A, Gougos A, et al. Regulated expression on human macrophages of endoglin, an Arg-Gly-Asp-containing surface antigen. Eur J Immunol. (1992) 22:393–7.
19. Torsney E, Charlton R, Parums D, Collis M, Arthur HM. Inducible expression of human endoglin during inflammation and wound healing in vivo. Inflamm Res Off J Eur Histamine Res Soc Al. (2002) 51:464–70. doi: 10.1007/PL00012413
20. Docherty NG, López-Novoa JM, Arevalo M, Düwel A, Rodriguez-Peña A, Pérez-Barriocanal F, et al. Endoglin regulates renal ischaemia-reperfusion injury. Nephrol Dial Transpl. (2006) 21:2106–19. doi: 10.1093/ndt/gfl179
21. Dagdeviren A, Müftüoglu SF, Cakar AN, Ors U. Endoglin (CD 105) expression in human lymphoid organs and placenta. Ann Anat Anat Anz. (1998) 180:461–9.
22. Rossi E, Sanz-Rodriguez F, Eleno N, Düwell A, Blanco FJ, Langa C, et al. Endothelial endoglin is involved in inflammation: role in leukocyte adhesion and transmigration. Blood (2013) 121:403–15. doi: 10.1182/blood-2012-06-435347
23. Rossi E, Smadja DM, Boscolo E, Langa C, Arevalo MA, Pericacho M, et al. Endoglin regulates mural cell adhesion in the circulatory system. Cell Mol Life Sci. (2016) 73:1715–39. doi: 10.1007/s00018-015-2099-4
24. Rossi E, Pericacho M, Bachelot-Loza C, Pidard D, Gaussem P, Poirault-Chassac S, et al. Human endoglin as a potential new partner involved in platelet–endothelium interactions. Cell Mol Life Sci. (2018) 75:1269–84. doi: 10.1007/s00018-017-2694-7
25. Shovlin CL. Hereditary haemorrhagic telangiectasia: pathophysiology, diagnosis and treatment. Blood Rev. (2010) 24:203–19. doi: 10.1016/j.blre.2010.07.001
26. Faughnan ME, Palda VA, Garcia-Tsao G, Geisthoff UW, McDonald J, Proctor DD, et al. International guidelines for the diagnosis and management of hereditary haemorrhagic telangiectasia. J Med Genet. (2011) 48:73–87. doi: 10.1136/jmg.2009.069013
27. McAllister KA, Grogg KM, Johnson DW, Gallione CJ, Baldwin MA, Jackson CE, et al. Endoglin, a TGF-β binding protein of endothelial cells, is the gene for hereditary haemorrhagic telangiectasia type 1. Nat Genet. (1994) 8:345–51. doi: 10.1038/ng1294-345
28. Gallione CJ, Repetto GM, Legius E, Rustgi AK, Schelley SL, Tejpar S, et al. A combined syndrome of juvenile polyposis and hereditary haemorrhagic telangiectasia associated with mutations in MADH4 (SMAD4). Lancet (2004) 363:852–9. doi: 10.1016/S0140-6736(04)15732-2
29. Wooderchak-Donahue WL, McDonald J, O'Fallon B, Upton PD, Li W, Roman BL, et al. BMP9 mutations cause a vascular-anomaly syndrome with phenotypic overlap with hereditary hemorrhagic telangiectasia. Am J Hum Genet. (2013) 93:530–7.
30. Ruiz-Llorente L, Gallardo-Vara E, Rossi E, Smadja DM, Botella LM, Bernabeu C. Endoglin and alk1 as therapeutic targets for hereditary hemorrhagic telangiectasia. Expert Opin Ther Targets (2017) 21:933–47. doi: 10.1080/14728222.2017.1365839
31. Rossi E, Lopez-Novoa JM, Bernabeu C. Endoglin involvement in integrin-mediated cell adhesion as a putative pathogenic mechanism in hereditary hemorrhagic telangiectasia type 1 (HHT1). Front Genet. (2015) 5:457. doi: 10.3389/fgene.2014.00457
32. Tual-Chalot S, Oh SP, Arthur HM. Mouse models of hereditary hemorrhagic telangiectasia: recent advances and future challenges. Front Genet. (2015) 6:25. doi: 10.3389/fgene.2015.00025
33. Bourdeau A, Dumont DJ, Letarte M. A murine model of hereditary hemorrhagic telangiectasia. J Clin Invest. (1999) 104:1343–51. doi: 10.1172/JCI8088
34. Li DY, Sorensen LK, Brooke BS, Urness LD, Davis EC, Taylor DG, et al. Defective Angiogenesis in mice lacking endoglin. Science (1999) 284:1534–7. doi: 10.1126/science.284.5419.1534
35. Arthur HM, Ure J, Smith AJH, Renforth G, Wilson DI, Torsney E, et al. Endoglin, an Ancillary TGFβ receptor, is required for extraembryonic angiogenesis and plays a key role in heart development. Dev Biol. (2000) 217:42–53. doi: 10.1006/dbio.1999.9534
36. Mahmoud M, Allinson KR, Zhai Z, Oakenfull R, Ghandi P, Adams RH, et al. Pathogenesis of arteriovenous malformations in the absence of endoglin. Circ Res. (2010) 106:1425–33. doi: 10.1161/CIRCRESAHA.109.211037
37. Nomura-Kitabayashi A, Anderson GA, Sleep G, Mena J, Karabegovic A, Karamath S, et al. Endoglin is dispensable for angiogenesis, but required for endocardial cushion formation in the midgestation mouse embryo. Dev Biol. (2009) 335:66–77. doi: 10.1016/j.ydbio.2009.08.016
38. Tian H, Mythreye K, Golzio C, Katsanis N, Blobe GC. Endoglin mediates fibronectin/α5β1 integrin and TGF-β pathway crosstalk in endothelial cells. EMBO J. (2012) 31:3885–900. doi: 10.1038/emboj.2012.246
39. Conley BA, Koleva R, Smith JD, Kacer D, Zhang D, Bernabéu C, et al. Endoglin controls cell migration and composition of focal adhesions function of the cytosolic domain. J Biol Chem. (2004) 279:27440–9. doi: 10.1074/jbc.M312561200
40. Sanz-Rodriguez F, Guerrero-Esteo M, Botella L-M, Banville D, Vary CPH, Bernabéu C. Endoglin regulates cytoskeletal organization through binding to ZRP-1, a member of the Lim family of proteins. J Biol Chem. (2004) 279:32858–68. doi: 10.1074/jbc.M400843200
41. Tian H, Huang JJ, Golzio C, Gao X, Hector-Greene M, Katsanis N, et al. Endoglin interacts with VEGFR2 to promote angiogenesis. FASEB J Off Publ Fed Am Soc Exp Biol. (2018) 32:2934–49. doi: 10.1096/fj.201700867RR
42. Lee NY, Blobe GC. The Interaction of endoglin with β-Arrestin2 regulates transforming growth factor-β-mediated ERK activation and migration in endothelial cells. J Biol Chem. (2007) 282:21507–17. doi: 10.1074/jbc.M700176200
43. Rudini N, Felici A, Giampietro C, Lampugnani M, Corada M, Swirsding K, et al. VE-cadherin is a critical endothelial regulator of TGF-beta signalling. EMBO J. (2008) 27:993–1004. doi: 10.1038/emboj.2008.46
44. Xu G, Barrios-Rodiles M, Jerkic M, Turinsky AL, Nadon R, Vera S, et al. Novel protein interactions with endoglin and activin receptor-like kinase 1: potential role in vascular networks. Mol Cell Proteomics MCP (2014) 13:489–502. doi: 10.1074/mcp.M113.033464
45. Seghers L, de Vries MR, Pardali E, Hoefer IE, Hierck BP, ten Dijke P ten, et al. Shear induced collateral artery growth modulated by endoglin but not by ALK1. J Cell Mol Med. (2012) 16:2440–50. doi: 10.1111/j.1582-4934.2012.01561.x
46. Fernandez-L A, Sanzrodriguez F, Zarrabeitia R, Perezmolino A, Hebbel R, Nguyen J, et al. Blood outgrowth endothelial cells from Hereditary Haemorrhagic Telangiectasia patients reveal abnormalities compatible with vascular lesions. Cardiovasc Res. (2005) 68:235–48. doi: 10.1016/j.cardiores.2005.06.009
47. Peter MR, Jerkic M, Sotov V, Douda DN, Ardelean DS, Ghamami N, et al. Impaired resolution of inflammation in the endoglin heterozygous mouse model of chronic colitis. Mediators Inflamm. (2014) 2014:767185. doi: 10.1155/2014/767185
48. Dupuis-Girod S, Giraud S, Decullier E, Lesca G, Cottin V, Faure F, et al. Hemorrhagic hereditary telangiectasia (Rendu-Osler disease) and infectious diseases: an underestimated association. Clin Infect Dis Off Publ Infect Dis Soc Am. (2007) 44:841–5. doi: 10.1086/511645
49. Lebrin F, Srun S, Raymond K, Martin S, Brink S van den, Freitas C, et al. Thalidomide stimulates vessel maturation and reduces epistaxis in individuals with hereditary hemorrhagic telangiectasia. Nat Med. (2010) 16:420–8. doi: 10.1038/nm.2131
50. Thalgott J, Dos-Santos-Luis D, Lebrin F. Pericytes as targets in hereditary hemorrhagic telangiectasia. Front Genet. (2015) 6:37. doi: 10.3389/fgene.2015.00037
51. Guerrero-Esteo M, Lastres P, Letamendía A, Pérez-Alvarez MJ, Langa C, López LA, et al. Endoglin overexpression modulates cellular morphology, migration, and adhesion of mouse fibroblasts. Eur J Cell Biol. (1999) 78: 614–23.
52. O'Connor JC, Farach-Carson MC, Schneider CJ, Carson DD. Coculture with prostate cancer cells alters endoglin expression and attenuates transforming growth factor-beta signaling in reactive bone marrow stromal cells. Mol Cancer Res. (2007) 5:585–603. doi: 10.1158/1541-7786.MCR-06-0408
53. Tian H, Ketova T, Hardy D, Xu X, Gao X, Zijlstra A, et al. Endoglin mediates vascular maturation by promoting vascular smooth muscle cell migration and spreading. Arterioscler Thromb Vasc Biol. (2017) 37:1115–26. doi: 10.1161/ATVBAHA.116.308859
54. Lyden D, Hattori K, Dias S, Costa C, Blaikie P, Butros L, et al. Impaired recruitment of bone-marrow–derived endothelial and hematopoietic progenitor cells blocks tumor angiogenesis and growth. Nat Med. (2001) 7:1194–201. doi: 10.1038/nm1101-1194
55. Guerin CL, Loyer X, Vilar J, Cras A, Mirault T, Gaussem P, et al. Bone-marrow-derived very small embryonic-like stem cells in patients with critical leg ischaemia: evidence of vasculogenic potential. Thromb Haemost. (2015) 113:1084–94. doi: 10.1160/TH14-09-0748
56. Smadja DM. Bone marrow very small embryonic-like stem cells: new generation of autologous cell therapy soon ready for prime time? Stem Cell Rev. (2017) 13:198–201. doi: 10.1007/s12015-017-9718-4
57. Smadja DM, Bièche I, Silvestre J-S, Germain S, Cornet A, Laurendeau I, et al. Bone morphogenetic proteins 2 and 4 are selectively expressed by late outgrowth endothelial progenitor cells and promote neoangiogenesis. Arterioscler Thromb Vasc Biol. (2008) 28:2137–43. doi: 10.1161/ATVBAHA.108.168815
58. d'Audigier C, Susen S, Blandinieres A, Mattot V, Saubamea B, Rossi E, et al. Egfl7 represses the vasculogenic potential of human endothelial progenitor cells. Stem Cell Rev. (2018) 14:82–91. doi: 10.1007/s12015-017-9775-8
59. Medina RJ, Barber CL, Sabatier F, Dignat-George F, Melero-Martin JM, Khosrotehrani K, et al. Endothelial progenitors: a consensus statement on nomenclature. Stem Cells Transl Med. (2017) 6:1316–20. doi: 10.1002/sctm.16-0360
60. Banno K, Yoder MC. Tissue regeneration using endothelial colony-forming cells: promising cells for vascular repair. Pediatr Res. (2018) 83:283–90. doi: 10.1038/pr.2017.231
61. Rossi E, Goyard C, Cras A, Dizier B, Bacha N, Lokajczyk A, et al. Co-injection of mesenchymal stem cells with endothelial progenitor cells accelerates muscle recovery in hind limb ischemia through an endoglin-dependent mechanism. Thromb Haemost. (2017) 117:1908–18. doi: 10.1160/TH17-01-0007
62. Silvestre J-S, Smadja DM, Lévy BI. Postischemic revascularization: from cellular and molecular mechanisms to clinical applications. Physiol Rev. (2013) 93:1743–802. doi: 10.1152/physrev.00006.2013
63. Bennis Y, Sarlon-Bartoli G, Guillet B, Lucas L, Pellegrini L, Velly L, et al. Priming of late endothelial progenitor cells with erythropoietin before transplantation requires the CD131 receptor subunit and enhances their angiogenic potential. J Thromb Haemost. (2012) 10:1914–28. doi: 10.1111/j.1538-7836.2012.04835.x
64. Lee JH, Lee SH, Choi SH, Asahara T, Kwon S-M. The sulfated polysaccharide fucoidan rescues senescence of endothelial colony-forming cells for ischemic repair. Stem Cells Dayt Ohio (2015) 33:1939–51. doi: 10.1002/stem.1973
65. Stalin J, Harhouri K, Hubert L, Garrigue P, Nollet M, Essaadi A, et al. Soluble CD146 boosts therapeutic effect of endothelial progenitors through proteolytic processing of short CD146 isoform. Cardiovasc Res. (2016) 111:240–51. doi: 10.1093/cvr/cvw096
66. Green LA, Njoku V, Mund J, Case J, Yoder M, Murphy MP, et al. Endogenous transmembrane TNF-alpha protects against premature senescence in endothelial colony forming cells. Circ Res. (2016) 118:1512–24. doi: 10.1161/CIRCRESAHA.116.308332
67. Kim H, Prasain N, Vemula S, Ferkowicz MJ, Yoshimoto M, Voytik-Harbin SL, et al. Human platelet lysate improves human cord blood derived ECFC survival and vasculogenesis in three dimensional (3D) collagen matrices. Microvasc Res. (2015) 101:72–81. doi: 10.1016/j.mvr.2015.06.006
68. Tasev D, van Wijhe MH, Weijers EM, van Hinsbergh VWM, Koolwijk P. Long-term expansion in platelet lysate increases growth of peripheral blood-derived endothelial-colony forming cells and their growth factor-induced sprouting capacity. PLoS ONE (2015) 10:e0129935. doi: 10.1371/journal.pone.0129935
69. Caiado F, Dias S. Endothelial progenitor cells and integrins: adhesive needs. Fibrogenesis Tissue Repair (2012) 5:4. doi: 10.1186/1755-1536-5-4
70. Guerin CL, Rossi E, Saubamea B, Cras A, Mignon V, Silvestre J, et al. Human very small embryonic-like cells support vascular maturation and therapeutic revascularization induced by endothelial progenitor cells. Stem Cell Rev Rep. (2017) 13:552–60. doi: 10.1007/s12015-017-9731-7
71. Smadja DM. Bone marrow cell therapy in cardiovascular disease drives us slowly to a better identification of the active cell component. Stem Cell Res Ther. (2014) 5:16. doi: 10.1186/scrt405
72. Shafiee A, Patel J, Wong HY, Donovan P, Hutmacher DW, Fisk NM, et al. Priming of endothelial colony-forming cells in a mesenchymal niche improves engraftment and vasculogenic potential by initiating mesenchymal transition orchestrated by NOTCH signaling. FASEB J. (2017) 31:610–24. doi: 10.1096/fj.201600937
73. Foubert P, Matrone G, Souttou B, Leré-Déan C, Barateau V, Plouët J, et al. Coadministration of endothelial and smooth muscle progenitor cells enhances the efficiency of proangiogenic cell-based therapy. Circ Res. (2008) 103:751–60. doi: 10.1161/CIRCRESAHA.108.175083.
74. Lin R-Z, Moreno-Luna R, Zhou B, Pu WT, Melero-Martin JM. Equal modulation of endothelial cell function by four distinct tissue-specific mesenchymal stem cells. Angiogenesis (2012) 15:443–55. doi: 10.1007/s10456-012-9272-2
75. Kang K-T, Lin R-Z, Kuppermann D, Melero-Martin JM, Bischoff J. Endothelial colony forming cells and mesenchymal progenitor cells form blood vessels and increase blood flow in ischemic muscle. Sci Rep. (2017) 7:770. doi: 10.1038/s41598-017-00809-1
76. Smadja DM, Levy M, Huang L, Rossi E, Blandinières A, Israel-Biet D, et al. Treprostinil indirectly regulates endothelial colony forming cell angiogenic properties by increasing VEGF-A produced by mesenchymal stem cells. Thromb Haemost. (2015) 114:735–47. doi: 10.1160/TH14-11-0907.
Keywords: endoglin, endothelial progenitors, EPC, ECFCs, HHT1, integrins, TGF-β
Citation: Rossi E, Bernabeu C and Smadja DM (2019) Endoglin as an Adhesion Molecule in Mature and Progenitor Endothelial Cells: A Function Beyond TGF-β. Front. Med. 6:10. doi: 10.3389/fmed.2019.00010
Received: 27 June 2018; Accepted: 14 January 2019;
Published: 30 January 2019.
Edited by:
Peter S. Steyger, Oregon Health and Science University, United StatesReviewed by:
Eleni Papakonstantinou, Aristotle University of Thessaloniki, GreeceCopyright © 2019 Rossi, Bernabeu and Smadja. This is an open-access article distributed under the terms of the Creative Commons Attribution License (CC BY). The use, distribution or reproduction in other forums is permitted, provided the original author(s) and the copyright owner(s) are credited and that the original publication in this journal is cited, in accordance with accepted academic practice. No use, distribution or reproduction is permitted which does not comply with these terms.
*Correspondence: Elisa Rossi, ZWxpc2Eucm9zc2lAcGFyaXNkZXNjYXJ0ZXMuZnI=
Disclaimer: All claims expressed in this article are solely those of the authors and do not necessarily represent those of their affiliated organizations, or those of the publisher, the editors and the reviewers. Any product that may be evaluated in this article or claim that may be made by its manufacturer is not guaranteed or endorsed by the publisher.
Research integrity at Frontiers
Learn more about the work of our research integrity team to safeguard the quality of each article we publish.