- 1Dhawan Lab at Mowat Labs, Institute of Liver Studies, King's College London, King's College Hospital, London, United Kingdom
- 2Paediatric Liver, GI and Nutrition Centre, King's College London, King's College Hospital, London, United Kingdom
Hepatocyte transplantation has emerged as an alternative to liver transplant for liver disease. Hepatocytes encapsulated in alginate microbeads have been proposed for the treatment of acute liver failure, as they are able to provide hepatic functions while the liver regenerates. Furthermore, they do not require immunosuppression, as the alginate protects the hepatocytes from the recipient's immune cells. Mesenchymal stromal cells are very attractive candidates for regenerative medicine, being able to differentiate into cells of the mesenchymal lineages and having extensive proliferative ability. When co-cultured with hepatocytes in two-dimensional cultures, they exert a trophic role, drastically improving hepatocytes survival and functions. In this study we aimed to (i) devise a high throughput system (HTS) to allow testing of a variety of different parameters for cell encapsulation and (ii) using this HTS, investigate whether mesenchymal stromal cells could have beneficial effects on the hepatocytes when co-encapsulated in alginate microbeads. Using our HTS platform, we observed some improvement of hepatocyte behavior with MSCs, subsequently confirmed in the low throughput analysis of cell function in alginate microbeads. Therefore, our study shows that mesenchymal stromal cells may be a good option to improve the function of hepatocytes microbeads. Furthermore, the platform developed may be used for HTS studies on cell encapsulation, in which several conditions (e.g., number of cells, combinations of cells, alginate modifications) could be easily compared at the same time.
Introduction
Although liver transplantation represents the treatment of choice for patients with end-stage liver disease and liver-based metabolic disorders, in the last three decades human hepatocyte transplantation has emerged as a potential alternative (1–5). Cell transplantation offers several advantages over liver transplantation, being less invasive and readily available, as the cells can be cryopreserved. It also increases the number of recipients who could benefit from the same donor liver, as it is estimated that a cell number equivalent to 10–15% of the recipient liver cell mass is sufficient to replace the liver function. Furthermore, native liver remains in place allowing potential regeneration in patients with acute liver failure (ALF), or future gene therapy treatment for patients with genetic diseases (6, 7).
Intraperitoneal transplantation of human hepatocytes encapsulated in alginate microbeads is an attractive option for the management of patients with ALF, as hepatocytes microbeads can provide hepatic functions while the patient's own liver regenerates, with the advantage of not requiring any immunosuppression, as the alginate protects transplanted hepatocytes from the recipient's immune cells (8, 9). The technique has been proven successful in a rat model of acute liver injury (10). However, the scarce availability of good quality human hepatocytes as a result of the frequent long ischemia time of donor tissues, their short life span after isolation, and the damages incurred after cryopreservation represent major limitations for further development of this technique. Therefore, although theoretically readily available for cell transplantation, hepatocytes are not always suitable to this aim as their quality is often sub-optimal.
Mesenchymal stromal cells (MSC) are multipotent cells found within all mammalian supportive stromal tissues, mainly isolated from bone marrow, umbilical cord and adipose tissues, and able to differentiate in vitro into mesenchymal tissue cells, i.e., adipocytes, osteoblasts, and chondrocytes (11–13). We and other groups have shown that MSC drastically improve the survival of hepatocytes and their liver-specific functions in standard cell culture conditions (14–16). Therefore, the main aim of this study was to investigate whether the co-encapsulation of human hepatocytes with MSC in alginate microbeads improved hepatocytes viability and functions.
Because alginate microbead encapsulation is a tedious process with very low throughput, this study also aimed at developing a new platform for fast production of cell alginate microdisks, that would eventually allow comparison of numerous encapsulation conditions—cell types, alginate chemistry, alginate combination, etc. This HTS is based on the cross-linking of alginate directly in situ, in cell culture wells. Once this HTS was setup, we used it to compare the function of hepatocytes on their own and with MSCs, and validated the results using actual alginate microbeads.
Alginate, or alginic acid, is a polysaccharide polyanionic linear co-polymer containing blocks of (1,4)-linked β-D-mannuronic (M block) and α-L-guluronic (G block) acids (17–19). Alginate is usually available as sodium-alginate, a sodium salt of alginic acid, where the carboxyl ends of G and M blocks are ionically bound to sodium. Alginate gelation process is done by the addition of divalent cations, such as calcium ions, which replace sodium ions and take part in the interchain ionic binding between G blocks, or cross-linking, with formation of a three-dimensional network (Figure 1). Alginate has been widely used as a biomaterial in cell encapsulation for regenerative medicine applications, as it is highly biocompatible and has low immunogenic properties (20–23). Two main methods can be used for alginate ionic cross-linking, diffusion and internal gelation. The diffusion is mediated by a rapid transfer of gelling ions (e.g., Ca2+) from a surrounding solution into the polymer network. It is used when cells are encapsulated in alginate microbeads using an encapsulator, such as the Buchi encapsulator. In this process, cell-alginate droplets are formed in the encapsulator and fall into a CaCl2 bath, where they instantaneously crosslink, forming a gel, through the diffusion of calcium ions into the droplet (9, 17). Conversely, the internal gelation is obtained when a low soluble source of divalent ions, such as calcium carbonate (CaCO3), is directly mixed with an alginate solution into which the calcium ions are released by addition of a slow acidifier, e.g., D-glucono-δ-lactone (GDL). As GDL is a proton donor and CaCO3 a 2-protons acceptor, the adjustment of the molar ratio at 1:2 (CaCO3:GDL) allows to keep a neutral pH, compatible with cell encapsulation (24–29).
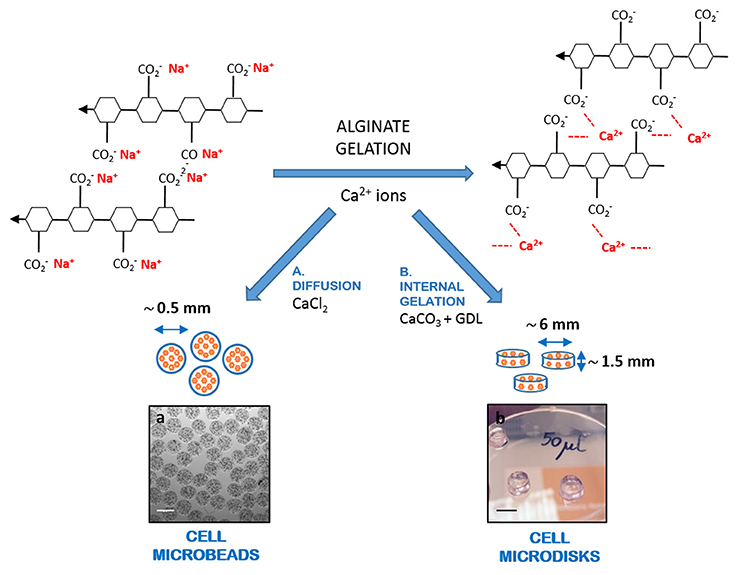
Figure 1. Schematic representation of alginate gelation. Alginate gelation is mediated by the replacement of sodium ions with divalent calcium ions, allowing three-dimensional cross-linking. Gelation can be obtained through (a) diffusion, where calcium ions diffuse from a solution containing a highly soluble calcium salt (e.g., CaCl2) to the alginate gels; or (b) internal gelation, where a low soluble source of calcium (e.g., CaCO3) is mixed with the alginate, along with a slow acidifier (e.g., GDL), with production of a gel that assumes the shape of the container where it forms. Gelation by diffusion is used for cell microbeads production, while internal gelation is proposed here for the production of alginate microdisks. Scale bars: (a) 500 μm; (b) 5 mm.
Although the gelation based on diffusion is extremely fast, the production of alginate microbeads using an encapsulator can be very time-consuming, as the main chamber must be sterilized before every production (9), and replaced for each experimental condition variation. By contrast, the internal gelation approach, despite being slower than the diffusion, allows a direct in situ cross-linking of cell-alginate suspension and has the great potential of providing a high throughput screening (HTS) platform, allowing a rapid and parallel testing of different conditions at the same time, thus saving time when a number of encapsulation conditions are compared.
Therefore, the second aim of this study was to investigate whether this new platform for cell encapsulation in alginate, based on internal gelation with production of microdisks, could provide similar results to those obtained by cell encapsulation in alginate microbeads and be used for a variety of cell function analysis.
We first showed that the new proposed HTS platform was able to detect trends seen in the microbeads, as encapsulated hepatocytes in alginate microdisks, showed a similar viability and function variation over time. We then used the new platform to study the effects of co-encapsulation of hepatocytes and mesenchymal stromal cells in alginate microdisks and we found that hepatocytes functions were partially improved by MSC addition. To validate these results, we encapsulated hepatocytes with or without MSC in alginate microbeads. We found that all the hepatic functions analyzed were significantly enhanced by MSC co-encapsulation, confirming the results observed in alginate microdisks and further supporting the use of our HTS platform as a reliable method for the initial pre-screening of encapsulation conditions.
Materials and Methods
Human Cell Isolation
All human tissues were approved for research use in accordance with the Research Ethics Committee of King's College Hospital. Written informed consent was obtained from donor relatives or patients.
Human hepatocytes (HC) were isolated from donor liver tissues rejected or unused for orthotopic liver transplantation. Isolation of human hepatocytes was carried out using a modified collagenase perfusion technique (30). Briefly, major hepatic vessels were cannulated and perfused with Hank's buffered salt solution (HBSS, Lonza) containing 0.5 mM ethylene glycol-bis(2-aminoethylether)-N,N,N′,N′-tetraacetic acid (EGTA, Sigma Aldrich) and 4.6 mM 4-(2-Hydroxyethyl)piperazine-1-ethanesulfonic acid (HEPES, Sigma Aldrich). The liver was then flushed with plain HBSS to remove any residue of EGTA. Finally, the tissue was perfused with Eagle's minimum essential medium (EMEM, Lonza) containing 0.05% (w/v) of collagenase P (Roche) at 37°C. Once digested, the tissue was minced and sieved. Hepatocytes were purified by washing 3 times in ice-cold EMEM and centrifuged at 50 g at 4°C for 5 min. Cell number and viability were determined by trypan blue exclusion test. Cells were cryopreserved in University of Wisconsin solution (Bridge to Life) with 5% (w/v) glucose and 10% (v/v) dimethylsulfoxide (DMSO, Sigma Aldrich), using a controlled rate freezer (Kryo 10, Planer Products) and stored at −140°C for later use.
Mesenchymal stromal cells (MSC) were isolated from the Wharton's jelly (WJ), by mechanical dissection, as already described (31), with minor modifications. Briefly, cords from C-sections were obtained from the Anthony Nolan Trust and collected in phosphate buffered saline (PBS, Gibco) with 40 μg/mL of gentamicin (Sigma Aldrich). Cords were cut into sections to expose the WJ, which was minced into very fine pieces (1–3 mm2). The explants were plated on sterile petri dishes, allowed to adhere for up to 5 min and covered with MSC culture medium, Minimum Essential Medium α (MEMα Life Technologies), 5% (v/v) platelet lysate (Stemulate Cook Regentec), 40 μg/mL of gentamicin. Cord samples were then incubated at 37°C, 5% CO2, and medium half replaced every 3-6 days. Gentamicin was used in the MSC medium for the first 1–2 weeks of culture and then replaced by penicillin/streptomycin (100 U/mL and 100 μg/mL, respectively). Once cell cultures were established, they were expanded, quality controlled, and cryopreserved in MEMα containing 4% (w/v) human albumin (Zenalb®20) and 10% (v/v) DMSO. Cells were positive for stem cells markers (i.e., CD73, CD90, and CD105, expression >75%) and negative for hematopoietic markers (i.e., CD14, CD34, and CD45, <2%) (32).
Encapsulation of Cells in Alginate Microbeads or Microdisks
Human hepatocyte were encapsulated with or without mesenchymal stromal cells in alginate microbeads (MB) using an IE-50R encapsulator (Inotech Encapsulation AG, Dottikon, Switzerland) as previously described (10, 15). Briefly, ultra-pure sodium alginate (PRONOVA SLG20; NovaMatrix, Sandvika, Norway) was dissolved in 0.9% (w/v) NaCl (sterile saline, Baxter) to give a final concentration of 1.5% alginate solution (w/v) and mixed with cells. Microbeads were produced with a 250 μm nozzle, polymerized in 100 mM CaCl2 solution for 10 min and washed twice with 0.9% (w/v) NaCl to remove excess Ca2+ ions.
To prepare “in situ” cross-linked alginate microdisks (MDs), an internal gelation strategy with some modifications was used (25). Briefly, alginate solution in 0.9% NaCl was mixed with cells (same density as in the microbeads) in the presence of an aqueous suspension of CaCO3 (Sigma Aldrich) and D-glucono-delta-lactone (GDL, Sigma Aldrich). The pH was kept neutral by adjusting the molar ratio of CaCO3/GDL and the final alginate concentration was 1.5% (w/v). Two different concentrations of calcium carbonate were tested (44 and 52 mM, for microdisks 1 and 2, respectively). Polymerization was obtained at 37°C for 20 min.
The same volume of microbeads/microdisks (50 μL) was used in each well of a 96-well microplate.
Cell Microbeads/Microdisks Culture
Microbeads and microdisks were cultured in culture medium consisting of Williams E (Sigma Aldrich) supplemented with 10% (v/v) heat-inactivated fetal calf serum (GE Healthcare Hyclone), 2 mM L-glutamine (Gibco), 10 mM Hepes (Gibco), 10 mg/L insulin, 5.5 mg/L transferrin, 670 μg/L sodium selenite (ITS, Gibco), 10−7 M dexamethasone (Sigma Aldrich), 100 U/mL penicillin, and 100 μg/mL streptomycin (Gibco). Fifty microliters of MB or MD were maintained in 200 μL of culture medium per well of a 96-well plate in a humidified incubator at 37°C and 5% CO2. Medium was replaced every 2–3 days.
Images on microbeads were taken using an inverted microscope (Leica Microsystems Ltd., Milton Keynes, UK).
Assessment of Cell Viability and Total Protein Content
Cell viability was determined using a calcein-AM assay. Briefly, supernatant was removed and microbeads or microdisks were depolymerized in situ by adding 200 μL of 50 mM ethylenediaminetetraacetic acid (EDTA, Sigma Aldrich), as previously described (23). Dissolution of alginate microbeads or microdisks was allowed by incubation with EDTA for 5 min at 37°C. Cells were recovered by centrifugation, resuspended in 100 μL of PBS and transferred in different wells of a black 96–well plate. Immediately before the detection, 100 μL of 4 μM calcein-AM (Santa Cruz) in PBS was added per well. As calcein-AM penetrates the cells and is converted into green fluorescent calcein by cellular esterases present only in viable cells, cell viability was assessed by measuring the green fluorescence emitted by the cells every minute for 30 min using FLUOstar Omega plate reader (BMG Labtech, Aylesbury, United Kingdom) with an excitation wavelength of 495 nm and an emission wavelength of 515 nm. Results were calculated as relative fluorescence units (RFU)/minute and represented as a percentage over baseline at day 0.
For total protein content measurement, microbeads, or microdisks were depolymerized as described above. Released cells were collected by centrifugation and lysed using a modified lysis buffer, as previously described (50 mM Tris-HCl pH 7.5, 100 mM NaCl, 50 mM NaF, 1 mM Na3VO4, 30 mM sodium pyrophosphate, 0.5% NP-40, and 0.5 mM PMSF, Sigma Aldrich) (33). Cells lysate were centrifuged at 14,000 rpm for 10 min to remove cell debris. Quantitation of total protein was performed by using bicinchoninic acid (BCA) Protein Assay Kit (PierceTM), according to the manufacturer's instructions.
Tests of Hepatocyte-Specific Functions
Human albumin and alpha1-antitrypsin secreted in the culture medium over 24 h by microbeads or microdisks and/or released in rat plasma were quantified using a human albumin/alpha1-antritrypsin Enzyme Linked Immunosorbent Assays (ELISA). Briefly, an ELISA plate was coated with 10 μg/ml of goat anti-human albumin antibody or 10 μg/ml of goat anti-human alpha1-antitrypsin (Bethyl Laboratories, Tx, USA) in coating buffer (50 mM carbonate-bicarbonate, pH 9.6, Sigma Aldrich) for 1 h, washed with wash buffer (50 mM Tris, 140 mM NaCl, 0.05% Tween 20, pH 8.0, Sigma Aldrich) and blocked with blocking buffer (1% bovine serum albumin, 50 mM Tris, 140 mM NaCl, pH 8.0, Sigma Aldrich) for 30 min. One hundred microliters of standards (400–6.25 ng/L) or samples were added and incubated at room temperature for 1 h. The plate was washed and a Horseradish Peroxidase (HRP)-conjugated goat anti-human albumin antibody or anti-human alpha1-antritrypsin antibody (1 mg/mL, Bethyl Laboratories, Tx, USA) added at a 1:150,000 or 1:50,000 dilution, respectively, in diluent (50 mM Tris, 140 mM NaCl, 1% bovine serum albumin, 0.05% Tween 20, pH 8.0, Sigma Aldrich) for 1 h. 3,3′,5,5′-tetramethylbenzidine (TMB) substrate solution (Insight Biotechnology) was added and incubated in the dark for 10 min. The reaction was stopped with 2M H2SO4. The optical density was measured at λ = 450 nm on a FLUOstar Omega plate reader.
For urea synthesis measurement, microbeads or microdisks were washed twice with PBS and incubated with 5 mM ammonium chloride in serum-free medium for 6 h at 37°C, 5% CO2. The supernatant was collected and analyzed using a QuantiChrom Urea Assay Kit (Universal Biologicals, Cambridge, UK), according to the manufacturer's instructions.
In vivo Studies
Sprague Dawley male rats 8–10 weeks old and weighing between 200 and 300 g were used in this study. Animals were maintained in conventional housing facilities and received standard care. The experiments were performed after acclimatization for at least 7 days.
Rats were transplanted intraperitoneally with alginate microbeads containing either HC alone or co-encapsulated with MSC, or transplant medium (sham) (n = 3 each group). The microbeads were produced as described above and resuspended in transplant medium (CMRL) at a ratio 2:1 (microbeads:CMRL). Each animal received microbeads suspension at a dose of 10 ml/kg.
Blood sampling was performed at days 1, 3, and 7 after transplantation and plasma obtained through centrifugation at 2,000 × g for 15 min. As human cells were used, human albumin and human alpha1-antitrypsin released in the plasma were analyzed (ELISA assay, see above) as an indirect method to detect microbeads performance.
Statistical Analysis
Statistical analyses were performed using GraphPad Prism 7.01 software (GraphPad, CA, USA). Differences between groups were assessed using two-way ANOVA with Sidak (2 groups) or Tukey (>2 groups) multiple testing correction. Differences were considered statistically significant when p-values were lower than 0.05. All data are presented as mean values ± standard error of the mean deviation (SEM).
Results
Comparison of Alginate Microbeads and Microdisks for Hepatocyte Encapsulation
A preliminary study was performed to identify the best conditions to crosslink the alginate in situ, directly in the wells of a 96-well plate. To this aim, alginate was mixed with increasing concentrations of CaCO3 (from 20 to 60 mM) and the gelling process was triggered by addition of GDL. The microdisks were observed for up to 10 days and assessed for their ability to efficiently keep an even shape, without showing any obvious signs of shrinkage. The best CaCO3 concentrations allowing the production of stable microdisks were 44 and 52 mM, hereafter named MD1 and MD2, subsequently tested for cell encapsulation. Lower concentration of CaCO3 resulted in the formation of microdisks that dissolved over time.
Primary human hepatocytes were then encapsulated in alginate microdisks (MD1 or MD2) or microbeads (MB). Cell viability was similar in all the conditions tested at different time points, except at day 1 when hepatocytes in MD2 showed a significant lower viability (46.4 ± 14.5%) than in MB (73.2 ± 5.7, p ≤ 0.05) (Figure 2A). Likewise, the total protein content (TP) measured on hepatocytes encapsulated in microbeads or microdisks was similar, showing a significant difference only at day 1, when cells were encapsulated in MD1 (TP = 32.6 ± 1.5 μg, p < 0.05), but not in MD2 (TP = 36.3 ± 2.4 μg), compared to MB (TP = 41.7 ± 3.2 μg) (Figure 2B).
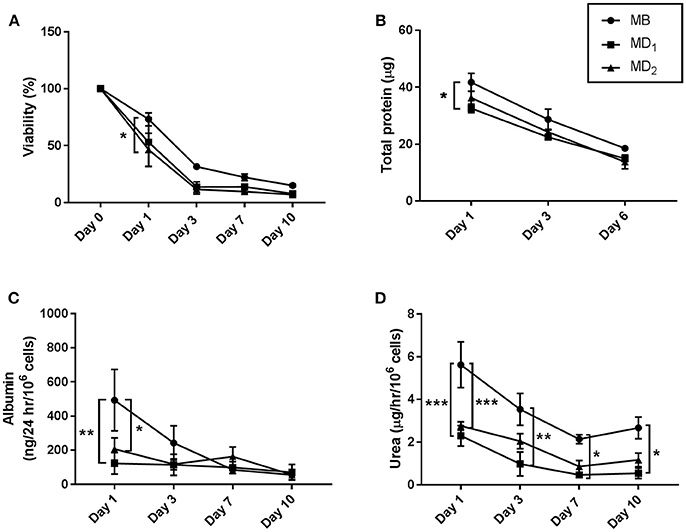
Figure 2. Viability, total protein content, albumin and urea production of hepatocytes encapsulated in alginate microbeads (MB) or microdisks (MD), containing 44 mM (MD1), or 52 mM (MD2) of CaCO3. (A) Cell viability measured by calcein-AM assay and expressed as % over baseline value at day 0. Cells were released from the alginate by incubation of microbeads/microdisks with 50 mM EDTA. (B) Total protein content was assessed after alginate microbeads/microdisks depolymerization as above. (C) Albumin synthesis was measured by ELISA from microdisk/microbead supernatants. (D) Urea production measured on disk/bead supernatants following incubation with 5 mM ammonium chloride for 6 h. N = 3. Data represent mean ± SD. *p < 0.05; **p ≤ 0.01; ***p ≤ 0.001.
The ability of hepatocytes to produce albumin and urea was tested as a direct method to measure hepatocytes-specific functions. Albumin assay showed significant differences between MB and MD at day 1, when hepatocytes encapsulated in MB released higher amount of albumin (493 ± 180 ng/24 h/106 cells) than in MD1 (123 ± 63 ng/24 h/106 cells, p ≤ 0.01) and MD2 (207 ± 65 ng/24 h/106 cells, p ≤ 0.05). However, a similar amount of albumin was released in the different conditions at later time points (Figure 2C). Similarly, the production of urea was significantly decreased at day 1 in MD1 and MD2 (2.3 ± 0.5 and 2.8 ± 0.2 μg/h/106 cells, respectively) compared to MB (5.6 ± 1.1 μg/h/106 cells, p ≤ 0.001). Whilst the urea produced by hepatocytes encapsulated in MD1 was significantly lower than in MB in all the time points analyzed, no significant difference was observed in MD2 at late time points (Figure 2D).
Co-encapsulation of Human Hepatocytes and Mesenchymal Stromal Cells in Alginate Microdisks
As the function of hepatocytes appeared most similar to microbeads in the MD2 conditions, we decided to use these microdisks as a new platform for direct in situ alginate cross-linking to investigate the effect of mesenchymal stromal cells (MSC) co-encapsulation with hepatocytes (HC).
Cell viability was expressed as percentage of the baseline value at day 0, in order to compare microdisks containing HC with or without MSC. Both groups showed similar viability, except at day 7, when HC co-encapsulated with MSC showed higher viability than HC alone (13.9 ± 1.7 vs. 58.9 ± 18.8% for HC and HC+MSC microdisks, respectively, p ≤ 0.05) (Figure 3A).
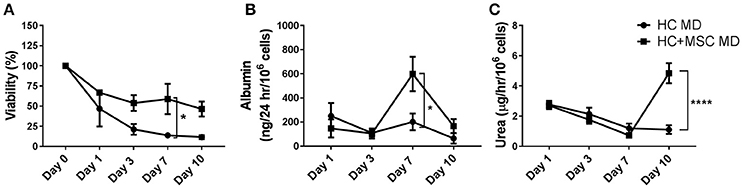
Figure 3. Viability, albumin and urea production of hepatocytes (HC) encapsulated in alginate microdisks with and without mesenchymal stromal cells (MSC). (A) Cell viability measured by calcein-AM assay and expressed as % over baseline value at day 0. Cells were released by incubation of microdisks with EDTA. (B) Albumin synthesis measured by ELISA on cell supernatants. (C) Urea production measured using a colorimetric assay on cell supernatant following incubation with 5 mM ammonium chloride for 6 h. Ureogenesis is expressed as μg of urea released in 1 h by 106 cells. N = 3. Data represent mean ± SEM. *p < 0.05; ****p ≤ 0.0001.
Similarly, albumin production was significantly improved when HC were co-encapsulated with MSC 7 days after encapsulation (201 ± 69 vs. 598 ± 143 ng/24 h/106 cells for HC and HC+MSC microdisks, respectively, p ≤ 0.05) (Figure 3B). Interestingly, urea production was significantly improved in the co-encapsulated cells only later, at day 10 (1.1 ± 0.3 vs. 4.8 ± 0.7 μg/h/106 cells, p ≤ 0.0001) (Figure 3C).
Co-encapsulation of Human Hepatocytes and Mesenchymal Stromal Cells in Alginate Microbeads
As albumin and urea production were significantly improved when HC were co-encapsulated with MSC in alginate microdisks, we decided to produce alginate microbeads containing either HC or HC+MSC, to see whether these results could be validated. The alginate microbeads obtained using HC with or without MSC were similar in shape and size and did not show any signs of shrinkage over time (Figure 4).
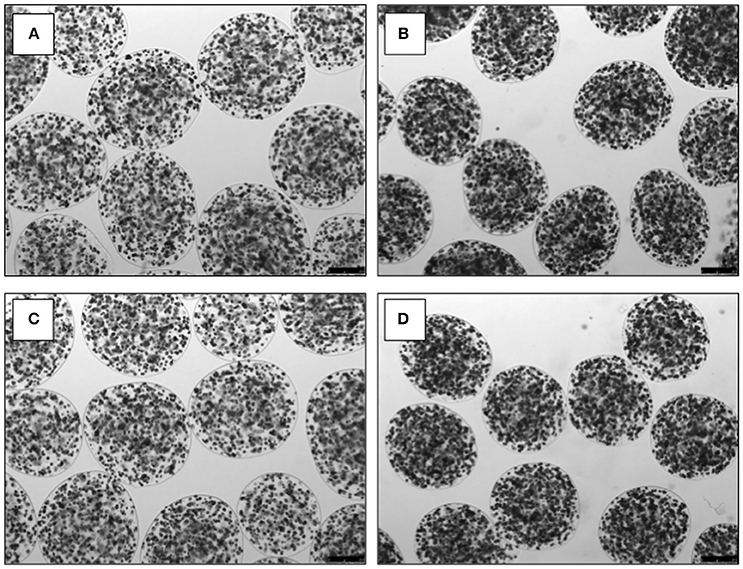
Figure 4. Images of alginate microbeads containing hepatocytes (A,C) or hepatocytes and mesenchymal stromal cells (B,D). Images on microbeads were taken at day 1 (A,B) and day 7 (C,D), using an inverted microscope (Leica Microsystems Ltd., Milton Keynes, UK). Scale bar: 250 μm.
Cell viability did not show any significant difference in the groups analyzed (Figure 5A). Albumin and urea production were measured to assess hepatocyte-specific functions. Albumin released in the supernatant was significantly increased in the co-encapsulated cells compared to HC alone at day 7 (85 ± 5 vs. 1516 ± 182 ng/24 h/106 for HC and HC+MSC microbeads, respectively, p ≤ 0.05) and day 10 (56 ± 28 vs. 1321 ± 780 ng/24 h/106 cells, p ≤ 0.05) (Figure 5B). Similarly, urea production was significantly improved by the addition of MSC at late time points (2.3 ± 0.4 vs. 6.0 ± 0.6μg/h/106 cells at day 7, p ≤ 0.01, and 2.1 ± 0.2 vs. 8.0 ± 1.4 μg/h/106 cells at day 10, p ≤ 0.0001, for HC and HC+MSC microbeads, respectively) (Figure 5C).
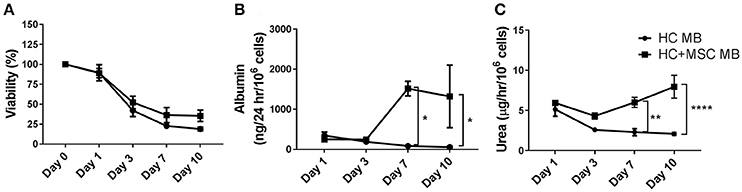
Figure 5. Viability, albumin and urea production of hepatocytes (HC) encapsulated in alginate microbeads with and without mesenchymal stromal cells (MSC). (A) Cell viability measured by calcein-AM assay and expressed as % over baseline value at day 0, after release from the alginate. (B) Albumin synthesis measured by ELISA on cell supernatants. (C) Urea production measured using a colorimetric assay on cell supernatant following incubation with 5 mM ammonium chloride for 6 h. Ureogenesis is expressed as μg of urea released in 1 h by 106 cells. N = 3. Data represent mean ± SEM. *p < 0.05; **p < 0.01; ****p ≤ 0.0001.
Function of Co-encapsulated Human Hepatocytes and Mesenchymal Stromal Cells Microbeads in vivo
Normal Sprague Dawley rats were transplanted intraperitoneally with alginate microbeads containing either hepatocytes alone or co-encapsulated with MSC. Human cells were used so that the human proteins detected in rat plasma could be used as an indirect measurement of microbeads functions. Plasma samples obtained from the animals were tested for human albumin (Alb) and alpha1-antitrypsin (AAT) levels (Figure 6). Interestingly, both human proteins were significantly higher in the animals transplanted with a combination of HC and MSC compared to HC alone the day after transplantation (Alb: 43.4 ± 5.6 vs. 12.8 ± 4.8 ng/ml, p ≤ 0.0001; AAT: 34.9 ± 5.4 vs. 14.1 ± 9.3 ng/ml, p ≤ 0.01). As expected, these proteins were absent in the control animal transplanted only with transplant medium (sham). Three days after transplantation there was a decrease in the levels of human proteins in rat plasma, with significant differences between the groups analyzed only for alpha1-antitrypsin (2.4 ± 2.4 vs. 16.5 ± 1.4 ng/ml, p ≤ 0.05, in HC vs. HC+MSC microbeads transplanted groups, respectively). The human proteins became undetectable at day 7.
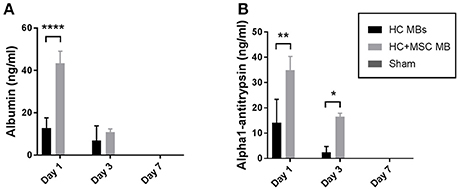
Figure 6. Human liver-specific proteins released in rat plasma after microbeads transplantation. Alginate microbeads containing HC with and without MSC were transplanted intraperitoneally in normal Sprague-Dawley rats. Blood samples were collected at day 1, 3, and 7 and plasma obtained through centrifugation. Human albumin (A) and alpha1-antitrypsin (B) levels were measured by ELISA. Data represent mean ± SEM. *p < 0.05; **p < 0.01; ****p ≤ 0.0001.
Discussion
Acute Liver Failure (ALF) has a high mortality risk in patients listed for liver transplantation not receiving an allograft (34). Transplantation of human hepatocytes encapsulated in alginate microbeads (MB) represents a promising alternative to liver transplantation, as cells provide missing hepatic functions while the patient's own liver regenerates. Furthermore, the presence of alginate allows protection of the hepatocytes from immune cells, thus not requiring immunosuppression after transplantation. Human primary hepatocytes are isolated from donor livers that are unused for liver transplantation and offered for cell isolation. Unfortunately, these marginal livers frequently have long ischemia time, high content of fats, giving rise to hepatocytes of poor quality and with limited survival (35). An ideal treatment of ALF with encapsulated human hepatocytes should provide hepatic functions for several days, thus allowing the regeneration of the native liver. We tried to address this issue co-encapsulating human hepatocytes (HC) with mesenchymal stromal cells (MSC), knowing that MSC are able to improve HC functions when co-cultured in 2-D cell cultures, as already shown by us and other groups (15).
Although the encapsulation of cells in alginate microbeads is a relatively quick process, it requires prior sterilization of the main chamber containing the microbeads, thus making the whole technique very time-consuming when a number of different conditions are compared. An ideal platform to test a multitude of encapsulation conditions at the same time may be represented by thin layer microdisks made of alginate produced directly in cell culture plates. Unfortunately, it is not possible to prepare alginate microdisks by simply adding CaCl2 to an alginate solution in a cell culture well, as the very rapid binding of calcium ions to the G blocks of the alginate determines the formation of uneven disks. Galateanu et al. were able to prepare thin alginate layers directly in culture dishes, by placing the alginate solution between two filter papers soaked in CaCl2 or calcium gluconate solution. The release of calcium ions from the paper was sufficiently slow to allow the production of even microdisks (36). Although effective, this method was not compatible with the development of a high throughput screening platform, as it was time-consuming and required the removal of filter papers from each disk after production to allow exchanges of gases, nutrients and cell products, between the gels and cell culture medium.
We decided to test a different approach for HTS cell encapsulation: in situ cross-linking the alginate in cell culture wells via internal gelation, using CaCO3 and GDL. Internal gelation has been already proven safe for cell encapsulation, having been proposed for regenerative medicine applications, for instance for cell delivery in tissue regeneration therapy when a delayed in situ crosslinking is needed, e.g., bone regeneration applications (37, 38). Nevertheless, this approach was never tested for HTS applications with the aim of screening cell encapsulation conditions to use for cell microbeads production. In other words, the two main methods for ionic alginate gelation, i.e., internal gelation and ions diffusion, have never been compared to see whether they could support encapsulated cells to a similar extent.
Such a comparison is essential, considering that the internal structure of alginate microbeads and microdisks is very different. The microbeads, produced by ions diffusion, exhibit an inhomogeneous alginate distribution, as the alginate concentration gradually decreases toward the center of the gel. By contrast, the microdisks, obtained by internal gelation, have a homogenous structure as the concentration of calcium is even throughout the gel. Furthermore, the size of the microbeads and microdisks is very different, the volume of each microdisk being more than 50 times larger than the microbead. Overall, we observed that hepatocytes encapsulated in microbeads performed better than in microdisks in terms of viability, total protein content and main hepatic functions. Between the two microdisks polymerization conditions used, MD2 showed better cell functions than what was observed in MD1. We believe that the optimization of microdisks encapsulation conditions is paramount when this approach is proposed for HTS applications. In fact, different alginates with specific molecular weight and G/M composition may require fine adjustments of CaCO3 concentration.
Since hepatocytes encapsulated in alginate microdisks MD2, although inferior to microbeads the day after encapsulation, showed a similar trend in terms of functions at later time points, we decided to use the microdisks to study the effect of the co-encapsulation of hepatocytes with mesenchymal stromal cells. Our results indicated that cell viability, albumin production and ureogenesis were partially improved by the addition of MSC (Figure 3). However, when the co-encapsulation was tested in alginate microbeads, hepatocyte ability of producing albumin was not just improved by MSC, as seen in the microdisks, but also increased over time, in a way that 1 week after production beads containing HC+MSC were able to synthesize more than 10 times the amount of albumin produced at day 1. A similar trend was observed in a previous study (15), where 2-D co-cultures of HC with MSC resulted in more than 10-fold increased albumin starting at day 7 and keep increasing up to day 15. Whether this effect is due to the potential of MSCs to differentiate into hepatocytes or to their stimulatory/trophic effects is still unclear. Furthermore, hepatocytes co-encapsulated with MSC in alginate microbeads produced a higher amount of urea compared to HC on their own. This feature was observed in the microdisks only at day 10, while it was anticipated in the microbeads, starting at day 7. A possible reason for this difference in timing and in the overall lower levels of cell functions observed in the microdisks may be related to a differential cell oxygenation between microbeads and microdisks. Cells encapsulated in the microbeads will be submitted to a lower oxygenation gradient than when embedded in microdisks, due to the smaller size. The lower oxygen tension could affect the cells in a different way when they are encapsulated in microbeads or microdisks, thus explaining the difference observed (39–41).
Although the performance of the cells encapsulated in alginate microdisks did not seem as good as what observed in alginate microbeads, the beneficial effect of MSC addition was detectable in the microdisks too, even though to a less extent to what observed in the microbeads. Hence, for finer comparison, after the initial screening, the production of microbeads was necessary to detect the full extent of differences between the two conditions. This suggests that cell encapsulation in microdisks could be a valuable tool for high throughput pre-screening studies to identify gross differences between the conditions analyzed, such as combinations of cells, as described here in our small scale study, and potentially in larger investigations to compare different alginate modifications (e.g., peptide, microfiber, minerals), to titrate cell density for alginate encapsulation, or testing the encapsulated cells with different compounds. The same testing can be done using microbeads, however the production of microdisks is much faster, thus more compatible with HTS tests.
The improved functions observed in vitro were further confirmed in vivo. In fact, a higher amount of human liver-specific proteins were detected in the plasma of rats transplanted with HC and MSC co-encapsulated microbeads compared to HC microbeads. However, human albumin and alpha1-antitrypsin were detected only up to 3 days after transplantation. A possible reason for this rapid decline may be related to the fact that human cells were transplanted in a rat recipient. Although immune cells are not able to penetrate the microbeads, the human proteins released can be targeted by the recipient's immunity and destroyed.
Our in vivo study was not performed in a model of acute liver failure, as this application was beyond the main scope of our investigation. However, these results warrant the investigation of the therapeutic potential of our co-encapsulated cells microbeads and compare them to other cell microbeads already described in the literature. The co-encapsulation of hepatocytes and mesenchymal stromal cells in alginate microbeads for the treatment of acute liver failure was already proposed by Shi et al. (42), using cells isolated from rats and an encapsulation protocol based on the coating of microbeads with poly-L-Lysine (PLL) and an additional outer layer of alginate, followed by depolymerization of the inner core, with the aim of improving cell-to-cell contact. Although the results were promising, we believe that our approach may offer more realistic clinical applications, as the presence of PLL, potentially immunogenic in vivo, is not ideal for the use of microbeads in patients. Also, the depolymerized core possibly allows proliferation of the cells, that, we think, may be dangerous as it could affect the stability of the microbeads. Furthermore, it has been described that PLL is a powerful inhibitor of miRNA biogenesis (43) with pronounced effects on hepatocyte function, such as alteration of the expression of miRNAs involved in the regulation of insulin sensitivity and cell proliferation (44). Meier et al. developed a protocol for the co-encapsulation of hepatocytes and MSC in hybrid poly(ethylene glycol)-alginate hydrogel microbeads, which, unlike poly-L-lysine-based hydrogels, have an excellent biological acceptance (45). However, cells are able to proliferate in these microbeads, facing the same risks described above for the microbeads with depolymerized center.
In conclusion, we have shown in this study that alginate microdisks, produced by internal gelation process, represent a possible first high throughput screening method to analyse and compare several conditions at the same time. Although the functions of cells encapsulated in alginate microdisks were overall lower than what observed in the microbeads, when this method was used to test the co-encapsulation of hepatocytes with mesenchymal stromal cells, it was able to detect improved functions compared to the microdisks containing only hepatocytes. These results were confirmed and further refined when using alginate microbeads. On the basis of the improved functions observed when MSC are co-encapsulated with hepatocytes, it seems that they could represent a valuable tool to improve the efficacy of hepatocyte alginate microbeads for the treatment of acute liver failure, although further experiments are required to fully support this statement.
Data Availability
The raw data supporting the conclusions of this manuscript will be made available by the authors, without undue reservation, to any qualified researcher.
Author Contributions
VI performed the experiments and wrote the first draft of the article. VI, CF, AD, and EF contributed to the conception and design of the study. FM contributed to alginate microdisks characterization. RM, CF, VI, CL, RF-D, and SW isolated primary hepatocytes. VI, RF-D, and SW isolated MSC. All the authors contributed to manuscript revision, read and approved the submitted version.
Funding
This study was funded by National Institute for Health Research (NIHR), Invention for Innovation (i4i), United Kingdom. The views expressed are those of the authors and not necessarily those of the NIHR. The authors acknowledge MowatLabs for financial support.
Conflict of Interest Statement
The authors declare that the research was conducted in the absence of any commercial or financial relationships that could be construed as a potential conflict of interest.
The reviewer VML and handling Editor declared their shared affiliation.
Acknowledgements
The authors acknowledge all the members—past and present—of Dhawan Lab who have contributed to the hepatocyte transplantation project.
References
1. Dhawan A, Mitry RR, Hughes RD. Hepatocyte transplantation for liver-based metabolic disorders. J Inherit Metabol Dis. (2006) 29:431–5. doi: 10.1007/s10545-006-0245-8
2. Dhawan A, Puppi J, Hughes RD, Mitry RR. Human hepatocyte transplantation: current experience and future challenges. Nat Rev Gastroenterol Hepatol. (2010) 7:288–98. doi: 10.1038/nrgastro.2010.44
3. Puppi J, Strom SC, Hughes RD, Bansal S, Castell JV, Dagher I, et al. Improving the techniques for human hepatocyte transplantation: report from a consensus meeting in London. Cell Transpl. (2012) 21:1–10. doi: 10.3727/096368911X566208
4. Iansante V, Mitry RR, Filippi C, Fitzpatrick E, Dhawan A. Human hepatocyte transplantation for liver disease: current status and future perspectives. Pediatric Res. (2018) 83:232–40. doi: 10.1038/pr.2017.284
5. Lee CA, Dhawan A, Iansante V, Lehec S, Khorsandi SE, Filippi C, et al. Cryopreserved neonatal hepatocytes may be a source for transplantation: evaluation of functionality toward clinical use. Liver Transpl. (2018) 24:394–406. doi: 10.1002/lt.25015
6. Bilir BM, Guinette D, Karrer F, Kumpe DA, Krysl J, Stephens J, et al. Hepatocyte transplantation in acute liver failure. Liver Transpl. (2000) 6:32–40. doi: 10.1002/lt.500060113
7. Khan AA, Shaik MV, Parveen N, Rajendraprasad A, Aleem MA, Habeeb MA, et al. Human fetal liver-derived stem cell transplantation as supportive modality in the management of end-stage decompensated liver cirrhosis. Cell Transpl. (2010) 19:409–18. doi: 10.3727/096368909X484707
8. Jitraruch S, Dhawan A, Hughes RD, Filippi C, Lehec SC, Glover L, et al. Cryopreservation of hepatocyte microbeads for clinical transplantation. Cell Transpl. (2017) 26:1341–54. doi: 10.1177/0963689717720050
9. Mitry RR, Jitraruch S, Iansante V, Dhawan A. Alginate encapsulation of human hepatocytes and assessment of microbeads. Methods Mol Biol. (2017) 273–81. doi: 10.1007/978-1-4939-6506-9_19
10. Jitraruch S, Dhawan A, Hughes RD, Filippi C, Soong D, Philippeos C, et al. Alginate microencapsulated hepatocytes optimised for transplantation in acute liver failure. PLoS ONE (2014) 9:e113609. doi: 10.1371/journal.pone.0113609
11. Uccelli A, Moretta L, Pistoia V. Mesenchymal stem cells in health and disease. Nat Rev Immunol. (2008) 726–36. doi: 10.1038/nri2395
12. Le Blanc K, Mougiakakos D. Multipotent mesenchymal stromal cells and the innate immune system. Nat Rev Immunol. (2012) 383–96. doi: 10.1038/nri3209
13. Le Blanc K, Davies LC. Mesenchymal stromal cells and the innate immune response. Immunol Lett. (2015) 168:140–6. doi: 10.1016/j.imlet.2015.05.004
14. Gómez-Aristizábal A, Davies JE. Human umbilical cord perivascular cells improve rat hepatocyte function ex vivo. Tissue Eng Part A (2012) 18:2487–96. doi: 10.1089/ten.TEA.2011.0669
15. Fitzpatrick E, Wu Y, Dhadda P, Hughes RD, Mitry RR, Qin H, et al. Co-culture with mesenchymal stem cells results in improved viability and function of human hepatocytes. Cell Transpl. (2015) 24:73–83. doi: 10.3727/096368913X674080
16. Alzebdeh DA, Matthew HW. Metabolic oscillations in co-cultures of hepatocytes and mesenchymal stem cells: effects of seeding arrangement and culture mixing. J Cell Biochem. (2017) 118:3003–15. doi: 10.1002/jcb.25962
17. Lee KY, Mooney DJ. Alginate: properties and biomedical applications. Prog Polymer Sci. (2012) 37:106–26. doi: 10.1016/j.progpolymsci.2011.06.003
18. Sun J, Tan H. Alginate-based biomaterials for regenerative medicine applications. Materials (2013) 1285–309. doi: 10.3390/ma6041285
19. Andersen T, Auk-Emblem P, Dornish M. 3D Cell culture in alginate hydrogels. Microarrays (2015) 4:133–61. doi: 10.3390/microarrays4020133
20. Paige KT, Cima LG, Yaremchuk MJ, Schloo BL, Vacanti JP, Vacanti CA. De novo cartilage generation using calcium alginate-chondrocyte constructs. Plastic Reconst Surg. (1996) 97:168–78. doi: 10.1097/00006534-199601000-00027
21. Kong HJ, Smith MK, Mooney DJ. Designing alginate hydrogels to maintain viability of immobilized cells. Biomaterials (2003) 24:4023–9. doi: 10.1016/S0142-9612(03)00295-3
22. Masuda K, Sah RL, Hejna MJ, Thonar EJMA. A novel two-step method for the formation of tissue-engineered cartilage by mature bovine chondrocytes: the alginate-recovered-chondrocyte (ARC) method. J Orthopaedic Res. (2003) 21:139–48. doi: 10.1016/S0736-0266(02)00109-2
23. Fonseca KB, Bidarra SJ, Oliveira MJ, Granja PL, Barrias CC. Molecularly designed alginate hydrogels susceptible to local proteolysis as three-dimensional cellular microenvironments. Acta Biomaterialia (2011) 7:1674–82. doi: 10.1016/j.actbio.2010.12.029
24. Ingar Draget K, Østgaard K, Smidsrød O. Homogeneous alginate gels: a technical approach. Carbohydr Polymers (1990) 14:159–178. doi: 10.1016/0144-8617(90)90028-Q
25. Kuo CK, Ma PX. Ionically crosslinked alginate hydrogels as scaffolds for tissue engineering: part 1. Structure, gelation rate and mechanical properties Biomaterials (2001) 22:511–21. doi: 10.1016/S0142-9612(00)00201-5
26. Andersen T, Melvik JE, Gåserød O, Alsberg E, Christensen BE. Ionically gelled alginate foams: physical properties controlled by type, amount and source of gelling ions. Carbohydr Polymers (2014) 99:249–56. doi: 10.1016/j.carbpol.2013.08.036
27. Fonseca KB, Gomes DB, Lee K, Santos SG, Sousa A, Silva EA, et al. Injectable MMP-sensitive alginate hydrogels as hMSC delivery systems. Biomacromolecules (2014) 15:380–90. doi: 10.1021/bm4016495
28. Maia FR, Fonseca KB, Rodrigues G, Granja PL, Barrias CC. Matrix-driven formation of mesenchymal stem cell-extracellular matrix microtissues on soft alginate hydrogels. Acta Biomater. (2014) 10:3197–208. doi: 10.1016/j.actbio.2014.02.049
29. Schmitt A, Rödel P, Anamur C, Seeliger C, Imhoff AB, Herbst E, et al. Calcium alginate gels as stem cell matrix -making paracrine stem cell activity available for enhanced healing after surgery. PLoS ONE (2015) 10:e0118937. doi: 10.1371/journal.pone.0118937
30. Mitry RR, Hughes RD, Aw MM, Terry C, Mieli-Vergani G, Girlanda R, et al. Human hepatocyte isolation and relationship of cell viability to early graft function. Cell Transplan. (2003) 12:69–74. doi: 10.3727/000000003783985197
31. Badraiq H, Cvoro A, Galleu A, Simon M, Miere C, Hobbs C, et al. Effects of maternal obesity on Whartons Jelly mesenchymal stromal cells. Sci Rep. (2017) 7:17595. doi: 10.1038/s41598-017-18034-1
32. Dominici M, Le Blanc K, Mueller I, Slaper-Cortenbach I, Marini FC, Krause DS, et al. Minimal criteria for defining multipotent mesenchymal stromal cells. The International Society for Cellular Therapy position statement. Cytotherapy (2006) 8:315–7. doi: 10.1080/14653240600855905
33. Iansante V, Choy PM, Fung SW, Liu Y, Chai JG, Dyson J, et al. PARP14 promotes the Warburg effect in hepatocellular carcinoma by inhibiting JNK1-dependent PKM2 phosphorylation and activation. Nat Commun. (2015) 6:7882. doi: 10.1038/ncomms8882
34. Dhawan A. Etiology and prognosis of acute liver failure in children. Liver Transpl. (2008) 14:S80–4. doi: 10.1002/lt.21641
35. Terry C, Dhawan A, Mitry RR, Lehec SC, Hughes RD. Optimization of the cryopreservation and thawing protocol for human hepatocytes for use in cell transplantation. Liver Transpl. (2010) 16:229–37. doi: 10.1002/lt.21983
36. Galateanu B, Dimonie D, Vasile E, Nae S, Cimpean A, Costache M. Layer-shaped alginate hydrogels enhance the biological performance of human adipose-derived stem cells. BMC Biotechnol. (2012) 12:35. doi: 10.1186/1472-6750-12-35
37. Oliveira SM, Barrias CC, Almeida IF, Costa PC, Pena Ferreira MR, Bahia MF, et al. Injectability of a bone filler system based on hydroxyapatite microspheres and a vehicle with in situ gel-forming ability. J Biomed Mater Res B Appl Biomater. (2008) 87:49–58. doi: 10.1002/jbm.b.31066
38. Bidarra SJ, Barrias CC, Fonseca KB, Barbosa MA, Soares RA, Granja PL. Injectable in situ crosslinkable RGD-modified alginate matrix for endothelial cells delivery. Biomaterials (2011) 32:7897–904. doi: 10.1016/j.biomaterials.2011.07.013
39. Bhogal RH, Curbishley SM, Weston CJ, Adams DH, Afford SC. Reactive oxygen species mediate human hepatocyte injury during hypoxia/reoxygenation. Liver Transpl. (2010) 16:1303–13. doi: 10.1002/lt.22157
40. Buravkova LB, Andreeva ER, Gogvadze V, Zhivotovsky B. Mesenchymal stem cells and hypoxia: where are we? Mitochondrion (2014) 19:105–12. doi: 10.1016/J.MITO.2014.07.005
41. Qin HH, Filippi C, Sun S, Lehec S, Dhawan A, Hughes RD. Hypoxic preconditioning potentiates the trophic effects of mesenchymal stem cells on co-cultured human primary hepatocytes. Stem Cell Res Ther. (2015) 6:237. doi: 10.1186/s13287-015-0218-7
42. Shi XL, Zhang Y, Gu JY, Ding YT. Coencapsulation of hepatocytes with bone marrow mesenchymal stem cells improves hepatocyte-specific functions. Transplantation (2009) 88:1178–85. doi: 10.1097/TP.0b013e3181bc288b
43. Watashi K, Yeung ML, Starost MF, Hosmane RS, Jeang KT. Identification of small molecules that suppress microRNA function and reverse tumorigenesis. J Biol Chem. (2010) 285:24707–16. doi: 10.1074/jbc.M109.062976
44. Lauschke VM, Vorrink SU, Moro SML, Rezayee F, Nordling Å, Hendriks DFG, et al. Massive rearrangements of cellular MicroRNA signatures are key drivers of hepatocyte dedifferentiation. Hepatology (2016) 64:1743–56. doi: 10.1002/hep.28780
Keywords: hepatocytes, mesenchymal stromal cells, alginate, hydrogel, microbeads, high throughput screening, acute liver failure, regenerative medicine
Citation: Iansante V, Dhawan A, Masmoudi F, Lee CA, Fernandez-Dacosta R, Walker S, Fitzpatrick E, Mitry RR and Filippi C (2018) A New High Throughput Screening Platform for Cell Encapsulation in Alginate Hydrogel Shows Improved Hepatocyte Functions by Mesenchymal Stromal Cells Co-encapsulation. Front. Med. 5:216. doi: 10.3389/fmed.2018.00216
Received: 27 April 2018; Accepted: 16 July 2018;
Published: 09 August 2018.
Edited by:
Roberto Gramignoli, Karolinska Institutet (KI), SwedenReviewed by:
Bruno Christ, Leipzig University, GermanyRonzitti Giuseppe, Genethon, France
Volker Martin Lauschke, Karolinska Institutet (KI), Sweden
Copyright © 2018 Iansante, Dhawan, Masmoudi, Lee, Fernandez-Dacosta, Walker, Fitzpatrick, Mitry and Filippi. This is an open-access article distributed under the terms of the Creative Commons Attribution License (CC BY). The use, distribution or reproduction in other forums is permitted, provided the original author(s) and the copyright owner(s) are credited and that the original publication in this journal is cited, in accordance with accepted academic practice. No use, distribution or reproduction is permitted which does not comply with these terms.
*Correspondence: Valeria Iansante, dmFsZXJpYS5pYW5zYW50ZUBrY2wuYWMudWs=
Anil Dhawan, YW5pbC5kaGF3YW5Aa2NsLmFjLnVr
Céline Filippi, Y2VsaW5lLmZpbGlwcGlAa2NsLmFjLnVr