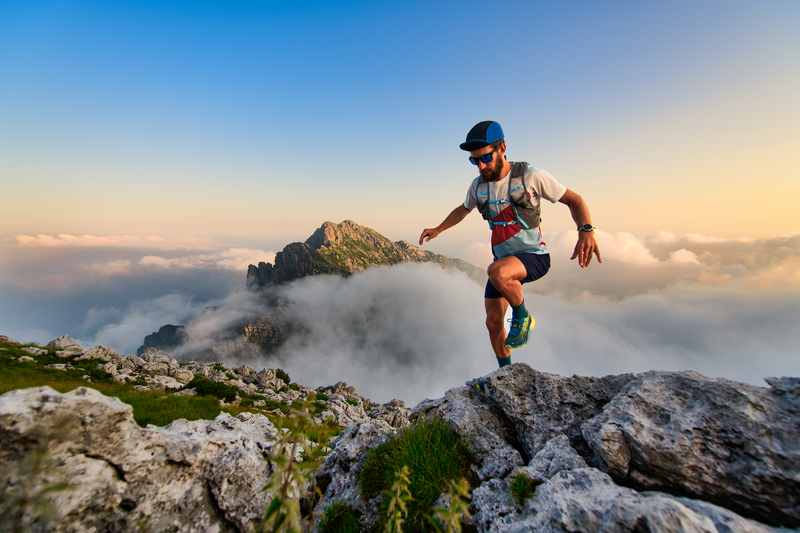
94% of researchers rate our articles as excellent or good
Learn more about the work of our research integrity team to safeguard the quality of each article we publish.
Find out more
ORIGINAL RESEARCH article
Front. Mech. Eng. , 19 August 2020
Sec. Engine and Automotive Engineering
Volume 6 - 2020 | https://doi.org/10.3389/fmech.2020.00067
This article is part of the Research Topic Advances in Compression Ignition Natural Gas – Diesel Dual Fuel Engines View all 8 articles
Studies in diesel dual fuel operation (DDF), introducing compressed natural gas (CNG) from the intake pipe and ignited by a liquid fuel injection in the combustion chamber have been conducted with conventional diesel engines. The present study investigated the effects of trade-off improvements between NOx and smoke emissions with next generation bio-alcohol blended FAME fuel ignition in DDF with a combination of EGR and supercharging. The CNG supply rates were set at 0% (diesel operation) and at 41–44% (DDF) on a heat energy basis, the boost pressures were set to two conditions with supercharger operation: 100 kPa (naturally aspirated operation, N/A) or 120 kPa (supercharged operation, S/C), and the EGR rates were varied from 0 to 25%. Blended fuels with a base fuel vs. alcohol ratio of 7: 3 were prepared using what in the following is termed PLME containing equal proportions of methyl palmitate (PME) and methyl laurate (LME) as the base fuel. The next generation bio-alcohols used here were iso-pentanol (C5) and iso-butanol (C4), and the engine performance, combustion characteristics, and exhaust emissions were investigated with iso-pentanol blended PLME (termed PLiP30), iso-butanol blended PLME (PLiB30), and neat PLME, as the ignition fuel. The results showed that with all ignition fuels, the DDF combining cooled EGR and supercharging improved the trade-off relation between NOx and smoke emissions significantly while maintaining relatively high brake thermal efficiencies.
From the viewpoints of CO2 emission reductions and energy security, natural gas could be a promising alternative fuel for internal combustion engines. A number of studies of diesel dual fuel operation (DDF) which introduce compressed natural gas (CNG) from the intake pipe and ignite it by a liquid fuel injected into the combustion chamber and have used conventional diesel engines. Dual fuel engines of this type require only limited modifications of presently used engines for cleaner operation with remarkable reductions in PM emissions and a relatively high thermal efficiency at high load conditions. Drawbacks include large amounts of unburned HC and CO emissions, specifically at low loads, which is a major factor to decrease thermal efficiency at partial loads. To improve partial load performance many studies with various approaches have been conducted (Mizushima et al., 2003; Ogawa et al., 2003; Aroonsrisopon et al., 2009; Wannatong et al., 2009; Abdelaal and Hegab, 2012; Taniguchi et al., 2012; Li et al., 2015; Yang et al., 2015; Guerry et al., 2016; Blasio et al., 2017).
From the viewpoint of environmental concerns, biodiesel as a diesel alternative fuel has been attracting attention, and some studies have been reported to apply biodiesel as an ignition fuel for the DDF mode. Paul et al. (2014) have conducted a study to apply pongamia pinnata methyl ester (PPME) as an ignition fuel to a diesel dual fuel engine in which CNG, as the primary fuel, is injected into the intake pipe. The experiments there were conducted in four modes: diesel mode using conventional diesel fuel and PPME, and Diesel-CNG and PPME-CNG dual fuel modes. The results showed that the DDF of the PPME-CNG has the best engine performance and emission characteristics of all the fuel combinations tested. In the PPME-CNG mode, the brake thermal efficiency increased, as well as the smoke opacity, CO emissions, and unburned hydrocarbon emissions significantly reduced, in total showing this as more effective in improving engine performance and emission characteristics than Diesel-CNG dual fuel mode. Çelebi et al. (2017) introduced CNG from an intake manifold and applied biodiesel-diesel blended fuels. The experiments were conducted with five kinds of ignition fuels: neat diesel, 20 and 40% by volume of canola oil biodiesel and sunflower oil biodiesel blended with diesel. In the Çelebi et al. investigation, the CNG flow rates were changed in three stages (5, 10, and 15 L/min), and the sound pressure level (SPL), vibration acceleration characteristics, and emission characteristics (CO, NOx, and CO2) were investigated at five different engine speeds (1,200–2,400 rpm). The results showed that compared with conventional diesel fuel, the biodiesel blends improved SPL, vibration acceleration, and CO emissions over those of ordinary diesel operation. The DDF with natural gas supplied to the intake manifold reduced vibration acceleration and SPL values, while CO emissions increased significantly. Also, the study reports that the biodiesel blended fuels increased NOx and CO2, while the NOx and CO2 emissions were improved by the CNG supply. The dual fuel engine offers reliable ignition by the diesel spray injected in the CNG-air pre-mixture, and it is possible to use low calorie gas fuels like pyrolysis gas from wood- and waste-biomass (Roy et al., 2009; Bora et al., 2014; Aklouche et al., 2017; Verma et al., 2017). The above features of DDF engines would suit cogeneration systems well, but substantial reductions in NOx emissions will be needed for this to be adopted.
It is well-known that a combined technique of EGR with supercharging is an effective measure to obtain simultaneous reductions in NOx and PM emissions. For that, the basic concept is that cooled EGR reduces NOx emissions due to lowered local oxygen concentrations and flame temperatures, while supercharging would be able to compensate for the output loss caused by increases in the EGR rates. There are some reports on the characteristics of DDF with gas fuels combined with EGR and supercharging. For instance, the combustion characteristics in DDF using high degrees of freedom in the injection variables with a common rail fuel injection system have investigated (Tomita et al., 2009; Azimov et al., 2011; Ogawa et al., 2015, 2016; Aksu et al., 2016). Iorio et al. (2017) examined the brake specific fuel consumption, combustion characteristics, and NOx emission characteristics using a compression ignition engine with three cylinders equipped with an electronically controlled methane injection system at the intake pipe. They also conducted optical measurements with an endoscope. Although neither EGR nor supercharging techniques were employed in that study, the analysis of the flame images by a two color method showed that compared with ordinary diesel combustion the DDF has lower flame temperatures and less soot combustion. The study concluded that this phenomenon is a reason why the DDF leads to low NOx and low PM emissions.
A few studies have reported investigations with a jerk type fuel injection system: Selim (2003) examined the influence of EGR rates (0–15%) and boost pressures (1–1.4 atm) on combustion noise reductions with a swirl chamber IDI engine with CNG-diesel dual fuel operation (that study used pure methane as a CNG substitute). The results showed remarkable reductions in the rate of maximum pressure rise when combining EGR and supercharging, but the influence on the engine performance and emission characteristics are unclear. A literature survey by the present authors showed only one other similar study, reported by Park and Terao (2003). That study examined the effects of combining supercharging and hot or cooled EGR with a cogeneration system by biogas. As shown here, the improvements with the DDF engine with conventional injection systems are limited.
The present authors have studied diesel dual fuel operation (DDF) with a small single cylinder DI diesel engine equipped with a jerk type injection system using CNG as the gaseous fuel. These reports used biodiesels or fatty acid methyl esters (FAMEs) as the ignition fuel (Kinoshita et al., 2009; Yoshimoto, 2010; Yoshimoto et al., 2010, 2018a), and reported the influence of the cetane number of the ignition fuel (Yoshimoto and Kinoshita, 2011; Yoshimoto et al., 2012), the effects of the intake air dilution by CO2 or N2 (Yoshimoto and Kinoshita, 2013), and the effects of boost pressures (Yoshimoto et al., 2016, 2017). One study (Yoshimoto et al., 2017) used 60% mass coconut oil methyl ester (CME) and 40% n-butanol blends (termed CMEB) as the ignition fuel in a DDF engine, and the influence of the boost pressure was examined. The results showed that with supercharging at 120 or 130 kPa boost pressures, the ignition delays of the CMEB shortened substantially, resulting in a brake thermal efficiency similar to diesel operation with conventional diesel fuel. In a previous study (Yoshimoto et al., 2018b), four kinds of next generation bio-alcohol isomer and diesel fuel blends were used in diesel operation and the effects of combining supercharging and EGR were examined. The bio-alcohol isomers were n-butanol, iso-butanol, n-pentanol, and iso-pentanol. Both with and without supercharging, all the bio-alcohol isomer blended fuels offer substantial improvements in the NOx and smoke emissions trade-off while maintaining good brake thermal efficiency. In a following study (Yoshimoto et al., 2019), two iso-pentanol blended fuels at a constant 3: 7 mass ratio were used with LME (methyl laurate) and with diesel fuel as the base fuel, and here the DDF was conducted with four ignition fuels: neat LME, neat diesel, iso-pentanol blended LME, and iso-pentanol blended diesel. The results showed that all of the bio-alcohol blended fuels improved the NOx and smoke emission trade-off substantially, while the brake thermal efficiency decreased significantly with increasing EGR rates even when supercharging was employed. The reason was considered to be that the degree of constant volume of combustion decreased due to a large increase in the ignition delay of the alcohol blended fuels, resulting in the brake thermal efficiency deterioration.
In the present study, iso-pentanol and iso-butanol were used as the next generation bio-alcohols because the Research and Development of the yield improvement with advanced high fermentation methods have been progressing (Peralta-Yahya and Keasling, 2010; Zheng et al., 2015). Using an equal proportion of PME (methyl palmitate) that has a higher cetane number than LME, and LME, as the base fuel, two bio-alcohol blended fuels at a constant mass ratio of 7: 3 were prepared and supplied as the ignition fuel for the DDF. It was found that the DDF with the two bio-alcohol blends, with ignition combining cooled EGR and supercharging improved the NOx and smoke emission trade-off relation substantially while maintaining relatively high values in the brake thermal efficiency. Recently, a number of studies with next generation bio-alcohol blended fuels have been carried out targeting diesel operation (Han et al., 2016; Ileri, 2016; Kumar and Saravanan, 2016; Imdadul et al., 2017; Saravanan et al., 2017; Anil Bhaurao et al., 2018; Killol et al., 2019; Pan et al., 2019). But, many of these studies have been conducted with n-butanol, iso-butanol, or n-pentanol, and there are few reports with iso-pentanol (Yoshimoto et al., 2018b).
The study used CNG (compressed natural gas) as the premixed with intake gas. Table 1 shows the particulars of the used gas. To ignite the pre-mixture, the experiments used two kinds of next generation bio-alcohol blended fuels and neat PLME. Here, the PLME stands for a blend with equal proportions of PME (methyl palmitate, purity > 95%) and LME (methyl laurate, purity > 96%). Using a PLME as the base fuel, two alcohol blends with a constant mass ratio of 7: 3 were prepared by iso-pentanol (termed PLiP30) and iso-butanol (PLiB30). In a previous paper, as a result of using 30% iso-pentanol blended LME fuel (LiP30) as the ignition fuel for the DDF, the ignition delay became very long and the brake thermal efficiency decreased significantly at a high EGR rate condition (Yoshimoto et al., 2019). One way to prevent such a deterioration of engine performance is to use PME with the high cetane number as the base fuel. However, as PME has a high melting point of about 35°C, it cannot be applied to the engine without heating the fuel. Therefore, the present study employed an equal proportion of PME and LME as a base fuel that can be handled at room temperatures while improving ignitability. The authors have conducted studies with butanol (including isomers) or pentanol blended fuels under diesel operation. For the alcohol blending, the base fuels such as diesel fuel, biodiesels, or FAME were used. Among these, for butanol, we investigated the influence of the isomer kind and blending ratio in detail (Fushimi et al., 2013; Yoshimoto et al., 2013, 2018b). Considering these results, we conducted the above experiments (Yoshimoto et al., 2019) with 30% iso-pentanol blended LME (LiP30) or 30% iso-pentanol blended diesel fuel (GiP30) in the DDF mode. As a result of the DDF with the LiP30 fuel, a deterioration in ignitability occurred as described above. In this study, the experiments were designed with PLME, which has a better ignitability than the LME as the base fuel, while iso-butanol as the blending component having a low cetane number is applied to the DDF. Considering this-, we decided that 30% iso-butanol is a suitable ratio for blending to PLME.
Table 2 shows the tested ignition fuels including iso-pentanol and iso-butanol, the blended components. The PME and LME have sixteen and twelve saturated carbon atoms and their cetane numbers are higher than ordinary diesel fuel, the ignitability of the PLME is good. The cetane number of iso-pentanol is unknown, but from the ignition delay characteristics examined by the authors, the cetane number of the iso-pentanol was estimated to be between 17 and 20 (Yoshimoto et al., 2018b). Here, the cetane number of the n-butanol is 17, and that of n-pentanol 20 (Kumar and Saravanan, 2015). As the PLiP30 and PLiB30 contain alcohols with a low boiling point, the 10% distillation temperatures are low and the evaporation characteristics are better than those of PLME.
Figure 1 shows the experimental apparatus: a test engine, a CNG supply unit, a supercharging system, and an EGR cooling device. The tested engine is a horizontal, four stroke, water-cooled small single cylinder direct injection diesel engine with a toroidal type combustion chamber and the specifications of the engine and the fuel injection system are shown in Table 3. The jerk type fuel injection system was set to standard diesel fuel specifications except for changing the plunger diameter to 7 mm from the original size of 6.5 mm. This is to increase the injected fuel quantity to maintain the specified engine output for the tested bio-fuels that have low heat values. The tested engine has a mechanical governor to maintain a constant engine speed and an eddy current type dynamometer was used to absorb the engine output.
As shown in Figure 1, the CNG supply unit has a CNG bottle, a pressure regulator, a mass flow controller, and a check valve. The CNG is fed directly into the intake-pipe, normal to the intake charge flow. The study employed a Roots type blower supercharger driven by a motor, making it possible to control the boost pressure independently from the engine operating variables. The boost pressures were 100 kPa (naturally aspirated operation, N/A here) and 120 kPa (supercharged operation, S/C). The back pressure in the exhaust pipe was set at a constant 110 kPa during the supercharged operation. The diesel dual fuel operation was carried out with the following procedure: the warm-up operation with the tested fuel was performed under ordinary diesel mode, next the CNG flow rate was increased while reducing the injected fuel quantity. This operation was carried out step by step, and finally the CNG supply rate was set at a constant 8 L/min (0°C, 1 atm) in all the experiments. During the measurements, the engine torque and speed were kept constant by finely adjusting the fuel injection quantity and the field current of the eddy current type dynamometer.
The EGR rate was adjusted by an EGR valve upstream of a diesel particulate filter (DPF). The experiments employed low-pressure loop type cooled EGR and the recirculated gas was introduced to the intake air upstream of the supercharger. The EGR rate was determined from Equation (1) and the CO2 concentration in the exhaust and intake gases was measured using an NDIR analyzer. Since it is necessary to estimate the EGR rate quickly during the experiments, the opening of the EGR valve was adjusted so the oxygen concentration reached the target value while monitoring the oxygen concentration of the intake air corresponded to the EGR rate. The oxygen concentration was monitored by a magnetic force type oxygen analyzer.
The intake air quantity was measured with a laminar flowmeter upstream of the supercharger. The gas temperatures in the intake pipe were controlled at 30 ± 2°C. During the experiments, the engine speed was a constant 1,900 rpm, the maximum brake torque conditions, 87 ± 2°C cooling water, and 70 ± 3°C lubricating oil. The engine load was set at a constant high load condition (BMEP = 0.67 MPa) where the trade-off relation between NOx and smoke emissions assumes critical values. The brake thermal efficiency, BTE was determined from Equation (2). Where, Ne is the braking power, ṁf is the mass flow rate of ignition fuel, ṁg is the mass flow rate of gaseous fuel, while LHVf and LHVg are the lower heating values of the ignition fuel and gaseous fuel, respectively. The flow rate of the ignition fuel was determined by time interval measurement per specified volume, and its mass flow rate was calculated from the density.
The combustion pressure was measured with a piezoelectric type pressure pick-up and the crank angle was recorded by a rotary encoder; the needle lift of the nozzle was monitored by a Hall-effect element. The signals recorded digitally in about 160 continuous cycles, and the rate of heat release and the degree of constant volume of combustion, ηglh determined from the average pressure of 50 cycles. The energy equilibrium of the gas in the cylinder as in Equation (3) and the degree of constant volume of combustion, ηglh, determined from Equation (4):
From any 50 continuous cycles, the ignition delay was determined from the crank angle interval between the start of the needle lift and the pressure rise due to the combustion. The detection of the rise timings of the needle lift and pressure signal necessary for the ignition delay analysis was carried out by using a method that automatically discriminates by programming based on the set threshold value for any 50 combustion cycles. The NOx emissions were measured using a CLD analyzer, the HC as ppm methane using a FID analyzer, the CO used an NDIR analyzer, and the smoke density an opacimeter.
With the dual fuel operation, the proportion of CNG supplied, Qg/Qt, was defined as the rate of the heat energy of the supplied CNG, Qg, to the total heat energy available in the cylinder, Qt. In a previous study (Yoshimoto et al., 2016), the experiments were carried out up to a 140 kPa boost pressure with 0–81% CNG supply rates using JIS No. 2 diesel fuel. Here, the HC and CO emissions increased substantially with increasing boost pressure and CNG supply: the optimum operating conditions were determined to be below 120 kPa boost pressures and below 42% CNG supply rates. In the present study, the DDF with supercharging was conducted at a constant 120 kPa boost pressure and the CNG flow rate was set at a constant 8 L/min (0°C, 1 atm) with and without supercharging. The equivalence ratio of the pre-mixture, ϕg, was 0.18–0.24 at the supercharged operation (S/C) and ϕg = 0.26–0.30 at a naturally aspirated condition (N/A). The proportion of CNG, Qg/Qt was 41–44%. The operating conditions are listed in Table 4.
Figure 2 shows the changes in brake thermal efficiency, ηe, smoke density, equivalence ratio of the total in-cylinder charge, ϕt, and exhaust temperature as a function of the EGR rate at two intake charge pressures, 100 and 120 kPa with the Roots type blower supercharger operating. Figure 3 shows the changes in emission characteristics. The left columns (Figures 2, 3) show the diesel operation (Figures 2A, 3A, Qg/Qt = 0%) and the right columns are the diesel dual fuel operation, DDF (Figures 2B, 3B, Qg/Qt = 41–44%), respectively. As the injection fuel (or ignition fuel), the experiments here used JIS No. 2 diesel fuel (gas oil) as a reference, neat PLME (equal proportions of methyl palmitate and methyl laurate), PLiP30 (70% PLME and 30% iso-pentanol blend), and PLiB30 (70% PLME and 30% iso-butanol blend); the supercharger power input was not considered in the thermal efficiency calculations. The upper limit of EGR rate was set to about 11% at a naturally aspirated condition as well as 25% at supercharged operation, respectively, because of the substantial increase in the smoke emissions under diesel operation with diesel fuel.
Figure 2. Plots of results with EGR and supercharging vs. brake thermal efficiency, smoke density, equivalence ratio of the total in-cylinder charge, and exhaust gas temperature with diesel fuel, PLME, PLiP30, and PLiB30 with diesel and DDF (BMEP=0.67 MPa). (A) Diesel operation and (B) DDF.
Figure 3. Plots with EGR and supercharging vs. emission characteristics with diesel fuel, PLME, PLiP30, and PLiB30 with diesel and DDF (BMEP = 0.67 MPa). (A) Diesel operation and (B) DDF.
As shown in the top panel of Figure 2A, the brake thermal efficiency ηe with diesel fuel without supercharging (N/A) with diesel operation decreased remarkably with increasing EGR rates. Here, the smoke emissions with the diesel fuel increased substantially (second panel of Figure 2A), and operation with high EGR rates could not be maintained. As shown in Figure 2B, the DDF without supercharging maintained extremely low smoke densities, and the ηe were maintained at relatively high values. As shown here (Figure 2B), this type of DDF engine introducing CNG from the intake pipe strongly suppresses smoke emissions in N/A operation employing EGR. The reason is that as the engine is operated at a constant output, the injected fuel quantity with the DDF decreases and this may cause the soot formation in the fuel rich zone to be suppressed, and become a major reason for the smoke reductions (Kinoshita et al., 2009).
Next, a discussion will be made of the influence of the presence or absence of supercharging on the brake thermal efficiency ηe focusing especially on the ordinary and DDF modes. As shown in the top panel of Figure 2A, the ηe with all the injection fuels under diesel operation with supercharging increased considerably over the tested EGR region, compared to the N/A operation. Here, the trend in the ηe increase is specifically significant with the neat PLME. The relation among the brake thermal efficiency ηe, the theoretical thermal efficiency ηth, the degree of constant volume of combustion ηglh, the combustion efficiency ηu, the cooling loss ϕw, and the mechanical efficiency ηm is:
The experimental variables set in this study such as with and without supercharging, EGR rates, and the two operation modes affect the ratio of specific heats of the working fluid. Therefore, the theoretical thermal efficiency, ηth of the fuel-air cycle shown in Equation (5) may have been complicatedly influenced by these experimental variables. However, simulation analysis is necessary to clarify the effect of the ratio of specific heats, which is outside the scope of this study. In this report discussion is limited to influencing factors that can be examined with the experimental results.
When supercharging is employed, the equivalence ratio of the total in-cylinder charge ϕt, decreases (third panel of Figure 2A). This is because the air density in the combustion chamber increases giving rise to the tendency toward reductions in local oxygen concentration shortages, resulting in the reductions in smoke emissions (solid curves, second panel of Figure 2A) and CO emissions (bottom panel of Figure 3A). This is the reason why the combustion efficiency ηu in Equation (5) improves, and may be a reason for the increases in the brake thermal efficiencies with supercharging. As shown in the top panel of Figure 2B, the ηe with the supercharged DDF was similar to that of the diesel operation using diesel fuel without EGR and there were also no improvements in the DDF without EGR. The reason may be that, as shown in the middle panel of Figure 3B, the HC emissions with DDF increased substantially even when the engine was operated without supercharging, resulting in the decrease in the combustion efficiency ηu.
Discussing the influence of the EGR rate on the brake thermal efficiency ηe, both modes of operation with supercharging showed that the ηe clearly decreased above the 20% EGR region. At the diesel operation, the smoke emissions (Figure 2A) and CO emissions (Figure 3A) increased substantially here. This is because the equivalence ratio of the total in-cylinder charge increases due to intake air quantity decreases by the EGR (third panel of Figure 2A). Therefore, it may be considered that the decreases in combustion efficiency ηu resulted in the decreases in ηe in the diesel operation with supercharging at high EGR rates. As shown in the bottom panels of Figure 2, all the tested fuels showed substantial reductions in exhaust gas temperature with the supercharging, regardless of operation mode. The reason for this is considered to be that the heat capacity of the in-cylinder charge increases due to the increasing air density brought about by the supercharging, resulting in a decrease in the mean gas temperature during combustion.
Considering the influence of injection or ignition fuel kind on the brake thermal efficiency ηe, the neat PLME without supercharging under diesel operation showed high values of ηe, compared with diesel fuel. This can be explained by the differences in smoke (second panel of Figure 2A) and CO emissions (bottom panel of Figure 3A). The PLME is an oxygenated fuel (oxygen content 13.4%) and the oxygen contained in the fuel would promote the oxidation reaction in the fuel-air mixture, resulting in decreases in smoke and CO emissions, increasing the combustion efficiency ηu. With supercharging under diesel operation, the ηe with the neat PLME also tends to be higher than the other fuels. In the DDF mode, regardless of supercharging, there is little effect of the fuel kind on the ηe, and a relatively high brake thermal efficiency was maintained in the tested EGR regions. As shown in the second panel of Figure 2A, the smoke emissions with the bio-alcohol blended PLME fuels compared to those of the neat PLME under supercharged operation decreased at high EGR rate conditions. It is clear that PLiP30 and PLiB30 with 14.8 and 15.9% oxygen content generates lower smoke emissions than the PLME which has 13.4% oxygen.
Figure 3 shows the changes in NOx, HC, and CO emissions as a function of the EGR rate. Regardless of operation mode, the NOx emissions decreased monotonously with increasing EGR and it is clear that EGR is an effective measure to achieve NOx reductions. The NOx reductions showed no clear trends by the kind of ignition fuel, with or without supercharging, and in all operation modes, except for the condition with diesel fuel under diesel operation with supercharging without EGR.
As shown in the middle panel of Figure 3B, the HC emissions with the DDF increased substantially at the N/A condition and this tendency became very strong at the S/C operation. It has been pointed out that the main source of HC (methane) emissions for diesel engines converted to dual fuel operation with CNG is the blow-through of CNG during the valve overlap period. The following two factors are considered as other reasons. With diesel dual fuel operation at the N/A condition and due to the CNG supply, unburned mixture easily enters the quench regions, giving rise to HC emission increases (Kinoshita et al., 2009). A further reason may be that there are parts of the CNG-air mixture that are not entrained into the spray flux of the ignition fuel. As the CNG-air pre-mixture is outside the flammable range, flames do not propagate here and this mixture may remain without taking part in the combustion, such a situation would also result in higher HC emissions (Yoshimoto et al., 2012). With supercharging, the CNG-air pre-mixture became very lean and flame propagating combustion deteriorated more, and it may be that such incomplete combustion, an expansion of the unburned regions, leads to substantial increases in HC emissions. The HC emissions with the DDF with supercharging showed a weakly decreasing trend with increasing EGR. The reason is not clear, but Srinivasan et al. (2007) have suggested the possibility of an active recycling effect, unburned hydrocarbons in the recirculated gas influences the re-burning of the hydrocarbons during the next combustion cycle.
Regardless of ignition fuel, the CO emissions increased substantially with increasing EGR at the N/A operation under the ordinary diesel mode (bottom panel in Figure 3A). The reason may be that the oxygen concentration of the in-cylinder charge decreases due to EGR. The addition of supercharging did however improve the CO emissions remarkably. Compared with the diesel operation, the CO emissions with the DDF showed high values across the tested EGR regions. The reasons would be similar to those causing HC emissions: the CNG-air pre-mixture became very lean and the incomplete combustion regions expanded. A further reason may be that the pre-mixture became much leaner due to longer ignition delays, as will be described in the next section.
Figure 4 shows the changes in indicator diagrams and heat release rates with and without EGR at the supercharged condition under two operation modes: the top panels are for diesel operation (Figures 4A,B, Qg/Qt = 0%) and the bottom panels are for DDF mode (Figures 4C,D, Qg/Qt = 41–44%). Plots in the four panels in Figures 4A,B vs. Figures 4C,D show that regardless of the addition of EGR, the ignition timings with the DDF operation were delayed more than in the ordinary diesel operation. This delay is especially remarkable when bio-alcohol blended PLME fuels were used as the ignition fuel.
Figure 4. Plots of results with EGR and supercharging vs. indicator diagrams and heat release rates with diesel fuel, PLME, PLiP30, and PLiB30 with diesel and DDF (BMEP = 0.67 MPa). (A,B) Diesel operation and (C,D) DDF.
Figure 5 plots combustion characteristics for all of the data of the present experiments. Here, it is noted that the bulk modulus of the fuel affects the timing of the start of fuel injection, and consequently the ignition characteristics. Especially with biodiesel, it has been reported that the bulk modulus varies depending on the kind of FAME (Tat and Van Gerpen, 2003). In ordinary diesel operation, the dynamic timings of the start of injections for the tested fuels were in the range of −5.0 to −4.6 °CA.ATDC. Compared with diesel fuel, the PLME showed a tendency for the start of injection to advance in the range of 0.1 to 0.3 °CA. However, no clear difference between alcohol blended PLME fuels and diesel fuel have been observed. It is also an important issue to clarify the fluctuation in the combustion characteristics shown here. The signals (data, values) of the in-cylinder pressure, nozzle needle lift, and crank angle were recorded for 160 continuous cycles. From many experiments with diesel fuel, biodiesels, and FAME fuels, it has been confirmed that when the CNG supply rate is below 77% the combustion fluctuation rate, COVimep (defined as the ratio of standard deviation in imep to the mean value of the imep) is low, and stable combustion is obtained. In the present experiments, the COVimep was below 3.5% under all conditions, so the cycle to cycle variation may be considered to be small. The fluctuation rate of the ignition delay, COVdelay was as high as about 10% only under the conditions of the DDF mode using PLiB30, N/A, and the EGR rate of 10.4%. However, the COVdelay was lower than 6% under all other conditions.
Figure 5. Plots of EGR and supercharging vs. combustion characteristics with diesel fuel, PLME, PLiP30, and PLiB30 with diesel and DDF (BMEP = 0.67 MPa). (A) Diesel operation and (B) DDF.
Comparing the top panels of Figures 5A,B, the ignition delays with the DDF are longer than those with diesel operation. A reason may be, as reported by Papagiannakis et al. (2008) that the cylinder charge temperature close to the point of the liquid fuel injection is lower with DDF due to the higher overall specific heat capacity of the CNG-air mixture. The ignition delays with supercharging were short and this trend was enhanced with the DDF mode, suggesting that applying supercharging in the DDF is an effective measure to shorten the ignition delay. The major reason why supercharging shortens the ignition delay is considered to be an effect of the in-cylinder pressure increases.
At the N/A condition, the ignition delay with the DDFD is reported as longer than with the diesel operation, and the premixed combustion after the ignition is enhanced (Yoshimoto et al., 2010). In the premixed combustion phase, the injected fuel spray in the cylinder forms a combustible mixture during the ignition delay, and combustion of this mixture occurs simultaneously with the ignition fuel in the regions where the CNG-air pre-mixture is entrained by the spray. This increases the maximum rate of heat release (dQ/dθ)max, resulting in the increases in the heat release in the premixed combustion phase shown in the middle panel of Figure 5B. It may be concluded that following the premixed combustion, both the entrained CNG from the ignition fuel spray and the previously injected fuel spray burn simultaneously. At all of the operation modes and with the addition of supercharging, the ignition delays with neat PLME were shorter than with other fuels for all the tested EGR rates. This may be ascribed to the cetane number of the PLME being higher than that of the diesel fuel, as shown in Table 2. As shown in Figures 5A,B, the ignition delays with the bio-alcohol blended PLME fuels (PLiP30 and PLiB30) increased substantially as well as the (dQ/dθ)max increased over the tested EGR range, compared with PLME. With the diesel operation, the degree of constant volume combustion, ηglh with the bio-alcohol blended PLME fuels are similar to those of the other injection fuels. This would suggest that after the ignition, the bio-alcohol blended PLME fuels the combustion proceeds at higher speeds. The reason may be considered to be that the mixture formation in the diffusion combustion phase is improved due to improvements in the evaporation characteristics and spray characteristics because of the low boiling point and lower kinematic viscosity of the PLiP30 and PLiB30 than that of diesel fuel. In the DDF, however, the ignition delays with the bio-alcohol blended PLME fuels became much longer, and the ηglh at the increased EGR conditions decreased substantially (bottom panel in Figure 5B). It is considered that such a delay in the combustion with the PLiP30 and PLiB30 resulted in the higher exhaust gas temperatures shown in the bottom panel of Figure 2B. The reason for the ignition delay increases in the bio-alcohol blended PLME fuels is due to the low cetane numbers of the bio-alcohols contained in the blends.
Figure 6 shows the trade-off relation between NOx and brake thermal efficiency, ηe as well as between NOx and smoke emissions with the tested fuels as a function of the EGR rate, and with and without supercharging under different operation modes. As shown in Figure 6A, the NOx emissions with diesel fuel under diesel operation decreased significantly when the cooled EGR was employed at the N/A condition (boost pressure: 100 kPa). But, the EGR rates could not be increased because of the substantial increases in the smoke emissions with diesel fuel. When supercharging was employed (boost pressure: 120 kPa), the decrease in the ηe is suppressed as well as the trade-off relation between the NOx and smoke emissions improved, but the smoke emissions with PLiB30 that has the highest oxygen content reached 15% at a 25% EGR rate (red arrow in the bottom panel in Figure 6A). In the DDF mode with the supercharging as shown in Figure 6B, all the ignition fuels achieved almost smokeless combustion over the tested EGR regions and the ηe maintained relatively high values in these regions. The base line performance in the present study, under diesel operation with diesel fuel at the N/A condition without EGR, was that the brake thermal efficiency was 34.9%, smoke emissions 14.7%, and NOx emissions 4.8 g/kWh (dark blue arrows in Figure 6A). The DDF operation with bio-alcohol blended PLME fuels with supercharging with 22–23% EGR conditions showed that although the brake thermal efficiency decreased around 3%, very low NOx emissions of 0.5–0.6 g/kWh and almost smokeless combustion was realized (orange arrows in Figure 6B).
Figure 6. Plots of trade-off relation results for NOx vs. brake thermal efficiency and NOx vs. smoke emissions with diesel fuel, PLME, PLiP30, and PLiB30 with diesel and DDF (BMEP = 0.67 MPa). (A) Diesel operation and (B) DDF.
Figure 7 shows the relationship between brake thermal efficiency, ηe and degree of constant volume of combustion, ηglh in the DDF as a function of the ignition delay with the parameters fuel kind and with and without supercharging. Figure 7A shows the case with LME based blends [results in a previous study (Yoshimoto et al., 2019)], and Figure 7B shows the PLME based blends (present study). The CNG flow rate was at an 8 L/min (0°C, 1 atm), and the blending ratio of bio-alcohol to base fuel was at 3: 7 (mass) in both experiments. Also, both experiments were conducted within a similar EGR range. Specifically, iso-pentanol blended LME experiments were carried out within 0 to 11.0% EGR under N/A conditions, 0 to 22.4% EGR in S/C conditions, while the EGR rates were 0 to 12.6% at N/A and 0 to 25.3% at S/C for the PLME based blends. As shown in Figures 7A,B, it is clear that the constant volume of combustion ηglh decreases as the ignition delay increases, resulting in a decrease in brake thermal efficiency. As the EGR rate increased, ignition delays with the iso-pentanol blended LME fuels (LiP30, Figure 7A) became substantially longer than those of iso-pentanol blended PLMEs (PLiP30, Figure 7B) or iso-butanol blended PLMEs (PLiB30, Figure 7B). The LiP30 which consists of a low cetane number LME than PLME, its ignition delay increased remarkably at a high EGR rate condition with supercharging. It is considered that this caused a significant decrease in the ηglh, resulting in substantial decrease in the brake thermal efficiency. From Figure 7, it is also clear that when the ignition delay exceeds 15 °CA, the degree of constant volume of combustion decreases and brake thermal efficiency decreases substantially. Therefore, it can be concluded that using PLME which is a base fuel with a high cetane number the present experiments prevented the deterioration in ignitability due to alcohol blending, resulted in the suppression of very much longer ignition delays, and the relatively higher brake thermal efficiency was obtained.
Figure 7. Plots of ignition delay vs. brake thermal efficiency and degree of constant volume of combustion with LME based blends and PLME based blends with DDF (BMEP=0.67 MPa). (A) LME based blends and (B) PLME based blends.
A diesel dual fuel engine with compressed natural gas (CNG) introduced from the intake pipe was operated by combining cooled EGR and supercharging with ignition fuels with next generation bio-alcohol blended FAME fuels. The engine used is a small single cylinder direct injection diesel engine equipped with a jerk type injection system. The ignition fuels used here were neat PLME (equal proportions of methyl palmitate and methyl laurate), blended fuel with 70% mass PLME and 30% iso-pentanol (termed PLiP30), and 70% PLME and 30% iso-butanol blend (PLiB30). The CNG supply rates defined on an energy basis were set at two conditions of 0% (diesel operation) and 41–44% (DDF). Two intake charge pressures: 100 kPa (naturally aspirated operation, N/A) and 120 kPa (supercharged operation, S/C) were employed and the EGR rates were varied from 0 to 25%. The influence of the ignition fuel on engine performance, combustion characteristics, and emissions were examined and compared for the two operation modes, DDF and diesel operation, combining supercharging and EGR. The results of the present study may be summarized as follows:
1. Diesel operation at the naturally aspirated condition with EGR increased the smoke emissions, and the EGR rates could not be increased above 13%, even with PLME which has a 13.4% oxygen content. When the supercharging was employed under the ordinary diesel mode, all the injection fuels showed increases in brake thermal efficiency, but increasing EGR rates caused increased smoke emissions.
2. Regardless of ignition fuel kind and with and without supercharging, the DDF improved the trade-off relation between NOx and smoke emissions while at high brake thermal efficiencies could be maintained over the whole of the tested EGR regions.
3. Considering trade-off improvements, the optimum conditions obtained with the tested bio-alcohol blends were as follows: under diesel operation with S/C at 23–25% EGR showed a brake thermal efficiency of 35.1%, smoke densities of 15–16%, and NOx emissions of 0.53–0.60 g/kWh. Similarly, under the DDF with S/C at 22–23% EGR showed a brake thermal efficiency of 33.7–34.0%, smoke densities of 1.2–1.5%, and NOx emissions of 0.53–0.59 g/kWh.
4. Comparing the results obtained with the next generation bio-alcohol blends under DDF with S/C at 22–23% EGR conditions to baseline performance (ordinary diesel mode, diesel fuel, N/A, without EGR), the brake thermal efficiency decreased 2.6–3.4%, while reduction rates in NOx and smoke emissions were 88–89% and 90–92%, respectively.
5. It was found that the cetane number of the base fuel used for alcohol blending has a strong influence on the engine performance in the DDF mode. Using PLME which is a base fuel with a high cetane number prevented the deterioration in ignitability due to alcohol blending, resulting in the relatively higher brake thermal efficiency that was realized.
In this study, diesel dual fuel operation was investigated supplying CNG to the intake pipe with next generation bio-alcohol blended FAME fuels as the ignition fuel. As a result of the combination of EGR and supercharging, it was found that NOx and smoke emissions could be significantly reduced while suppressing the deterioration of the brake thermal efficiency. However, a large amount of HC was discharged in the dual fuel operation in this experiment, and most of this is methane with a high GHG effect. This is a major drawback, and its solution remains as a problem to be solved. To suppress unburned CNG emissions, measures such as CNG direct injection in the cylinder or preventing blow-through of CNG during the valve overlap period will be necessary.
All datasets generated for this study are included in the article/supplementary material.
To improve the trade-off relation between NOx and smoke emissions, diesel dual fuel operation (DDF) which introduces natural gas from the intake pipe and injects biofuel into the combustion chamber was conducted combining supercharging and cooled EGR. Natural gas is an attractive alternative fuel for engines and the DDF of this type has the advantages that only limited modifications are needed. The research contributes to energy security and improving the NOx and smoke emission trade-off. The study used blended fuels with next generation bio-alcohols and fatty acid methyl esters (FAME) as the ignition fuel. As the next generation bio-alcohols can be produced from non-food based sources like cellulosic biomass, this system realizes a petroleum free fuel. Recently, a number of studies with next generation bio-alcohol blended fuels have been carried out targeting ordinary diesel operation. However, many of these studies have been conducted with n-butanol, iso-butanol, or n-pentanol, and there are few reports with iso-pentanol. Also, there are no reports with the DDF. The present study concluded that there are conditions that may improve the NOx and smoke emissions trade-off about 90% while suppressing the deterioration of the brake thermal efficiency by about 3%.
YY have made a substantial and direct contribution to the work. EK and TO have made an intellectual contribution. All authors contributed to manuscript revision and approved the submitted version.
This study was supported by a grant-in-aid-for scientific research (17K07031) from the JSPS.
The authors declare that the research was conducted in the absence of any commercial or financial relationships that could be construed as a potential conflict of interest.
The authors wish to thank the students at the Heat Energy Laboratory, Niigata Institute of Technology, for their cooperation.
Abdelaal, M. M., and Hegab, A. H. (2012). Combustion and emission characteristics of a natural gas-fueled diesel engine with EGR. Energy Conv. Manage. 64, 301–312. doi: 10.1016/j.enconman.2012.05.021
Aklouche, F. Z., Loubar, K., Bentebbiche, A., Awad, S., and Tazerout, M. (2017). Experimental investigation of the equivalence ratio influence on combustion, performance and exhaust emissions of a dual fuel diesel engine operating on synthetic biogas fuel. Energy Conv. Manage. 152, 291–299. doi: 10.1016/j.enconman.2017.09.050
Aksu, C., Kawahara, N., Tsuboi, K., Kondo, M., and Tomita, E. (2016). Extension of PREMIER combustion operation range using split micro pilot fuel injection in a dual fuel natural gas compression ignition engine: a performance-based and visual investigation. Fuel 185, 243–253. doi: 10.1016/j.fuel.2016.07.120
Anil Bhaurao, W, Banerjee, S., and Banerjee, R. (2018). Experimental and chemical kinetic study of the impact of n-butanol blending on the gross engine performance of a CRDI engine. Energy Conv. Manage. 178, 400–414. doi: 10.1016/j.enconman.2018.10.031
Aroonsrisopon, T., Salad, M., Wirojsakunchai, E., Wannatong, K., Siangsanorh, S., and Akarapanjavit, N. (2009). Injection Strategies for Operational Improvement of Diesel Dual Fuel Engines Under Low Load Conditions. SAE Technical Paper. (No. 2009-01-1855). doi: 10.4271/2009-01-1855
Azimov, U., Tomita, E., and Kawahara, N. (2011). Ignition, Combustion and Exhaust Emission Characteristics of Micro-Pilot Ignited Dual-Fuel Engine Operated Under PREMIER Combustion Mode. SAE Technical Paper. (No. 2011-01-1764). doi: 10.4271/2011-01-1764
Blasio, G. D., Belgiorno, G., and Beatrice, C. (2017). Effects on performances, emissions and particle size distributions of a dual fuel (methane-diesel) light-duty engine varying the compression ratio. Appl. Energy 204, 726–740. doi: 10.1016/j.apenergy.2017.07.103
Bora, B. J., Saha, U. K., Chatterjee, S., and Veer, V. (2014). Effect of compression ratio on performance, combustion and emission characteristics of a dual fuel diesel engine run on raw biogas. Energy Conv. Manage. 87, 1000–1009. doi: 10.1016/j.enconman.2014.07.080
Çelebi, K., Uludamar, E., Tosun, E., Yildizhan, S., Aydin, K., and Ozcanli, M. (2017). Experimental and artificial neural network approach of noise and vibration characteristic of an unmodified diesel engine fuelled with conventional diesel, and biodiesel blends with natural gas addition. Fuel 197, 159–173. doi: 10.1016/j.fuel.2017.01.113
Fushimi, K., Kinoshita, E., and Yoshimoto, Y. (2013). Effect of Butanol Isomer on Diesel Combustion Characteristics on Butanol/Gas Oil Blend. SAE Technical Paper. (No. 2013-32-9097). doi: 10.4271/2013-32-9097
Graboski, M. S., McCormick, R. L., Alleman, T. L., and Herring, A. M. (2003). The Effect of Biodiesel Composition on Engine Emissions From a DDC Series 60 Diesel Engine. NREL/SR-510-31461. National Renewable Energy Laboratory, 1–81. doi: 10.2172/15003583
Guerry, E. S., Raihan, M. S., Srinivasan, K. K., Krishnan, S. R., and Sohail, A. (2016). Injection timing effects on partially premixed diesel–methane dual fuel low temperature combustion. Appl. Energy 162, 99–113. doi: 10.1016/j.apenergy.2015.10.085
Han, X., Yang, Z., Wang, M., Tjong, J., and Zheng, M. (2016). Clean combustion of n-butanol as a next generation biofuel for diesel engines. Appl. Energy 198, 347–359. doi: 10.1016/j.apenergy.2016.12.059
Ileri, E. (2016). Experimental study of 2-ethylhexyl nitrate effects on engine performance and exhaust emissions of a diesel engine fueled with n-butanol or 1-pentanol diesel–sunflower oil blends 2016. Energy Conv. Manage. 118, 320–330. doi: 10.1016/j.enconman.2016.04.015
Imdadul, H. K., Rashed, M. M., Shahin, M. M., Masjuki, H. H., Kalam, M. A., Kamruzzaman, M., et al. (2017). Quality improvement of biodiesel blends using different promising fuel additives to reduce fuel consumption and NO emission from CI engine. Energy Conv. Manage. 138, 327–337. doi: 10.1016/j.enconman.2017.01.077
Iorio, S. D., Magno, A., Mancaruso, E., and Vaglieco, B. M. (2017). Analysis of the effects of diesel/methane dual fuel combustion on nitrogen oxides and particle formation through optical investigation in a real engine. Fuel Proces. Technol. 159, 200–210. doi: 10.1016/j.fuproc.2017.01.009
Killol, A., Reddy, N., Paruvada, S., and Murugan, S. (2019). Experimental studies of a diesel engine run on biodiesel n-butanol blends. Renew. Energy. 135, 687–700. doi: 10.1016/j.renene.2018.12.011
Kinoshita, E., Ueda, Y., and Yoshimoto, Y. (2009). Combustion characteristics of a dual fuel diesel engine with coconut oil methyl ester as an ignition fuel. Trans. JSME, Series B 75, 1706–1711. doi: 10.1299/kikaib.75.756_1706
Kumar, B. R., and Saravanan, S. (2015). Effect of exhaust gas recirculation (EGR) on performance and emissions of a constant speed DI diesel engine fueled with pentanol/diesel blends. Fuel 160, 217–226. doi: 10.1016/j.fuel.2015.07.089
Kumar, B. R., and Saravanan, S. (2016). Effects of iso-butanol/diesel and n-pentanol/diesel blends on performance and emissions of a DI diesel engine under premixed LTC (low temperature combustion) mode. Fuel 170, 49–59. doi: 10.1016/j.fuel.2015.12.029
Li, W., Liu, Z., Wang, Z., and Dou, H. (2015). Experimental and theoretical analysis of effects of atomic, diatomic and polyatomic inert gases in air and EGR on mixture properties, combustion, thermal efficiency and NOx emissions of a pilot-ignited NG engine. Energy Conv. Manage. 105, 1082–1095. doi: 10.1016/j.enconman.2015.08.052
Mizushima, N., Ito, S., Kusaka, J., and Daisho, Y. (2003). Improvement of Combustion in a Dual Fuel Natural Gas Engine With Half the Number of Cylinders. SAE Technical Paper. (No. 2003-01-1938). doi: 10.4271/2003-01-1938
Ogawa, H., Miyamoto, N., Li, C., Nakazawa, S., and Akao, K. (2003). Smokeless and low NOx combustion in a dual-fuel diesel engine with induced natural gas as the main fuel. Int. J. Eng. Res. 4, 1–9. doi: 10.1177/146808740300400101
Ogawa, H., Shibata, G., Goto, J., and Jiang, L. (2016). Performance Improvements in a Natural Gas Dual Fuel Compression Ignition Engine With 250 MPa Pilot Injection of Diesel Fuel as an Ignition Source. SAE Technical Paper. (No. 2016-01-2306). doi: 10.4271/2016-01-2306
Ogawa, H., Zhao, P., Kato, T., and Shibata, G. (2015). Improvement of Combustion and Emissions in a Dual Fuel Compression Ignition Engine With Natural Gas as the Main Fuel. SAE Technical Paper. (No. 2015-01-0863). doi: 10.4271/2015-01-0863
Pan, M., Huang, R., Liao, J., Jia, C., Zhou, X., Huang, H., et al. (2019). Experimental study of the spray, combustion, and emission performance of a diesel engine with high n-pentanol blending ratios. Energy Conv. Manage. 194, 1–10. doi: 10.1016/j.enconman.2019.04.054
Papagiannakis, R. G., Hountalas, D. T., Rakopoulos, C. D., and Rakopoulos, D. C. (2008). Combustion and Performance Characteristics of a DI Diesel Engine Operating From Low to High Natural Gas Supplement Ratios at Various Operating Conditions. SAE Technical Paper. (No. 2008-01-1392). doi: 10.4271/2008-01-1392
Park, J. S., and Terao, H. (2003). Performance characteristics of a biogas cogeneration system using supercharging together with EGR. J. JSAM 65, 93–100. doi: 10.11357/jsam1937.65.6_93
Paul, A., Panua, R. S., Debroy, D., and Bose, P. K. (2014). Effect of compressed natural gas dual fuel operation with diesel and Pongamia pinnata methyl ester (PPME) as pilot fuels on performance and emission characteristics of a CI (compression ignition) engine. Energy 68, 495–509. doi: 10.1016/j.energy.2014.03.026
Peralta-Yahya, P. P., and Keasling, J. D. (2010). Advanced biofuel production in microbes. Biotechnol. J. 5, 147–162. doi: 10.1002/biot.200900220
Roy, M. M., Tomita, E., Kawahara, N., Harada, Y., and Sakane, A. (2009). Effect of Fuel Injection Parameters on Engine Performance and Emissions of a Supercharged Producer Gas-Diesel Dual Fuel Engine. SAE Technical Paper. (No. 2009-01-184). doi: 10.4271/2009-01-1848
Saravanan, S., Kumar, B. R., Varadharajan, A., Balaji Sethuramasamyraja, D. R., and Narayana rao, G. L. (2017). Optimization of DI diesel engine parameters fueled with iso-butanol/diesel blends – response surface methodology approach. Fuel 203, 658–670. doi: 10.1016/j.fuel.2017.04.083
Selim, M. Y. E. (2003). A Study of Some Combustion Characteristics of Dual Fuel Engine Using EGR. SAE Technical Paper. (No. 2003-01-0766). doi: 10.4271/2003-01-0766
Srinivasan, K. K., Krishnan, S. R., Qi, Y., Midkiff, K. C., and Yang, H. (2007). Analysis of diesel pilot-ignited natural gas low-temperature combustion with hot exhaust gas recirculation. Combust. Sci. Technol. 179, 1737–1776. doi: 10.1080/00102200701259882
Taniguchi, S., Masubuchi, M., Kitano, K., and Mogi, K. (2012). Feasibility Study of Exhaust Emissions in a Natural Gas Diesel Dual Fuel (DDF) Engine. SAE Technical Paper. (No. 2012-01-1649). doi: 10.4271/2012-01-1649
Tat, M. E., and Van Gerpen, J. H. (2003). Measurement of biodiesel speed of sound and its impact on injection timing. NREL/SR 510–31462. doi: 10.2172/15003584
Tomita, E., Harada, Y., Kawahara, N., and Sakane, A. (2009). Effect of EGR on Combustion and Exhaust Emissions in Supercharged Dual-Fuel Natural Gas Engine Ignited With Diesel Fuel. SAE Technical Paper. (No. 2009-01-1832). doi: 10.4271/2009-01-1832
Verma, S., Das, L. M., Bhatti, S. S., and Kaushik, S. C. (2017). A comparative exergetic performance and emission analysis of pilot diesel dual-fuel engine with biogas, CNG and hydrogen as main fuels. Energy Conv. Manage. 151, 764–777. doi: 10.1016/j.enconman.2017.09.035
Wannatong, K., Akarapanyavit, N., Siengsanorh, S., Aroonsrisopon, T., and Chanchaona, S. (2009). New Diesel Dual Fuel Concepts: Part Load Improvement. SAE Technical Paper. (No. 2009-01-1797). doi: 10.4271/2009-01-1797
Yang, B., Xi, C., Wei, X., Zeng, K., and Lai, M. C. (2015). Parametric investigation of natural gas port injection and diesel pilot injection on the combustion and emissions of a turbocharged common rail dual-fuel engine at low load. Appl. Energy 143, 130–137. doi: 10.1016/j.apenergy.2015.01.037
Yoshimoto, Y. (2010). Combustion Characteristics of a Dual Fuel Diesel Engine With Natural Gas (Study With Fatty Acid Methyl Esters Used as Ignition Fuels). SAE Technical Paper. (No. 2010-32-0050). doi: 10.4271/2010-32-0050
Yoshimoto, Y., Enkhjargal, T., Kinoshita, E., and Otaka, T. (2018b). Combustion improvements by C4/C5 bio-alcohol isomer blended fuels combined with supercharging and EGR in a diesel engine. Int. J. Mech. Indus. Aerospace Sci. 11, 1–9. doi: 10.5281/zenodo.1474930
Yoshimoto, Y., Kaneko, K., and Kinoshita, E. (2010). Combustion characteristics of a dual fuel diesel engine with CNG as the main fuel (Study for methyl oleate used as an ignition fuel). Trans. JSME Series B 76, 916–923. doi: 10.1299/kikaib.76.765_916
Yoshimoto, Y., and Kinoshita, E. (2011). Combustion characteristics of a dual fuel diesel engine with natural gas (influence of cetane number of ignition fuel). ASME Power 2011:55362. doi: 10.1115/POWER2011-55362
Yoshimoto, Y., and Kinoshita, E. (2013). Influence of Intake Air Dilution With N2 or CO2 Gases on the Combustion Characteristics of a Dual Fuel Diesel Engine With Natural Gas. SAE Technical Paper. (No. 2013-01-2691). doi: 10.4271/2013-01-2691
Yoshimoto, Y., Kinoshita, E., Luge, S., and Ohmura, T. (2012). Combustion characteristics of a dual fuel diesel engine with natural gas (lower limit of cetane number for ignition of the fuel). SAE Int. J. Fuels Lubr. 5, 1165–1173. doi: 10.4271/2012-01-1690
Yoshimoto, Y., Kinoshita, E., and Otaka, T. (2017). “Influence of boost pressure on the combustion characteristics of a dual fuel diesel engine ignited by biofuels with natural gas,” in: The Ninth International Conference on Modeling and Diagnostics for Advanced Engine Systems (COMODIA 2017) (Okayama: Japan Society of Mechanical Engineers). doi: 10.1299/jmsesdm.2017.9.C204
Yoshimoto, Y., Kinoshita, E., and Otaka, T. (2018a). Effects of combining EGR and supercharging on the combustion characteristics of a diesel dual fuel engine with induced natural gas, Trans. JSME 84, 1–15. doi: 10.1299/transjsme.18-00219
Yoshimoto, Y., Kinoshita, E., and Otaka, T. (2019). Influence of the Kind of Fuel Kind in the Ignition of Diesel Dual Fuel Operation With Introduced Natural Gas Combining EGR and Supercharging. SAE Technical Paper. (No. 2019-32-0581)
Yoshimoto, Y., Kinoshita, E., Shanbu, L., and Ohmura, T. (2013). Influence of 1-butanol addition on diesel combustion with palm oil methyl ester/gas oil blends. Energy 61, 44–51. doi: 10.1016/j.energy.2012.11.039
Yoshimoto, Y., Yamada, M., Kinoshita, E., and Fushimi, K. (2016). Influence of supercharging on the combustion characteristics of a dual fuel diesel engine with induced natural gas. Trans. JSME 82, 1–11. doi: 10.1299/transjsme.15-00542
Zheng, J., Tashiro, Y., Wang, Q., and Sonomoto, K. (2015). Recent advances to improve fermentative butanol production: genetic engineering and fermentation technology. J. Biosci. Bioeng. 119, 1-9. doi: 10.1016/j.jbiosc.2014.05.023
DDF: diesel dual fuel operation
DDFD: diesel dual fuel operation with diesel fuel ignition
CNG: compressed natural gas
EGR: exhaust gas recirculation
N/A: naturally aspirated operation
S/C: supercharged operation
FAME: fatty acid methyl ester
PME: methyl palmitate
LME: methyl laurate
PLME: equal proportion of PME and LME
PLiP30: 70% PLME and 30% iso-pentanol blend (mass ratio)
PLiB30: 70% PLME and 30% iso-butanol blend (mass ratio)
LiP30: 70% LME and 30% iso-pentanol blend (mass ratio)
DPF: diesel particulate filter
CLD: chemiluminescence detector
FID: flame ionization detector
NDIR: non-dispersed infrared detector
Qg/Qt: CNG supply rate
Qg: heat energy of supplied CNG
Qt: total heat energy available in cylinder
θ: crank angle
Q: heat release in cylinder
QE: heating value of fuel
QC: cooling heat to cylinder wall
P: in-cyinder gas pressure
V: in-cyinder gas volume
κ: ratio of specific heat
ε: compression ratio
εθ:compression ratio at crank angle
ϕg: equivalence ratio of CNG-air pre-mixture
ϕt: equivalence ratio of total in-cylinder charge
BMEP: brake mean effective pressure
BTE, ηe: brake thermal efficiency
Ne: brake power
ṁ: mass flow rate
LHV: lower heating value
ηu: combustion efficiency
ηglh: degree of constant volume of combustion
(dQ/dθ)max: maximum heat release rate
Keywords: diesel dual fuel operation, alternative fuel, fatty acid methyl ester, next generation bio-alcohol, EGR, supercharging, trade-off improvement
Citation: Yoshimoto Y, Kinoshita E and Otaka T (2020) Trade-off Improvements by Combining EGR and Supercharging Ignited by Next Generation Bio-alcohol Blended FAME Fuels in Diesel Dual Fuel Operation Using Natural Gas. Front. Mech. Eng. 6:67. doi: 10.3389/fmech.2020.00067
Received: 28 January 2020; Accepted: 07 July 2020;
Published: 19 August 2020.
Edited by:
Hongsheng Guo, National Research Council Canada (NRC-CNRC), CanadaReviewed by:
Hannu Jaaskelainen, Ecopoint Inc., (DieselNet), CanadaCopyright © 2020 Yoshimoto, Kinoshita and Otaka. This is an open-access article distributed under the terms of the Creative Commons Attribution License (CC BY). The use, distribution or reproduction in other forums is permitted, provided the original author(s) and the copyright owner(s) are credited and that the original publication in this journal is cited, in accordance with accepted academic practice. No use, distribution or reproduction is permitted which does not comply with these terms.
*Correspondence: Yasufumi Yoshimoto, eW9zaW1vdG9AbWNlLm5paXQuYWMuanA=
Disclaimer: All claims expressed in this article are solely those of the authors and do not necessarily represent those of their affiliated organizations, or those of the publisher, the editors and the reviewers. Any product that may be evaluated in this article or claim that may be made by its manufacturer is not guaranteed or endorsed by the publisher.
Research integrity at Frontiers
Learn more about the work of our research integrity team to safeguard the quality of each article we publish.