- 1Science and Technology on Microsystem Laboratory, Shanghai Institute of Microsystem and Information Technology, Chinese Academy of Sciences, Shanghai, China
- 2University of Chinese Academy of Sciences (UCAS), Beijing, China
- 3State Key Laboratory of Proteomics, Beijing Proteome Research Center, National Center for Protein Sciences (Beijing), Beijing Institute of Lifeomics, Beijing, China
- 4National Engineering Research Center for Protein Drugs (NERCPD), Beijing, China
In this work, we combined photoreceptor protein with graphene based field effect transistors to achieve a high responsivity photodetector. Three different aromatic hydrocarbons were used to functionalize graphene with carboxyl groups via π-π stacking interactions, and 1-pyrenebutyric acid exhibited the best result for photoreceptor protein immobilization. Besides, the modification procedure of photoreceptor protein was also optimized by using a novel protein stack way on the device. Unlike conventional self-assembly method, photoreceptor proteins were cross-linked before immobilized on graphene thin film and yielded a higher modification density. Finally, the photocurrent response was significantly enhanced from −1% to −9.62%. These results bring key opportunity for graphene field effect transistor based photodetection, and the surface functionalization method are expected to help achieve high sensing performance on protein-based biosensors.
Introduction
As a typical thin-film carbon material, graphene has unique structure and excellent properties (Geim and Novoselov, 2007; Castro Neto et al., 2009; Afsahi et al., 2018). The ultra-high carrier mobility for both electrons and holes, broad light absorption from 300 nm to 2.5 μm, excellent mechanical properties and strength make it an ideal material in photonics over other materials (Xia et al., 2009; Bonaccorso et al., 2010; Mak et al., 2012). But the low light absorption results in low responsivity (Nair et al., 2008; Park et al., 2009). Enhancing responsivity is central to graphene-based photodetectors. Great efforts have been done in opening the band gap of graphene or designing the device structure to improve the photoresponse, and significant achievements have been made (Wang et al., 2008; Mueller et al., 2010; Avouris and Freitag, 2014; Liu et al., 2014). But these complex structures increase the complexity and cost of the process.
Photoreceptor proteins have been reported to provide high sensitivity to low-intensity light, low cost of fabrication and good wavelength selectivity (Jolley et al., 2005; Ciesielski et al., 2010; Nabiev et al., 2010; Conrad et al., 2014). Recent years witnessed a great number of advances in the research field of photoreceptors, which opens the door of utilizing photoreceptors as a novel photosensitive material in the fabrication of biophotosensors (Gibson and Woodward, 1992; Kim et al., 2014). Photoreceptor proteins based near-infrared (NIR) photodetector (Gong et al., 2017), photovoltaic device (Das et al., 2004) and wavelength selective photodetector (Lu et al., 2012) have been manufactured. Despite the progress in biophotosensors, the bioconjugation strategies between photoreceptor proteins and transducers has always been the key to their performance, such as protein conformation, activity and stability (Bhakta et al., 2015; Liebana and Drago, 2016).
With excellent biocompatibility, graphene is promise for loading photoreceptor proteins and can serve as a redox center of the active substances (De Leo et al., 2015; Kwon et al., 2015; Suvarnaphaet and Pechprasarn, 2017; Dedelaite et al., 2018). Due to the two-dimensional honeycomb structure of graphene, photoreceptor proteins can be modified to graphene by π-π stacking interactions (Liu et al., 2011). π-π stacking functional groups can give graphene new properties, while retaining the mechanical, chemical, and electronic properties (Georgakilas et al., 2012; Kuila et al., 2012). These original properties of graphene are critical to the signal conversion of biosensors. However, the traditional monolayer self-assembly method leads to low modification efficiency of photoreceptor proteins. Therefore, optimizing the spatial assembly of photoreceptor proteins is a critical factor for the effective application of biophotosensors.
In this work, we constructed a highly responsive biophotoconductor based on graphene field effect transistor (GFET). The graphene channel was modified with blue light using flavin (BLUF) from photoreceptor protein AppA (Jung et al., 2005; Masuda et al., 2005; Mathes et al., 2012; Masuda, 2013; Fudim et al., 2015). First of all, we used three carboxyl molecules, namely 1-pyenecarboxylic acid (PCA), 1-naphthylacetic acid (NAA) and 1-pyrenebutyric acid (PBA) to functionalize graphene through π-π interaction and selected the most suitable carboxyl molecule by comparing the performance of the obtained devices. Then, unlike the traditional monolayer self-assembly method, we adopted a cross-linking modification method to improve the density of modified graphene. The protein molecules were first joined and then modified onto the graphene surface. This work illustrates a practical functionalization scheme and shows how to improve the performance of the biophotoconductor by judicious selection of the functional molecules and protein assembly methods.
Experimental
Materials and Reagents
The pristine graphene films growth by chemical vapor deposition (CVD) method on copper foils were purchased from 2D Carbon (China). The 1-pyrenecarboxylic acid (PCA), 1-pyrenebutyric acid (PBA), N-(3-dimethylaminopropyl)-N-ethylcarbodiimide hydrochloride (EDC), N-hydroxysuccinimide (NHS), 4-morpholineethanesulfonic acid (MES), phosphate-buffered saline (PBS) and ethanolamine were purchased from Sigma-Aldrich (United States). 1-naphthylacetic acid (NAA) was purchased from TCI (Japan). Acetonitrile used to dissolve PCA, NAA, and PBA was purchased from Sinopharm Chemical Reagent Co., Ltd., (China). The expression and purification of BLUF have been reported (Tong et al., 2020).
Device Fabrication
The graphene field effect transistors were fabricated on a 4-inch silicon substrate. Firstly, a dense layer of silicon dioxide (300 nm) was fabricated on the silicon substrate as an insulating layer. Then the 20 nm titanium/100 nm gold source-drain electrodes were deposited onto the SiO2 substrate by photolithography, magnetron sputtering and lift-off process. Graphene film was transferred onto SiO2 surface by using PMMA [poly(methyl methacrylate)] as a sacrificial layer, and then patterned by O2 plasma. Finally, the wafer was subjected to thermal annealing in a 5:4 mixture of argon and hydrogen gas at 300°C for 4 h. The graphene channel could be electrically gated using the back gate.
Surface Modification
The devices were first immersed in 1 mM aromatic hydrocarbon (PCA, NAA, or PBA) solutions at room temperature for 2 h to create a layer of carboxylic acid groups, and then rinsed with alcohol and deionized water. The carboxylic acid groups were then activated in a 20 μl of 1:1 mixture of 0.4 M EDC and 0.1 M NHS (0.1 M MES, pH 5.2) solution for 1 h at room temperature. After that, the device was rinsed in 0.1 M MES (pH 5.2). Subsequently, the BLUF proteins can be covalently attached onto the graphene surface through the reactive NHS-ester groups. As for single layer BLUF, the device was treated with 10 μl 1 mg/ml BLUF solution overnight at 4°C. As for cross-linking BLUF, the device was exposed to a 30 μl of 1:1:1 mixture of 0.4 M EDC, 0.1 M NHS and 1 mg/ml BLUF overnight at 4°C. After immobilizing BLUF and rinsing with 1 × PBS (pH 7.4), 1 mM ethanolamine in 1 × PBS solution (pH 7.4) was added to the device for 20 min to deactivate and block the excess reactive groups remained on the graphene surface. Finally, the BLUF modified device was rinsed with deionized water and used for subsequent photodetection.
Sensing Apparatus and Parameters
Optical imaging was used to observe whether the transferred graphene was damaged, multilayered or wrinkled. Raman spectroscopy (DXR, Thermo Fisher, United States) was taken to explore the quality of graphene. Atomic force microscopy (AFM) was taken to determine the surface topology and thickness of BLUF-modified graphene films (NT-MDT, Russia). FT-IR (Fourier transform infrared) spectroscopy (A540/3, Bruker) was taken to verify the chemical composition of the surface of graphene before and after modification. The hydrophobicity of the substrate during modification process was measured by a contact angle meter. Electrical measurements were conducted using a Keithley 4200 semiconductor (Keithley Instruments Inc., Cleveland, OH, United States). The light sensing measurements were conducted using a UV LED spotlight source (BJ Jing Zhen Da Tec., China) with a wavelength of 450 nm.
Results and Discussion
Fabrication and Characterization of GFET
Figure 1A shows the schematic representation of the GFET. The single layer graphene manufactured by chemical vapor deposition (CVD) process was transferred to the wafer by using PMMA as a supporting layer. Then the PMMA layer was removed by acetone. The residues associated with transfer may affect the stability of subsequent biomolecule connections, which were removed by annealing. Raman spectroscopy is an effect method to characterize the structure of graphene including the number of layers, defects and disorder (Ferrari and Basko, 2013). Raman analysis was performed with a 514 nm laser to measure the distinctive peaks of pristine graphene (Figure 1B). The D band is nearly free, and the intensity of 2D (at 2690 cm–1) peak is about twice of the G (at 1590 cm–1) peak. Therefore, a low defect monolayer graphene was successfully covered on the substrate.
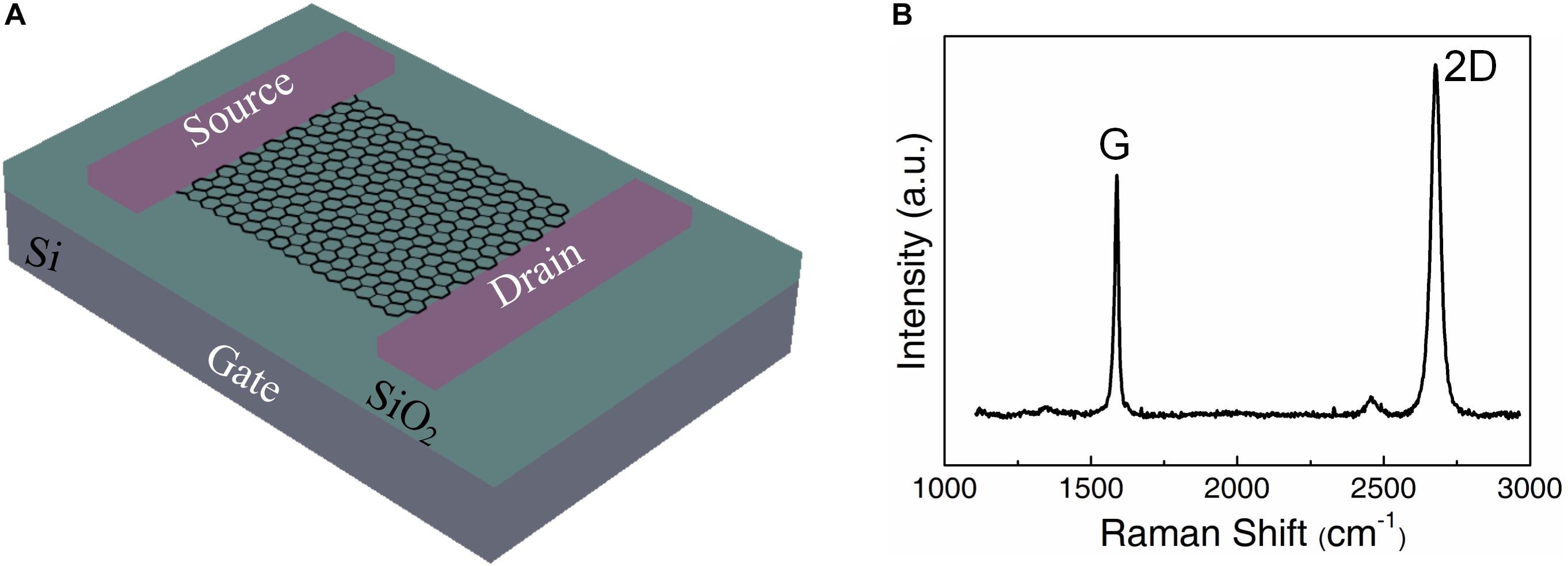
Figure 1. Fabrication and characterization of the graphene field effect transistor. (A) The schematic representation of the pristine graphene substrate. (B) The Raman spectrum of pristine graphene.
Selection of Carboxyl Molecules
First, the modification effects of different carboxyl molecules were studied. As shown in Figure 2A, we used these three carboxyl molecules to assemble a single layer of BLUF-K5 (a kind of BLUF with five amino groups) onto graphene. The surface of graphene was first carboxylated through non-covalent π-π stacking interactions. Then the terminal carboxylic acid groups were reactivated by a mixed solution of EDC and NHS. The reactive NHS-ester groups can bind molecules with amino groups. Thus, BLUF-K5 can be attached on the graphene surface through chemically stable peptide bonding.
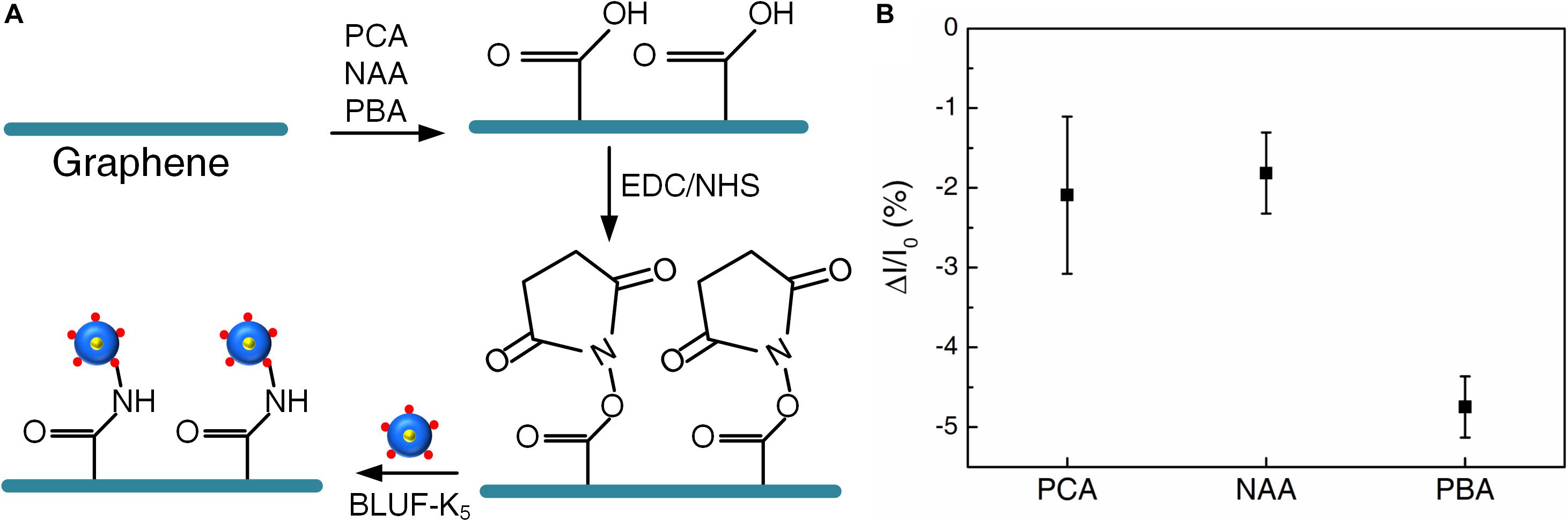
Figure 2. (A) The surface modification process by different carboxyl molecules: PCA, NAA, PBA. (B) Photocurrent responses of the devices under 450 nm illumination. Bias voltage is 10 mV.
In order to measure the optoelectronic performance of the devices, we placed the devices under a 450 nm light at 350 W/m2 and collected the photocurrent signals at room temperature. Photogenerated carriers were generated after the absorption of light by BLUF (Masuda, 2013), which affected the carrier concentration of graphene. The functionalization of BLUF makes graphene n-doped. The conductivity was decreased due to the photoexcited proteins injecting holes into n-doped graphene (Tong et al., 2020). Thus, ΔI/I0 was used as an indicator of the change in photoconductivity. As shown in Figure 2B, the performance of the device functionalized through PBA was significantly better than PCA and NAA. The photocurrent response of the device functionalized through PBA was about −5%, which was twice that of the other two and had strong stability. PBA yielded an efficient method for the connection between graphene and photoreceptor protein.
Selection of Protein Assembly Methods
Consequently, PBA was selected as the graphene carboxyl functionalized molecule to study the best method for protein assembly. We used two kinds of BLUF with different numbers of amino groups, BLUF-K1 with one amino group and BLUF-K5 with five amino groups. BLUF proteins were assembled in four different ways. The schematic illustration is shown in Figure 3. The contact angles and thicknesses were measured to analyze the protein density of these four assembly methods.
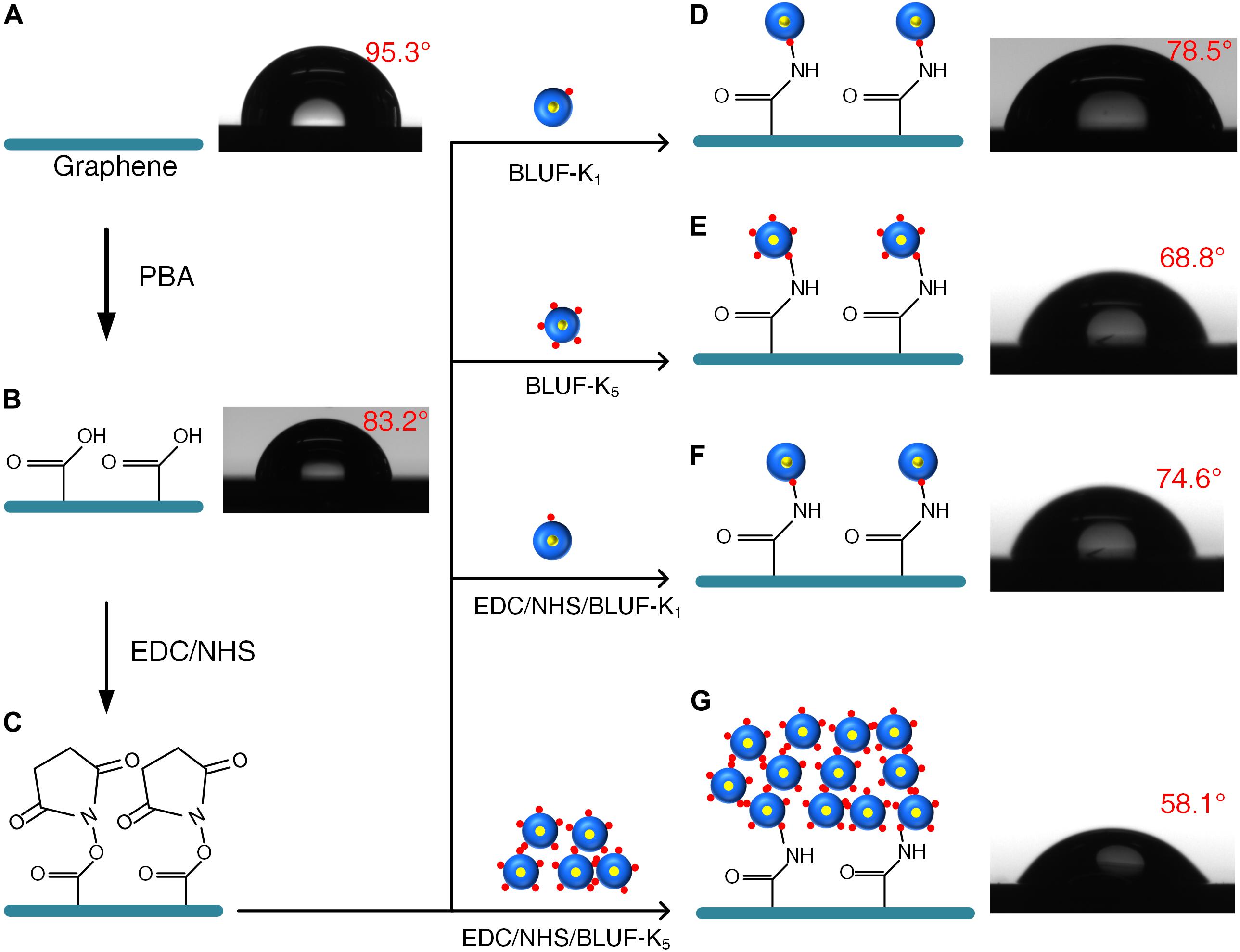
Figure 3. The surface modification process and contact angle images of the devices. (A) The pristine graphene with a contact angle of 95.3°. (B) Surface modification with PBA. The PBA modified graphene with a contact angle of 83.2°. (C) EDC/NHS conjugation. (D) Self-assembled monolayer of BLUF-K1. The contact angle is 78.5°. (E) Self-assembled monolayer of BLUF- K5. The contact angle is 68.8°. (F) Cross-linking of BLUF-K1. The contact angle is 74.6°. (G) Cross-linking of BLUF-K5. The contact angle is 58.1°.
Before modification, the contact angle of graphene was about 95.3° (Figure 3A). Due to the hydrophilic nature of the carboxylic acid groups, the contact angle was significantly reduced to 83.2° after the modification of PBA (Figure 3B). As for the self-assembly method, BLUF proteins were directly linked to NHS-ester groups to form a self-assembled monolayer (SAM). As shown in Figures 3D,E, the reactive NHS-esters bound to the BLUF proteins and a layer of protein molecules was modified on the graphene surface. The thickness of SAM BLUF-K1 was about 4.9 nm (Figure 4a), which was slightly lower than 5.6 nm of SAM BLUF-K5 (Figure 4b). However, the number of protein molecules linked by this monolayer self-assembly method is relatively small.
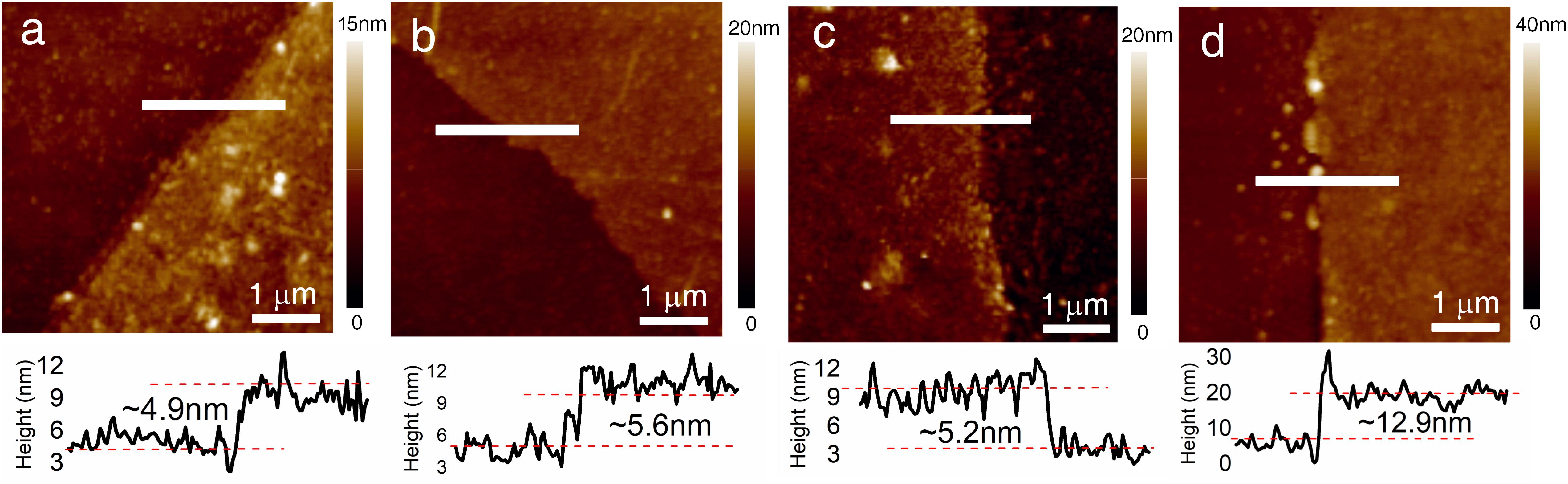
Figure 4. The AFM images of graphene hybrid with different BLUF. (a) Self-assembled monolayer of BLUF- K1. The thickness is approximately 4.9 nm when measured along the horizontal white line in AFM image. (b) Self-assembled monolayer of BLUF- K5. The thickness is approximately 5.6 nm when measured along the horizontal white line in AFM image. (c) Cross-linking of BLUF-K1. The thickness is approximately 5.2 nm when measured along the horizontal white line in AFM image. (d) Cross-linking of BLUF-K5. The thickness is approximately 12.9 nm when measured along the horizontal white line in AFM image.
Since this kind of photodetection does not require one-to-one recognition between BLUF proteins and light, we mixed EDC, NHS and BLUF proteins instead of directly adding BLUF proteins to promote cross-linking between BLUF proteins. On the other hand, BLUF can also react with the NHS-ester groups. Since BLUF-K1 has only one amino group, the cross-linked BLUF-K1 has no excess amino groups attached to graphene. Therefore, BLUF-K1 modified to graphene was still a single molecule without cross-linking (Figure 3F), leaving a monolayer of 5.2 nm thickness (Figure 4c). For BLUF-K1, the cross-linking and SAM modification methods produce the same devices, resulting in similar contact angles and thicknesses.
To enhance cross-linking, we used BLUF-K5 with five amino groups. Through cross-linking, BLUF-K5 could be connected with each other irregularly, and the unreacted amino groups could be linked with graphene. The contact angle of the SAM BLUF-K5 substrate was approximately 68.8° and that of the cross-linked BLUF-K5 increased to 58.1°. The thickness of cross-linked BLUF-K5 was 12.9 nm (Figure 4d), which was about twice that of SAM BLUF-K5. These results indicate that the density of photosensitive units was greatly increased through the cross-linking of BLUF-K5.
The FT-IR spectra of graphene before and after modification with BLUF were measured to confirm the groups for conjugation (Figure 5). As for pristine graphene, the spectra had a wide absorption peak from 1475 cm–1 to 1645 cm–1, which represented the C skeleton vibration. The main vibration peaks of proteins are amide I and amide II bands. After modification with BLUF, the amide II band at 1685 cm–1 and amide I band at 1592 cm–1 which were associated with the N-H bend and C = O stretch modes, respectively, were observed.
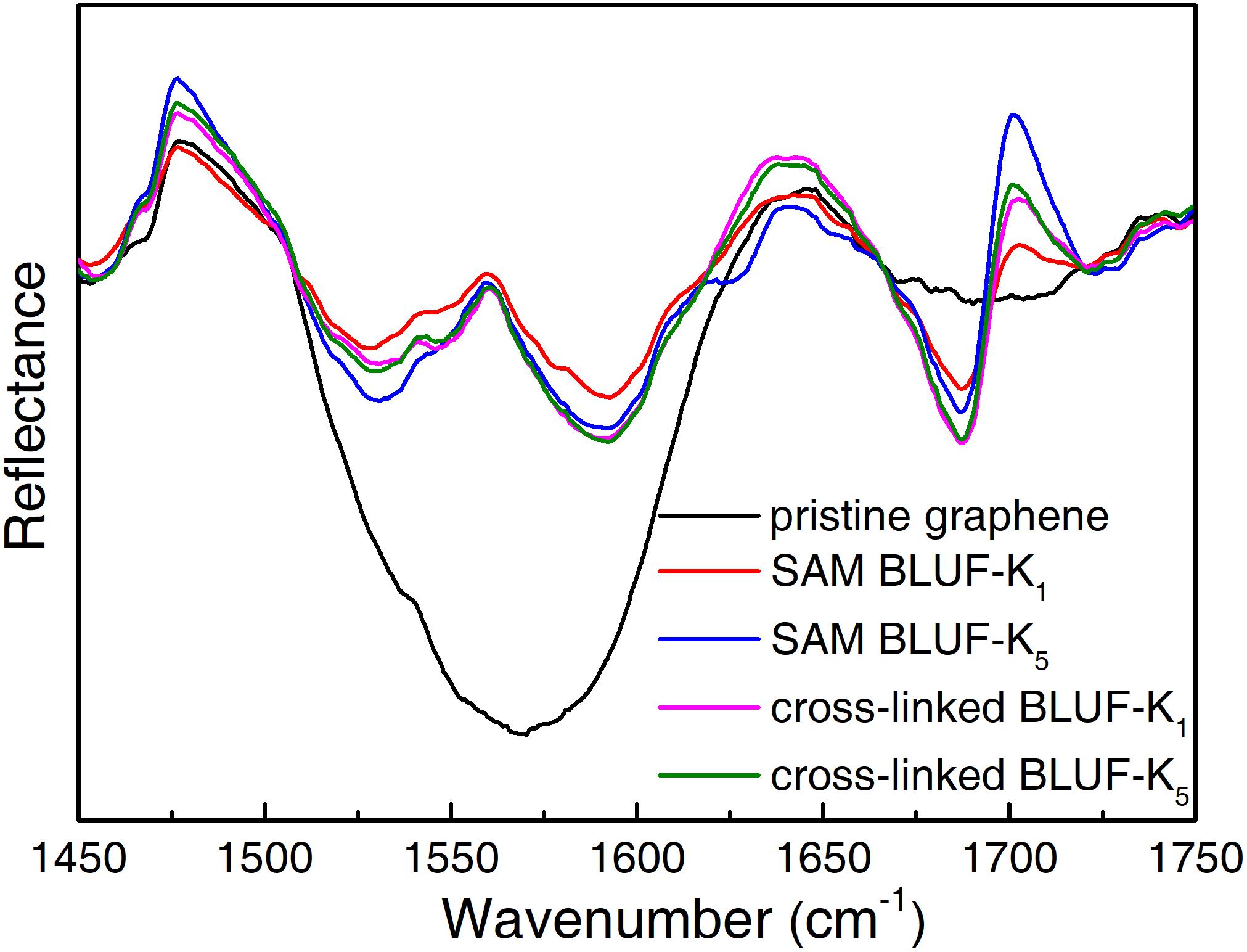
Figure 5. FT-IR reflectance spectra of pristine graphene (black) and after modification with BLUF through PBA.
We then investigated the photoelectric response of these four protein assembly methods. The photocurrent response of the device after each modification process was measured as control experiments (Figure 6). Before modification, the photocurrent change of the pristine graphene device was almost negligible. This is consistent with the too low light absorption rate of graphene. After treated with PBA and EDC/NHS, the graphene surface was no longer clean and some functional groups were attached. The device had a slight photocurrent change within −2% under illumination due to the little absorption from these functional groups.
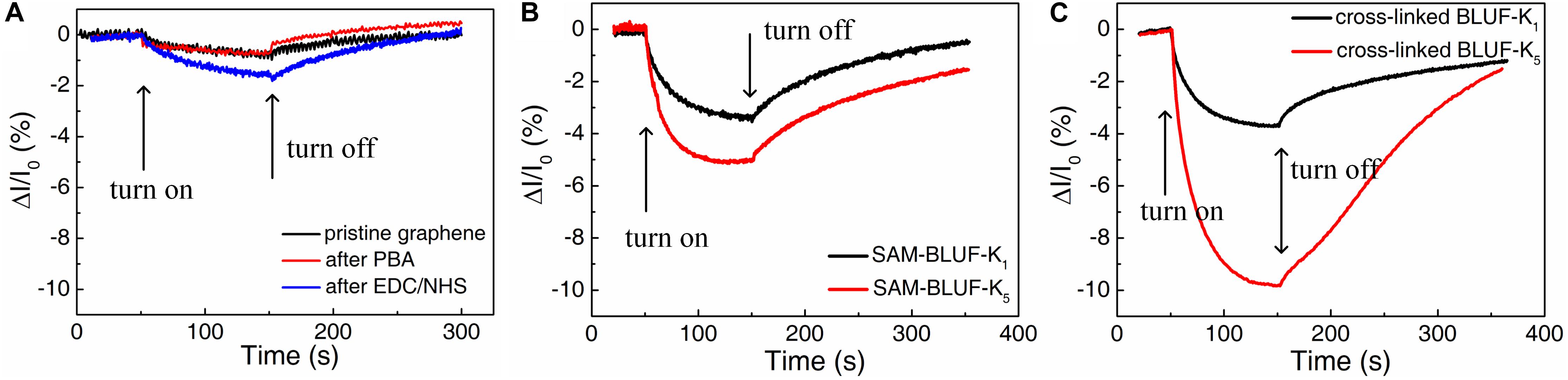
Figure 6. The real-time photocurrent response for the devices with different functionalization. (A) Before the modification of protein. (B) The devices functionalized with self-assembled monolayer of BLUF-K1 (black) and BLUF-K5 (red), respectively. (C) The devices functionalized with cross-linked BLUF-K1 (black) and BLUF-K5 (red), respectively. Responses were shown to 450 nm light under 350 W/m2. Bias voltage is 10 mV.
The real-time photocurrent responses of the proteins modified on graphene by these four ways have different properties. Compared with pristine graphene, the attachment of BLUF greatly reduced the photocurrent. The photocurrent of the device modified with BLUF-K1 through SAM was reduced by about 3.4% (Figure 6B). And for the device modified with five amino groups BLUF-K5 through SAM, the photocurrent was decreased to approximately −5.1%. Therefore, BLUF with five amino groups can be more easily modified onto the graphene surface. But the effect of this enhancement is not very obvious. Because the number of proteins modified on graphene through the SAM method is only one layer, this amount is limited by the area. Although the BLUF-K5 has five amino groups, it does not cause a qualitative change in the amount of modification directly. Thus, longitudinally enhanced cross-linking is one way to increase the protein density.
Then we measured the photocurrent response of the devices manufactured by modifying the proteins through cross-linking (Figure 6C). When exposed to light, the device modified with BLUF-K1 was reduced by about 3.7%. However, the device modified with BLUF-K5 was significantly reduced by about 9.62%. Compared to the self-assembly method, the device obtained by the modification of a single amino group BLUF did not improve the photodetection performance by cross-linking. Proteins with multiple amino groups can significantly improve the performance of photodetection. BLUF-K5 can be arbitrarily linked to the remaining BLUF-K5. But even by the method of cross-linking, BLUF with a single amino group can only be attached to graphene in a single layer. This kind of cross-linking modification method can greatly enhance the responsivity of the detector. But it requires that the protein molecules are linked together. Therefore, there must be enough amino groups on the protein molecules to ensure the cross-linking between each other.
Conclusion
In conclusion, we have successfully developed an effective method to achieve highly responsive light detection based on graphene through a biomimetic way. Graphene functionalized by aromatic compounds through non-covalent π-π stacking interaction did not disrupt its original structure and electrical property. 1-pyrenebutyric acid is a relatively stable graphene carboxylated molecule with higher response. Graphene has good biocompatibility with BLUF. BLUF modified to the device exhibited its biological function of highly light absorption. Moreover, the density of the modified protein was increased by cross-linking modification method, which greatly enhanced the photocurrent response of graphene. From a broad perspective, we created a significant improvement of the photodetection performance of pristine graphene by optimizing the carboxylated molecules and protein stack ways.
Data Availability Statement
The raw data supporting the conclusions of this article will be made available by the authors, without undue reservation.
Author Contributions
JT designed and performed the experiments, contributed to analysis of data, and preparation of the manuscript. LZ performed protein expression and purification. YW and TL designed the experiments, contributed to analysis of data, and preparation of the manuscript. All authors contributed to the article and approved the submitted version.
Funding
This work was supported by the National Key Research and Development Program of China (Nos. 2018YFA0208500 and 2017YFA0207103).
Conflict of Interest
The authors declare that the research was conducted in the absence of any commercial or financial relationships that could be construed as a potential conflict of interest.
References
Afsahi, S., Lerner, M. B., Goldstein, J. M., Lee, J., Tang, X., and Bagarozzi, D. A.Jr. et al. (2018). Novel graphene-based biosensor for early detection of Zika virus infection. Biosens. Bioelectron. 100, 85–88. doi: 10.1016/j.bios.2017.08.051
Avouris, P., and Freitag, M. (2014). Graphene photonics, plasmonics, and optoelectronics. IEEE J. Select. Top. Quant. Electron. 20:12. doi: 10.1109/jstqe.2013.2272315
Bhakta, S. A., Evans, E., Benavidez, T. E., and Garcia, C. D. (2015). Protein adsorption onto nanomaterials for the development of biosensors and analytical devices: a review. Anal. Chim. Acta 872, 7–25. doi: 10.1016/j.aca.2014.10.031
Bonaccorso, F., Sun, Z., Hasan, T., and Ferrari, A. C. (2010). Graphene photonics and optoelectronics. Nat. Photon. 4, 611–622. doi: 10.1038/nphoton.2010.186
Castro Neto, A. H., Guinea, F., Peres, N. M. R., Novoselov, K. S., and Geim, A. K. (2009). The electronic properties of graphene. Rev. Mod. Phys. 81, 109–162. doi: 10.1103/RevModPhys.81.109
Ciesielski, P. N., Faulkner, C. J., Irwin, M. T., Gregory, J. M., Tolk, N. H., Cliffel, D. E., et al. (2010). Enhanced photocurrent production by photosystem I multilayer assemblies. Adv. Funct. Mater. 20, 4048–4054. doi: 10.1002/adfm.201001193
Conrad, K. S., Manahan, C. C., and Crane, B. R. (2014). Photochemistry of flavoprotein light sensors. Nat. Chem. Biol. 10, 801–809. doi: 10.1038/nchembio.1633
Das, R., Kiley, P. J., Segal, M., Norville, J., Yu, A. A., Wang, L. Y., et al. (2004). Integration of photosynthetic protein molecular complexes in solid-state electronic devices. Nano Lett. 4, 1079–1083. doi: 10.1021/nl049579f
De Leo, F., Magistrato, A., and Bonifazi, D. (2015). Interfacing proteins with graphitic nanomaterials: from spontaneous attraction to tailored assemblies. Chem. Soc. Rev. 44, 6916–6953. doi: 10.1039/c5cs00190k
Dedelaite, L., Rodriguez, R. D., Andriukonis, E., Hietschold, M., Zahn, D. R. T., and Ramanavicius, A. (2018). Surfaces functionalized by graphene oxide nanosheets for single cell investigations. Sens. Act. B Chem. 255, 1735–1743. doi: 10.1016/j.snb.2017.08.187
Ferrari, A. C., and Basko, D. M. (2013). Raman spectroscopy as a versatile tool for studying the properties of graphene. Nat. Nanotechnol. 8, 235–246. doi: 10.1038/nnano.2013.46
Fudim, R., Mehlhorn, J., Berthold, T., Weber, S., Schleicher, E., Kennis, J. T. M., et al. (2015). Photoinduced formation of flavin radicals in BLUF domains lacking the central glutamine. FEBS J. 282, 3161–3174. doi: 10.1111/febs.13297
Geim, A. K., and Novoselov, K. S. (2007). The rise of graphene. Nat. Mater. 6, 183–191. doi: 10.1038/Nmat1849
Georgakilas, V., Otyepka, M., Bourlinos, A. B., Chandra, V., Kim, N., Kemp, K. C., et al. (2012). Functionalization of graphene: covalent and non-covalent approaches, derivatives and applications. Chem. Rev. 112, 6156–6214. doi: 10.1021/cr3000412
Gibson, T. D., and Woodward, J. R. (1992). Protein stabilization in biosensor systems. ACS Symp. Ser. 487, 40–55. doi: 10.1021/bk-1992-0487.ch005
Gong, Y., Liu, Q., Gong, M., Wang, T., Zeng, G., Chan, W.-L., et al. (2017). High-performance photodetectors based on effective exciton dissociation in protein-adsorbed multiwalled carbon nanotube nanohybrids. Adv. Opt. Mater. 5:1600478. doi: 10.1002/adom.201600478
Jolley, C., Ben-Shem, A., Nelson, N., and Fromme, P. (2005). Structure of plant photosystem I revealed by theoretical modeling. J. Biol. Chem. 280, 33627–33636. doi: 10.1074/jbc.M500937200
Jung, A., Domratcheva, T., Tarutina, M., Wu, Q., Ko, W. H., Shoeman, R. L., et al. (2005). Structure of a bacterial BLUF photoreceptor: insights into blue light-mediated signal transduction. Proc. Natl. Acad. Sci. U.S.A. 102, 12350–12355. doi: 10.1073/pnas.0500722102
Kim, Y., Shin, S. A., Lee, J., Yang, K. D., and Nam, K. T. (2014). Hybrid system of semiconductor and photosynthetic protein. Nanotechnology 25:20. doi: 10.1088/0957-4484/25/34/342001
Kuila, T., Bose, S., Mishra, A. K., Khanra, P., Kim, N. H., and Lee, J. H. (2012). Chemical functionalization of graphene and its applications. Prog. Mater. Sci. 57, 1061–1105. doi: 10.1016/j.pmatsci.2012.03.002
Kwon, O. S., Song, H. S., Park, S. J., Lee, S. H., An, J. H., Park, J. W., et al. (2015). An ultrasensitive, selective, multiplexed superbioelectronic nose that mimics the human sense of smell. Nano Lett. 15, 6559–6567. doi: 10.1021/acs.nanolett.5b02286
Liebana, S., and Drago, G. A. (2016). “Bioconjugation and stabilisation of biomolecules in biosensors,” in Biosensor Technologies for Detection of Biomolecules, ed. P. Estrela (London: Portland Press Ltd), 59–68. doi: 10.1042/ebc20150007
Liu, C. H., Chang, Y. C., Norris, T. B., and Zhong, Z. H. (2014). Graphene photodetectors with ultra-broadband and high responsivity at room temperature. Nat. Nanotechnol. 9, 273–278. doi: 10.1038/nnano.2014.31
Liu, F., Choi, K. S., Park, T. J., Lee, S. Y., and Seo, T. S. (2011). Graphene-based electrochemical biosensor for pathogenic virus detection. Biochip J. 5, 123–128. doi: 10.1007/s13206-011-5204-2
Lu, Y., Lerner, M. B., Qi, Z. J., Mitala, J. J., Lim, J. H., Discher, B. M., et al. (2012). Graphene-protein bioelectronic devices with wavelength-dependent photoresponse. Appl. Phys. Lett. 100:3. doi: 10.1063/1.3678024
Mak, K. F., Ju, L., Wang, F., and Heinz, T. F. (2012). Optical spectroscopy of graphene: from the far infrared to the ultraviolet. Solid State Commun. 152, 1341–1349. doi: 10.1016/j.ssc.2012.04.064
Masuda, S. (2013). Light detection and signal transduction in the BLUF photoreceptors. Plant Cell Physiol. 54, 171–179. doi: 10.1093/pcp/pcs173
Masuda, S., Hasegawa, K., and Ono, T. (2005). Light-induced structural changes of apoprotein and chromophore in the sensor of blue light using FAD (BLUF) domain of AppA for a signaling state. Biochemistry 44, 1215–1224. doi: 10.1021/bi047876t
Mathes, T., van Stokkum, I. H. M., Stierl, M., and Kennis, J. T. M. (2012). Redox modulation of flavin and tyrosine determines photoinduced proton-coupled electron transfer and photoactivation of BLUF photoreceptors. J. Biol. Chem. 287, 31725–31738. doi: 10.1074/jbc.M112.391896
Mueller, T., Xia, F., and Avouris, P. (2010). Graphene photodetectors for high-speed optical communications. Nat. Photon. 4, 297–301. doi: 10.1038/nphoton.2010.40
Nabiev, I., Rakovich, A., Sukhanova, A., Lukashev, E., Zagidullin, V., Pachenko, V., et al. (2010). Fluorescent quantum dots as artificial antennas for enhanced light harvesting and energy transfer to photosynthetic reaction centers. Angew. Chem. Int. Edition 49, 7217–7221. doi: 10.1002/anie.201003067
Nair, R. R., Blake, P., Grigorenko, A. N., Novoselov, K. S., Booth, T. J., Stauber, T., et al. (2008). Fine structure constant defines visual transparency of graphene. Science 320, 1308–1308. doi: 10.1126/science.1156965
Park, J., Ahn, Y. H., and Ruiz-Vargas, C. (2009). Imaging of photocurrent generation and collection in single-layer graphene. Nano Lett. 9, 1742–1746. doi: 10.1021/nl8029493
Suvarnaphaet, P., and Pechprasarn, S. (2017). Graphene-based materials for biosensors: a review. Sensors 17:2161. doi: 10.3390/s17102161
Tong, J., Zhang, P., Zhang, L., Zhang, D., Beratan, D. N., Song, H., et al. (2020). A robust bioderived wavelength-specific photosensor based on BLUF proteins. Sens. Actuat. B Chem. 310:127838. doi: 10.1016/j.snb.2020.127838
Wang, F., Zhang, Y. B., Tian, C. S., Girit, C., Zettl, A., Crommie, M., et al. (2008). Gate-variable optical transitions in graphene. Science 320, 206–209. doi: 10.1126/science.1152793
Keywords: photoreceptor protein, graphene, modification, cross-linking, photodetection, biosensor
Citation: Tong J, Zhang L, Wang Y and Li T (2020) High Response Photodetection by Applying the Optimized Photoreceptor Protein Modification on Graphene Based Field Effect Transistors. Front. Mater. 7:222. doi: 10.3389/fmats.2020.00222
Received: 10 April 2020; Accepted: 18 June 2020;
Published: 10 July 2020.
Edited by:
Yifan Yao, Université de Strasbourg, FranceReviewed by:
Jialiang Wang, University of Wisconsin–Madison, United StatesGang Zhao, University of Jinan, China
Copyright © 2020 Tong, Zhang, Wang and Li. This is an open-access article distributed under the terms of the Creative Commons Attribution License (CC BY). The use, distribution or reproduction in other forums is permitted, provided the original author(s) and the copyright owner(s) are credited and that the original publication in this journal is cited, in accordance with accepted academic practice. No use, distribution or reproduction is permitted which does not comply with these terms.
*Correspondence: Yi Wang, wangy@serene-t.com; Tie Li, tli@mail.sim.ac.cn