- 1National Joint Engineering Research Center for Abrasion Control and Molding of Metal Materials, Collaborative Innovation Center of Non-ferrous Metals of Henan Province, Henan Key Laboratory of Non-ferrous Materials Science & Processing Technology, School of Materials Science and Engineering, Henan University of Science and Technology, Luoyang, China
- 2Henan Key Laboratory of High-Temperature Structural and Functional Materials, Henan University of Science and Technology, Luoyang, China
- 3School of Materials Science and Energy Engineering, Foshan University, Foshan, China
- 4Key Laboratory of Function-oriented Porous Materials, College of Chemistry and Chemical Engineering, Luoyang Normal University, Luoyang, China
Environmental pollution and energy shortage make the development of clean energy more and more urgent. As a kind of clean renewable energy, hydrogen has attracted more attention recently. WO3-based materials have emerged as one of the most promising candidates for electrocatalytic hydrogen evolution reaction (HER) due to their attractive electrocatalytic activity, low cost, as well as electrochemical durability. In this minireview, we systematically provide an overview of WO3-based materials applied for HER, including pure WO3, doped WO3, and WO3-based composite materials. Furthermore, the strategies to enhance their electrocatalytic performance are summarized and discussed, such as morphological engineering, doping, as well as compositing with other materials. Finally, the limitation and challenges of WO3-based materials for HER and their prospects for future research are proposed. We believe that this minireview will be favorable for scientists to seek more promising HER electrocatalysts.
Introduction
Nowadays, the discovery and use of fossil fuels (such as coal and petroleum) have made great contributions to the development of human society (Wang Y. et al., 2018; Hao et al., 2019; Liu G. L. et al., 2020; Ma et al., 2020; Yu et al., 2020). However, the fast development of human society brought excessive emission of carbon dioxides and overuse of non-renewable resources, resulting in many serious problems, such as global warming, climate change, sharp decline in energy reserves, and so on (Liu G. et al., 2019; Zhao et al., 2019; Wang W. et al., 2020; Zou et al., 2020). Recently, a series of renewable energy resources such as wind energy, solar energy, tidal energy, and hydropower have been intensively studied and emerged as alternatives for fossil fuels (Peng et al., 2017; Liu G. et al., 2018). Meanwhile, electrochemical energy store (Guo et al., 2019; Wang F. et al., 2018; Wang et al., 2019e; Wang R. et al., 2020; Liu Y. et al., 2019; Yuan et al., 2019; Li M. et al., 2020; Song et al., 2020; Sui et al., 2020), electrocatalysis (Chen et al., 2019a; Li Y. et al., 2019; Wang et al., 2019c, Wang et al., 2019d; Xiao et al., 2019), and other new energy technologies have also developed rapidly in recent years (Ma et al., 2013, 2017; Zhang et al., 2014, 2018; Cheng et al., 2019; Li Y. et al., 2019; Zheng et al., 2020). It is worth noting that hydrogen fuel is of great concern because of its high energy density and abundant natural resources. Moreover, the product of hydrogen combustion is water, which is pollution-free and can effectively reduce the emission of greenhouse gases and toxic gases (Yu et al., 2019).
At present, the production of hydrogen by electrochemical water splitting has caught extensive attention because of its simple and flexible operation (Zou and Zhang, 2015). However, hydrogen production is hindered by the high overpotential of the hydrogen evolution reaction (HER) and the low hydrogen production rate (Lei et al., 2020). Therefore, in order to overcome these defects and promote the production of H2, it is urgent to introduce efficient electrocatalysts. Platinum is acknowledgedly regarded as one of the best electrocatalysts for HER (Ma et al., 2019); nevertheless, further application is limited by its high price. Therefore, it is necessary to explore proper non-noble metals for electrocatalytic hydrogen evolution.
As a transition metal oxide, tungsten oxide (WO3) is expected to be a hopeful candidate to substitute Pt as an electrocatalyst for efficient HER due to its outstanding redox capability, low cost, and high stability (Chandrasekaran et al., 2019; Wang et al., 2019b). Hence, more and more attention has been paid to investigating WO3-based materials for electrocatalytic HER. For example, Chen Y. et al. (2018) reported an article about crumpled graphene/tungsten disulfide/tungsten trioxide with high electrocatalytic HER performance. And recently, Tian et al. (2019) reported unique highly dispersed Pt atom clusters on WO3@CFC (carbon fiber cloth) as superior electrocatalysts for HER. Furthermore, Huang et al. (2019) gave a comprehensive review on recent progress in WO3-based materials as photoanodes for water oxidation. However, to the best of our knowledge, a critical review that exclusively puts a spotlight on WO3-based materials for electrocatalytic HER has not been reported.
In this minireview, we will give a comprehensive description of WO3-based materials, mainly including stoichiometric WO3, non-stoichiometric WO3–x, doped WO3, and WO3-based composite materials with their application in the field of electrocatalytic HER in recent years. Their micro/nanostructures and electrocatalytic performances for HER are systematically summarized, as shown in Supplementary Table S1. Furthermore, we also present some rational proposals to facilitate breakthroughs in the future. We hope that this minireview could draw more attention to WO3-based electrocatalysts and boost their practical applications.
Nanostructured Tungsten Oxide
Stoichiometric WO3
In recent years, the electrocatalytic performances of WO3 have been greatly enhanced by utilizing nano/micrometer-sized WO3, mainly due to their high surface area to volume ratio. The nanostructured stoichiometric WO3 with different morphologies as electrocatalysts for HER includes nanorods (Ham et al., 2010), nanowires (NWs) (Phuruangrat et al., 2010), nanoplates (Hu et al., 2015; Nayak et al., 2017), nanoparticles (Ganesan and Gedanken, 2008), and so on.
For example, Ham et al. (2010) developed a facile hydrothermal method to prepare monoclinic WO3 (m-WO3) nanoplates and nanorods (Supplementary Figures S1A,B). The cyclic voltammetry results show the decent stability of m-WO3 nanoplates and nanorods for HER (Supplementary Figures S1C,D). When they are tested at −0.2 V in 1 M H2SO4, m-WO3 nanoplates and nanorods shows cathodic current densities of 17.58 and 23.86 mA cm–2, respectively (Supplementary Figure S1E), and the Tafel slopes of m-WO3 nanoplates (122 mV dec–1) or nanorods (113 mV dec–1) are lower than those of commercial bulk m-WO3 (135 mV dec–1), indicating superior performances for HER (Supplementary Figure S1F). Phuruangrat et al. (2010) employed a novel microwave-assisted hydrothermal (MH) method to fabricate 1D hexagonal WO3 NWs (hex-WO3). The as-synthesized hex-WO3 samples display different morphology and sizes when the MH time was adjusted from 3 to 12 h (Supplementary Figures S2A–D). The specific activity for HER of hex-WO3 NWs fabricated by MH at −0.1 V is far higher than that of hex-WO3 NWs fabricated by conventional hydrothermal method (CH) and commercial WO3 (Supplementary Figure S2E). Furthermore, the Tafel slope and the exchange current density of hex-WO3 NWs by MH are 116 mV dec–1 and 6.61 mA cm–2, respectively, elucidating better electrocatalytic kinetics of hex-WO3 than those of commercial WO3 (157 mV dec–1 and 0.27 mA cm–2) (Supplementary Figure S2F). The performance enhancement of hex-WO3 NW samples can be attributed to their high aspect ratio and crystallinity.
Non-stoichiometric WOx<3
The performances of tungsten oxide can also be adjusted and controlled by defects in its architecture (Royer et al., 2014; Zheng et al., 2017). Several oxygen-deficient WOx<3 nanomaterials have been reported to promote the electrocatalytic HER efficiencies, such as mesoporous WO2.83 (Cheng et al., 2018), WO3–x/Ni foam (NF) (Yi et al., 2018), and monoclinic WO3–x (Sharma et al., 2019). For instance, Zheng et al. (2017) synthesized two-dimensional WO3 nanosheets with rich O vacancies via a liquid exfoliation method. The structure of the O vacancies model was built based on WO3 (010) (√2 × √2) R45° slab with the surface of all terminal oxygen atoms as well as one bridging oxygen atom removed (Figure 1A). At 10 mA cm–2, the overpotential of as-synthesized sample is 38 mV and the Tafel slope is also 38 mV dec–1, which is close to the optimal performances of benchmarking electrochemical catalyst Pt/C (Figure 1B). Unique O vacancies were verified by density-functional-theory (DFT) calculation on WO3 to bring in gap states around the Fermi level, which obviously increased hydrogen absorption and reduced H2 adsorption free energy (ΔGH*) (Figure 1C).
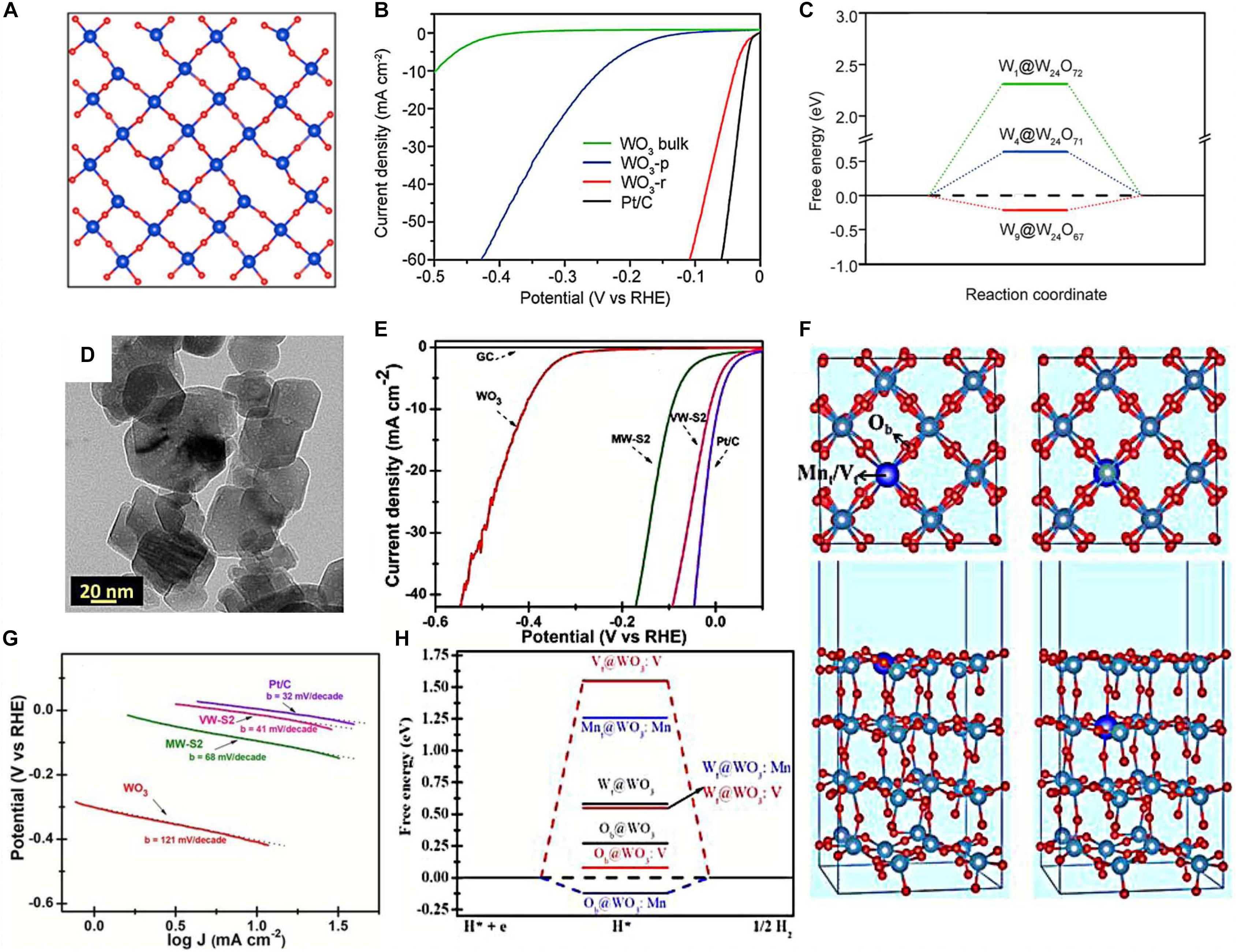
Figure 1. (A) Crystal structure of WO3 (010) (√2 × √2) R45° slab with all terminal O atoms as well as one bridging O atom removed (denoted as W24O67). (B) Polarization curves measured in H2 saturated 0.5 M H2SO4 solution for WO3-r NSs. (C) Free energy illustration for H2 adsorption at the W site on the WO3 (010) slab with different O vacancies. Reprinted with permission from Zheng et al. (2017). Copyright 2017, American Chemical Society. (D) Transmission electron microscope (TEM) image of VW-S2 sample. (E) Polarization curves of different samples. (F) Top (upper) and side (lower) view of Mn/V-doped WO3 (001) surfaces with a dopant in the surface and subsurface layer, including typical adsorption sites on systems (gray—W, blue—Mn/V, and red—O atoms). (G) Tafel slope of VW-S2. (H) Free energy illustration of H adsorption at a series of sites on V-doped WO3 surfaces. Reprinted with permission from Chandrasekaran et al. (2019). Copyright 2019, The Royal Society of Chemistry.
Hetero-atom Doped WO3
Besides the method of introducing O vacancies in WO3, hetero-atom doping with a metallic element is also an efficient way to promote electron transfer and shorten proton diffusion paths.
In 2014, Xie et al. (2014) reported nanostructured Ta-doped WO3 as an efficient electrocatalyst for HER. The as-prepared Ta-doped WO3 NWs show excellent performance. Later, metallic element doping in WO3 for electrocatalytic HER was further developed, and the mechanism was demonstrated by Chandrasekaran et al. (2019). They prepared Mn-doped WO3 and V-doped WO3 via the hydrothermal route, and the particle sizes are 50–70 and 20–30 nm, respectively (Figure 1D). The electrochemical results indicate that the V-doped WO3 (3 wt.% denoted as VW-S2) exhibits the optimal performance for HER. The overpotential of VW-S2 at 10 mA cm–2 is 38 mV versus the reversible hydrogen electrode (RHE) (Figure 1E). Moreover, the Tafel slope is 41 mV per decade, which is lower than that of Mn-doped WO3 (MW-S2) (68 mV dec–1) and undoped WO3 (121 mV dec–1), close to the benchmarking Pt/C (32 mV dec–1) (Figure 1G). Additionally, the value of free energy ΔGH* under the circumstances shown in Figure 1F is ideal (0.08 eV at Ob sites), which gains significant reduction (Figure 1H). DFT calculation indicates that band gap reduction in WO3 is on account of V doping, resulting in great conductivity, and the high HER activity could be ascribed to the V element bonding with terminal oxygen in the lattice of WO3.
WO3-Based Binary Composites
To further enhance electrocatalytic properties for HER, WO3 has been composited with other materials, such as carbon (Wondimu et al., 2018a, b), metals (Li W. et al., 2019; Tian et al., 2019), and metal-based compounds (Yang et al., 2016; Shang et al., 2017).
WO3/Carbon Composites
Carbon materials are often utilized as conductive materials due to their superior electronic conductivity and outstanding chemical durability (Wu et al., 2019). Hence, compositing WO3 with carbon materials is an efficient way to improve the HER performances (Wondimu et al., 2018a, b; Hu et al., 2019). For example, Wondimu et al. (2018a) developed novel electrocatalyst tungsten oxide NWs with rich O vacancies supported by nitrogen doped-reduced graphene oxide (N-rGO) (denoted as WOxNWs/N-rGO), which exhibits better HER performance than Fe-WOxP/rGO approaching to Pt/C. The overpotential as well as the Tafel slope are only 40 mV at 10 mA cm–2 and 38.2 mV dec–1, respectively, which are close to the state-of-the-art Pt/C. The superior performance could be mainly put down to O vacancies and the network between the WOxNWs and N-rGO.
Not only could the rGO assist the WO3-based materials to be more excellent and efficient, but also other carbon materials could increase the conductivity and further enhance the electrocatalytic properties for HER, such as WO3/C (Zheng and Mathe, 2011), WO3/Carbon nanotube (CNT) (Chekin et al., 2013), and WO3–x@nitrogen-doped carbon (NC) (Chen J. et al., 2018). More details on the electrocatalytic performances could be found in Supplementary Table S1.
WO3/Metal-Based Material Composites
Besides carbon materials, construction of WO3 with metal and metal-based materials is regarded as another effective way to enhance the charge transfer and promote the synergistic effect between them (Wang et al., 2019a, b). Up to now, many metal-based materials have been composited with WO3, such as Pt (Li W. et al., 2019; Tian et al., 2019), Ag (Ma et al., 2019), WX2 (X = S) (Yang et al., 2016; Shang et al., 2017), Se (Fominski et al., 2016), and Al2O3 (Zou et al., 2019). For instance, Yang et al. (2016) in situ synthesized WO3⋅2H2O/WS2 hybrid electrocatalyst for HER with superior electrocatalytic properties through a facile anodizing treatment. Furthermore, to minimize the use of precious metals, Tian et al. (2019) fabricated an effective low-Pt electrocatalyst with high oxygen vacancies in WO3@CFC (carbon fiber cloth) denoted as Pt/def-WO3@CFC. Firstly, the WO3 nanoplates were grown on carbon fiber cloth through a hydrothermal method, followed by heat treatment under the atmosphere of 5 vol.% H2 and 95 vol.% Ar to produce O vacancies on WO3. And the Pt/def-WO3@CFC was obtained after Pt nanoclusters dispersed on the defect of WO3 via thermal reduction (Figure 2A). The lattice fringe spacing are 0.374 and 0.365 nm, corresponding to the (002) and (020) planes of WO3, respectively (Figure 2B). And the spherical aberration-corrected high-angle annular dark-field scanning transmission electron microscopy (HAADF-STEM) image shows that the number of O vacancies is relatively low (Figure 2C). But after the loading of Pt, an obvious decrease in intensity is seen with a reduction in the electron spin resonance (ESR) peak, indicating the substitution of O vacancies by Pt clusters. The HER performance shows a Tafel slope of 61 mV dec–1 and an overpotential of 42 mV at 10 mA cm–2 in 0.5 M H2SO4 (Figure 2D), which are comparable to those of the state-of-the-art commercial Pt/C catalyst (η10 = 34 mV, Tafel slope = 46 mV dec–1). And it is worthy to mention that the activity of low-Pt catalyst Pt/def-WO3@CFC is 3.3 times that of commercial Pt/C, which could be attributed to the synergistic effect between the Pt and defective WO3 (Figure 2D). Pt atoms are tightly tied in the position of O vacancies, hindering their aggregation or growing during the period of hydrogen evolution, which definitely reveals the significant functions of defect-rich nanostructures (Chen et al., 2019b; Zhu et al., 2019).
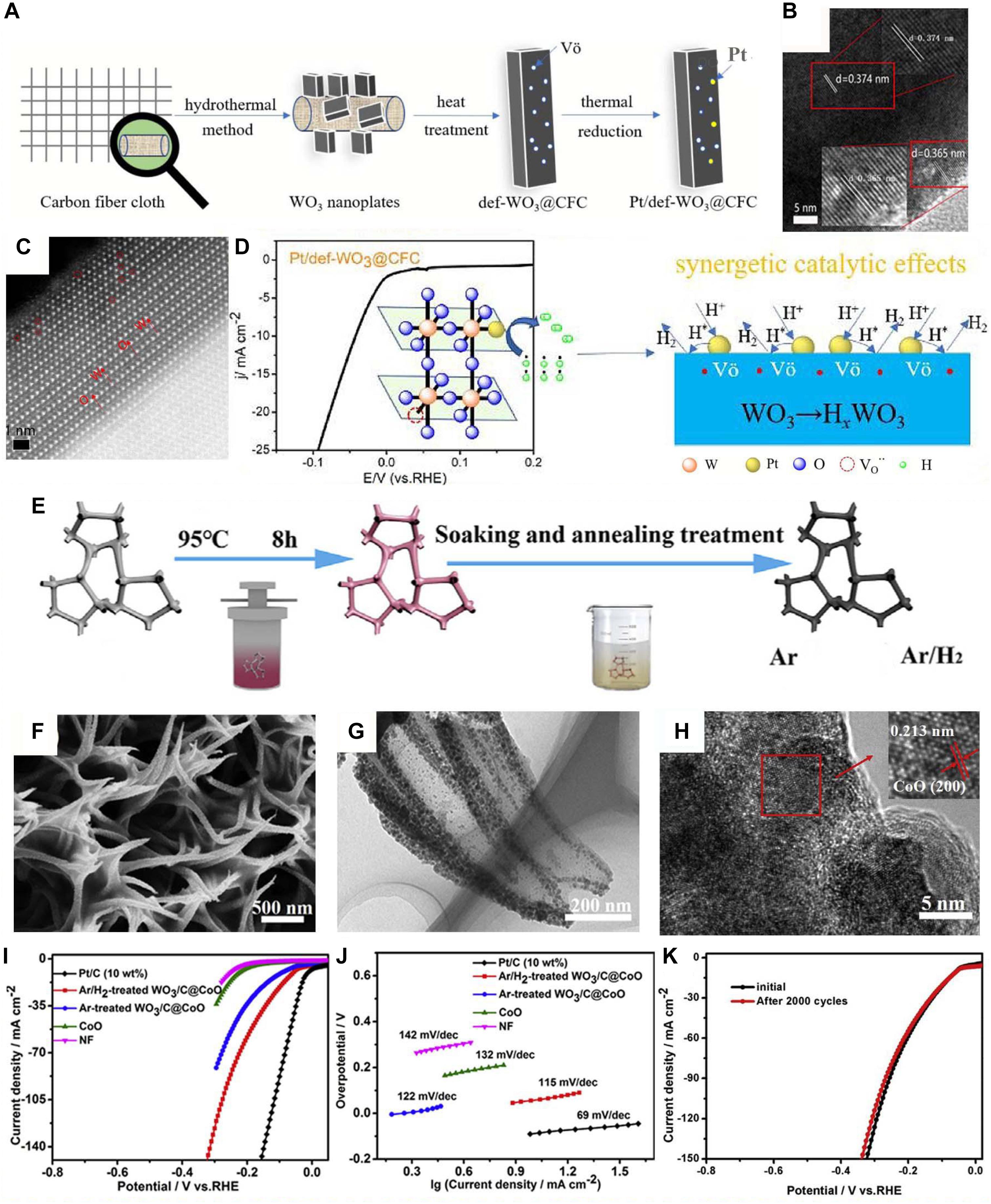
Figure 2. (A) Scheme diagram for the synthetic process of Pt/def-WO3@CFC. (B) High-resolution transmission electron microscope (HRTEM) of WO3 NPs. (C) Spherical aberration-corrected image of Pt/def-WO3@CFC at atomic scale. (D) Linear sweep voltammetry (LSV) curves in 0.5 M H2SO4 at a scan rate of 1 mV/s and the schematic model for Pt/WO3 nanostructure with O vacancies. Reprinted with permission from Tian et al. (2019). Copyright 2019, The Royal Society of Chemistry. (E) Scheme diagram of the formation of WO3/C@CoO/NF. (F) Scanning electron microscope (SEM), (G) TEM, and (H) HRTEM images of Ar/H2-treated WO3/C@CoO/NF. (I) The LSV curves and (J) the Tafel slopes of different samples. (K) The LSV curves of Ar/H2-treated WO3/C@CoO/NF before and after 2,000 cycles. Reprinted with permission from Lv et al. (2018a). Copyright 2018, Elsevier.
Others
Besides the above mentioned, there are still some WO3-based composite electrocatalysts with enhanced HER performance. The electrocatalyst SiO2/WO3–x nanofacets calcined in situ at 500°C for 5 h (denote as 500-5) by Ketpang et al. (2013) exhibits an overpotential of 170 mV owing to the interaction between them. And nano-zeolite with 3 wt.% WO3 prepared via the work of Anis and Hashaikeh (2018) is also a promising candidate for HER with a lower Tafel slope of 31.9 mV dec–1 due to the high surface areas and decent durability brought by zeolites. However, their specific mechanisms need deep insight on the assistance of DFT calculation.
WO3-Based Ternary Composites
Recently, WO3-based ternary composite electrocatalysts for HER receive more and more attention due to the great synergy effect between the components (Choudhary et al., 2017; Chen Y. et al., 2018). For instance, Lv et al. (2018a) synthesized WO3/C@CoO on NF observed as octopus tentacles via facile hydrothermal treatment as well as following thermal treatment. As shown in Figure 2E, a CoO NWs precursor grows on NF after hydrothermal treatment. The SEM and TEM images (Figures 2F–H) showed the morphology of the WO3/C@CoO nanostructure. When it is measured as an electrocatalyst for HER, the overpotential at 10 mA cm–2 is tested as 55 mV (Figure 2I). The Tafel slope is 115 mV–dec–1 (Figure 2J), which is smaller than that of argon-treated WO3/C@CoO/NF (122 mV dec–1) and CoO NWs (132 mV dec–1). And the outstanding HER stability is still maintained after 2,000 cycles (Figure 2K). The excellent electrocatalytic performance is mainly due to the distinctive structure and robust synergistic effect among WO3, CoO, and NF. Another ternary CoSe2/WSe2/WO3 hybrid NWs on carbon cloth (CC) were synthesized by Lv et al. (2018b). The high performance indicates the significant functions of unique nanostructure and strong synergistic effects, which prompts Ar/H2-treated WO3/C@CoO/NF and CoSe2/WSe2/WO3 NWs/CC to become remarkable electrocatalysts for HER (Du et al., 2018).
Conclusion and Outlooks
In summary, we briefly review the recent developments of WO3-based materials for electrocatalytic HER. The essential synthetic methods and processes, various nanostructures, and robust performances are generally discussed. From these studies, it is clear that the strategies mentioned above are useful and meaningful for optimizing the electrocatalytic performance of WO3. However, we should recognize that the practical application of WO3-based electrocatalysts for HER is still in early stage and is facing many challenges. First, the majority of works are focusing on the nanostructure morphology and electrocatalytic performances, but the understanding of mechanism is not seriously taken. Second, the latest modern characterization techniques, such as in situ X-ray technique, in situ electron microscopy, and in situ scanning probe technology, should be well used to explore the working mechanisms. Third, DFT calculation is also an efficient way for us to gain insight into the nanostructure–composition–performance relationships, which may be important in the development of catalysts in the future. In addition, among these recent advances, the electrocatalysts introduced O vacancies that almost exhibit outstanding performances close to those of the benchmarking Pt/C. Decent electrical conductivity, appropriate Gibbs free energy ΔGH*, and large active surface areas will possibly render the introduction of O vacancies as a promising strategy and the orientation for better HER process. Last, the electrocatalytic stability should also be seriously considered. We hope this minireview will be helpful and will inspire more innovative ideas for WO3-based or even transition metal oxide materials as electrocatalysts for HER.
Author Contributions
YL, WZ, and SW conceived the idea. YXL and XZ wrote the draft. All authors contributed to the writing, discussion, and revision of the final version of the manuscript.
Funding
This work was supported by Nature Science Foundation of Guangdong Province (No. 2018A030313779). This work was also supported by the Program for Changjiang Scholars and Innovative Research Team in University (IRT_16R21), the Chinese 02 Special Fund (2017ZX02408003), the Scientific and Technological Project of Henan Province (182102210297), Open Fund of National Joint Engineering Research Center for abrasion control and molding of metal materials (HKDNM201807), Scientific Research Starting Foundation for Ph.D. of Henan University of Science and Technology (13480065), Science Foundation for Youths of Henan University of Science and Technology (2013QN006), and the Student Research Training Plan of Henan University of Science and Technology (2019031).
Conflict of Interest
The authors declare that the research was conducted in the absence of any commercial or financial relationships that could be construed as a potential conflict of interest.
Supplementary Material
The Supplementary Material for this article can be found online at: https://www.frontiersin.org/articles/10.3389/fmats.2020.00105/full#supplementary-material
References
Anis, S. F., and Hashaikeh, R. (2018). Electrochemical water splitting using nano-zeolite Y supported tungsten oxide electrocatalysts. J. Nanopart. Res. 20:47. doi: 10.1007/s11051-018-4153-2
Chandrasekaran, S., Zhang, P., Peng, F., Bowen, C., Huo, J., and Deng, L. (2019). Tailoring the geometric and electronic structure of tungsten oxide with manganese or vanadium doping toward highly efficient electrochemical and photoelectrochemical water splitting. J. Mater. Chem. A 7, 6161–6172. doi: 10.1039/c8ta12238e
Chekin, F., Bagheri, S., and Abd Hamid, S. B. (2013). Synthesis of tungsten oxide nanorods by the controlling precipitation reaction: application for hydrogen evolution reaction on a wo3 nanorods/carbon nanotubes composite film modified electrode. J. Chin. Chem. Soc. 60, 447–451. doi: 10.1002/jccs.201200298
Chen, H., Liu, J., Liu, P., Wang, Y., Xiao, H., Yang, Q., et al. (2019a). Carbon-confined magnesium hydride nano-lamellae for catalytic hydrogenation of carbon dioxide to lower olefins. J. Catal. 379, 121–128. doi: 10.1016/j.jcat.2019.09.022
Chen, H., Liu, P., Li, J., Wang, Y., She, C., Liu, J., et al. (2019b). MgH2/CuxO Hydrogen Storage Composite with Defect-Rich Surfaces for Carbon Dioxide Hydrogenation. ACS Appl. Mater. Interf. 11, 31009–31017. doi: 10.1021/acsami.9b11285
Chen, J., Yang, H., Sang, X., Su, Z., Li, D., and Wang, Q. (2018). Oxygen vacancy rich tungsten oxide with nitrogen doped mesoporous carbon as matrix for overall water splitting and 4-nitrophenol reductive removal. Solid State Sci. 83, 23–30. doi: 10.1016/j.solidstatesciences.2018.06.010
Chen, Y., Ren, R., Wen, Z., Ci, S., Chang, J., Mao, S., et al. (2018). Superior electrocatalysis for hydrogen evolution with crumpled graphene/tungstendisulfide/tungsten trioxide ternary nanohybrids. Nano Energy 47, 66–73. doi: 10.1016/j.nanoen.2018.02.023
Cheng, H., Klapproth, M., Sagaltchik, A., Li, S., and Thomas, A. (2018). Ordered mesoporous WO2.83: selective reduction synthesis, exceptional localized surface plasmon resonance and enhanced hydrogen evolution reaction activity. J. Mater. Chem. A 6, 2249–2256. doi: 10.1039/c7ta09579a
Cheng, N., Zhao, J., Fan, L., Liu, Z., Chen, S., Ding, H., et al. (2019). Sb-MOFs derived Sb nanoparticles@porous carbon for high performance potassium-ion batteries anode. Chem. Commun. 55, 12511–12514. doi: 10.1039/c9cc06561j
Choudhary, R., Patra, S., Madhuri, R., and Sharma, P. K. (2017). Cow Dung Derived PdNPs@WO3 Porous Carbon Nanodiscs as Trifunctional Catalysts for Design of Zinc-Air batteries and overall water splitting. ACS Sustain. Chem. Eng. 5, 9735–9748. doi: 10.1021/acssuschemeng.7b01541
Du, H., Kong, R.-M., Guo, X., Qu, F., and Li, J. (2018). Recent progress in transition metal phosphides with enhanced electrocatalysis for hydrogen evolution. Nanoscale 10, 21617–21624. doi: 10.1039/c8nr07891b
Fominski, V. Y., Grigoriev, S. N., Romanov, R. I., Volosova, M. A., Grunin, A. I., and Teterina, G. D. (2016). The formation of a hybrid structure from tungsten selenide and oxide plates for a hydrogen-evolution electrocatalyst. Technic. Phys. Lett. 42, 555–558. doi: 10.1134/s1063785016060055
Ganesan, R., and Gedanken, A. (2008). Synthesis of WO(3) nanoparticles using a biopolymer as a template for electrocatalytic hydrogen evolution. Nanotechnology 19, 025702–025702. doi: 10.1088/0957-4484/19/02/025702
Guo, X., Zhang, Y.-Z., Zhang, F., Li, Q., Anjum, D. H., Liang, H., et al. (2019). A novel strategy for the synthesis of highly stable ternary SiOx composites for Li-ion-battery anodes. J. Mater. Chem. A 7, 15969–15974. doi: 10.1039/c9ta04062e
Ham, D. J., Phuruangrat, A., Thongtem, S., and Lee, J. S. (2010). Hydrothermal synthesis of monoclinic WO3 nanoplates and nanorods used as an electrocatalyst for hydrogen evolution reactions from water. Chem. Eng. J. 165, 365–369. doi: 10.1016/j.cej.2010.09.00
Hao, X., Zhao, Q., Su, S., Zhang, S., Ma, J., Shen, L., et al. (2019). Constructing multifunctional interphase between Li1.4Al0.4Ti1.6(PO4)(3) and Li Metal by magnetron sputtering for highly stable solid-state lithium metal batteries. Adv. Energy Mater. 9:1901604. doi: 10.1002/aenm.201901604
Hu, G., Li, J., Liu, P., Zhu, X., Li, X., Ali, R. N., et al. (2019). Enhanced electrocatalytic activity of WO3@NPRGO composite in a hydrogen evolution reaction. Appl. Surf. Sci. 463, 275–282. doi: 10.1016/j.apsusc.2018.08.227
Hu, W.-H., Han, G.-Q., Dong, B., and Liu, C.-G. (2015). Facile Synthesis of Highly Dispersed WO3 center dot H2O and WO3 nanoplates for electrocatalytic hydrogen evolution. J. Nanomater. 2015:346086. doi: 10.1155/2015/346086
Huang, J., Yue, P., Wang, L., She, H., and Wang, Q. (2019). A review on tungsten-trioxide-based photoanodes for water oxidation. Chin. J. Catalysis 40, 1408–1420. doi: 10.1016/S1872-2067(19)63399-1
Ketpang, K., Kim, M., Kim, S., and Shanmugam, S. (2013). High performance catalyst for electrochemical hydrogen evolution reaction based on SiO2/WO3-x nanofacets. Int. J. Hydrogen Energy 38, 9732–9740. doi: 10.1016/j.ijhydene.2013.05.112
Lei, Y., Wang, Y., Liu, Y., Song, C., Li, Q., Wang, D., et al. (2020). Realizing the atomic active center for hydrogen evolution electrocatalysts. Angewandte Chem. doi: 10.1002/anie.201914647 [Epub ahead of print].
Li, M., Feng, W., and Wang, X. (2020). Complex hollow structures of Cobalt(II) sulfide as a cathode for Lithium-Sulfur batteries. Int. J. Electrochem. Sci. 15, 526–534. doi: 10.20964/2020.01.77
Li, W., Hu, Z.-Y., Zhang, Z., Wei, P., Zhang, J., Pu, Z., et al. (2019). Nano-single crystal coalesced PtCu nanospheres as robust bifunctional catalyst for hydrogen evolution and oxygen reduction reactions. J. Catalysis 375, 164–170. doi: 10.1016/j.jcat.2019.05.031
Li, Y., Xu, Y., Liu, Y., and Pang, H. (2019). Exposing {001} Crystal Plane on Hexagonal Ni-MOF with Surface-Grown Cross-Linked Mesh-structures for electrochemical energy storage. Small 15, 7317–7321. doi: 10.1002/smll.201902463
Liu, G., Cui, J., Luo, R., Liu, Y., Huang, X., Wu, N., et al. (2019). 2D MoS2 grown on biomass-based hollow carbon fibers for energy storage. Appl. Surf. Sci. 469, 854–863. doi: 10.1016/j.apsusc.2018.11.067
Liu, Y., Wang, H., Yang, K., Yang, Y., Ma, J., Pan, K., et al. (2019). Enhanced electrochemical performance of Sb2O3 as an Anode for Lithium-Ion batteries by a stable cross-linked binder. Appl. Sci. Basel 9:2677. doi: 10.3390/app9132677
Liu, G., Li, M., Wu, N., Cui, L., Huang, X., Liu, X., et al. (2018). Single-crystalline particles: an effective way to Ameliorate the Intragranular cracking, thermal stability, and capacity fading of the LiNi0.6Co0.2Mn0.2O2 Electrodes. J. Electrochem. Soc. 165, A3040–A3047. doi: 10.1149/2.0491813jes
Liu, G. L., Wu, H. H., Meng, Q. Q., Zhang, T., Sun, D., Jin, X. Y., et al. (2020). Role of the anatase/TiO2(B) heterointerface for ultrastable high-rate lithium and sodium energy storage performance. Nanoscale Horizons 5, 150–162. doi: 10.1039/c9nh00402e
Lv, Y., Liu, Y., Chen, C., Wang, T., and Zhang, M. (2018a). Octopus tentacles-like WO3/C@CoO as high property and long life-time electrocatalyst for hydrogen evolution reaction. Electrochim. Acta 281, 1–8. doi: 10.1016/j.electacta.2018.05.145
Lv, Y., Liu, Y., Liu, Y., Chen, Z., and Zhang, M. (2018b). CoSe2/WSe2/WO3 hybrid nanowires on carbon cloth for efficient hydrogen evolution reaction. J. Alloys Compounds 768, 889–895. doi: 10.1016/j.jallcom.2018.07.285
Ma, J., Ma, Z., Liu, B., Wang, S., Ma, R., and Wang, C. (2019). Composition of Ag-WO3 core-shell nanostructures as efficient electrocatalysts for hydrogen evolution reaction. J. Solid State Chem. 271, 246–252. doi: 10.1016/j.jssc.2018.12.020
Ma, J., Ren, F., Wang, G., Xiong, Y., Li, Y., and Wen, J. (2017). Electrochemical performance of melt-spinning Al-Mg-Sn based anode alloys. Int. J. Hydrogen Energy 42, 11654–11661. doi: 10.1016/j.ijhydene.2017.02.185
Ma, J., Wen, J., Li, Q., and Zhang, Q. (2013). Electrochemical polarization and corrosion behavior of Al-Zn-In based alloy in acidity and alkalinity solutions. Int. J. Hydrogen Energy 38, 14896–14902. doi: 10.1016/j.ijhydene.2013.09.046
Ma, J. L., Zhang, Y., Qin, C. H., Ren, F. Z., and Wang, G. X. (2020). Effects of polystyrene sulfonate/graphene and Mn3O4/graphene on property of aluminum(zinc)-air batteries. Int. J. Hydrogen Energy 45, 13025–13034. doi: 10.1016/j.ijhydene.2020.02.222
Nayak, A. K., Verma, M., Sohn, Y., Deshpande, P. A., and Pradhan, D. (2017). Highly active Tungsten Oxide nanoplate electrocatalysts for the hydrogen evolution reaction in acidic and near neutral electrolytes. ACS Omega 2, 7039–7047. doi: 10.1021/acsomega.7b01151
Peng, T., Hou, X., Liu, C., Yu, Q., Luo, R., Yan, H., et al. (2017). Controlled synthesis of hierarchical CoMn2O4 nanostructures for flexible all-solid-state battery-type electrodes. J. Solid State Electrochem. 21, 1579–1587. doi: 10.1007/s10008-017-3516-4
Phuruangrat, A., Ham, D. J., Hong, S. J., Thongtem, S., and Lee, J. S. (2010). Synthesis of hexagonal WO3 nanowires by microwave-assisted hydrothermal method and their electrocatalytic activities for hydrogen evolution reaction. J. Mater. Chem. 20, 1683–1690. doi: 10.1039/b918783a
Royer, S., Duprez, D., Can, F., Courtois, X., Batiot-Dupeyrat, C., Laassiri, S., et al. (2014). Perovskites as substitutes of noble metals for heterogeneous catalysis: dream or reality. Chem. Rev. 114, 10292–10368. doi: 10.1021/cr500032a
Shang, X., Rao, Y., Lu, S.-S., Dong, B., Zhang, L.-M., Liu, X.-H., et al. (2017). Novel WS2/WO3 heterostructured nanosheets as efficient electrocatalyst for hydrogen evolution reaction. Mater. Chem. Phys. 197, 123–128. doi: 10.1016/j.matchemphys.2017.05.027
Sharma, L., Kumar, P., and Halder, A. (2019). Phase and vacancy modulation in tungsten oxide: electrochemical hydrogen evolution. Chemelectrochem 6, 3420–3428. doi: 10.1002/celc.201900666
Song, C., Feng, W., Wang, X., and Shi, Z. (2020). Enhanced electrochemical performance of Li1.2Mn0.54Ni0.13Co0.13O2 cathode material with bamboo essential oil. Ionics 26, 661–672. doi: 10.1007/s11581-019-03233-9
Sui, D., Xu, L., Zhang, H., Sun, Z., Kan, B., Ma, Y., et al. (2020). A 3D cross-linked graphene-based honeycomb carbon composite with excellent confinement effect of organic cathode material for lithium-ion batteries. Carbon 157, 656–662. doi: 10.1016/j.carbon.2019.10.106
Tian, H., Cui, X., Zeng, L., Su, L., Song, Y., and Shi, J. (2019). Oxygen vacancy-assisted hydrogen evolution reaction of the Pt/WO3 electrocatalyst. J. Mater. Chem. A 7, 6285–6293. doi: 10.1039/c8ta12219a
Wang, F., Liu, Y., Zhao, Y., Wang, Y., Wang, Z., Zhang, W., et al. (2018). Facile Synthesis of Two-Dimensional Porous MgCo2O4 Nanosheets as Anode for Lithium-Ion batteries. Appl. Sci. 8:22. doi: 10.3390/app8010022
Wang, Y., Wang, Z., Lei, D., Lv, W., Zhao, Q., Ni, B., et al. (2018). Spherical Li Deposited inside 3D Cu skeleton as anode with ultrastable performance. ACS Appl. Mater. Interf. 10, 20244–20249. doi: 10.1021/acsami.8b04881
Wang, G., Chen, Y., Chen, C., Kang, X., Yang, C., Wang, F., et al. (2019a). Self-stabilized and strong-Adhesive Supramolecular polymer protective layer enables ultrahigh-rate and large-capacity Lithium-Metal Anode. Angewandte Chemie 59, 2055–2060. doi: 10.1002/anie.201913351
Wang, G., Xiong, X., Zou, P., Fu, X., Lin, Z., Li, Y., et al. (2019b). Lithiated zinc oxide nanorod arrays on copper current collectors for robust Li metal anodes. Chem. Eng. J. 378:122243. doi: 10.1016/j.cej.2019.122243
Wang, J., Xiao, X., Liu, Y., Pan, K., Pang, H., and Wei, S. (2019c). The application of CeO2-based materials in electrocatalysis. J. Mater. Chem. A 7, 17675–17702. doi: 10.1039/c9ta04804a
Wang, P., Pu, Z., Li, W., Zhu, J., Zhang, C., Zhao, Y., et al. (2019d). Coupling NiSe2-Ni2P heterostructure nanowrinkles for highly efficient overall water splitting. J. Catalysis 377, 600–608. doi: 10.1016/j.jcat.2019.08.005
Wang, X., Yang, C., Xiong, X., Chen, G., Huang, M., Wang, J.-H., et al. (2019e). A robust sulfur host with dual lithium polysulfide immobilization mechanism for long cycle life and high capacity Li-S batteries. Energy Storage Mater. 16, 344–353. doi: 10.1016/j.ensm.2018.06.015
Wang, R., Cao, X., Zhao, D., Zhu, L., Xie, L., Liu, J., et al. (2020). Wet-chemistry synthesis of Li4Ti5O12 as anode materials rendering high-rate Li-ion storage. Int. J. Energy Res. 44, 4211–4223. doi: 10.1002/er.5020
Wang, W., Sun, K., and Liu, H. (2020). Effects of different aluminum sources on morphologies and properties of ceramic floor tiles from red mud. Construct. Build. Mater. 241:118119. doi: 10.1016/j.conbuildmat.2020.118119
Wondimu, T. H., Chen, G.-C., Chen, H.-Y., Kabtamu, D. M., Bayeh, A. W., Wang, K.-C., et al. (2018a). High catalytic activity of oxygen- vacancy- rich tungsten oxide nanowires supported by nitrogendoped reduced graphene oxide for the hydrogen evolution reaction. J. Mater. Chem. A 6, 19767–19774. doi: 10.1039/c8ta07000h
Wondimu, T. H., Chen, G.-C., Kabtamu, D. M., Chen, H.-Y., Bayeh, A. W., Huang, H.-C., et al. (2018b). Highly efficient and durable phosphine reduced iron-doped tungsten oxide/reduced graphene oxide nanocomposites for the hydrogen evolution reaction. Int. J. Hydrog. Energy 43, 6481–6490. doi: 10.1016/j.ijhydene.2018.02.080
Wu, N., Shen, J., Sun, L., Yuan, M., Shao, Y., Ma, J., et al. (2019). Hierarchical N-doped graphene coated 1D cobalt oxide microrods for robust and fast lithium storage at elevated temperature. Electrochim. Acta 310, 70–77. doi: 10.1016/j.electacta.2019.04.115
Xiao, X., Zhang, G., Xu, Y., Zhang, H., Guo, X., Liu, Y., et al. (2019). A new strategy for the controllable growth of MOF@PBA architectures. J. Mater. Chem. A 7, 17266–17271. doi: 10.1039/c9ta05409j
Xie, X., Mu, W., Li, X., Wei, H., Jian, Y., Yu, Q., et al. (2014). Incorporation of tantalum ions enhances the electrocatalytic activity of hexagonal WO3 nanowires for hydrogen evolution reaction. Electrochim. Acta 134, 201–208. doi: 10.1016/j.electacta.2014.04.122
Yang, L., Zhu, X., Xiong, S., Wu, X., Shan, Y., and Chu, P. K. (2016). Synergistic WO3 center dot 2H(2)O Nanoplates/WS2 Hybrid catalysts for high-efficiency hydrogen evolution. ACS Appl. Mater. Interf. 8, 13966–13972. doi: 10.1021/acsami.6b04045
Yi, L., Zhi, C., Yongkang, L., Taihong, W., and Zhang, M. (2018). Oxygen vacancy improves the hydrogen evolution reaction property of WO3-x nanosheets. Nano Struct. Nano Obj. 15, 114–118. doi: 10.1016/j.nanoso.2017.08.017
Yu, M., Yin, Z., Yan, G., Wang, Z., Guo, H., Li, G., et al. (2020). Synergy of interlayer expansion and capacitive contribution promoting sodium ion storage in S, N-Doped mesoporous carbon nanofiber. J. Power Sources 449:227514. doi: 10.1016/j.jpowsour.2019.227514
Yu, P., Wang, F., Shifa, T. A., Zhan, X., Lou, X., Xia, F., et al. (2019). Earth abundant materials beyond transition metal dichalcogenides: a focus on electrocatalyzing hydrogen evolution reaction. Nano Energy 58, 244–276. doi: 10.1016/j.nanoen.2019.01.017
Yuan, M., Guo, X., Liu, Y., and Pang, H. (2019). Si-based materials derived from biomass: synthesis and applications in electrochemical energy storage. J. Mater. Chem. A 7, 22123–22147. doi: 10.1039/c9ta06934h
Zhang, D., Yan, H., Lu, Y., Qiu, K., Wang, C., Zhang, Y., et al. (2014). NiCo2O4 nanostructure materials: morphology control and electrochemical energy storage. Dalton Trans. 43, 15887–15897. doi: 10.1039/c4dt02276a
Zhang, D., Zhang, Y., Luo, Y., Zhang, Y., Li, X., Yu, X., et al. (2018). High-performance asymmetrical supercapacitor composed of rGO-enveloped nickel phosphite hollow spheres and N/S co-doped rGO aerogel. Nano Res. 11, 1651–1663. doi: 10.1007/s12274-017-1780-3
Zhao, Q., Hao, X., Su, S., Ma, J., Hu, Y., Liu, Y., et al. (2019). Expanded-graphite embedded in lithium metal as dendrite-free anode of lithium metal batteries. J. Mater. Chem. A 7, 15871–15879. doi: 10.1039/c9ta04240g
Zheng, H., and Mathe, M. (2011). Hydrogen evolution reaction on single crystal WO(3)/C nanoparticles supported on carbon in acid and alkaline solution. Int. J. Hydrogen Energy 36, 1960–1964. doi: 10.1016/j.ijhydene.2010.11.052
Zheng, T., Sang, W., He, Z., Wei, Q., Chen, B., Li, H., et al. (2017). Conductive Tungsten oxide nanosheets for highly efficient hydrogen evolution. Nano Lett. 17, 7968–7973. doi: 10.1021/acs.nanolett.7b04430
Zheng, Z., Li, P., Huang, J., Liu, H., Zao, Y., Hu, Z., et al. (2020). High performance columnar-like Fe2O3@carbon composite anode via yolk@shell structural design. J. Energy Chem. 41, 126–134. doi: 10.1016/j.jechem.2019.05.009
Zhu, Y., Zhang, Z., Li, W., Lei, Z., Cheng, N., Tan, Y., et al. (2019). Highly exposed active sites of defect-Enriched Derived MOFs for Enhanced Oxygen reduction reaction. ACS Sustain. Chem. Eng. 7, 17855–17862. doi: 10.1021/acssuschemeng.9b04380
Zou, P., Lin, Z., Fan, M., Wang, F., Liu, Y., and Xiong, X. (2020). Facile and efficient fabrication of Li3PO4-coated Ni-rich cathode for high-performance lithium-ion battery. Appl. Surf. Sci. 504:144506. doi: 10.1016/j.apsusc.2019.144506
Zou, X., and Zhang, Y. (2015). Noble metal-free hydrogen evolution catalysts for water splitting. Chem. Soc. Rev. 44, 5148–5180. doi: 10.1039/c4cs00448e
Keywords: tungsten oxide, doping, composites, electrocatalyst, hydrogen evolution reaction
Citation: Li Y, Zhai X, Liu Y, Wei H, Ma J, Chen M, Liu X, Zhang W, Wang G, Ren F and Wei S (2020) WO3-Based Materials as Electrocatalysts for Hydrogen Evolution Reaction. Front. Mater. 7:105. doi: 10.3389/fmats.2020.00105
Received: 10 March 2020; Accepted: 06 April 2020;
Published: 15 May 2020.
Edited by:
Liang Huang, Huazhong University of Science and Technology, ChinaReviewed by:
Qiaobao Zhang, Xiamen University, ChinaXunhui Xiong, South China University of Technology, China
Copyright © 2020 Li, Zhai, Liu, Wei, Ma, Chen, Liu, Zhang, Wang, Ren and Wei. This is an open-access article distributed under the terms of the Creative Commons Attribution License (CC BY). The use, distribution or reproduction in other forums is permitted, provided the original author(s) and the copyright owner(s) are credited and that the original publication in this journal is cited, in accordance with accepted academic practice. No use, distribution or reproduction is permitted which does not comply with these terms.
*Correspondence: Yong Liu, bGl1eW9uZzIwOUBoYXVzdC5lZHUuY24=; Wanhong Zhang, emhhbmd3aEBoYXVzdC5lZHUuY24=; Shizhong Wei, d3N6QGhhdXN0LmVkdS5jbg==
†These authors have contributed equally to this work